- 1Department of Entomology, Max Planck Institute for Chemical Ecology, Jena, Germany
- 2Department of Biochemistry, Max Planck Institute for Chemical Ecology, Jena, Germany
Many protein families harbor pseudoenzymes that have lost the catalytic function of their enzymatically active counterparts. Assigning alternative function and importance to these proteins is challenging. Because the evolution toward pseudoenzymes is driven by gene duplication, they often accumulate in multigene families. Plant cell wall-degrading enzymes (PCWDEs) are prominent examples of expanded gene families. The pectolytic glycoside hydrolase family 28 (GH28) allows herbivorous insects to break down the PCW polysaccharide pectin. GH28 in the Phytophaga clade of beetles contains many active enzymes but also many inactive counterparts. Using functional characterization, gene silencing, global transcriptome analyses, and recordings of life history traits, we found that not only catalytically active but also inactive GH28 proteins are part of the same pectin-digesting pathway. The robustness and plasticity of this pathway and thus its importance for the beetle is supported by extremely high steady-state expression levels and counter-regulatory mechanisms. Unexpectedly, the impact of pseudoenzymes on the pectin-digesting pathway in Phytophaga beetles exceeds even the influence of their active counterparts, such as a lowered efficiency of food-to-energy conversion and a prolongation of the developmental period.
Introduction
Though plants contain all the nutrients herbivorous insects need, dependence on plants as a food source is challenging for two reasons: nutrient amounts and ratios are highly variable, and nutrient requirements are not uniform over an insect’s life cycle (Schoonhoven et al., 2005). Because a large proportion of ingested food consists of macromolecules – proteins and polysaccharides – which can be limited in a plant diet, herbivorous insects have adapted to this scarcity by evolving specific digestive capacities (Mattson, 1980; Lehane and Billingsley, 1996; Chapman, 1998). The intake of these macromolecules is regulated (Behmer, 2009) before their degradation by hydrolases to release amino acids and sugars that can be absorbed and used by insects as sources of nitrogen and metabolic energy, respectively.
Starch, the main storage polysaccharide in plants, is of great importance as the source of energy, which fuels insect growth and development. This polysaccharide can be easily digested by amylases, which are widespread in herbivorous insects (Kaur et al., 2014). In addition to starch, plant cell wall (PCW) polysaccharides are major carbohydrate constituents of green plants and can make up half of a leaf’s dry weight (Anand et al., 2010). Every growing plant cell is encased in a primary wall that is composed of approximately 90% polysaccharides (McNeil et al., 1984). PCW polysaccharides include cellulose and hemicellulose fibers that are further embedded in a pectin polysaccharide matrix (Schols et al., 2009). This carbohydrate network can be broken down by plant cell wall-degrading enzymes (PCWDEs), which mainly belong to different families of carbohydrate esterases, polysaccharide lyases and glycoside hydrolases (Gilbert, 2010). For a long time, indirect evidence related to the activity of insects’ endogenous PCWDEs has accumulated, but despite more recent attempts to clone and characterize these enzymes (Calderon-Cortes et al., 2012), little is known about their relevance in herbivorous insects with respect to nutrient acquisition.
In contrast to the more limited information available for their role in insects, PCWDEs have been extensively studied in plant-pathogenic microbes (Walton, 1994; Kubicek et al., 2014), in particular, pectin-degrading polygalacturonases (PGs), which belong to the glycoside hydrolase family 28 (GH28). Genes encoding GH28 PGs are widespread in plant pathogens (Reignault et al., 2008), and they were present in the most recent common ancestor of fungi, which illustrates their primary importance at the pathogen-plant interface (Chang et al., 2015). PGs have been shown to be key players during plant infestation by these microbes, as (i) they are the first enzymes secreted to weaken the plant cell wall and (ii) they are important virulence factors (De Lorenzo and Ferrari, 2002; Kars et al., 2005; Lagaert et al., 2009; Sprockett et al., 2011).
More recently, endogenous – and apparently widespread – PG encoding genes were identified in a number of herbivorous insect orders, including Hemiptera (mirid bugs), Phasmatodea (stick insects), Hymenoptera (gall wasps) and Coleoptera (mainly “Phytophaga”: see below) (Allen and Mertens, 2008; Celorio-Mancera et al., 2008; Pauchet et al., 2010; Hearn, 2013; Kirsch et al., 2014; McKenna et al., 2016; Shelomi et al., 2016). Functional characterization in vitro revealed that the corresponding proteins were active PGs that hydrolyze the homogalacturonan pectin backbone synergistically, releasing galacturonic acid oligomers and monomers (Kirsch et al., 2014, 2016; McKenna et al., 2016; Shelomi et al., 2016). However, in beetles, inactive GH28s were identified that cannot bind their predicted pectin substrate due to amino acid substitutions at crucial positions (Kirsch et al., 2014, 2016). Despite this, all GH28 family members of beetles and stick insects were shown to be specifically expressed in gut tissue, with the corresponding enzymes being secreted into the gut lumen (Shen et al., 2003; Kirsch et al., 2012, 2016; Shelomi et al., 2014; Evangelista et al., 2015). PGs of mirid bugs are expressed in salivary glands and are injected into the plant during piercing (Shackel et al., 2005; Celorio-Mancera et al., 2008). Taken together, these factors strongly indicate a digestive function for PGs and their central role in pectin hydrolysis in vivo.
Remarkably, insect genes encoding PGs seemed to have been acquired by horizontal gene transfer (HGT) (Kirsch et al., 2014, 2016; Shelomi et al., 2016). Especially in the “Phytophaga” beetles – the hyper-diverse beetle clade that includes weevils, longhorned beetles and leaf beetles (Marvaldi et al., 2009) – several rounds of loss and replacement have affected the evolutionary history of the beetle GH28 gene family (Kirsch et al., 2014). PGs persisted after the initial HGT early in the evolution of “Phytophaga” beetles, and their genes have duplicated and are under continued action of purifying selection while the corresponding proteins have functionally diversified. These facts strongly indicate that an important function of PGs is to promote herbivory in this clade of beetles, which represents about 50% of all herbivorous insects (Strong et al., 1984).
Additionally, symbionts of herbivorous insects can encode for pectinase activity (Calderon-Cortes et al., 2012; Engel et al., 2012). A remarkable example shows how a “Phytophaga” beetle host benefits from a symbiont’s pectolytic activity (Salem et al., 2017): when the pectinase-encoding symbiont is removed from its Cassida rubiginosa leaf beetle host, insect survival is highly reduced. The symbiont most likely compensates for the loss of the Cassida endogenous PG genes, and host survival depends on pectin digestion facilitated by the symbiont. The lack of beetle PGs in the Phytophaga clade, as in Cassida, is so far unusual. However, this system clearly exemplifies the importance of a single PCWDE family for the fitness of C. rubiginosa beetles.
To directly test the biological relevance of the endogenous pectin-degrading ability of an insect, we analyze the effects of gene silencing on (i) the performance of a leaf beetle, (ii) the enzymatic activities and (iii) the global gene expression. We simultaneously silenced the three endo-PGs of the mustard leaf beetle Phaedon cochleariae, and, in another RNAi treatment, three GH28 family members that had most likely lost their PG enzymatic activity (Kirsch et al., 2014). This simultaneous knockdown, enabled us to test whether inactive GH28 family members continue to play a role in pectin hydrolysis, their ancestral function, even if they are not hydrolyzing polygalacturonan. Gene silencing allows us to study the significance of active enzymes and their pseudoenzyme counterparts and to understand why they became inactive toward a substrate during evolution while still under strong purifying selection (Kirsch et al., 2014).
Materials and Methods
Insect and Plant Rearing
Phaedon cochleariae was reared in the laboratory (15°C, long day conditions, 16-h/8-h light/dark period) on Chinese cabbage (Brassica rapa ssp. pekinensis) leaves for several generations. Larvae used for RNA interference (RNAi) experiments stemmed from an over-night egg laying of mass-reared adults. Egg-containing leaves were separated, and emerging larvae were fed with Chinese cabbage until injected with double-stranded RNA. Cabbage plants used for bioassays (B. rapa ssp. pekinensis var. Cantonner Witkropp) were reared in the greenhouse (21°C, 55% humidity, long day conditions, 14-h/10-h light/dark period), and larvae were fed with middle-aged leaves from 6- to 8-week-old non-flowering plants.
Heterologous Expression and Enzymatic Assays
Sf9 insect cells were cultivated in GIBCO Sf-900 II SFM (Thermo Fisher Scientific, MA, United States) on 6-well plates at 27°C until 70–90% confluence was achieved. Transfection was performed with FuGENE® HD (Promega, WI, United States) following the manufacturer’s protocol using the GH28 pIB/V5-His TOPO TA (Thermo Fisher Scientific, MA, United States) constructs described previously (Kirsch et al., 2014). At 72 h after transfection, the culture medium of Sf9 cells was harvested and concentrated 10-fold using Pierce Concentrators 20 ml with a 20 kDa cutoff (Thermo Fisher Scientific, MA, United States). Culture medium was further dialyzed against water at 4°C for 48 h using Slide-A-Lyzer Dialysis Cassettes with a 10 kDa cutoff, followed by desalting with Zeba Desalt Spin Columns with a 7 kDa cutoff (both Thermo Fisher Scientific, MA, United States) according to the manufacturer’s guidelines. The crude protein extract of transient heterologously expressed GH28 family members was used for Western blot analyses and enzyme assays. Expressed proteins were detected by Western blots using an anti-V5 HRP antibody (Thermo Fisher Scientific, MA, United States) and the SuperSignal West HisProbe Kit (Thermo Fisher Scientific, MA, United States).
