- 1Program of Cellular and Molecular Biology, Institute of Biomedical Sciences, Faculty of Medicine, Universidad de Chile, Santiago, Chile
- 2Millennium Nucleus of Ion Channels-Associated Diseases, Santiago, Chile
- 3The Wound Repair, Treatment and Health (WoRTH) Initiative, Santiago, Chile
Cell migration is a key process in cancer metastasis, allowing malignant cells to spread from the primary tumor to distant organs. At the molecular level, migration is the result of several coordinated events involving mechanical forces and cellular signaling, where the second messenger Ca2+ plays a pivotal role. Therefore, elucidating the regulation of intracellular Ca2+ levels is key for a complete understanding of the mechanisms controlling cellular migration. In this regard, understanding the function of Transient Receptor Potential (TRP) channels, which are fundamental determinants of Ca2+ signaling, is critical to uncovering mechanisms of mechanotransduction during cell migration and, consequently, in pathologies closely linked to it, such as cancer. Here, we review recent studies on the association between TRP channels and migration-related mechanotransduction events, as well as in the involvement of TRP channels in the migration-dependent pathophysiological process of metastasis.
Introduction
Cells are capable of accurately sensing and effectively responding to diverse stimuli in the external environment (Clapham, 2003). In response to mechanical stimuli, such as fluid shear stress or extracellular matrix stiffness, activation of intracellular signals leads to a robust cellular response in a process referred to as mechanotransduction (Jaalouk and Lammerding, 2009). Mechanotransduction is crucial to numerous physiological processes, including embryonic development and homeostasis of adult organs (Vogel and Sheetz, 2006; Wozniak and Chen, 2009). These processes are the result of diverse cellular responses that together underlie the mechanotransduction events that allow adaptation to physiological demands. Those cellular responses include proliferation, differentiation, survival, death, and migration (Jaalouk and Lammerding, 2009; Sun et al., 2016). Among these processes cell migration is distinct in that mechanical forces play a central role, in both being sensed and forming the response that is generated by the cells, which then promotes the movements needed to carry out a determined physiological function. Recent research has led to the identifcation of a number of novel molecular components of the pathways underlying migration-associated mechanotransduction, helping to elucidate the molecular mechanisms crucial to this important cellular response. Among these, Transient Receptor Potential (TRP) channels have emerged as key players in cell migration (Schwab et al., 2012). A number of studies have defined specific roles for these channels in diverse aspects of mechanotransduction processes (Clapham, 2003; Sharif-Naeini et al., 2008; Yin and Kuebler, 2010; Kuipers et al., 2012; Eijkelkamp et al., 2013). Here, we discuss the role of TRP channels in cell migration from a mechanostransduction perspective, and the specific role they play in the migration-dependent pathophysiological phenomenon of cancer metastasis.
Mechanotransduction Mechanisms Shaping Cell Migration
Cell migration is a process involved in numerous and diverse important physiological and pathophysiological events, including wound healing, the immune response, embryonic development and metastasis of cancer cells (Locascio and Nieto, 2001; Luster et al., 2005; Bravo-Cordero et al., 2012; Shaw and Martin, 2016). Molecular mechanisms controlling migration are highly dependent on biochemical signals derived from mechanical events, in which the actin cytoskeleton and cell adhesion structures play a key role, since they sense the mechanical forces from the extracellular environment and, in response, generate mechanical forces for migration (Lauffenburger and Horwitz, 1996). Migration is characterized by dynamic cycles consisting of four general steps: protrusion of the leading edge, adhesion to extracellular matrix (ECM), generation of traction forces and detachment of the trailing edge (Lauffenburger and Horwitz, 1996). Migration starts with the polarization and extension of actin-based protrusions, initially filopodium and later lamellipodium, in the direction of migration. In the case of filopodia, actin filaments are organized as long parallel bundles and its formation is dependent on Rho-GTPase Cdc42 activity; conversely, lamellipodia present a branching network of actin filaments and its formation is dependent on the action of Rho-GTPase Rac1 (Hall, 1998; Small et al., 2002; Mattila and Lappalainen, 2008). The forces generated in the edge of lamellipodia by actin assembly are responsible for the formation of protrusions and nascent adhesions at the leading edge of migrating cell (Choi et al., 2008). These nascent adhesions assemble rapidly in response to the attachment of transmembrane receptor integrins to the ECM which subsequently leads to the recruitment of the intracellular adaptor proteins talin and paxillin that link integrins to the F–actin cytoskeleton (Laukaitis et al., 2001; Zaidel-Bar et al., 2003). As the migration process progresses, nascent adhesions can either disassemble or mature into focal complexes and focal adhesions, increasing their size by mechanical tension, and promoting the recruitment of additional proteins (vinculin, alpha-actinin, VASP and Focal Adhesion Kinase or FAK) that generate and stabilize the focal complex, followed by the addition of zyxin and tyrosine phosphatases that lead to formation of focal adhesions (Zaidel-Bar et al., 2003). Mature focal adhesions associate with the end of stress fibers, structures composed of bundles of actin and myosin II that have a high contractile capability (Burridge and Wittchen, 2013). The formation of both stress fibers and focal adhesions are dependent on Rho-GTPase RhoA activity (Ridley and Hall, 1992). The actin-myosin cytoskeleton contraction generates the force necessary to pull the cell body forward (Lauffenburger and Horwitz, 1996). Finally, in the rear of migrating cells, focal adhesions disassemble, promoting cell retraction at the trailing edge, and allowing for forward cellular movement (Ridley et al., 2003).
Ca2+ signaling plays a critical role in each of these steps. During cell migration, intracellular Ca2+ signaling events are regulated in their amplitude, as well as their spatial and temporal characteristics allowing for an effective control of the consecutive events occurring in the different compartments of the migrating cell (Wei et al., 2012). Ca2+ is distributed in a front-to-rear increasing gradient in migrating cells (Brundage et al., 1991; Hahn et al., 1992). In the leading edge of the cell, Ca2+ increases occur as “flickers,” directing the cycles of lamellipodia retraction and nascent focal complex formation (Giannone et al., 2007; Wei et al., 2009). Ca2+ signaling induces the myosin-light chain (MLC) kinase-dependent phosphorylation of MLC, promoting the contraction of myosin II and the consequent retraction of the actin bundle, including the attachment of the leading edge and stabilization of the nascent focal adhesions (Tsai and Meyer, 2012). In the rear of migrating cells, Ca2+ activates the Ca2+-dependent protease calpain which catalyzes the cleavage of focal adhesion proteins such as integrins, talin, vinculin and FAK (Glading et al., 2002), promoting the disassembly of these structures and, thereby, allowing trailing edge retraction. Additionally, Ca2+/calmodulin activates the serine/threonine phosphatase calcineurin, which mediates the recycling of integrins from the sites of disassembly of trailing edge focal adhesions (Lawson and Maxfield, 1995). Finally, Ca2+ acts upstream of cytoskeleton rearrangement and focal adhesion dynamics, modulating the activity of Rho GTPases Rac1 and RhoA, which activates several effectors that promote the lamellipodia formation and regulate the thickness of stress fibers, respectively (Small et al., 2002; Price et al., 2003; Dovas et al., 2006).
As stated above, mechanical forces participate in all steps of migration for effective cell movement. The mechanical forces that are generated in the processes previously described promote successive rounds of cell adhesion, contraction and retraction in response to cellular signals controlling cell movement, such that migration is the net result of several mechanotransduction events. For instance, mechanical stretching of the nascent adhesion protein talin leads to a conformational change in talin that leads to presentation of its cryptic vinculin binding sites (del Rio et al., 2009), leading to the subsequent recruitment of vinculin that is necessary for maturation of nascent adhesions into focal complexes and focal adhesions (Zaidel-Bar et al., 2003). Integrins transmit tension from the actomyosin cytoskeleton to ECM protein fibronectin, producing the conformational changes in fibronectin that are crucial to fibronectin assembly (Zhong et al., 1998). As previously mentioned, each of these molecular events shaping cell migration are controlled by Ca2+ signaling. Accordingly, the plasma membrane ion channels mediating Ca2+ entry into cells are fundamental components of the mechanotransduction that underlies cell migration. Among these channels, certain members of the TRP channel superfamily play a particularly critical role in mechanotransduction events during cell migration.
Transient Receptor Potential (TRP) Channels
TRP channels constitute a superfamily of polymodal non-selective cation channels, formed as tetramers of subunits whose structure is characterized by six transmembrane domains, with the channel pore located between domains 5 and 6, and with cytoplasmic amino (N)- and carboxyl (C)-terminal regions (Nilius and Owsianik, 2011). In mammals, these channels are categorized into six subgroups, based on sequence homology: Canonical TRP channels (TRPC), Melastatin TRP channels (TRPM), Vanilloid TRP channels (TRPV), Mucolipin TRP channels (TRPML), Polycystin TRP channels (TRPP), and Ankyrin TRP channels (TRPA) (Nilius and Owsianik, 2011). TRP channels can be activated by a variety of stimuli, including endogenous and exogenous ligands as well as physical-chemical stimuli, such as temperature, pH changes, tension, osmolarity, and pressure (Clapham, 2003; Nilius and Owsianik, 2011). In general, the mechanosensitivity of plasma membrane ion channel activity has been proposed to be due to either channel gating being controlled by lateral tension at the membrane (the bilayer model) or by a linkage of the channels to ECM and/or subcortical actin cytoskeleton (the tethered model) (Marshall and Lumpkin, 2012). It is presumed that such mechanisms mediate could also allow mechanical changes to regulate TRP channel activity. Indeed, TRP channel activity can be modified by alterations in plasma membrane phospholipids, such as phosphatidylinositol-4,5-biphosphate (PIP2) (Nilius et al., 2008), or by changes in cell structural components that alter their gating, such as actin cytoskeleton (Liu and Montell, 2015). Since most TRP channels mediate Ca2+ entry, these plasma membrane proteins constitute important candidate molecules controlling cell migration mechanisms from the mechanotransduction perspective. In fact, there is increasing evidence that TRP channels are key regulators of Ca2+ signaling mediating the mechanotransduction shaping cell migration, and in particular a role for TRP channels in actin cytoskeleton rearrangement and focal adhesions dynamics (Figure 1).