For qualitative analysis of breakdown products of the beetle GH28 family members by thin layer chromatography (TLC), 16 μl of a 2.5% (w/v) PCW suspension was added to 20 μl of crude recombinant protein extract and 4 μl citrate-phosphate buffer pH 5.0 to a final concentration of 20 mM. In the negative control (−), GH28 was substituted with 20 μl of water. Assays were analyzed as previously described (Kirsch et al., 2014). Chinese cabbage PCW substrate was prepared after PCW enrichment and protein extraction as described for Arabidopsis hypocotyls (Feiz et al., 2006). Approximately 32 g of Brassica rapa ssp. pekinensis leaves (fresh weight) were ground in 0.4 mM sucrose in 5 mM sodium acetate pH 4.6 for 15 min in a blender at full speed at 4°C. Approximately 1 ml of Protease Inhibitor Cocktail (for plant cell and tissue extracts; Sigma-Aldrich, Germany) was added per 30 g of plant material. After 30 min of incubation while stirring at 4°C, the mixture was centrifuged (15 min, 4000 × g, 4°C) to separate the cell walls from soluble cytoplasmic components. The supernatant was discarded and the pellet resuspended in 0.6 mM sucrose in 5 mM sodium acetate pH 4.6, centrifuged again and the resulting supernatant was discarded. The procedure was repeated with 1 mM sucrose in 5 mM sodium acetate pH 4.6. The pellet was washed with 3 l of pre-cooled 5 mM sodium acetate buffer, pH 4.6 on Miracloth (Merk Millipore, Germany) filtration material (pore size 22–25 μm). The cell wall material was ground in liquid nitrogen in a mortar and lyophilized afterward. Cell wall-associated proteins were extracted from the lyophilized cell wall samples in two steps. 0.65 g of dry material was resuspended in 25 ml of 0.2 mM calcium chloride in 5 mM sodium acetate pH 4.6 by vortexing at room temperature for 10 min and pelleted (15 min, 4000 × g, 4°C). The pellet was extracted the same way with 1.0 mM sodium chloride in 5 mM sodium acetate pH 4.6. After the protein supernatant was separated from the PCW pellet, the lyophilized and re-suspended pellet was used for enzymatic assays. The presence of degradable pectin and cellulose was verified by analyzing the breakdown products obtained after incubating the plant cell wall preparation with commercially available pectinase and cellulase mixtures as well as with gut extracts from P. cochleariae (Supplementary Figure S1).
RNA Interference
Double-stranded RNAs of PCO-GH28-1, 3, 5, 6, 7, and 9 were prepared using the MEGAscript RNAi Kit (Thermo Fisher Scientific, MA, United States) according to the manufacturer’s instructions. Templates for synthesis were amplified from the corresponding expression vectors (pIBV5) from a previous study (Kirsch et al., 2014) using primers with overhangs containing the minimum T7 polymerase promotor sequence needed for transcription (Supplementary Table S1). Off-target effects were predicted by searching all possible 21-mers of both RNA strands against our in-house P. cochleariae transcriptome database, allowing for one mismatch. Five-day-old larvae (early second instar) were weighed before injection, and only those weighing 1.1–1.4 mg were used to ensure high survivorship (determines the lower limit) and at the same time a maximum of days in the larval stage (determines the upper limit). Larvae were immobilized on sticky tape and injected with 100 nl containing 100 ng of dsRNA of each GH28 as a pool or 300 ng of dsRNA of GFP using a Nano2010 injector (World Precision Instruments, FL, United States) oriented with a manual micromanipulator. Injected and non-injected larvae were kept in clear ventilated plastic boxes (20 × 20 × 6 cm) containing a moistened tissue and cabbage leaves as food under standard rearing conditions.
Monitoring Larval Development and Food Consumption
One day post injection, larvae were weighed and transferred individually [n = 50 for each treatment: GH28-active (28a), GH28-inactive (28i), GFP injection control] to Petri dishes (diameter: 60 mm) in order to document food consumption and development. Petri dishes, equipped with a leaf disc [diameter: 18 mm (day 1–4 pi), 20 mm (day 4–6 pi), 22 mm (day 6–7 post injection)] on a filter paper moistened with 100 μl sterile water, were sealed with Parafilm M to prevent desiccation and kept under standard rearing conditions. Leaf discs were changed every day and food was available ad libitum. Larval weight was recorded on days 1 and 5 post injection to calculate larval weight gain. Leaf discs were photographed every day to calculate food consumption (cm2 leaf area) by image analysis (Schindelin et al., 2012).
Sample Preparation
Five days post injection, larvae were dissected for gene expression analysis and enzymatic assays. Dissection was executed in 20 mM citrate/phosphate buffer pH 5.0 containing a cocktail of protease inhibitors (Complete EDTA-free, Roche, Germany). Intact whole guts were transferred in 200 μl of the same buffer chilled on ice, opened on one side and soaked in the buffer. The resulting buffer/gut content mixture was kept on ice during gut dissection and immediately centrifuged afterward (5,000 g, 5 min, 4°C). The supernatant was collected and stored at −20°C until use. The remaining gut tissue was transferred to 450 μl RL buffer of the RNA extraction kit and frozen at −20°C. Five replicates per treatment of four larval gut tissues and gut content each were taken for downstream analyses of gene expression and PG activity.
Expression Analyses
To compare gene expression in larvae injected with dsRNA targeting the active and inactive GH28 family members with GFP control, reverse transcription quantitative PCR (RT-qPCR) was performed. Each assay was set up in two technical replicates for each of the five biological replicates. As P. cochleariae has nine PG family members (Kirsch et al., 2014), we included both the silenced and the non-silenced ones in our analyses. RNA extraction was performed using the innuPrep DNA/RNA Mini kit (Analytik, Jena, Germany) following the manufacturer’s instructions. After RNA integrity on a 1% agarose gel was checked, 500 ng of total RNA from each pool was reverse-transcribed with a 3:1 mix of random and oligo-dT20 primers. RT-qPCR was performed in optical 96-well plates on a CFX Connect detection system (BioRad, CA, United States). All steps were performed with the Verso 2-Step QRT-PCR Kit (SYBR Green + Separate ROX Vial; Thermo Fisher Scientific, MA, United States) following the manufacturer’s instructions. The PCR program was as follows: 95°C for 15 min, then 40 cycles at 95°C for 15 s, 58°C for 30 s, and 72°C for 30 s, and afterward a melt cycle from 55 to 95°C in 0.5-s increments. All primers were designed using Primer3 (version 0.4.0) and are listed in Supplementary Table S1. Specific amplification of each transcript was verified by dissociation curve analysis. A standard curve for each primer pair was determined in the CFX Manager (version 3.1) based on Cq values (quantitation cycle) of qPCR running with a dilution series of cDNA pools. The efficiency and amplification factors of each qPCR primer pair based on the slope of the standard curve was calculated with the help of the efficiency calculator1. Elongation factor 1α (EF1α; HE962191) was used as reference gene, and quantities of the genes of interest were expressed as RNA molecules of GOI/1000 RNA molecules of EF1α. The Cq values were determined from two technical replicates of each of the five biological replicates, and error bars indicate the standard error of the mean.
Quantification of Total Polygalacturonase Activity of Gut Content
Gut content samples were desalted with Zeba Desalt Spin Columns with a 7 kDa cutoff (Thermo Fisher Scientific, MA, United States) according to the manufacturer’s guidelines, and protein concentrations were determined using the Bradford reagent (Bradford, 1976) using the Protein Assay Dye Reagent (BioRad, CA, United States). Quantitative assays measuring the release of reducing sugars after the hydrolysis of polygalacturonic acid were set up and analyzed as previously described (Kirsch et al., 2016) with slight modifications. In detail, 500 ng gut content protein was incubated with 0.2% polygalacturonic acid in a 20 mM citrate/phosphate buffer pH 5.0 at 40°C for 10, 30, 60, 120, and 240 min. As a negative control, protein samples were boiled before incubation. Each assay was set up in three technical replicates for each of the five biological replicates, and reducing groups released after substrate hydrolysis were quantified by the 3,5-dinitrosalicylic acid (DNS) method (Miller, 1959). Three solutions were prepared for analysis of these samples in advance as follows: solution 1 containing DNS, phenol and sodium hydroxide to a final concentration of 1%, 0.2% and 1% (w/v) in water, respectively. Solution 2 is a 100-fold stock of sodium sulfite to a final concentration of 0.5% (w/v) in water, and solution 3 is a sevenfold stock of potassium sodium tartrate (Rochelle Salt) to a final concentration of 40% (w/v) in water. A mixture of solutions 1 and 2 in a 99:1 ratio (v/v) was prepared fresh each time just before use and added to a sample to be analyzed in a 1:1 ratio (v/v) followed by heating for 5 min in a PCR cycler at 99°C. Solution 3 was added in a 1:6 ratio (v/v), and the whole mixture was cooled down to room temperature before reading the absorbance at 575 nm on an Infinite M200 microplate reader (Tecan, Switzerland). The amount of reducing acids released is calculated based on a galacturonic acid standard curve. PG activity is expressed as nmol GalA equivalents/min/μg gut content protein released. Error bars indicate the standard error of the mean.