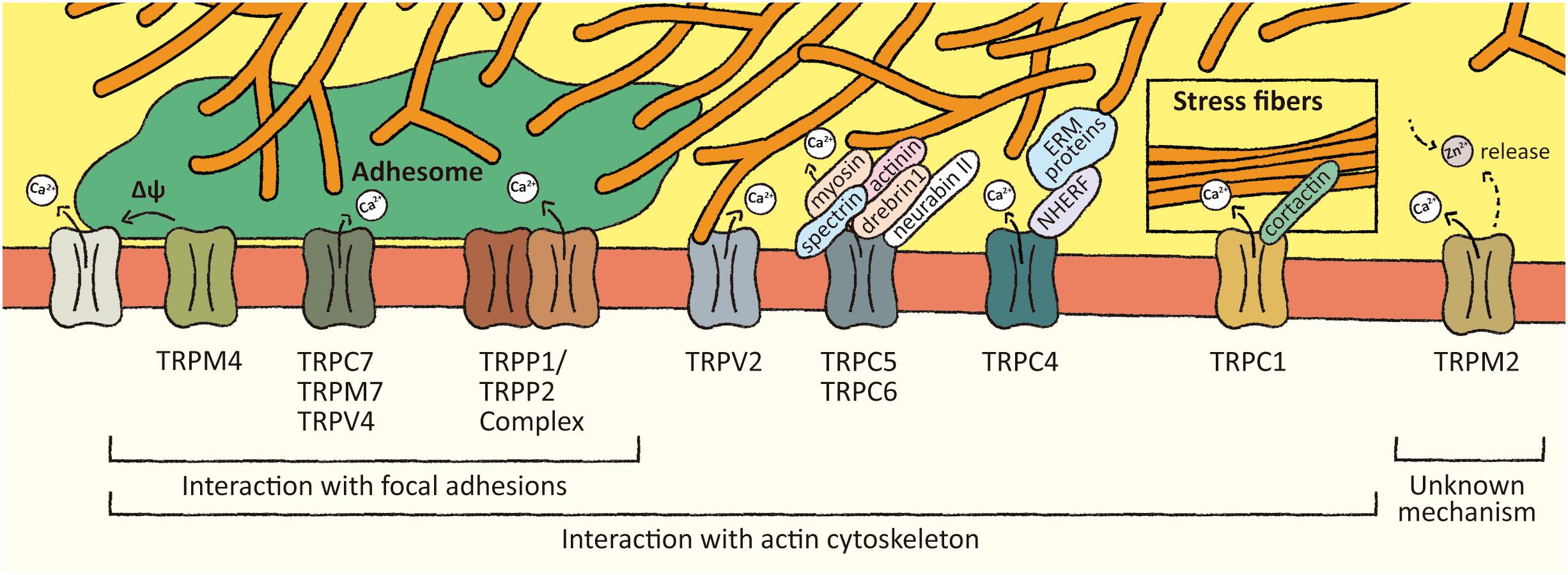
Figure 1. Association of TRP channels with mechanosensitive structures. Several members of TRP channels superfamily interact with focal adhesions and/or actin cytoskeleton-associated proteins. These interactions might regulate TRP channels activity leading to local changes in Ca2+ levels and membrane potential. These local effects might promote focal adhesions turnover and actin cytoskeleton remodeling.
Association of TRP Channels With Mechanosensitive Structures
TRP Channels and the Actin Cytoskeleton
Actin cytoskeleton dynamics are a trascendental aspect of cell migration. These processes control protrusion, adhesion, contraction, and retraction from the cell front to the rear (Gardel et al., 2010). These dynamics are regulated by a variety of actin-associated protein and signaling pathways that are highly dependent on changes in intracellular Ca2+ levels, as Ca2+ plays a crucial role in the reorganization of the actin cytoskeleton by modulating the function of actin-associated proteins (Pollard and Cooper, 2009; Tang and Gerlach, 2017). Plasma membrane ion channels play a fundamental role in regulating diverse signaling pathways by mediating the flux of ions across the plasma membrane. Among these, the cation permeable TRP channels are especially important in cell migration (Smani et al., 2014). The association of TRP channels with the actin cytoskeleton results in bidirectional communication: on the one hand, TRP channels mediate the flux of ions that promotes actin cytoskeleton reorganization, and conversely, the actin cytoskeleton and associated proteins induce changes in TRP channel location, protein–protein interactions and channel gating, thereby modulating TRP channel function (Smani et al., 2014).
Actin Cytoskeleton-Mediated TRP Channel Regulation
Some members of the TRP superfamily directly interact with the actin cytoskeleton, while other members interact indirectly through other associated proteins. For instance, TRPC4, a non-selective cationic Ca2+ permeable channel member of the TRP Canonical subfamily. TRPC4 is activated by Gq/phospholipase C-coupled receptors and tyrosine kinases, and has been implicated in endothelial permeability, vasodilation and cell proliferation (Zhu et al., 1996). TRPC4 interacts with NHERF, a molecular scaffold and regulatory factor of the Na+/H+ exchanger (Tang et al., 2000). NHERF is a two PDZ domain-containing protein associated with the actin cytoskeleton via interactions with members of ezrin/radixin/moesin family (ERM) (i.e., ezrin and moesin) through their N-terminal domains (Reczek et al., 1997). The C-termini of ERM proteins interact with F-actin in a manner dependent on oligomerization and ERM phosphorylation states (Tang et al., 2000). Thus, the interaction of TRPC4 with NHERF provides a physical link between these plasma membrane ion channels and the actin cytoskeleton. TRPC4 interacts with NHERF via a PDZ interacting motif present on the C-terminus of TRPC4. The C-terminus of TRPC4 also contains a PIP2 binding domain that underlies the PIP2-mediated inhibition of TRPC4 activity (Otsuguro et al., 2008). Interestingly, TRPC4/actin cytoskeleton association as mediated by the C-terminal PDZ binding motif is required for PIP2-dependent TRPC4 inhibition (Otsuguro et al., 2008). As such, the association of TRPC4 with the ERM/actin cytoskeleton through TRPC4-NHERF interaction regulates PIP2-mediated inhibition of TRPC4 activity (Otsuguro et al., 2008). TRPC4 is also associated with to the actin cytoskeleton in endothelial cells, although in this case TRPC4 interacts with the 4.1 protein, which, in turn, is associated with the actin cytoskeleton through spectrin (Cioffi et al., 2003). The association of TRPC4 with the actin cytoskeleton is necessary for TRPC4 to function as a Store Operated Channel (SOC) (Cioffi et al., 2003). These data indicate that the TRPC4/actin cytoskeleton association occurs through different protein complexes, and that this association plays a key role of regulating TRPC4 activity.
TRPC1 is a non-selective cation channel that has also been proposed to be a component of SOC (Rychkov and Barritt, 2007). TRPC1 is also associated with the actin cytoskeleton in myoblasts (Formigli et al., 2009). In this case, TRPC1 can be co-immunoprecipitated with the actin-binding protein cortactin, and this interaction is increased by the sphingosine-1-phosphate-induced formation of stress fibers (Formigli et al., 2009). The cortactin-mediated interaction of TRPC1 with stress fibers is essential for the localization of TRPC1 in cell membrane lipid rafts; such localization is necessary for the TRPC1 channel activity, indicating that in myoblasts TRPC1/actin stress fiber interaction is required for channel activity (Formigli et al., 2009).
Two other members of the Canonical TRP subfamily, TRPC5 and TRPC6, interact with actin cytoskeletal proteins. Immunopurification of TRPC5 and TRPC6 from rat brain lysates and mass spectrometry-based analyses of associated proteins led to identification of a number of cytoskeletal proteins, including spectrin, myosin, actin, actinin, debrin 1 and neurabin II, the latter a F-actin binding protein that has a single PDZ domain (Goel et al., 2005). However, the functional consequences of the association of these TRP channels with these cytoskeletal proteins has not been elucidated.
TRPV4 is a member of the vanilloid subfamily of TRP channels. TRPV4 is a Ca2+-permeable TRP channel that was identified as a receptor for a variety of physical and chemical stimuli, such as hypotonicity, moderate heat and synthetic or endogenous agonists (Garcia-Elias et al., 2014). Interestingly, TRPV4 interacts with actin in a number of different cell types (Becker et al., 2009; Goswami et al., 2010). TRPV4 and actin co-localize in highly dynamic pro-migratory membrane structures, such as filopodia and lamellipodia edges (Becker et al., 2009). However, more stable structures, such as F-actin bundles and stress fibers, lack TRPV4. The interaction between TRPV4 and F-actin is required for the activation of these channels by hypotonicity and underlies TRPV4-mediated volume decrease (Becker et al., 2009). TRPV4 also interacts with other cytoskeletal elements including tubulin and neurofilament proteins (Suzuki et al., 2003; Goswami et al., 2010). The TRPV4 C-terminal domain interacts directly with both tubulin and actin, and for both major cytoskeletal proteins in both with their soluble and polymeric forms. Moreover, TRPV4 co-localizes in vivo with both actin-based microfilament and tubulin-based microtubule enriched structures at submembrane regions. Elevated expression and/or activation of TRPV4 induces striking morphological changes affecting lamellipodial and filopodial structures (Goswami et al., 2010). There is evidence that TRPV4 preferentially associates with actin filaments in a process that involves phosphorylated Ser824 in its C-terminal tail (Shin et al., 2012). This presumably direct TRPV4-actin interaction promotes TRPV4 cell surface expression, its single channel activity and consequent Ca2+ influx (Shin et al., 2012).
Another example of direct interaction of a plasma membrane TRP channel with the actin cytoskeleton is TRPV2, a non-selective cation channel with a relatively high Ca2+ permeability, and whose activity is promoted by heat (Shibasaki, 2016). TRPV2 acts as a mechanosensitive ion channel in the digestive tract and during neuronal development (Mihara et al., 2010; Shibasaki et al., 2010). Recently studies show that that local application of mechanical stimuli promotes actin reorganization via TRPV2 activation in PC12 cells. TRPV2 physically interacts with actin and actin cytoskeleton changes are required for its activation. Thus the actin cytoskeleton is critical for TRPV2 activation by mechanical stimuli, which subsequently feeds back to impact reorganization of the actin cytoskeleton (Sugio et al., 2017).
TRP Channel-Promoted Actin Cytoskeleton Remodeling
The actin cytoskeleton plays a vital role in maintaining the structural integrity of cells and in producing the force necessary to accomplish basic cellular functions, including cytokinesis, contractility, adhesion and migration. Many members of the TRP family act to impact these processes by promoting changes in the actin cytoskeleton. For example, activation of TRPC channels under conditions of hypoxic stress promotes Ca2+-dependent MLC phosphorylation, necessary for contraction of actin and myosin filaments, thus generating a contraction of the endothelial cells that disrupts the blood brain barrier, leading to hypoxia-induced increases in its permeability (Hicks et al., 2010).