RNA-Seq Analysis
RNA samples used for RNA-Seq were the same as those used for RT-qPCR. Transcriptome sequencing was carried out for four biological replicates per treatment group and a total of 16 RNA samples using poly(A)+ enriched RNA fragmented to an average of 250 nucleotides. Sequencing was carried out by the Max Planck Genome Center, Cologne, on a HiSeq2500 sequencing system (Illumina, CA, United States) platform using paired-end (2 × 150 bp) reads, yielding approximately 20–30 million reads for each of the 16 samples. Quality control measures, including the filtering of high-quality reads based on fastq file scores, the removal of reads containing primer/adapter sequences and trimming of the read length, were carried out using CLC Genomics Workbench v10.12. The same software was used for de novo transcriptome assembly, combining randomly sampled batches of 15 Mio reads of two replicate samples each of the four RNA-seq treatment groups, using a total of 120 Mio reads and selecting the presumed optimal consensus transcriptome as previously described (Vogel et al., 2014). The final de novo reference gut transcriptome assembly (backbone) of P.cochleariae contained 72,572 contigs (minimum contig size = 250 bp) with an N50 contig size of 1,217 bp and a maximum contig length of 26,428 bp. The transcriptome was annotated using BLAST, Gene Ontology and InterProScan searches implemented in BLAST2GO PRO v5.13 as previously described (Pöppel et al., 2015).
Digital gene expression analysis was carried out using CLC Genomics workbench v10.1 to generate BAM mapping files, and QSeq (DNAStar Inc., United States) to remap the Illumina reads from all 16 samples onto the reference transcriptome. The final step was to count the sequences to estimate the expression levels, using previously described parameters for mapping and normalization (Jacobs et al., 2016), but changing the read assignment quality options to require at least 80% of the total read bases and at least 90% of bases matching within each read to be assigned to a specific contig. To control for the effect of global normalization using the RPKM (reads per kilobase per million reads) algorithm, we analyzed a number of highly conserved housekeeping genes, including those encoding GAPDH, ribosomal proteins RpS4e, RpS18, and RpL7 and eukaryotic translation initiation factor 5a. The overall variation in expression level for these housekeeping genes was lower than 1.2-fold, indicating they were not differentially expressed. RpS18 and RpL7 genes were used as reference gene and are shown in the heat map to confirm similar expression levels of control genes across treatment groups. The log2 (RPKM) values (normalized mapped read values; geometric means of the biological replicate samples) were subsequently used to calculate fold-change values. To identify differentially expressed genes, we used Student’s t-test (as implemented in Qseq) corrected for multiple testing using the Benjamini–Hochberg procedure to control the false discovery rate (FDR). The differential expression (fold-change values) of the GH28 genes, and the statistical significance thereof (Student’s t-test; FDR-corrected p-values), are summarized in Supplementary Table S2.
The short read data have been deposited in the EBI short read archive (SRA) with the following sample accession numbers: ERS2876704–ERS2876707. The complete study can also be accessed directly using the following URL: http://www.ebi.ac.uk/ena/data/view/PRJEB29501.
Statistical Analysis
The dependency of PG activity and GH28 expression levels on the different treatments was tested with one-way ANOVA analyses and the Tukey HSD test in order to find differences among the groups, both implemented in SigmaPlot 12.0. PG activity and expression level analyses are based on the means of technical replicates for each of the five biological replicates. Values for expression level of GH28-1 and GH28-4 were not normally distributed and failed the Equal Variance Test. The influence of different treatments on the expression of GH28-1 and GH28-4 were therefore investigated using the generalized least squares method (gls from the nlme library (Pinheiro et al., 2018) to account for the variance in heterogeneity of the residuals. The varIdent variance structure [varIdent(form = ∼1 | treatment)] was used. The influence of the treatment was determined by removing the explanatory variable and comparing the simpler model with the full model using a likelihood ratio test (Zuur et al., 2009). Differences between factor levels were determined by factor level reduction (Crawley, 2013). To compare weight gain over time in RNAi-treated larvae, we calculated the relative growth rate for a period of 5 days. The amount of leaf eaten was recorded at the same time. These two parameters were used to calculate the food-to-energy conversion efficiency. Statistical analyses were based on 50 replicates per treatment.
The dependency of developmental times (number of days until molting from second to third instar, termination of feeding, pupation, eclosion) on the treatment and the amount of consumed leaf material was determined by analyzing covariance with the different treatments as categorical and the amount of consumed leaf material as continuous explanatory variables. Differences between factor levels were determined by factor level reduction (Crawley, 2013). All data were analyzed with R version 3.4.1 (R Development Core Team, 2017).
Results
Silencing GH28 Genes Is Specific
Phaedon cochleariae has nine GH28 family members. Three act as endo-PGs (28-1, 5, 9), one as an oligogalacturonase (28-4) hydrolyzing trigalacturonic acid released by the endo-PGs (Kirsch et al., 2014). In addition, five GH28s (28-2, 3, 6, 7, 8) do not show any activity toward pectic substrates or other PCW polysaccharides and possess amino acid substitutions in functionally important sites (Kirsch et al., 2014, this study, Supplementary Figure S2). To test for the biological impact of active and inactive GH28s, three genes each of active and inactive GH28 were silenced simultaneously: active endo-PGs, called 28a (28-1, 5, 9), and inactive GH28 pseudoenzymes, called 28i (28-3, 6, 7). Following RNAi, RT-qPCR revealed a significant and specific down-regulation of target genes compared to the GFP injection control (Figure 1A). It clearly illustrated the feasibility of simultaneously silencing several genes at once. In addition, the transcript abundances of GH28s not targeted through RNAi was not affected by any of the treatments, confirming the specificity of silencing genes of high sequence similarity (Figure 1B).
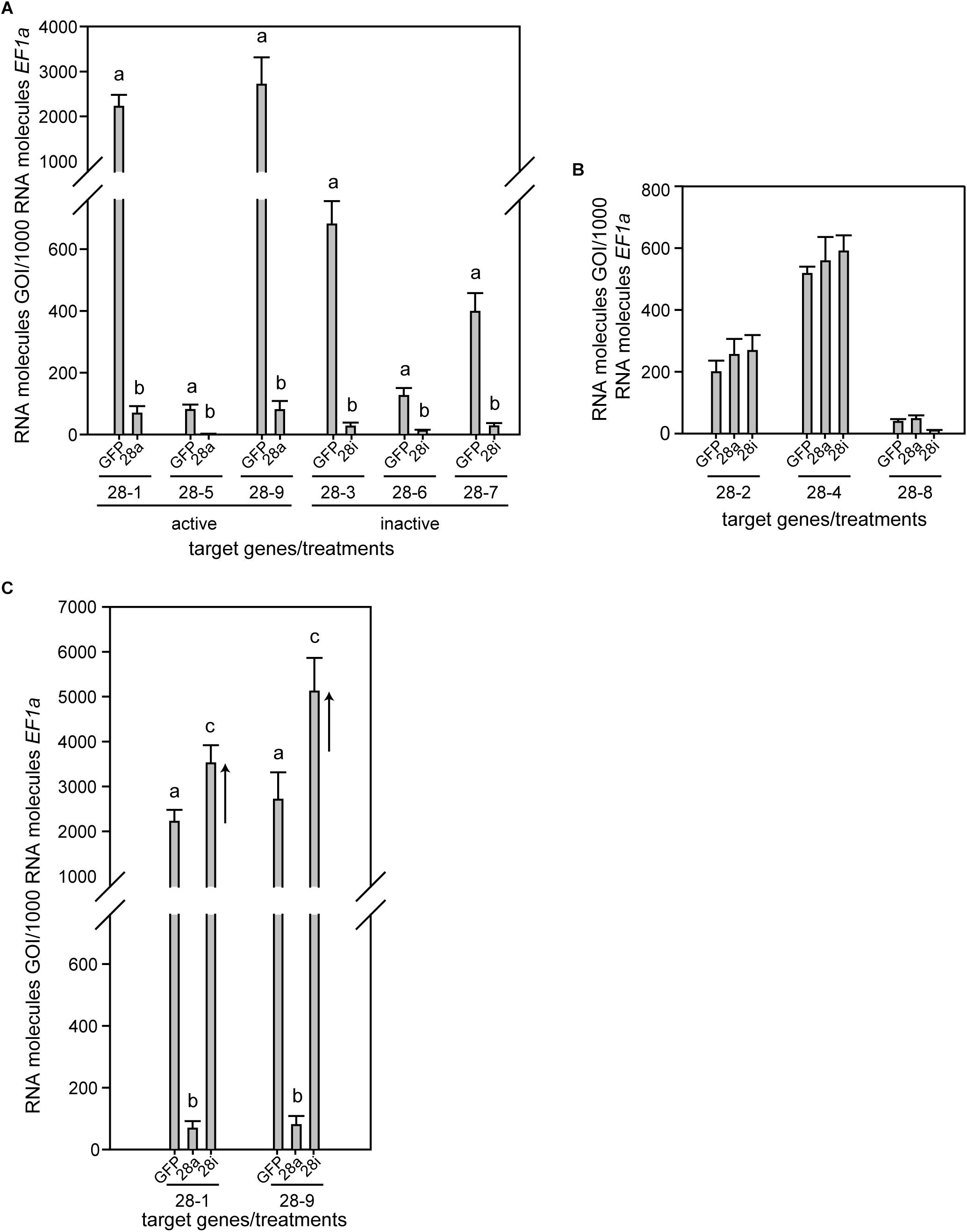
Figure 1. Expression pattern of P. cochleariae GH28s comparing injection control (GFP), active GH28 silencing (28a), and inactive GH28 silencing (28i). Expression of (A) GH28 targets, (B) untargeted GH28s, and (C) the up-regulation of active GH28s when silencing their inactive GH28 counterparts. Transcript abundances are expressed as RNA molecules of gene of interest (GOI) per 1000 RNA molecules of the reference gene elongation factor 1-alpha (EF-1α).