One of the most common mechanisms by which TRP channels control the actin cytoskeleton is through the activation of Rho GTPases. As mentioned above, Rac1 and Cdc42 play key roles in generating actin-rich lamellipodial and filopodial membrane extensions, respectively. These processes are initiated by peripheral actin polymerization and they are required for both cell spreading and migration (Hall, 1998). Conversely, RhoA is primarily associated with actin stress fiber formation and actomyosin contractility (Ridley and Hall, 1992). TRPC5 and TRPC6 channel activity promotes the activation of Rac1 and RhoA, respectively. TRPC5 suppresses stress fiber and focal adhesion formation through Rac1 activation, promoting a motile phenotype (Tian et al., 2010). Conversely, growth factor-induced Rac1 activation induces TRPC5 membrane translocation, which has been linked to TRPC5 localization in growth cones and filopodia of hippocampal neurons and the subsequent TRPC5-dependent regulation of growth cone motility and morphology (Greka et al., 2003; Bezzerides et al., 2004). Conversely, through RhoA activation TRPC6 activity promotes formation of stress fibers and adhesions resulting in a contractile and stationary cell phenotype (Tian et al., 2010). The mechanisms whereby TRPC6 produces these effects have been studied in a number of different contexts. TRPC6 activity promotes endothelial cell contraction and increases endothelial permeability through enhanced intracellular Ca2+ signaling and subsequent PKCα and RhoA activation, which favors MLC phosphorylation and actin stress fiber formation (Singh et al., 2007). In the context of proteinuric kidney disease, TRPC6 overexpression generates RhoA activation and consequent stress fiber formation, thus promoting cell retraction that hinders the extension of foot processes and may lead to proteinuria (Jiang et al., 2011).
TRPV4 function has also been linked to RhoA activity. TRPV4 upregulation promotes actin polymerization and stress fiber formation through RhoA activation in lung fibroblasts derived from patients with idiopatic pulmonary fibrosis, acting as a key mechanosensor for myofibroblast differentiation (Rahaman et al., 2014). Furthermore, TRPV4 participates in the intraocular pressure-induced increase in trabecular meshwork cell stiffness by mediating mechanical stretch-promoted Ca2+ entry that is required for cytoskeleton remodeling in the form of enhanced stress fibers (Ryskamp et al., 2016). Conversely, exogenous expression of TRPV4 in breast cancer cells accelerates actin dynamics and is correlated with a higher activation of cofilin, a protein that promotes the severing of actin filaments (Lee et al., 2016).
Numerous TRPM subfamily members have also been linked to cytoskeleton remodeling processes. TRPM4, a Ca2+-activated non-selective monovalent cation channel (Vennekens and Nilius, 2007), interacts with diverse proteins involved in actin cytoskeleton dynamics and regulates Ca2+ increases, FAK activation and Rac1 GTPase activity. All of these processes impact the actin cytoskeleton reorganization that is involved in cellular attachment and lamellipodia formation, mechanisms underlying both cell spreading and migration (Caceres et al., 2015). Another member of the TRPM subfamily linked to actin cytoskeleton regulation is TRPM7, a TRP channel that is permeable to Na+, Mg2+ and Ca2+, and whose activity is dependent on Mg2+ and intracellular ATP levels (Visser et al., 2014). This channel is exceptional in that it contains a C-terminal protein kinase domain (Visser et al., 2014). Knockdown of TRPM7 in fibroblasts leads to a decrease in actin stress fibers and an increase in cortical actin (Su et al., 2011). This correlates with decreased activity of RhoA GTPase, suggesting a role for TRPM7 in RhoA regulation. Additionally, TRPM7 knockdown leads to reduced Rac1 Rho-GTPase activity and alters temporal aspects of Cdc42 Rho-GTPase activation, consequently decreasing lamellipodia formation and impairing the directionality of cell migration (Su et al., 2011).
Beyond TRP channel-mediated Rho GTPase regulation, these ion channels participate in the dynamic modulation of the actin cytoskeleton through Rho GTPase-independent mechanisms. In the context of myoblast migration and differentiation, TRPC1 channel activity is necessary for calpain activation and the consequent calpain-promoted cleavage of actin-binding protein MARCKS (Louis et al., 2008), which has been linked to an increase in actin polymerization rates (Tapp et al., 2005). Conversely, TRPM7 associates with the actomyosin cytoskeleton and, through its C-terminal protein kinase domain, phosphorylates the C-terminal region of myosin IIA heavy chain, which is critical for filament assembly, regulating filament stability and protein localization (Clark et al., 2008). Thus, TRPM7 also participates in Ca2+-independent mechanisms for actin-cytoskeleton rearrangement. Interestingly, another member of TRPM channel family, TRPM2, also promotes actin remodeling during cell migration independent of its canonical ion channel activity. TRPM2 is a Ca2+ and monovalent cation-permeable ion channel, whose activity is both temperature-sensitive and activated by several ligands and reactive oxygen species (Kashio and Tominaga, 2017). TRPM2 promotes H2O2-induced filopodia formation in HeLa and PC-3 cells independent of TRPM2-mediated Ca2+ entry, but dependent on TRPM2-mediated intracellular Zn2+ release (Li et al., 2016). This corroborates the idea that actin cytoskeleton rearrangement mechanisms linked to TRP activity go beyond the canonical pathways involved in Ca2+ signaling or Rho GTPases activity. Thus, there remain numerous unexplored aspects of TRP channel function in mechanotransduction during cell migration.
TRP Channels and Focal Adhesions
Focal adhesions are dynamic structures that connect the F-actin cytoskeleton with the ECM through transmembrane receptor integrins (Singer, 1982; Horwitz et al., 1986; Hynes, 1992; Wehrle-Haller and Imhof, 2002; Winograd-Katz et al., 2014). Focal adhesions contain more than 160 proteins and have pleiotropic functions: adhesion to ECM, binding to actin filaments, cellular signaling and adaptors (Winograd-Katz et al., 2014). A distinct set of Ca2+-sensitive proteins, including protein kinases and calpain proteases, regulate the assembly and disassembly of these structures (Giannone et al., 2004; Chan et al., 2010). As such, regulation of Ca2+ levels in close proximity to focal adhesions plays an important role in the dynamics of these multiprotein complexes that directly impact cell migration. Growing evidence linking focal adhesions and TRP channels has opened a novel perspective to understand the migration processes.
TRPV4 has been described as a mechanical strain-activated channel, as force applied to β1 integrin promotes rapid TRPV4 activation (Matthews et al., 2010). Moreover, there is evidence supporting a direct role for TRPV4 in focal adhesion dynamics, and particularly on the disassembly of these structures. TRPV4 co-localizes with the focal adhesion marker paxillin, overexpression of a defective form of TRPV4 that is unable to bind PIP2 is correlated with lower calpain activity, and cell lines overexpressing TRPV4 show smaller focal adhesions. Conversely, TRPV4 silencing as well as overexpression of the PIP2 binding defective TRPV4 mutant results in larger focal adhesions (Mrkonjic et al., 2015). This leads to a model whereby TRPV4 channel regulates local Ca2+ levels, which in turn activate protein kinases and calpains involved in the disassembly process (Mrkonjic et al., 2015). A pore-dead TRPV4 mutant mimics the effects on focal adhesions promoted by the non-PIP2-interacting TRPV4 mutant, suggesting that TRPV4-mediated Ca2+ entry is responsible for all these effects (Mrkonjic et al., 2015).
Certain members of the TRPM and TRPC subfamilies also participate in focal adhesion regulation. TRPM7 regulates focal adhesion disassembly and turnover via m-Calpain, by promoting an increase of intracellular Ca2+ levels (Su et al., 2006). TRPM4 localizes to focal adhesions where it promotes an increase of intracellular Ca2+ levels and induces focal adhesion disassembly via regulation of FAK and paxillin activities (Caceres et al., 2015). Similar to TRPM4 channels, TRPC7 localizes to focal adhesions during the Syndecan-4-mediated maintenance of myofibroblast phenotype. Moreover, expression of a non-phosphorylatable TRPC7 mutant (i.e., S714A substitution) leads to aberrant increases in intracellular Ca2+ levels and induces the loss of focal adhesions (Gopal et al., 2015).
TRPP1 (also known as Polycystin-1) forms a mechanosensor heterodimer with the six transmembrane domain protein TRPP2 (also known as Polycystin-2) (Retailleau and Duprat, 2014). Within the TRPP1/TRPP2 complex, TRPP1 is proposed to function as a mechanosensor, while TRPP2 acts as the ion channel to mediate Ca2+ influx (Retailleau and Duprat, 2014). Consistent with the notion of the TRPP1/TRPP2 heterodimer acts as a mechanosensitive ion channel, TRPP1 is associated with mechanotransductor focal adhesions. Moreover, TRPP1 localizes to focal adhesions and associates with focal adhesion components such as FAK, paxillin and vinculin (Wilson et al., 1999; Geng et al., 2000; Joly et al., 2006). Interestingly, TRPP1 activity has been linked to FAK and paxillin phosphorylation and to adhesion complex formation (Joly et al., 2006). However, whether the proposed role of TRPP1 in regulating focal adhesions is dependent on its heterodimerization with TRPP2 has not been elucidated.
TRP Channels and Cell Migration
As discussed above, several members of the TRP channel superfamily have been associated with structures and processes fundamental for mechanotransduction in cell migration, in particular the actin cytoskeleton and focal adhesions (Table 1). However, we have not yet addressed the nature and extent of how this translates into a functional role for TRP channels in impacting cell migration.
TRPM7 has been linked to several mechanotrasduction mechanisms in cell migration. First, in human embryonic lung fibroblasts, TRPM7 is activated by membrane tension at the leading edge of migrating cells, promoting Ca2+ entry, which activates the IP3R2 and thereby produces the local Ca2+ flickers necessary for cell migration (Wei et al., 2009). TRPM7 overexpression has also been linked to loss of cell adhesion through m-Calpain activation (Su et al., 2006). Accordingly, TRPM7 knockdown induces an increase in the number of focal adhesions and contractility in the breast cancer cell line MDA-MB-231, which correlates with a reduced migratory/invasive phenotype (Middelbeek et al., 2012). The contribution of TRPM7 function to cell migration of human nasopharyngeal carcinoma cells involves Ca2+-induced Ca2+ release, where TRPM7 activity promotes Ryanodine Receptor activation and, consequently, increases intracellular Ca2+ levels and cell migration (Chen et al., 2010). Thus, TRPM7 has been demonstrated to directly impact migration of diverse cell types through its mechanotransduction actions as mediated by intracellular Ca2+. TRPM2 channel activity also promotes cell migration, in this case H2O2-induced migration in HeLa cells (Li et al., 2016). Consistent with the impact on actin remodeling detailed above, the role of TRPM2 in cell migration is independent of the increased Ca2+ signals mediated by these channels, but dependent on Zn2+ release (Li et al., 2016).