No Global Changes but Treatment-Specific GH28 Induction
To obtain a more global view of silencing specificity as well as treatment-specific responses to gene expression levels, global gene expression analyses using RNA-Seq were performed. Transcript abundance was calculated based on four biological replicates for each of the treatments (GFP, 28a, 28i). We compared gene expression changes in 28a and 28i treatment samples relative to the GFP control but did not find a complex pattern of differentially expressed genes. More specifically, there were no significant gene expression changes except for the 28a and 28i targeted GH28 encoding genes. This gene expression pattern is exemplarily shown for transcripts encoding a variety of GH families (Figure 2). Whereas targeted genes showed strong down-regulation compared to the control, the other GH families were not significantly affected. Among such families were further PCWDE-like cellulases (GH45) or xylanases (GH11), which obviously were not affected by the down-regulation of GH28s. Nevertheless, although not significant based on RNA-Seq data, the mRNA levels of the endo-PG 28-1 and 28-9 were higher in 28i than in the GFP control. To analyze this relationship in more detail, we performed RT-qPCR to measure the expression of all GH28 genes for each treatment (Supplementary Figure S3). When the genes encoding active endo-PGs were silenced the expression of the genes encoding the remaining GH28 family members did not change (Supplementary Figure S3). Strikingly, when the genes encoding the inactive GH28s were down-regulated, the expression of the genes encoding two active endo-PGs (28-1, 9) was significantly up-regulated at the same time (Figure 1C). Although the genes encoding 28-1 and 28-9 display the highest steady-state expression levels among all GH28 genes, they are still inducible to even higher levels (Supplementary Figure S3). In contrast, the genes encoding inactive GH28s are not differentially expressed when active PGs are silenced using RNAi. Thus, the differential expression of GH28 genes seems to depend on their function.
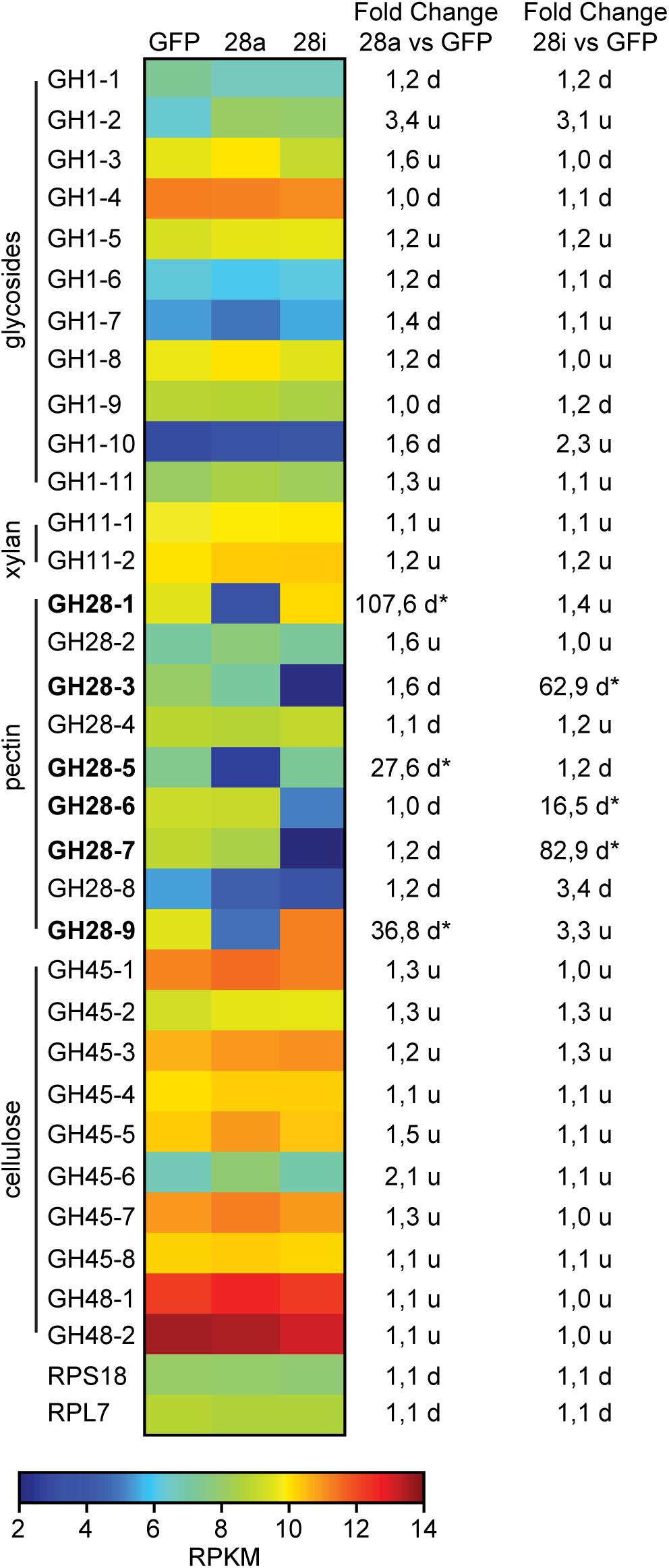
Figure 2. Heat map showing the relative expression levels of different glycoside hydrolase families (GH1 to 48) comparing the injection control (GFP) with the active (28a) and inactive (28i) GH28-silenced P. cochleariae larvae. Substrates of the different GH families are on the left. Silencing targets are indicated in bold and significant differences are shown with an asterisk (∗<0.05). Ribosomal protein 7 (RPL7) and ribosomal protein S18 (RPS18) are shown to confirm the uniform expression of these housekeeping genes across treatments. The map is based on log2-transformed RPKM values (blue represents weakly expressed genes, and red represents strongly expressed genes). The fold-change values presented on the right side were calculated based on the log2-transformed RPKM values.
Correlation of Silencing With PG Activity
To investigate the potential impact of gene silencing on gut PG activity, the release of polygalacturonic acid breakdown products by gut content was quantified. Endo-PG silenced larvae (28a) showed drastically reduced gut PG activity, with only about 10% remaining compared to the control (Figure 3). These results confirmed that the recombinant proteins characterized previously as active endo-PGs (28-1, 5, 9) in vitro (Kirsch et al., 2014) were indeed responsible for the gut PG activity observed in vivo. PG activity correlated with the GH28 expression level in the 28a treatment. The PG activity in the 28i treatment did not differ from that of the GFP control. This similarity is surprising, as the up-regulation of the genes encoding the two dominant endo-PGs in the 28i treatment should have resulted in an increased gut PG activity. Thus, PG activity seems not to correlate with the counter-regulation of the genes encoding the active PGs induced by the 28i silencing.
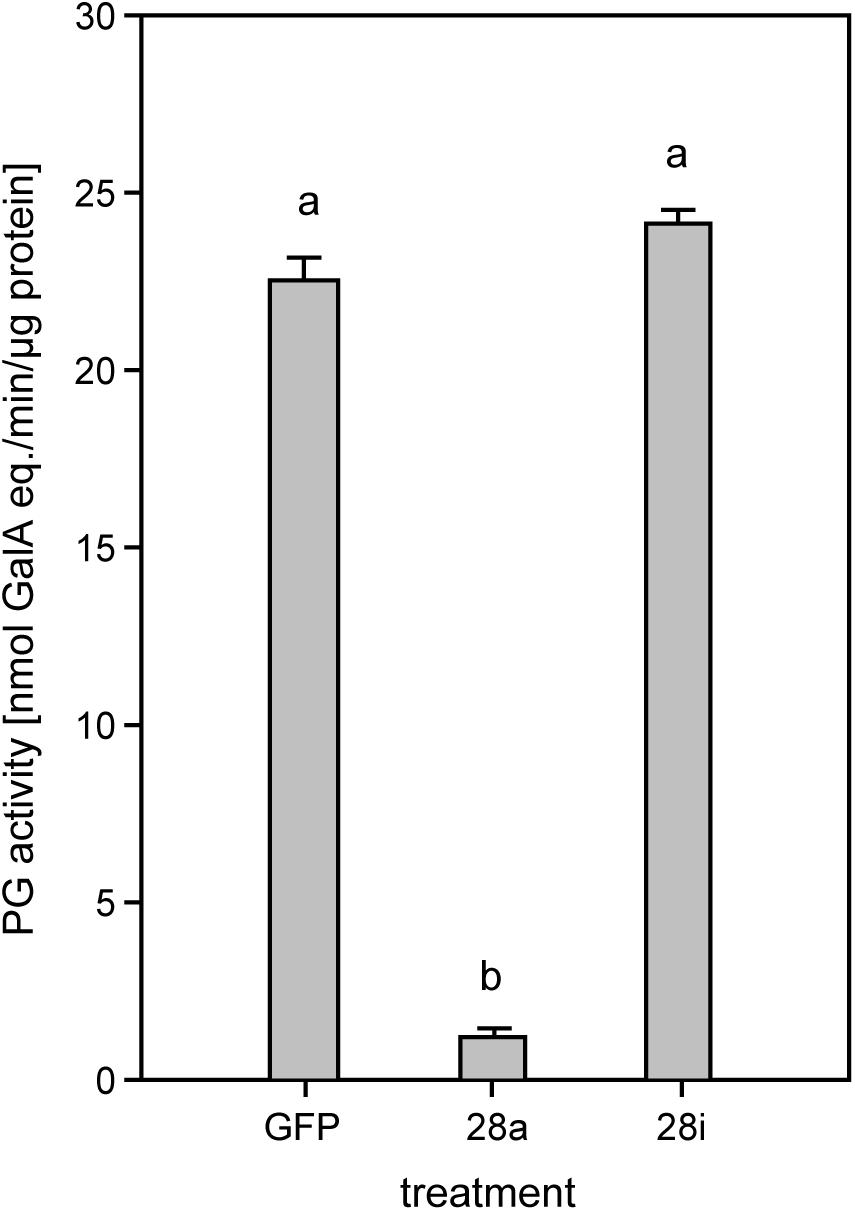
Figure 3. Quantification of PG activity in the P. cochleariae gut content. Hydrolytic activity in the larval guts of the injection control (GFP), active (28a), and inactive (28i) GH28-silenced larvae is shown. Activity is expressed in nmol-reducing uronic acids released per min and μg of gut content protein.