Another member of the TRPM channel subfamily linked to cell migration is the TRPM4 channel. TRPM4 has been implicated in several mechanisms of mechanotransduction in cell migration. Enhanced TRPM4 activity promotes focal adhesion disassembly, actin cytoskeleton reorganization as evaluated through cell spreading, Rho-GTPase Rac1 activation and FAK activation (Caceres et al., 2015). TRPM4 regulates mouse embryonic fibroblast cell migration in a Rac1-dependent manner, and pharmacological TRPM4 inhibition delays skin wound healing in vivo (Caceres et al., 2015). Interestingly, TRPM4 inhibition decreases intracellular Ca2+ signaling (Caceres et al., 2015). There is increasing evidence that although the TRPM4 channel is not Ca2+-permeable, it can act to indirectly regulate intracellular Ca2+ levels (Launay et al., 2004; Gonzales et al., 2014). Thus, it is plausible that enhanced activity of TRPM4, through an indirect effect on intracellular Ca2+, acts to promotes the processes detailed above that lead to cell migration. TRPV4 channels are also involved in mechanotransduction-promoted cell migration. As detailed above, expression of a mutant TRPV4 defective in PIP2 binding inhibits focal adhesion turnover (Mrkonjic et al., 2015). This results in a non-directional migratory pattern, characterized by fast and persistent movements followed by stalling and turning motion (Mrkonjic et al., 2015). Conversely, TRPV4 overexpression leads to a continuous loss of directionality. Thus, it is likely that TRPV4 participates in directionality of migrating cells and formation of trailing edge adhesions, although a causal relationship between both effects has not been clearly established (Mrkonjic et al., 2015). Beyond their effects on focal adhesions, TRPV4 channels have been linked to the regulation of cell stiffness by modulating the actin cytoskeleton. TRPV4 accelerates actin dynamics by promoting F-actin depolymerization, thereby contributing to reduced cell stiffness of breast cancer cells. This feature is essential for endothelial transmigration and extravasation, the process through which metastatic cells traverse the endothelial lining to access the circulatory system and colonize distant organs (Lee et al., 2016). It should be noted that this result is seemingly contradictory to a report that TRPV4 promotes stress fiber formation and cell rigidity in trabecular meshwork cells (Ryskamp et al., 2016). However, the latter study did not address cell migration, but instead the structural stiffness of trabecular meshwork cells in the context of glaucoma disease. It is important to emphasize that to understand the role of TRPV4 in actin cytoskeleton dynamics it is necessary to consider the cell model and the pathophysiological context in which these studies are contextualized.
Another member of the TRPV family associated with cell migration is TRPV2. It has been reported that TRPV2 is activated by the lysophospholipids, lysophosphatidylcholine and lysophosphatidylinositol, and TRPV2-knockdown in the prostate cancer cell line PC3 decreases lysophospholipid-induced migration (Monet et al., 2009). Moreover, exogenous expression of TRPV2 increases migration in the prostate cancer cell line LNCaP (Monet et al., 2010). TRPV2 has been linked to adrenomedullin-promoted adhesion and migration of prostate (PC-3) and urothelial (T24/83) cancer cells in a mechanism involving TRPV2 translocation to the cell membrane and increased cytoplasmic Ca2+ levels (Oulidi et al., 2013). However, the role of TRPV2 in regulating specific mechanotransduction mechanisms in cell migration is still undetermined.
Among TRPC subfamily members, TRPC5 activity induces Rac1 activation and subsequent suppression of stress fiber and focal adhesion formation (Tian et al., 2010). Consistent with these mechanistic and structural effects, TRPC5 activity has been linked to higher Angiotensin-I receptor-promoted podocytes migration (Tian et al., 2010). TRPC5-mediated Ca2+ signals triggered by sphingosine-1-phosphate in vascular smooth muscle cells have been associated with increased cell migration (Xu et al., 2006), although whether these effects are dependent on the impact of TRPC5 activity to suppress stress fibers and focal adhesions has not been defined. TRPC1-mediated Ca2+ entry has been linked to calpain activation in skeletal myoblasts, and this activity is necessary for cell migration during myogenesis via a mechanism that presumably involves the calpain-promoted cleavage of the actin-binding protein MARCKS (Louis et al., 2008). Consistent with the impact of TRPC1 on actin dynamics, TRPC1 silencing prevents polarization and yields defective lamellipodia formation and reduced migration in a migrating MDCK model (Fabian et al., 2008).
TRP Channels and Cell Migration: Implications for Cancer Metastasis
Metastasis is the cause of more than 90% of cancer deaths (Siegel et al., 2011). It is a hallmark of cancer that is characterized by the spread of malignant cells from the primary tumor to distant organs (Talmadge and Fidler, 2010; Hanahan and Weinberg, 2011). In this process, cancer cells from the primary tumor, invade the basal membrane and reach lymphatic and blood vessels. The cancer cells then transit through these systems and extravasate to form distant metastatic foci. Therefore, to metastasize, malignant cells must acquire migration and invasion properties (Friedl and Wolf, 2003). Increasing evidence shows that the stiffness of solid tumors enhances the mechanical forces in tumor cells, leading to the activation of mechanotransduction signals that promote several pro-carcinogenic responses, including cell invasion and metastasis (Kopanska et al., 2016; Wei and Yang, 2016; Broders-Bondon et al., 2018). Thus, the mechanotransduction events occurring during metastasis development provide a new perspective to understand mechanisms involved in cancer progression. As a consequence, the TRP channels that are involved in mechansotransduction are emerging as prominent actors in this context. Some members of the TRP channel superfamily contribute to metastasis, presumably by promoting the cancer cell’s acquisition of a migratory and invasive phenotype, which are fundamental for the development of this advanced stage of the disease.
TRPM7 is the best studied TRP channel in the context of metastasis. In primary breast cancer tumors, higher expression of TRPM7 mRNA correlates with diminished distant metastasis free-survival and free-recurrence survival (Middelbeek et al., 2012). These data are supported by elevated levels of TRPM7 mRNA in metastatic breast cancer compared to primary tumors (Meng et al., 2013). TRPM7 protein is also overexpressed in ductal adenocarcinoma compared to non-tumoral tissue (Guilbert et al., 2009), and TRPM7 levels are increased in invasive areas of Estrogen Receptor negative invasive ductal cancer (Guilbert et al., 2013). TRPM7 silencing in the triple negative breast cancer cells MDA-MB-231 decreased their metastatic potential in vivo by reducing their migratory ability, but not their viability (Middelbeek et al., 2012). TRPM7 has also been linked to pancreatic cancer (Yee et al., 2015; Rybarczyk et al., 2017). TRPM7 protein expression correlates with primary tumor size and stage of malignant pancreatic tumors, and is expressed at higher levels in metastatic tumors and in primary metastasizing tumors than normal tissue (Yee et al., 2015). TRPM7 is also overexpressed in pancreatic ductal adenocarcinoma, in which higher TRPM7 levels in primary tumors correlate with higher levels of lymph node metastasis (Rybarczyk et al., 2017). Pancreatic cancer cell lines also exhibit elevated levels of TRPM7 whose silencing decreases their invasive capacity (Yee et al., 2015). TRPM7 is also highly expressed in nasopharyngeal tumors, with higher TRPM7 mRNA levels in metastatic tumors than in primary tumors, and TRPM7 expression is absent in normal nasopharyngeal tissue (Chen et al., 2015). Moreover, elevated TRPM7 expression is associated with poor prognosis and metastasis in this type of cancer (Chen et al., 2015). These clinical data are supported by in vitro assays showing that TRPM7 knockdown decreases the migration and invasion of metastatic nasopharyngeal cancer cells, and that TRPM7 overexpression results in increases in both processes in non-metastatic nasopharyngeal cancer cells (Chen et al., 2015). TRPM7 is also associated with bladder cancer, such that TRPM7 mRNA and protein expression are higher in bladder tumor tissue compared to adjacent non-tumoral tissue, and elevated TRPM7 expression correlates with recurrence, metastasis and a poorer prognosis for this disease (Gao et al., 2017). Consistent with this, the TRPM7 knockdown decreases the migration and invasion of bladder cancer cells in vitro (Gao et al., 2017). Recently, another TRPM channel subfamily member has been associated to metastatic processes. It has been described that TRPM2 is functionally expressed in gastric cells and that is related to migration and invasion ability of gastric cancer cells in vitro as well as to tumor formation capacity and epithelial-mesenchymal transition markers expression in vivo (Almasi et al., 2019).
Vanilloid TRP channels subfamily members whose activity has been linked to mechanostransduction and migration have also been associated with cancer metastasis. As such, TRPV4 mRNA expression has been linked to a diminished distant metastasis free-survival in breast cancer samples (Lee et al., 2016). TRPV4 knockdown decreases migration, invasion, and transendothelial migration, but not the viability, of breast cancer cells in vitro as well as the number of metastatic lung nodules in vivo (Lee et al., 2016). Higher TRPV2 mRNA expression has also been observed in metastatic prostate cancer samples compared to localized prostate cancer samples (Monet et al., 2010). Xenograft tumor-based in vivo approaches in cancer prostate cells suggest that TRPV2 silencing diminishes the weight of tumors and the expression of invasion markers, namely MMP2, MMP9 and cathepsine B (Monet et al., 2010).
TRPC5 channels have also been linked to cancer metastasis. TRPC5 has been associated with colon cancer progression, and TRPC5 expression is higher in colon cancer samples than in paired normal tissue and also higher in metastatic lymph nodes than in paired primary tumors. Patients with elevated TRPC5 expression patients show a poorer overall survival and free-metastasis survival (Chen et al., 2017a). TRPC5 silencing in highly migratory and invasive colon cancer cells inhibits the migration and invasion, whereas exogenous expression of TRPC5 in colon cancer cells with a weak migration and invasion ability promotes an increase in these parameters (Chen et al., 2017b).
In summary, several members of TRP channel superfamily that have been demonstrated to act in mechanotransduction events translated in a migratory response have also shown to be relevant to metastasis of several types of cancer. These data raise the question whether other TRP channels reviewed here could have a similar pathophysiological potential, which represent a interesting challenge for future research.
Conclusion
Increasing evidence demonstrates the role of numerous members of the TRP channel superfamily in mechanotransduction and cell migration. However, in many cases the details of precise role of TRP channels in the underlying mechanisms remain poorly understood. The further dissection of the molecular events regulated by TRP channels constitute an interesting research topic. For example, questions remain as to the specific subcellular localization of these channels during cell migration processes. Moreover, the structural role of TRP channels as molecular platforms for organizing cell signaling complexes and other cellular structures remain largely unexplored. Recently, evidence for non-conducting functions of ion channels support these ideas. For example, the non-conducting or physical contribution of certain ion channels in the maintenance of cellular structures, in this case ER-PM junctions, has recently been demonstrated (Fox et al., 2015; Kirmiz et al., 2018). Thus, ion channels might not only regulate local ion fluxes, but may also serve as “transduction hubs” between plasma membrane structures such as focal adhesions and intracellular compartments. Moreover, since several members of the TRP channel superfamily are stretch-activated, these molecules could contribute to the mechanosensitive-dependent changes of the dynamic structure of these subcellular interactions during cell migration-related processes. Thus, the identification of the TRP channel-associated interactome found at these structures is an intriguing topic to be elucidated. This will provide new insights into the role of these molecules on the regulation of cell migration and mechanotransduction-related processes (Figure 2).