Correlation of Silencing With Life History Traits
The reduction of specific digestion-related enzymatic activity could lead to suboptimal nutrient release, which could in turn affect growth and development. To resolve the impact of impaired PG activity and thus less pectin breakdown in the gut, the amount of food ingested as well as the weight gain of P. cochleariae larvae were recorded. The change in weight as a function of the amount of food ingested is an indicator of food-to-energy conversion efficiency and thus a measurement of how efficiently nutrients can be released in the gut and subsequently used. The weight gain per cm2 leaf eaten was significantly lower in the 28i treatment, compared with the 28a treatment (Figure 4), indicating a less efficient food-to-energy conversion in the larvae for which the inactive PGs were silenced. Although inactive GH28s presumably do not impact pectin hydrolysis, our results suggest they have an important function in processing plant material and digestion. This connection seems counter-intuitive, as the silencing of pseudoenzymes seems to have a higher impact on food-to-energy conversion efficiency than the silencing of their active relatives. To test if these differences among treatments influenced larval development, we recorded time to pupation and time to eclosion in days after experimental injections. We further tested if developmental time depends on the treatment and the food consumed by the analysis of covariance. The time until pupation and eclosion depended on the treatment (pupation: F = 24.058, p < 0.001; eclosion: F = 5.692, p = 0.001) and the consumed food (pupation: F = 6.673, p < 0.001; eclosion: F = 14.538, p < 0.001; Figures 5A,B). More precisely, the larvae for which inactive GH28s were silenced developed more slowly with the same amount of food ingested compared to the other treatments, none of which showed any difference. Thus, the silencing of inactive GH28s prolongs the developmental period, which supports the important function of inactive GH28s.
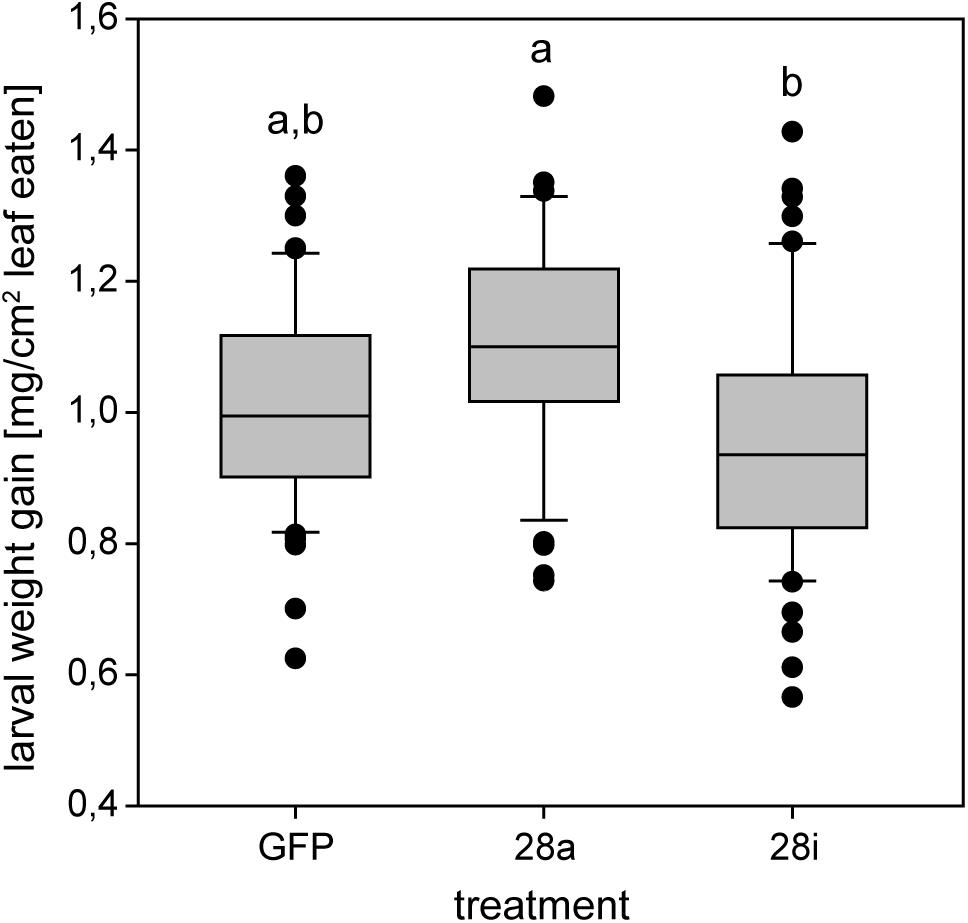
Figure 4. Efficiency of food-to-energy conversion from early second- to third-instar P. cochleariae larvae. Injection control (GFP) and the two silencing treatments are compared and the efficiency is calculated as mg larval weight gain per cm2 leaf eaten over time.
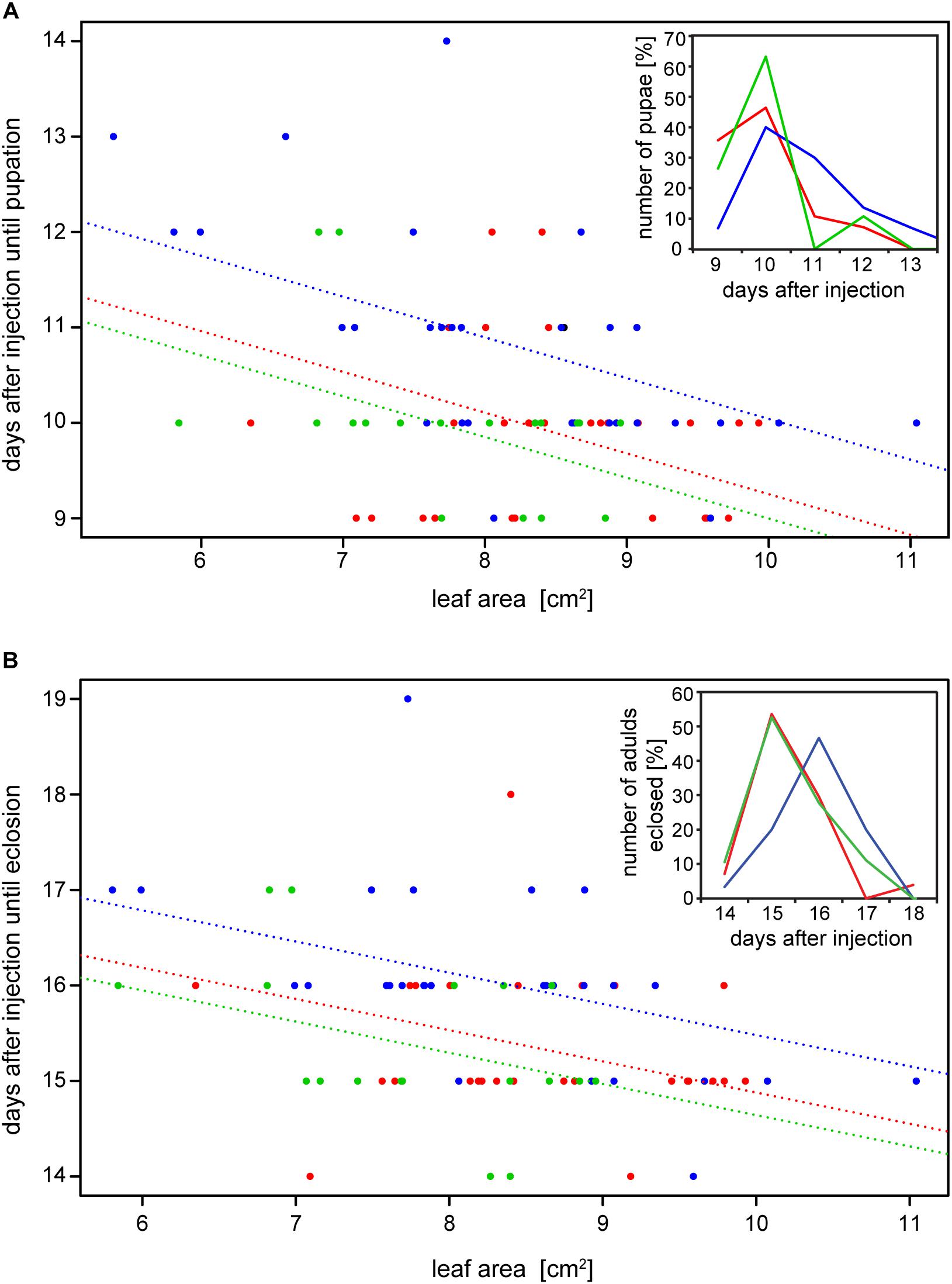
Figure 5. Dependency of (A) time to pupation and (B) time to eclosion from the amount of the consumed leaf area and the treatment (GFP: red, 28a: green and 28i: blue). Insertions show the number of pupae and adults that emerged in percentages over days after injection, respectively.