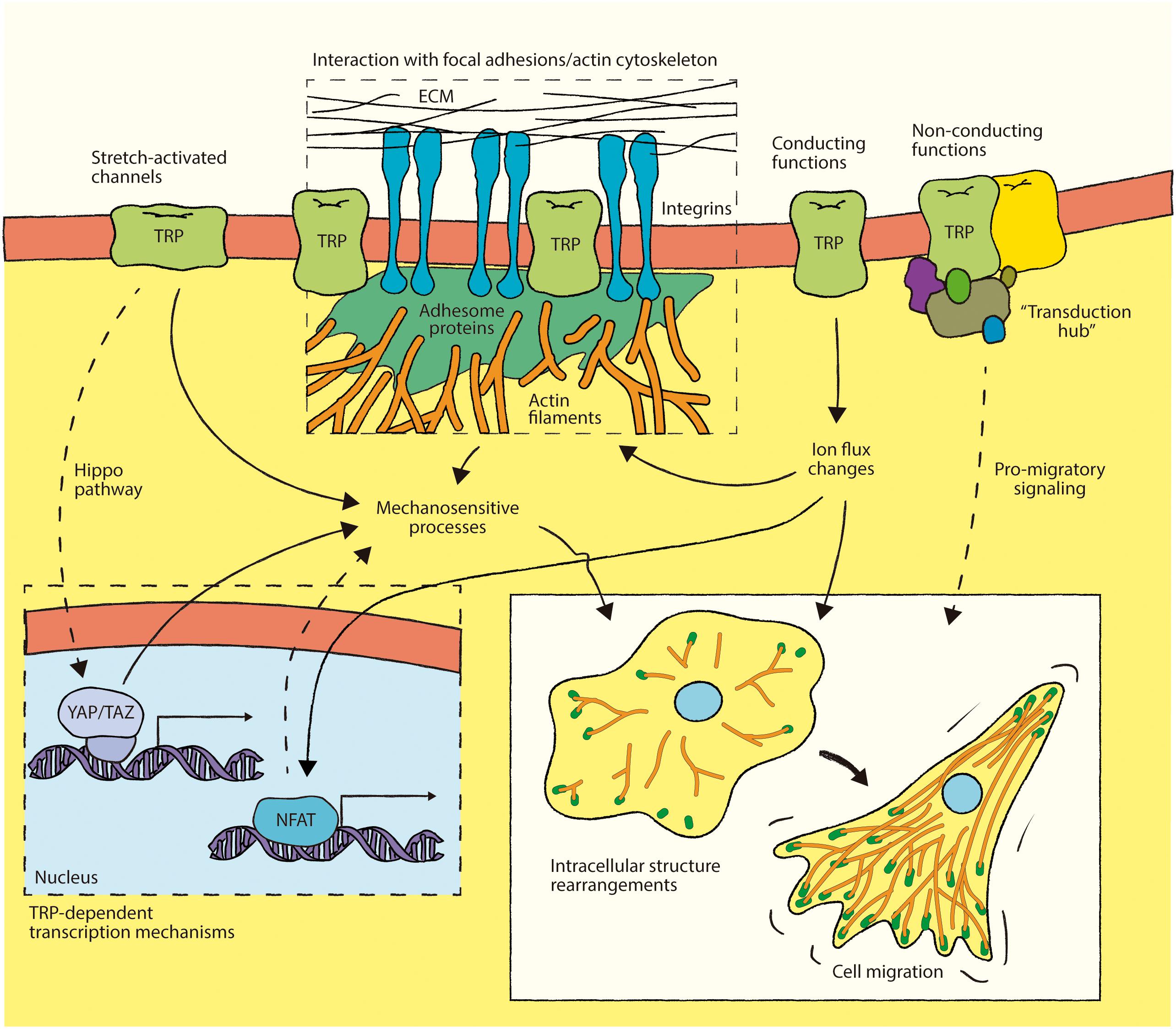
Figure 2. Integration of TRP channel involvement in mechanotransduction-associated mechanisms during cell migration. Some TRP channels interact with proteins at focal adhesions and/or with the actin cytoskeleton. These associations might lead to focal adhesion turnover and actin cytoskeleton rearrangement through a mechanism involving the conducting function of TRP channels, whereby Ca2+ influx plays a key role. In addition, non-conducting effects of TRP channels might contribute to regulation of focal adhesions and actin dynamics during cell migration. The putative role of TRP channels as structural component or “transduction hubs” in these mechanosensitive structures appears as a novel perspective to understand the involvement of these channels in mechanotransduction during cell migration. Furthermore, TRP channels could promote pro-migratory long-term effects by gene reprogramming (e.g., Hippo pathway- and/or NFAT activation-mediated), representing a putative new mechanism through which these ion channels could contribute to cell migration.
Finally, the role of TRP channels in mechanosensitive-dependent gene expression programs is still unknown. Recent findings have demonstrated the role of different TRP channels in NFAT-dependent transcription mechanisms (Kuwahara et al., 2006; Nakayama et al., 2006; Onohara et al., 2006; Numaga et al., 2010; Weber et al., 2010; Gao et al., 2012). There is also emerging information on the role of the YAP/TAZ transcriptional co-activators related to the Hippo pathway on mechanosensitive-related gene expression programs (Piccolo et al., 2014; Moroishi et al., 2015; Dupont, 2016; Figure 2). As such, the study of the crosstalk between TRP-dependent signaling pathways and these transcriptional mechanisms might contribute to our understanding of the roles of these in channels in long-term modulation of cell migration and mechanotransduction-dependent mechanisms, such as during developmental and differentiation programs, and under pathophysiological conditions, such as cancer.
Consistent with their role in cellular migration, certain TRP channels are associated with progression and metastasis of several types of cancer, likely due to their pro-migratory actions. Therefore, the emerging information on the novel molecular and cellular roles of TRP channels in physiological mechanostransduction and cell migration may also provide fundamental new insights into important pathophysiological processes. Future research in this area could help to clarify the emerging role of TRP channels in cancer metastasis and lead to the identification of novel targets for the development of a battery of new therapeutic alternatives to fight cancer.
Author Contributions
JC wrote the first draft of the manuscript. JC, DM, CB, JR, ND, IA, and OC wrote sections of the manuscript. OC conceived the idea. All authors contributed to the manuscript revision, read, and approved its final version.
Funding
This study was supported by the FONDECYT Grant 1160518 to OC. The Millennium Nucleus of Ion Channels-Associated Diseases (MiNICAD) was a Millennium Nucleus supported by the Iniciativa Científica Milenio of the Ministry of Economy, Development and Tourism (Chile). JC was supported by the FONDECYT Postdoctoral Grant 3180556.
Conflict of Interest Statement
The authors declare that the research was conducted in the absence of any commercial or financial relationships that could be construed as a potential conflict of interest.
Acknowledgments
We are grateful to Drs. JoAnne Engebrecht, Mónica Cáceres, and James S. Trimmer for their discussions and critical comments on the manuscript.
References
Almasi, S., Sterea, A. M., Fernando, W., Clements, D. R., Marcato, P., Hoskin, D. W., et al. (2019). TRPM2 ion channel promotes gastric cancer migration, invasion and tumor growth through the AKT signaling pathway. Sci. Rep. 9:4182. doi: 10.1038/s41598-019-40330-1
Becker, D., Bereiter-Hahn, J., and Jendrach, M. (2009). Functional interaction of the cation channel transient receptor potential vanilloid 4 (TRPV4) and actin in volume regulation. Eur. J. Cell Biol. 88, 141–152. doi: 10.1016/j.ejcb.2008.10.002
Bezzerides, V. J., Ramsey, I. S., Kotecha, S., Greka, A., and Clapham, D. E. (2004). Rapid vesicular translocation and insertion of TRP channels. Nat. Cell Biol. 6, 709–720. doi: 10.1038/ncb1150
Bravo-Cordero, J. J., Hodgson, L., and Condeelis, J. (2012). Directed cell invasion and migration during metastasis. Curr. Opin. Cell Biol 24, 277–283. doi: 10.1016/j.ceb.2011.12.004
Broders-Bondon, F., Nguyen Ho-Bouldoires, T. H., Fernandez-Sanchez, M. E., and Farge, E. (2018). Mechanotransduction in tumor progression: the dark side of the force. J. Cell Biol. 217, 1571–1587. doi: 10.1083/jcb.201701039
Brundage, R. A., Fogarty, K. E., Tuft, R. A., and Fay, F. S. (1991). Calcium gradients underlying polarization and chemotaxis of eosinophils. Science 254, 703–706. doi: 10.1126/science.1948048
Burridge, K., and Wittchen, E. S. (2013). The tension mounts: stress fibers as force-generating mechanotransducers. J. Cell Biol. 200, 9–19. doi: 10.1083/jcb.201210090
Caceres, M., Ortiz, L., Recabarren, T., Romero, A., Colombo, A., Leiva-Salcedo, E., et al. (2015). TRPM4 is a novel component of the adhesome required for focal adhesion disassembly, migration and contractility. PLoS One 10:e0130540. doi: 10.1371/journal.pone.0130540
Chan, K. T., Bennin, D. A., and Huttenlocher, A. (2010). Regulation of adhesion dynamics by calpain-mediated proteolysis of focal adhesion kinase (FAK). J. Biol. Chem. 285, 11418–11426. doi: 10.1074/jbc.M109.090746
Chen, J. P., Luan, Y., You, C. X., Chen, X. H., Luo, R. C., and Li, R. (2010). TRPM7 regulates the migration of human nasopharyngeal carcinoma cell by mediating Ca(2+) influx. Cell Calcium 47, 425–432. doi: 10.1016/j.ceca.2010.03.003
Chen, J. P., Wang, J., Luan, Y., Wang, C. X., Li, W. H., Zhang, J. B., et al. (2015). TRPM7 promotes the metastatic process in human nasopharyngeal carcinoma. Cancer Lett. 356(2 Pt B), 483–490. doi: 10.1016/j.canlet.2014.09.032
Chen, Z., Tang, C., Zhu, Y., Xie, M., He, D., Pan, Q., et al. (2017a). TrpC5 regulates differentiation through the Ca2+/Wnt5a signalling pathway in colorectal cancer. Clin. Sci. 131, 227–237. doi: 10.1042/CS20160759
Chen, Z., Zhu, Y., Dong, Y., Zhang, P., Han, X., Jin, J., et al. (2017b). Overexpression of TrpC5 promotes tumor metastasis via the HIF-1alpha-Twist signaling pathway in colon cancer. Clin. Sci. 131, 2439–2450. doi: 10.1042/CS20171069
Choi, C. K., Vicente-Manzanares, M., Zareno, J., Whitmore, L. A., Mogilner, A., and Horwitz, A. R. (2008). Actin and alpha-actinin orchestrate the assembly and maturation of nascent adhesions in a myosin II motor-independent manner. Nat. Cell Biol. 10, 1039–1050. doi: 10.1038/ncb1763
Cioffi, D. L., Wu, S., and Stevens, T. (2003). On the endothelial cell I(SOC). Cell Calcium 33, 323–336. doi: 10.1016/s0143-4160(03)00046-0
Clapham, D. E. (2003). TRP channels as cellular sensors. Nature 426, 517–524. doi: 10.1038/nature02196
Clark, K., Middelbeek, J., Lasonder, E., Dulyaninova, N. G., Morrice, N. A., Ryazanov, A. G., et al. (2008). TRPM7 regulates myosin IIA filament stability and protein localization by heavy chain phosphorylation. J. Mol. Biol. 378, 790–803. doi: 10.1016/j.jmb.2008.02.057
del Rio, A., Perez-Jimenez, R., Liu, R., Roca-Cusachs, P., Fernandez, J. M., and Sheetz, M. P. (2009). Stretching single talin rod molecules activates vinculin binding. Science 323, 638–641. doi: 10.1126/science.1162912
Dovas, A., Yoneda, A., and Couchman, J. R. (2006). PKCbeta-dependent activation of RhoA by syndecan-4 during focal adhesion formation. J. Cell Sci. 119(Pt 13), 2837–2846. doi: 10.1242/jcs.03020
Dupont, S. (2016). Role of YAP/TAZ in cell-matrix adhesion-mediated signalling and mechanotransduction. Exp. Cell Res. 343, 42–53. doi: 10.1016/j.yexcr.2015.10.034
Eijkelkamp, N., Quick, K., and Wood, J. N. (2013). Transient receptor potential channels and mechanosensation. Annu. Rev. Neurosci. 36, 519–546. doi: 10.1146/annurev-neuro-062012-170412
Fabian, A., Fortmann, T., Dieterich, P., Riethmuller, C., Schon, P., Mally, S., et al. (2008). TRPC1 channels regulate directionality of migrating cells. Pflugers Arch. 457, 475–484. doi: 10.1007/s00424-008-0515-4
Formigli, L., Sassoli, C., Squecco, R., Bini, F., Martinesi, M., Chellini, F., et al. (2009). Regulation of transient receptor potential canonical channel 1 (TRPC1) by sphingosine 1-phosphate in C2C12 myoblasts and its relevance for a role of mechanotransduction in skeletal muscle differentiation. J. Cell Sci. 122(Pt 9), 1322–1333. doi: 10.1242/jcs.035402
Fox, P. D., Haberkorn, C. J., Akin, E. J., Seel, P. J., Krapf, D., and Tamkun, M. M. (2015). Induction of stable ER-plasma-membrane junctions by Kv2.1 potassium channels. J. Cell Sci. 128, 2096–2105. doi: 10.1242/jcs.166009
Friedl, P., and Wolf, K. (2003). Tumour-cell invasion and migration: diversity and escape mechanisms. Nat. Rev. Cancer 3, 362–374. doi: 10.1038/nrc1075
Gao, H., Wang, F., Wang, W., Makarewich, C. A., Zhang, H., Kubo, H., et al. (2012). Ca(2+) influx through L-type Ca(2+) channels and transient receptor potential channels activates pathological hypertrophy signaling. J. Mol. Cell Cardiol. 53, 657–667. doi: 10.1016/j.yjmcc.2012.08.005
Gao, S. L., Kong, C. Z., Zhang, Z., Li, Z. L., Bi, J. B., and Liu, X. K. (2017). TRPM7 is overexpressed in bladder cancer and promotes proliferation, migration, invasion and tumor growth. Oncol. Rep. 38, 1967–1976. doi: 10.3892/or.2017.5883
Garcia-Elias, A., Mrkonjic, S., Jung, C., Pardo-Pastor, C., Vicente, R., and Valverde, M. A. (2014). The TRPV4 channel. Handb. Exp. Pharmacol. 222, 293–319. doi: 10.1007/978-3-642-54215-2_12
Gardel, M. L., Schneider, I. C., Aratyn-Schaus, Y., and Waterman, C. M. (2010). Mechanical integration of actin and adhesion dynamics in cell migration. Annu. Rev. Cell Dev. Biol. 26, 315–333. doi: 10.1146/annurev.cellbio.011209.122036
Geng, L., Burrow, C. R., Li, H. P., and Wilson, P. D. (2000). Modification of the composition of polycystin-1 multiprotein complexes by calcium and tyrosine phosphorylation. Biochim. Biophys. Acta 1535, 21–35. doi: 10.1016/s0925-4439(00)00079-x
Giannone, G., Dubin-Thaler, B. J., Rossier, O., Cai, Y., Chaga, O., Jiang, G., et al. (2007). Lamellipodial actin mechanically links myosin activity with adhesion-site formation. Cell 128, 561–575. doi: 10.1016/j.cell.2006.12.039
Giannone, G., Rondé, P., Gaire, M., Beaudouin, J., Haiech, J., Ellenberg, J., et al. (2004). Calcium rises locally trigger focal adhesion disassembly and enhance residency of focal adhesion kinase at focal adhesions. J. Biol. Chem. 279, 28715–28723. doi: 10.1074/jbc.M404054200
Glading, A., Lauffenburger, D. A., and Wells, A. (2002). Cutting to the chase: calpain proteases in cell motility. Trends Cell Biol. 12, 46–54. doi: 10.1016/s0962-8924(01)02179-1
Goel, M., Sinkins, W., Keightley, A., Kinter, M., and Schilling, W. P. (2005). Proteomic analysis of TRPC5- and TRPC6-binding partners reveals interaction with the plasmalemmal Na(+)/K(+)-ATPase. Pflugers Arch. 451, 87–98. doi: 10.1007/s00424-005-1454-y
Gonzales, A. L., Yang, Y., Sullivan, M. N., Sanders, L., Dabertrand, F., Hill-Eubanks, D. C., et al. (2014). A PLCgamma1-dependent, force-sensitive signaling network in the myogenic constriction of cerebral arteries. Sci. Signal. 7:2004732. doi: 10.1126/scisignal.2004732
Gopal, S., Sogaard, P., Multhaupt, H. A., Pataki, C., Okina, E., Xian, X., et al. (2015). Transmembrane proteoglycans control stretch-activated channels to set cytosolic calcium levels. J. Cell Biol. 210, 1199–1211. doi: 10.1083/jcb.201501060
Goswami, C., Kuhn, J., Heppenstall, P. A., and Hucho, T. (2010). Importance of non-selective cation channel TRPV4 interaction with cytoskeleton and their reciprocal regulations in cultured cells. PLoS One 5:0011654. doi: 10.1371/journal.pone.0011654
Greka, A., Navarro, B., Oancea, E., Duggan, A., and Clapham, D. E. (2003). TRPC5 is a regulator of hippocampal neurite length and growth cone morphology. Nat. Neurosci. 6, 837–845. doi: 10.1038/nn1092
Guilbert, A., Gautier, M., Dhennin-Duthille, I., Haren, N., Sevestre, H., and Ouadid-Ahidouch, H. (2009). Evidence that TRPM7 is required for breast cancer cell proliferation. Am. J. Physiol. Cell Physiol. 297, C493–C502. doi: 10.1152/ajpcell.00624.2008
Guilbert, A., Gautier, M., Dhennin-Duthille, I., Rybarczyk, P., Sahni, J., Sevestre, H., et al. (2013). Transient receptor potential melastatin 7 is involved in oestrogen receptor-negative metastatic breast cancer cells migration through its kinase domain. Eur. J. Cancer 49, 3694–3707. doi: 10.1016/j.ejca.2013.07.008
Hahn, K., DeBiasio, R., and Taylor, D. L. (1992). Patterns of elevated free calcium and calmodulin activation in living cells. Nature 359, 736–738. doi: 10.1038/359736a0
Hall, A. (1998). Rho GTPases and the actin cytoskeleton. Science 279, 509–514. doi: 10.1126/science.279.5350.509
Hanahan, D., and Weinberg, R. A. (2011). Hallmarks of cancer: the next generation. Cell 144, 646–674. doi: 10.1016/j.cell.2011.02.013
Hicks, K., O’Neil, R. G., Dubinsky, W. S., and Brown, R. C. (2010). TRPC-mediated actin-myosin contraction is critical for BBB disruption following hypoxic stress. Am. J. Physiol. Cell Physiol. 298, C1583–C1593. doi: 10.1152/ajpcell.00458.2009
Horwitz, A., Duggan, K., Buck, C., Beckerle, M. C., and Burridge, K. (1986). Interaction of plasma membrane fibronectin receptor with talin–a transmembrane linkage. Nature 320, 531–533. doi: 10.1038/320531a0
Hynes, R. O. (1992). Integrins: versatility, modulation, and signaling in cell adhesion. Cell 69, 11–25. doi: 10.1016/0092-8674(92)90115-s
Jaalouk, D. E., and Lammerding, J. (2009). Mechanotransduction gone awry. Nat. Rev. Mol. Cell Biol. 10, 63–73. doi: 10.1038/nrm2597
Jiang, L., Ding, J., Tsai, H., Li, L., Feng, Q., Miao, J., et al. (2011). Over-expressing transient receptor potential cation channel 6 in podocytes induces cytoskeleton rearrangement through increases of intracellular Ca2+ and RhoA activation. Exp. Biol. Med. 236, 184–193. doi: 10.1258/ebm.2010.010237
Joly, D., Ishibe, S., Nickel, C., Yu, Z., Somlo, S., and Cantley, L. G. (2006). The polycystin 1-C-terminal fragment stimulates ERK-dependent spreading of renal epithelial cells. J. Biol. Chem. 281, 26329–26339. doi: 10.1074/jbc.m601373200
Kashio, M., and Tominaga, M. (2017). The TRPM2 channel: a thermo-sensitive metabolic sensor. Channels 11, 426–433. doi: 10.1080/19336950.2017.1344801
Kirmiz, M., Palacio, S., Thapa, P., King, A. N., Sack, J. T., and Trimmer, J. S. (2018). Remodeling neuronal ER-PM junctions is a conserved nonconducting function of Kv2 plasma membrane ion channels. Mol. Biol. Cell 29, 2410–2432. doi: 10.1091/mbc.E18-05-0337
Kopanska, K. S., Alcheikh, Y., Staneva, R., Vignjevic, D., and Betz, T. (2016). Tensile forces originating from cancer spheroids facilitate tumor invasion. PLoS One 11:e0156442. doi: 10.1371/journal.pone.0156442
Kuipers, A. J., Middelbeek, J., and van Leeuwen, F. N. (2012). Mechanoregulation of cytoskeletal dynamics by TRP channels. Eur. J. Cell Biol. 91, 834–846. doi: 10.1016/j.ejcb.2012.05.006
Kuwahara, K., Wang, Y., McAnally, J., Richardson, J. A., Bassel-Duby, R., Hill, J. A., et al. (2006). TRPC6 fulfills a calcineurin signaling circuit during pathologic cardiac remodeling. J. Clin. Invest. 116, 3114–3126. doi: 10.1172/jci27702
Lauffenburger, D. A., and Horwitz, A. F. (1996). Cell migration: a physically integrated molecular process. Cell 84, 359–369. doi: 10.1016/s0092-8674(00)81280-5
Laukaitis, C. M., Webb, D. J., Donais, K., and Horwitz, A. F. (2001). Differential dynamics of alpha 5 integrin, paxillin, and alpha-actinin during formation and disassembly of adhesions in migrating cells. J. Cell Biol. 153, 1427–1440. doi: 10.1083/jcb.153.7.1427
Launay, P., Cheng, H., Srivatsan, S., Penner, R., Fleig, A., and Kinet, J. P. (2004). TRPM4 regulates calcium oscillations after T cell activation. Science 306, 1374–1377. doi: 10.1126/science.1098845
Lawson, M. A., and Maxfield, F. R. (1995). Ca(2+)- and calcineurin-dependent recycling of an integrin to the front of migrating neutrophils. Nature 377, 75–79. doi: 10.1038/377075a0
Lee, W. H., Choong, L. Y., Mon, N. N., Lu, S., Lin, Q., Pang, B., et al. (2016). TRPV4 regulates breast cancer cell extravasation, stiffness and actin cortex. Sci. Rep. 6:27903. doi: 10.1038/srep27903