Discussion
Herbivorous insects have an optimal carbon-to-nitrogen (C/N) ratio for food intake, which they often fail to achieve due to an unbalanced C/N plant diet (Behmer, 2009). In plants, starch is the main storage polysaccharide and the major source of carbohydrate-based energy in herbivorous insects. Fitness costs, such as developmental delay resulting from altered starch digestion in insects either through the ingestion of plant α-amylase inhibitors or the knockdown of amylase genes, illustrate the importance of carbohydrate accessibility (Pueyo et al., 1995; Borzoui et al., 2017; Laudani et al., 2017).
There is another potential carbohydrate source that is omnipresent in a herbivore’s diet but often overlooked: the plant cell wall (PCW). The PCW is rich in polysaccharides such as cellulose, various hemicelluloses and pectin, and this set is highly conserved (Albersheim et al., 1996). PCW-degrading enzymes (PCWDEs) are widely distributed in insects (Calderon-Cortes et al., 2012). Since many insects rely on a nitrogen-poor diet that is rich in cellulose, it is conceivable that herbivorous insects exploit this source of carbohydrates (Watanabe and Tokuda, 2010). Experiments with feeding termites a 13C-labeled cellulose diet showed that the 13C labels appear fixed in amino acids supplemented by the termites’ gut microbes, providing evidence that cellulose degradation increases nitrogen levels and is beneficial for some insects (Tokuda et al., 2014). In addition, silencing of a cellulase in larvae of the western corn rootworm Diabrotica v. virgifera lowered weight gain and increased the time to pupation compared to control larvae (Valencia et al., 2013). These results have to be taken with caution as the authors neither run off-target predictions nor showed a reduction of cellulase activity resulting from gene silencing. Thus, the observed phenotype cannot be correlated with lower cellulase activity with total certainty. Moreover, the knockdown of GH45 cellulases in the leaf beetle Gastrophysa viridula had no effect on larval fitness (Busch et al., 2018), indicating that the impact of cellulase activity in herbivorous insects depends on both species and context, such as the diet provided for feeding assays.
In addition to cellulose, herbivorous insects feeding on living plant material ingest high amounts of pectin. Whether insects benefit from pectin digestion is not clear. We found no effect on P. cochleariae larval development when silencing active PGs in the 28a treatment. Surprisingly, we detected an effect on insect fitness and on gene expression when silencing the inactive GH28s compared to the active ones. Furthermore, silencing GH28 pseudoenzymes lowered the food-to-energy conversion efficiency and lengthened the time required for development. These effects are similar to biological consequences caused by the suppression of digestive enzymes such as the amylases mentioned above, as well as α-glucosidases (Singh et al., 2015), proteases (Zhu-Salzman and Zeng, 2015), and lipases (Markwick et al., 2011). At first glance, the presence of fitness costs in insects of the 28i but not in those of the 28a treatment is counter-intuitive, as the silencing efficiency is comparable between the two treatments and the suppression of the PGs goes hand in hand with a drastic reduction in PG activity.
Gut enzymes are usually part of an intertwined and finely tuned digestive system, which can be regulated at multiple levels and in a manner that is not predictable. For example, the inhibition of proteases of the Phytophaga seed beetle Callosobruchus maculatus resulted in the differential expression of PCWDEs, including GH5 mannanases and GH28s (Chi et al., 2009; Nogueira et al., 2012). This effect on PCWDE expression indicates crosstalk between digestive enzymes involved in protein and polysaccharide breakdown, and, even more importantly, illustrates the impact of PCWDEs in insects coping with sub-optimal diets. The performance of P. cochleariae depends on host plant species as well as on plant quality (Müller and Müller, 2016, 2017) and pectin amount and structure generally differ between plants (Müller-Maatsch et al., 2016; Dranca and Oroian, 2018). The P. cochleariae laboratory strain used in our experiments is kept under optimal conditions and is adapted to Brassica species used for rearing since many generations. Therefore, decreased PG activity might have a strong effect on the larvae exposed to a challenging diet and ecologically relevant environment. The movement of the food bolus, and with that the amount of time PGs and pectin can interact in the gut, is highly variable in insects, ranging from hours to several days (Chapman, 1998). Thus, although PG activity in the 28a treatment is impaired, the interaction time of PGs and pectin in the gut might be enough for sufficient enzyme function. As Phytophaga beetles also possess gene families encoding active cellulases and hemicellulases (Acuña et al., 2012; Pauchet and Heckel, 2013; Pauchet et al., 2014; McKenna et al., 2016; Busch et al., 2017, 2018), it is unclear whether the lack of a specific PCWDE activity, such as that of PGs, is costly or can be compensated for by the concurrent action of other enzymatic functions.
As the function of the inactive GH28s is unknown, the diminished food-to-energy conversion efficiency and extended developmental delay caused by their silencing is hard to explain. Although challenging (Murphy et al., 2017), the functions already assigned to pseudoenzymes are extraordinarily diverse, ranging from regulators of their active counterparts or inhibitors of completely unrelated enzymes to being decoys that snatch away inhibitors to protect closely related enzymes (Rose et al., 2002; Bryant et al., 2008; Ma et al., 2017). To clarify the role of the inactive GH28 pseudoenzymes, we performed RNA-Seq-based global gene expression analysis, combined with RT-qPCR analyses of selected genes, to identify treatment-dependent changes in gene expression. Surprisingly, when treatments with the gfp injection control were compared, we did not find a complex pattern of differentially expressed genes in our analysis of the global transcriptome data. The only significant changes in gene expression are found in the GH28 genes that were down-regulated in the corresponding treatments. In addition, we found a slight up-regulation of active PGs when knocking down the inactive GH28s. RT-qPCR analyses revealed that the two active PGs (28-1 and 28-9) are significantly up-regulated in the 28i treatment. This induction indicates a crosstalk between active and inactive GH28 family members and shows that the expression levels depend on each other at least in one direction. However, the up-regulation of the expression of PGs in the 28i treatment does not fit with the observed PG enzyme activity levels. Although PG activity should be higher in the 28i compared to the gfp control treatment, due to higher PG expression levels, the activity levels do not differ. The only plausible explanation for this discrepancy is that the down-regulation of the inactive GH28s results in a reduction of PG activity in the gut, which is compensated for by the up-regulation of PGs. The possibility thus exists that the inactive GH28 proteins, although pseudoenzymes, are still linked to the pectolytic pathway, which, at least in part, could explain the observed developmental delay. Additional support for the synergistic character of active and inactive GH28s comes from the temporal and spatial co-expression of those genes (Kirsch et al., 2012, 2014, 2016).
It is clear that GH28-3 has lost the ability to hydrolyze any substrate due to a substitution of one of the three catalytic aspartate residues (the catalytic nucleophile) (Kirsch et al., 2014). However, the substitutions of catalytic important residues in GH28-7 and GH28-8 may have led to a complete loss of activity or to a drastic change in substrate specificity. Right now, we can only speculate on the specific function of the inactive GH28 proteins. On one hand, they have lost the ability to hydrolyze polygalacturonic acid, which makes them PG pseudoenzymes. On the other hand, it cannot be excluded that these proteins degrade specific sub-domains of pectin, but their activity cannot be tested due to unsuitable substrates available (e.g., specific sugar decorations or chain lengths).
Our data reveal that the loss of enzymatic activity toward an ancestral substrate does not mean that these proteins have completely lost their impact on the underlying pathway but, rather, suggests they may be important for the organism. The high steady-state expression level of active endo-PGs and their dynamic regulation stand for the robustness of the pectolytic system and thus its importance for herbivorous beetles. Knocking down inactive GH28s exceeds the impact of their active counterparts on the fitness of P. cochleariae suggesting that these pseudoenzymes play a non-negligible role in the physiology of this insect.
Author Contributions
RK contributed to the conception and design of the study. RK and HV performed the experiments. RK and HV analyzed the data. GK performed the statistical analysis. RK wrote the first draft of the manuscript. RK, GK, HV, and YP wrote the sections of the manuscript. All authors contributed to the revision, read and approved the final version of the manuscript for submission.
Funding
This work was supported by the Max Planck Society. RK was supported by the German Science Foundation (KI1917/1-1).
Conflict of Interest Statement
The authors declare that the research was conducted in the absence of any commercial or financial relationships that could be construed as a potential conflict of interest.
Acknowledgments
We thank Bianca Wurlitzer for P. cochleariae stock rearing and the greenhouse team for plant rearing. We thank Caroline Müller (Bielefeld University), David G. Heckel, and Franziska Beran (MPI for Chemical Ecology) for fruitful discussions regarding experimental setup, and Wiebke Häger (MPI for Chemical Ecology) for preparing the PCW substrate. We also thank Emily Wheeler, Boston, for editorial assistance.
Supplementary Material
The Supplementary Material for this article can be found online at: https://www.frontiersin.org/articles/10.3389/fphys.2019.00685/full#supplementary-material
Footnotes
- ^ http://www.thermoscientificbio.com/webtools/qpcrefficiency/
- ^ https://www.qiagenbioinformatics.com/
- ^ www.blast2go.de
References
Acuña, R., Padilla, B. E., Florez-Ramos, C. P., Rubio, J. D., Herrera, J. C., Benavides, P., et al. (2012). Adaptive horizontal transfer of a bacterial gene to an invasive insect pest of coffee. Proc. Natl. Acad. Sci. U.S.A. 109, 4197–4202. doi: 10.1073/pnas.1121190109
Albersheim, P., Darvill, A. G., Oneill, M. A., Schols, H. A., and Voragen, A. G. J. (1996). An hypothesis: The same six polysaccharides are components of the primary cell walls of all higher plants. Prog. Biotechnol. 14, 47–55. doi: 10.1016/S0921-0423(96)80245-0
Allen, M. L., and Mertens, J. A. (2008). Molecular cloning and expression of three polygalacturonase cDNAs from the tarnished plant bug, Lygus lineolaris. J. Insect Sci. 8, 1–14. doi: 10.1673/031.008.2701
Anand, A. A., Vennison, S. J., Sankar, S. G., Prabhu, D. I., Vasan, P. T., Raghuraman, T., et al. (2010). Isolation and characterization of bacteria from the gut of Bombyx mori that degrade cellulose, xylan, pectin and starch and their impact on digestion. J. Insect Sci. 10:107. doi: 10.1673/031.010.10701
Behmer, S. T. (2009). Insect herbivore nutrient regulation. Annu. Rev. Entomol. 54, 165–187. doi: 10.1146/annurev.ento.54.110807.090537
Borzoui, E., Nouri-Ganbalani, G., and Naseri, B. (2017). In vitro and in vivo effects of alpha-amylase inhibitor from Avena sativa seeds on life history and physiological characteristics of Sitotroga cerealella (Lepidoptera: Gelechiidae). J. Insect Sci. 17:108. doi: 10.1093/jisesa/iex088
Bradford, M. M. (1976). A rapid and sensitive method for the quantitation of microgram quantities of protein utilizing the principle of protein-dye binding. Anal. Biochem. 72, 248–254. doi: 10.1006/abio.1976.9999
Bryant, B., Blair, C. D., Olson, K. E., and Clem, R. J. (2008). Annotation and expression profiling of apoptosis-related genes in the yellow fever mosquito, Aedes aegypti. Insect Biochem. Mol. Biol. 38, 331–345. doi: 10.1016/j.ibmb.2007.11.012
Busch, A., Kunert, G., Heckel, D. G., and Pauchet, Y. (2017). Evolution and functional characterization of CAZymes belonging to subfamily 10 of glycoside hydrolase family 5 (GH5_10) in two species of phytophagous beetles. PLoS One 12:e0184305. doi: 10.1371/journal.pone.0184305
Busch, A., Kunert, G., Wielsch, N., and Pauchet, Y. (2018). Cellulose degradation in Gastrophysa viridula (Coleoptera: Chrysomelidae): functional characterization of two CAZymes belonging to glycoside hydrolase family 45 reveals a novel enzymatic activity. Insect Mol. Biol. 27, 633–650. doi: 10.1111/imb.12500
Calderon-Cortes, N., Quesada, M., Watanabe, H., Cano-Camacho, H., and Oyama, K. (2012). Endogenous plant cell wall digestion: a key mechanism in insect evolution. Annu. Rev. Ecol. Evol. Syst. 43, 45–71. doi: 10.1146/annurev-ecolsys-110411-160312
Celorio-Mancera, M. D., Allen, M. L., Powell, A. L., Ahmadi, H., Salemi, M. R., Phinney, B. S., et al. (2008). Polygalacturonase causes lygus-like damage on plants: cloning and identification of western tarnished plant bug (Lygus hesperus) polygalacturonases secreted during feeding. Arthropod Plant Interact. 2, 215–225. doi: 10.1007/s11829-008-9050-7
Chang, Y., Wang, S., Sekimoto, S., Aerts, A. L., Choi, C., Clum, A., et al. (2015). Phylogenomic analyses indicate that early fungi evolved digesting cell walls of algal ancestors of land plants. Genome Biol. Evol. 7, 1590–1601. doi: 10.1093/gbe/evv090
Chi, Y. H., Salzman, R. A., Balfe, S., Ahn, J. E., Sun, W., Moon, J., et al. (2009). Cowpea bruchid midgut transcriptome response to a soybean cystatin–costs and benefits of counter-defence. Insect Mol. Biol. 18, 97–110. doi: 10.1111/j.1365-2583.2008.00854.x
De Lorenzo, G., and Ferrari, S. (2002). Polygalacturonase-inhibiting proteins in defense against phytopathogenic fungi. Curr. Opin. Plant Biol. 5, 295–299. doi: 10.1016/S1369-5266(02)00271-6
Dranca, F., and Oroian, M. (2018). Extraction, purification and characterization of pectin from alternative sources with potential technological applications. Food Res. Int. 113, 327–350. doi: 10.1016/j.foodres.2018.06.065
Engel, P., Martinson, V. G., and Moran, N. A. (2012). Functional diversity within the simple gut microbiota of the honey bee. Proc. Natl. Acad. Sci. U.S.A. 109, 11002–11007. doi: 10.1073/pnas.1202970109
Evangelista, D. E., De Paula, F. F. P., Rodrigues, A., and Henrique-Silva, F. (2015). Pectinases from Sphenophorus levis Vaurie, 1978 (Coleoptera: Curculionidae): putative accessory digestive enzymes. J. Insect Sci. 15:168. doi: 10.1093/jisesa/ieu168
Feiz, L., Irshad, M., Pont-Lezica, R. F., Canut, H., and Jamet, E. (2006). Evaluation of cell wall preparations for proteomics: a new procedure for purifying cell walls from Arabidopsis hypocotyls. Plant Methods 2:10. doi: 10.1186/1746-4811-2-10
Gilbert, H. J. (2010). The biochemistry and structural biology of plant cell wall deconstruction. Plant Physiol. 153, 444–455. doi: 10.1104/pp.110.156646
Hearn, J. (2013). Exploring Population History and Gall Induction in Cynipid Gall Wasps Using Genomics and Transcriptomics. Ph.D. thesis, University of Edinburgh, Edinburgh.
Jacobs, C. G. C., Steiger, S., Heckel, D. G., Wielsch, N., Vilcinskas, A., and Vogel, H. (2016). Sex, offspring and carcass determine antimicrobial peptide expression in the burying beetle. Sci. Rep. 6:25409. doi: 10.1038/srep25409
Kars, I., Krooshof, G. H., Wagemakers, L., Joosten, R., Benen, J. A. E., and Van Kan, J. A. L. (2005). Necrotizing activity of five Botrytis cinerea endopolygalacturonases produced in Pichia pastoris. Plant J. 43, 213–225. doi: 10.1111/j.1365-313X.2005.02436.x
Kaur, R., Kaur, N., and Gupta, A. K. (2014). Structural features, substrate specificity, kinetic properties of insect alpha-amylase and specificity of plant alpha-amylase inhibitors. Pestic. Biochem. Physiol. 116, 83–93. doi: 10.1016/j.pestbp.2014.09.005
Kirsch, R., Gramzow, L., Theissen, G., Siegfried, B. D., Ffrench-Constant, R. H., Heckel, D. G., et al. (2014). Horizontal gene transfer and functional diversification of plant cell wall degrading polygalacturonases: key events in the evolution of herbivory in beetles. Insect Biochem. Mol. Biol. 52, 33–50. doi: 10.1016/j.ibmb.2014.06.008
Kirsch, R., Heckel, D. G., and Pauchet, Y. (2016). How the rice weevil breaks down the pectin network: Enzymatic synergism and sub-functionalization. Insect Bioch. Mol. Biol. 71, 72–82. doi: 10.1016/j.ibmb.2016.02.007
Kirsch, R., Wielsch, N., Vogel, H., Svatos, A., Heckel, D. G., and Pauchet, Y. (2012). Combining proteomics and transcriptome sequencing to identify active plant-cell-wall-degrading enzymes in a leaf beetle. BMC Genomics 13:587. doi: 10.1186/1471-2164-13-587
Kubicek, C. P., Starr, T. L., and Glass, N. L. (2014). Plant cell wall-degrading enzymes and their secretion in plant-pathogenic fungi. Annu. Rev. Phytopathol. 52, 427–451. doi: 10.1146/annurev-phyto-102313-045831
Lagaert, S., Belien, T., and Volckaert, G. (2009). Plant cell walls: Protecting the barrier from degradation by microbial enzymes. Semin. Cell Dev. Biol. 20, 1064–1073. doi: 10.1016/j.semcdb.2009.05.008
Laudani, F., Strano, C. P., Edwards, M. G., Malacrino, A., Campolo, O., Abd El Halim, H. M., et al. (2017). RNAi-mediated gene silencing in Rhynchophorus ferrugineus (Oliver) (Coleoptera: Curculionidae). Open Life Sci. 12, 214–222. doi: 10.1515/biol-2017-0025
Lehane, M. J., and Billingsley, P. F. (1996). The Biology of the Insect Midgut. Dordrecht: Springer.