Li, F., Abuarab, N., and Sivaprasadarao, A. (2016). Reciprocal regulation of actin cytoskeleton remodelling and cell migration by Ca2+ and Zn2+: role of TRPM2 channels. J. Cell Sci. 129, 2016–2029. doi: 10.1242/jcs.179796
Liu, C., and Montell, C. (2015). Forcing open TRP channels: mechanical gating as a unifying activation mechanism. Biochem. Biophys. Res. Commun. 460, 22–25. doi: 10.1016/j.bbrc.2015.02.067
Locascio, A., and Nieto, M. A. (2001). Cell movements during vertebrate development: integrated tissue behaviour versus individual cell migration. Curr. Opin. Genet. Dev. 11, 464–469. doi: 10.1016/s0959-437x(00)00218-5
Louis, M., Zanou, N., Van Schoor, M., and Gailly, P. (2008). TRPC1 regulates skeletal myoblast migration and differentiation. J. Cell Sci. 121(Pt 23), 3951–3959. doi: 10.1242/jcs.037218
Luster, A. D., Alon, R., and von Andrian, U. H. (2005). Immune cell migration in inflammation: present and future therapeutic targets. Nat. Immunol. 6, 1182–1190. doi: 10.1038/ni1275
Marshall, K. L., and Lumpkin, E. A. (2012). The molecular basis of mechanosensory transduction. Adv. Exp. Med. Biol. 739, 142–155. doi: 10.1007/978-1-4614-1704-0_9
Matthews, B. D., Thodeti, C. K., Tytell, J. D., Mammoto, A., Overby, D. R., and Ingber, D. E. (2010). Ultra-rapid activation of TRPV4 ion channels by mechanical forces applied to cell surface beta1 integrins. Integr. Biol. 2, 435–442. doi: 10.1039/c0ib00034e
Mattila, P. K., and Lappalainen, P. (2008). Filopodia: molecular architecture and cellular functions. Nat. Rev. Mol. Cell Biol. 9, 446–454. doi: 10.1038/nrm2406
Meng, X., Cai, C., Wu, J., Cai, S., Ye, C., Chen, H., et al. (2013). TRPM7 mediates breast cancer cell migration and invasion through the MAPK pathway. Cancer Lett. 333, 96–102. doi: 10.1016/j.canlet.2013.01.031
Middelbeek, J., Kuipers, A. J., Henneman, L., Visser, D., Eidhof, I., van Horssen, R., et al. (2012). TRPM7 is required for breast tumor cell metastasis. Cancer Res. 72, 4250–4261. doi: 10.1158/0008-5472.can-11-3863
Mihara, H., Boudaka, A., Shibasaki, K., Yamanaka, A., Sugiyama, T., and Tominaga, M. (2010). Involvement of TRPV2 activation in intestinal movement through nitric oxide production in mice. J. Neurosci. 30, 16536–16544. doi: 10.1523/JNEUROSCI.4426-10.2010
Monet, M., Gkika, D., Lehen’kyi, V., Pourtier, A., Vanden Abeele, F., Bidaux, G., et al. (2009). Lysophospholipids stimulate prostate cancer cell migration via TRPV2 channel activation. Biochim. Biophys. Acta 1793, 528–539. doi: 10.1016/j.bbamcr.2009.01.003
Monet, M., Lehen’kyi, V., Gackiere, F., Firlej, V., Vandenberghe, M., Roudbaraki, M., et al. (2010). Role of cationic channel TRPV2 in promoting prostate cancer migration and progression to androgen resistance. Cancer Res. 70, 1225–1235. doi: 10.1158/0008-5472.CAN-09-2205
Moroishi, T., Hansen, C. G., and Guan, K. L. (2015). The emerging roles of YAP and TAZ in cancer. Nat. Rev. Cancer 15, 73–79. doi: 10.1038/nrc3876
Mrkonjic, S., Garcia-Elias, A., Pardo-Pastor, C., Bazellieres, E., Trepat, X., Vriens, J., et al. (2015). TRPV4 participates in the establishment of trailing adhesions and directional persistence of migrating cells. Pflugers Arch. 467, 2107–2119. doi: 10.1007/s00424-014-1679-8
Nakayama, H., Wilkin, B. J., Bodi, I., and Molkentin, J. D. (2006). Calcineurin-dependent cardiomyopathy is activated by TRPC in the adult mouse heart. FASEB J. 20, 1660–1670. doi: 10.1096/fj.05-5560com
Nilius, B., and Owsianik, G. (2011). The transient receptor potential family of ion channels. Genome Biol. 12, 2011–2012.
Nilius, B., Owsianik, G., and Voets, T. (2008). Transient receptor potential channels meet phosphoinositides. EMBO J. 27, 2809–2816. doi: 10.1038/emboj.2008.217
Numaga, T., Nishida, M., Kiyonaka, S., Kato, K., Katano, M., Mori, E., et al. (2010). Ca2+ influx and protein scaffolding via TRPC3 sustain PKCbeta and ERK activation in B cells. J. Cell Sci. 123(Pt 6), 927–938. doi: 10.1242/jcs.061051
Onohara, N., Nishida, M., Inoue, R., Kobayashi, H., Sumimoto, H., Sato, Y., et al. (2006). TRPC3 and TRPC6 are essential for angiotensin II-induced cardiac hypertrophy. EMBO J. 25, 5305–5316. doi: 10.1038/sj.emboj.7601417
Otsuguro, K.-I., Tang, J., Tang, Y., Xiao, R., Freichel, M., Tsvilovskyy, V., et al. (2008). Isoform-specific inhibition of TRPC4 channel by phosphatidylinositol 4,5-bisphosphate. J. Biol. Chem. 283, 10026–10036. doi: 10.1074/jbc.M707306200
Oulidi, A., Bokhobza, A., Gkika, D., Vanden Abeele, F., Lehen’kyi, V., Ouafik, L., et al. (2013). TRPV2 mediates adrenomedullin stimulation of prostate and urothelial cancer cell adhesion, migration and invasion. PLoS One 8:e64885. doi: 10.1371/journal.pone.0064885
Piccolo, S., Dupont, S., and Cordenonsi, M. (2014). The biology of YAP/TAZ: hippo signaling and beyond. Physiol. Rev. 94, 1287–1312. doi: 10.1152/physrev.00005.2014
Pollard, T. D., and Cooper, J. A. (2009). Actin, a central player in cell shape and movement. Science 326, 1208–1212. doi: 10.1126/science.1175862
Price, L. S., Langeslag, M., ten Klooster, J. P., Hordijk, P. L., Jalink, K., and Collard, J. G. (2003). Calcium signaling regulates translocation and activation of Rac. J. Biol. Chem. 278, 39413–39421. doi: 10.1074/jbc.m302083200
Rahaman, S. O., Grove, L. M., Paruchuri, S., Southern, B. D., Abraham, S., Niese, K. A., et al. (2014). TRPV4 mediates myofibroblast differentiation and pulmonary fibrosis in mice. J. Clin. Invest. 124, 5225–5238. doi: 10.1172/JCI75331
Reczek, D., Berryman, M., and Bretscher, A. (1997). Identification of EBP50: a PDZ-containing phosphoprotein that associates with members of the ezrin-radixin-moesin family. J. Cell Biol. 139, 169–179. doi: 10.1083/jcb.139.1.169
Retailleau, K., and Duprat, F. (2014). Polycystins and partners: proposed role in mechanosensitivity. J. Physiol. 592, 2453–2471. doi: 10.1113/jphysiol.2014.271346
Ridley, A. J., and Hall, A. (1992). The small GTP-binding protein rho regulates the assembly of focal adhesions and actin stress fibers in response to growth factors. Cell 70, 389–399. doi: 10.1016/0092-8674(92)90163-7
Ridley, A. J., Schwartz, M. A., Burridge, K., Firtel, R. A., Ginsberg, M. H., Borisy, G., et al. (2003). Cell migration: integrating signals from front to back. Science 302, 1704–1709. doi: 10.1126/science.1092053
Rybarczyk, P., Vanlaeys, A., Brassart, B., Dhennin-Duthille, I., Chatelain, D., Sevestre, H., et al. (2017). The transient receptor potential melastatin 7 channel regulates pancreatic cancer cell invasion through the Hsp90alpha/uPA/MMP2 pathway. Neoplasia 19, 288–300. doi: 10.1016/j.neo.2017.01.004
Rychkov, G., and Barritt, G. J. (2007). TRPC1 Ca(2+)-permeable channels in animal cells. Handb. Exp. Pharmacol. 179, 23–52. doi: 10.1007/978-3-540-34891-7_2
Ryskamp, D. A., Frye, A. M., Phuong, T. T., Yarishkin, O., Jo, A. O., Xu, Y., et al. (2016). TRPV4 regulates calcium homeostasis, cytoskeletal remodeling, conventional outflow and intraocular pressure in the mammalian eye. Sci. Rep. 6:30583. doi: 10.1038/srep30583
Schwab, A., Fabian, A., Hanley, P. J., and Stock, C. (2012). Role of ion channels and transporters in cell migration. Physiol. Rev. 92, 1865–1913. doi: 10.1152/physrev.00018.2011
Sharif-Naeini, R., Dedman, A., Folgering, J. H., Duprat, F., Patel, A., Nilius, B., et al. (2008). TRP channels and mechanosensory transduction: insights into the arterial myogenic response. Pflugers Arch. 456, 529–540. doi: 10.1007/s00424-007-0432-y
Shaw, T. J., and Martin, P. (2016). Wound repair: a showcase for cell plasticity and migration. Curr. Opin. Cell Biol. 42, 29–37. doi: 10.1016/j.ceb.2016.04.001
Shibasaki, K. (2016). Physiological significance of TRPV2 as a mechanosensor, thermosensor and lipid sensor. J. Physiol. Sci. 66, 359–365. doi: 10.1007/s12576-016-0434-7
Shibasaki, K., Murayama, N., Ono, K., Ishizaki, Y., and Tominaga, M. (2010). TRPV2 enhances axon outgrowth through its activation by membrane stretch in developing sensory and motor neurons. J. Neurosci. 30, 4601–4612. doi: 10.1523/JNEUROSCI.5830-09.2010
Shin, S. H., Lee, E. J., Hyun, S., Chun, J., Kim, Y., and Kang, S. S. (2012). Phosphorylation on the Ser 824 residue of TRPV4 prefers to bind with F-actin than with microtubules to expand the cell surface area. Cell. Signal. 24, 641–651. doi: 10.1016/j.cellsig.2011.11.002
Siegel, R., Ward, E., Brawley, O., and Jemal, A. (2011). Cancer statistics, 2011: the impact of eliminating socioeconomic and racial disparities on premature cancer deaths. CA Cancer J. Clin. 61, 212–236. doi: 10.3322/caac.20121
Singer, I. I. (1982). Association of fibronectin and vinculin with focal contacts and stress fibers in stationary hamster fibroblasts. J. Cell Biol. 92, 398–408. doi: 10.1083/jcb.92.2.398
Singh, I., Knezevic, N., Ahmmed, G. U., Kini, V., Malik, A. B., and Mehta, D. (2007). Galphaq-TRPC6-mediated Ca2+ entry induces RhoA activation and resultant endothelial cell shape change in response to thrombin. J. Biol. Chem. 282, 7833–7843. doi: 10.1074/jbc.M608288200
Small, J. V., Stradal, T., Vignal, E., and Rottner, K. (2002). The lamellipodium: where motility begins. Trends Cell Biol. 12, 112–120. doi: 10.1016/s0962-8924(01)02237-1
Smani, T., Dionisio, N., López, J. J., Berna-Erro, A., and Rosado, J. A. (2014). Cytoskeletal and scaffolding proteins as structural and functional determinants of TRP channels. Biochim. Biophys. Acta Biomemb. 1838, 658–664. doi: 10.1016/j.bbamem.2013.01.009
Su, L. T., Agapito, M. A., Li, M., Simonson, W. T., Huttenlocher, A., Habas, R., et al. (2006). TRPM7 regulates cell adhesion by controlling the calcium-dependent protease calpain. J. Biol. Chem. 281, 11260–11270. doi: 10.1074/jbc.m512885200
Su, L. T., Liu, W., Chen, H. C., Gonzalez-Pagan, O., Habas, R., and Runnels, L. W. (2011). TRPM7 regulates polarized cell movements. Biochem. J. 434, 513–521. doi: 10.1042/BJ20101678
Sugio, S., Nagasawa, M., Kojima, I., Ishizaki, Y., and Shibasaki, K. (2017). Transient receptor potential vanilloid 2 activation by focal mechanical stimulation requires interaction with the actin cytoskeleton and enhances growth cone motility. FASEB J. 31, 1368–1381. doi: 10.1096/fj.201600686RR
Sun, Z., Guo, S. S., and Fassler, R. (2016). Integrin-mediated mechanotransduction. J. Cell Biol. 215, 445–456. doi: 10.1083/jcb.201609037
Suzuki, M., Hirao, A., and Mizuno, A. (2003). Microtubule-associated [corrected] protein 7 increases the membrane expression of transient receptor potential vanilloid 4 (TRPV4). J. Biol. Chem. 278, 51448–51453. doi: 10.1074/jbc.M308212200
Talmadge, J. E., and Fidler, I. J. (2010). AACR centennial series: the biology of cancer metastasis: historical perspective. Cancer Res. 70, 5649–5669. doi: 10.1158/0008-5472.CAN-10-1040
Tang, D. D., and Gerlach, B. D. (2017). The roles and regulation of the actin cytoskeleton, intermediate filaments and microtubules in smooth muscle cell migration. Respir. Res. 18, 017–0544.
Tang, Y., Tang, J., Chen, Z., Trost, C., Flockerzi, V., Li, M., et al. (2000). Association of mammalian trp4 and phospholipase C isozymes with a PDZ domain-containing protein. NHERF. J. Biol. Chem. 275, 37559–37564. doi: 10.1074/jbc.m006635200
Tapp, H., Al-Naggar, I. M., Yarmola, E. G., Harrison, A., Shaw, G., Edison, A. S., et al. (2005). MARCKS is a natively unfolded protein with an inaccessible actin-binding site: evidence for long-range intramolecular interactions. J. Biol. Chem. 280, 9946–9956. doi: 10.1074/jbc.M414614200
Tian, D., Jacobo, S. M. P., Billing, D., Rozkalne, A., Gage, S. D., Anagnostou, T., et al. (2010). Antagonistic regulation of actin dynamics and cell motility by TRPC5 and TRPC6 channels. Sci. Signal. 3:ra77. doi: 10.1126/scisignal.2001200
Tsai, F. C., and Meyer, T. (2012). Ca2+ pulses control local cycles of lamellipodia retraction and adhesion along the front of migrating cells. Curr. Biol. 22, 837–842. doi: 10.1016/j.cub.2012.03.037
Vennekens, R., and Nilius, B. (2007). Insights into TRPM4 function, regulation and physiological role. Handb. Exp. Pharmacol. 179, 269–285. doi: 10.1007/978-3-540-34891-7_16
Visser, D., Middelbeek, J., van Leeuwen, F. N., and Jalink, K. (2014). Function and regulation of the channel-kinase TRPM7 in health and disease. Eur. J. Cell Biol. 93, 455–465. doi: 10.1016/j.ejcb.2014.07.001
Vogel, V., and Sheetz, M. (2006). Local force and geometry sensing regulate cell functions. Nat. Rev. Mol. Cell Biol. 7, 265–275. doi: 10.1038/nrm1890
Weber, K. S., Hildner, K., Murphy, K. M., and Allen, P. M. (2010). Trpm4 differentially regulates Th1 and Th2 function by altering calcium signaling and NFAT localization. J. Immunol. 185, 2836–2846. doi: 10.4049/jimmunol.1000880
Wehrle-Haller, B., and Imhof, B. (2002). The inner lives of focal adhesions. Trends Cell Biol. 12, 382–389. doi: 10.1016/s0962-8924(02)02321-8
Wei, C., Wang, X., Chen, M., Ouyang, K., Song, L. S., and Cheng, H. (2009). Calcium flickers steer cell migration. Nature 457, 901–905. doi: 10.1038/nature07577
Wei, C., Wang, X., Zheng, M., and Cheng, H. (2012). Calcium gradients underlying cell migration. Curr. Opin. Cell Biol. 24, 254–261. doi: 10.1016/j.ceb.2011.12.002
Wei, S. C., and Yang, J. (2016). Forcing through tumor metastasis: the interplay between tissue rigidity and epithelial-mesenchymal transition. Trends Cell Biol. 26, 111–120. doi: 10.1016/j.tcb.2015.09.009
Wilson, P. D., Geng, L., Li, X., and Burrow, C. R. (1999). The PKD1 gene product, “polycystin-1,” is a tyrosine-phosphorylated protein that colocalizes with alpha2beta1-integrin in focal clusters in adherent renal epithelia. Lab. Invest. 79, 1311–1323.
Winograd-Katz, S. E., Fassler, R., Geiger, B., and Legate, K. R. (2014). The integrin adhesome: from genes and proteins to human disease. Nat. Rev. Mol. Cell Biol. 15, 273–288. doi: 10.1038/nrm3769
Wozniak, M. A., and Chen, C. S. (2009). Mechanotransduction in development: a growing role for contractility. Nat. Rev. Mol. Cell Biol. 10, 34–43. doi: 10.1038/nrm2592
Xu, S. Z., Muraki, K., Zeng, F., Li, J., Sukumar, P., Shah, S., et al. (2006). A sphingosine-1-phosphate-activated calcium channel controlling vascular smooth muscle cell motility. Circ. Res. 98, 1381–1389. doi: 10.1161/01.res.0000225284.36490.a2
Yee, N. S., Kazi, A. A., Li, Q., Yang, Z., Berg, A., and Yee, R. K. (2015). Aberrant over-expression of TRPM7 ion channels in pancreatic cancer: required for cancer cell invasion and implicated in tumor growth and metastasis. Biol. Open 4, 507–514. doi: 10.1242/bio.20137088
Yin, J., and Kuebler, W. M. (2010). Mechanotransduction by TRP channels: general concepts and specific role in the vasculature. Cell Biochem. Biophys. 56, 1–18. doi: 10.1007/s12013-009-9067-2
Zaidel-Bar, R., Ballestrem, C., Kam, Z., and Geiger, B. (2003). Early molecular events in the assembly of matrix adhesions at the leading edge of migrating cells. J. Cell Sci. 116(Pt 22), 4605–4613. doi: 10.1242/jcs.00792
Zhong, C., Chrzanowska-Wodnicka, M., Brown, J., Shaub, A., Belkin, A. M., and Burridge, K. (1998). Rho-mediated contractility exposes a cryptic site in fibronectin and induces fibronectin matrix assembly. J. Cell Biol. 141, 539–551. doi: 10.1083/jcb.141.2.539
Keywords: TRP channels, mechanotransduction, focal adhesion, actin cytoskeletal remodeling, cancer
Citation: Canales J, Morales D, Blanco C, Rivas J, Díaz N, Angelopoulos I and Cerda O (2019) A TR(i)P to Cell Migration: New Roles of TRP Channels in Mechanotransduction and Cancer. Front. Physiol. 10:757. doi: 10.3389/fphys.2019.00757
Received: 18 February 2019; Accepted: 31 May 2019;
Published: 18 June 2019.
Edited by:
Christoph Fahlke, Julich Research Centre, GermanyReviewed by:
Ian Scott Ramsey, Virginia Commonwealth University School of Medicine, United StatesAndreas H. Guse, University Medical Center Hamburg-Eppendorf, Germany
Copyright © 2019 Canales, Morales, Blanco, Rivas, Díaz, Angelopoulos and Cerda. This is an open-access article distributed under the terms of the Creative Commons Attribution License (CC BY). The use, distribution or reproduction in other forums is permitted, provided the original author(s) and the copyright owner(s) are credited and that the original publication in this journal is cited, in accordance with accepted academic practice. No use, distribution or reproduction is permitted which does not comply with these terms.
*Correspondence: Jimena Canales, amltZW5hY2FuYWxlc0BtZWQudWNoaWxlLmNs; Oscar Cerda, b3NjYXJjZXJkYUB1Y2hpbGUuY2w=