Ma, Z., Zhu, L., Song, T., Wang, Y., Zhang, Q., Xia, Y., et al. (2017). A paralogous decoy protects Phytophthora sojae apoplastic effector PsXEG1 from a host inhibitor. Science 355, 710–714. doi: 10.1126/science.aai7919
Markwick, N. P., Poulton, J., Mcghie, T. K., Wohlers, M. W., and Christeller, J. T. (2011). The effects of the broad-specificity lipase inhibitor, tetrahydrolipstatin, on the growth, development and survival of the larvae of Epiphyas postvittana (Walker) (Tortricidae, Lepidoptera). J. Insect Physiol. 57, 1643–1650. doi: 10.1016/j.jinsphys.2011.08.018
Marvaldi, A. E., Duckett, C. N., Kjer, K. M., and Gillespie, J. J. (2009). Structural alignment of 18S and 28S rDNA sequences provides insights into phylogeny of Phytophaga (Coleoptera: Curculionoidea and Chrysomeloidea). Zool. Scr. 38, 63–77. doi: 10.1111/j.1463-6409.2008.00360.x
Mattson, W. J. (1980). Herbivory in relation to plant nitrogen-content. Annu. Rev. Ecol. Syst. 11, 119–161. doi: 10.1146/annurev.es.11.110180.001003
McKenna, D. D., Scully, E. D., Pauchet, Y., Hoover, K., Kirsch, R., Geib, S. M., et al. (2016). Genome of the Asian longhorned beetle (Anoplophora glabripennis), a globally significant invasive species, reveals key functional and evolutionary innovations at the beetle-plant interface. Genome Biol. 17:227. doi: 10.1186/S13059-016-1088-8
McNeil, M., Darvill, A. G., Fry, S. C., and Albersheim, P. (1984). Structure and function of the primary cell walls of plants. Annu. Rev. Biochem. 53, 625–663. doi: 10.1146/annurev.bi.53.070184.003205
Miller, G. L. (1959). Use of Dinitrosalicylic acid reagent for determination of reducing sugars. Anal. Chem. 31, 426–428. doi: 10.1021/ac60147a030
Müller, T., and Müller, C. (2016). Adult beetles compensate for poor larval food conditions. J. Insect Physiol. 88, 24–32. doi: 10.1016/j.jinsphys.2016.02.009
Müller, T., and Müller, C. (2017). Phenotype of a leaf beetle larva depends on host plant quality and previous test experience. Behav. Process. 142, 40–45. doi: 10.1016/j.beproc.2017.05.017
Müller-Maatsch, J., Bencivenni, M., Caligiani, A., Tedeschi, T., Bruggeman, G., Bosch, M., et al. (2016). Pectin content and composition from different food waste streams. Food Chem. 201, 37–45. doi: 10.1016/j.foodchem.2016.01.012
Murphy, J. M., Farhan, H., and Eyers, P. A. (2017). Bio-Zombie: the rise of pseudoenzymes in biology. Biochem. Soc. Trans. 45, 537–544. doi: 10.1042/Bst20160400
Nogueira, F. C. S., Silva, C. P., Alexandre, D., Samuels, R. I., Soares, E. L., Aragao, F. J. L., et al. (2012). Global proteome changes in larvae of Callosobruchus maculatus (Coleoptera:Chrysomelidae:Bruchinae) following ingestion of a cysteine proteinase inhibitor. Proteomics 12, 2704–2715. doi: 10.1002/pmic.201200039
Pauchet, Y., and Heckel, D. G. (2013). The genome of the mustard leaf beetle encodes two active xylanases originally acquired from bacteria through horizontal gene transfer. Proc. R. Soc. Lond. B Biol. Sci. 280:20131021. doi: 10.1098/rspb.2013.1021
Pauchet, Y., Kirsch, R., Giraud, S., Vogel, H., and Heckel, D. G. (2014). Identification and characterization of plant cell wall degrading enzymes from three glycoside hydrolase families in the cerambycid beetle Apriona japonica. Insect Biochem. Mol. Biol. 49, 1–13. doi: 10.1016/j.ibmb.2014.03.004
Pauchet, Y., Wilkinson, P., Chauhan, R., and Ffrench-Constant, R. H. (2010). Diversity of beetle genes encoding novel plant cell wall degrading enzymes. PLoS One 5:e15635. doi: 10.1371/journal.pone.0015635
Pinheiro, J., Bates, D., Debroy, S., Sarkar, D., and R Core Team (2018). ”Linear and Nonlinear Mixed Effects Models”. R Package Version 3.1-137.
Pöppel, A. K., Vogel, H., Wiesner, J., and Vilcinskas, A. (2015). Antimicrobial peptides expressed in medicinal maggots of the blow fly Lucilia sericata show combinatorial activity against bacteria. Antimicrob. Agents Chemother. 59, 2508–2514. doi: 10.1128/AAC.05180-14
Pueyo, J. J., Morgan, T. D., Ameenuddin, N., Liang, C., Reeck, G. R., Chrispeels, M. J., et al. (1995). Effects of bean and wheat alpha-amylase inhibitors on alpha-amylase activity and growth of stored-product insect pests. Entomol. Exp. Appl. 75, 237–244. doi: 10.1111/j.1570-7458.1995.tb01932.x
R Development Core Team (2017). R: A Language and Environment for Statistical Computing. Vienna: R Foundation for Statistical Computing.
Reignault, P., Valette-Collet, O., and Boccara, M. (2008). The importance of fungal pectinolytic enzymes in plant invasion, host adaptability and symptom type. Eur. J. Plant Pathol. 120, 1–11. doi: 10.1007/s10658-007-9184-y
Rose, J. K. C., Ham, K. S., Darvill, A. G., and Albersheim, P. (2002). Molecular cloning and characterization of glucanase inhibitor proteins: coevolution of a counterdefense mechanism by plant pathogens. Plant Cell 14, 1329–1345. doi: 10.1105/tpc.002253
Salem, H., Bauer, E., Kirsch, R., Berasategui, A., Cripps, M., Weiss, B., et al. (2017). Drastic genome reduction in an herbivore’s pectinolytic symbiont. Cell 171, 1520–1531. doi: 10.1016/j.cell.2017.10.029
Schindelin, J., Arganda-Carreras, I., Frise, E., Kaynig, V., Longair, M., Pietzsch, T., et al. (2012). Fiji: an open-source platform for biological-image analysis. Nat. Methods 9, 676–682. doi: 10.1038/Nmeth.2019
Schols, H. A., Visser, R., and Voragen, A. (2009). Pectins and Pectinases. Wageningen: Wageningen Academic Publishers.
Schoonhoven, L. M., Van Loon, J. J. A., and Dicke, M. (2005). Insect–Plant Biology. New York, NY: Oxford University Press.
Shackel, K. A., Celorio-Mancera, M. D. L. P., Ahmadi, H., Greve, L. C., Teuber, L. R., Backus, E. A., et al. (2005). Micro-injection of Lygus salivary gland proteins to simulate feeding damage in alfalfa and cotton flowers. Arch. Insect Biochem. Physiol. 58, 69–83. doi: 10.1002/Arch.20033
Shelomi, M., Danchin, E. G. J., Heckel, D., Wipfler, B., Bradler, S., Zhou, X., et al. (2016). Horizontal gene transfer of pectinases from bacteria preceded the diversification of stick and leaf insects. Sci. Rep. 6:26388. doi: 10.1038/srep26388
Shelomi, M., Jasper, W. C., Atallah, J., Kimsey, L. S., and Johnson, B. R. (2014). Differential expression of endogenous plant cell wall degrading enzyme genes in the stick insect (Phasmatodea) midgut. BMC Genomics 15:917. doi: 10.1186/1471-2164-15-917
Shen, Z., Denton, M., Mutti, N., Pappan, K., Kanost, M. R., Reese, J. C., et al. (2003). Polygalacturonase from Sitophilus oryzae: possible horizontal transfer of a pectinase gene from fungi to weevils. J. Insect Sci. 3, 24.
Singh, B., Kaur, T., Kaur, S., Manhas, R. K., and Kaur, A. (2015). An alpha-glucosidase inhibitor from an endophytic Cladosporium sp. with potential as a biocontrol agent. Appl. Biochem. Biotechnol. 175, 2020–2034. doi: 10.1007/s12010-014-1325-0
Sprockett, D. D., Piontkivska, H., and Blackwood, C. B. (2011). Evolutionary analysis of glycosyl hydrolase family 28 (GH28) suggests lineage-specific expansions in necrotrophic fungal pathogens. Gene 479, 29–36. doi: 10.1016/j.gene.2011.02.009
Strong, D. R., Lawton, J. H., and Southwood, T. R. E. (1984). Insects on Plants. Community Patterns and Mechanisms. Oxford: Blackwell.
Tokuda, G., Tsuboi, Y., Kihara, K., Saitou, S., Moriya, S., Lo, N., et al. (2014). Metabolomic profiling of 13C-labelled cellulose digestion in a lower termite: insights into gut symbiont function. Proc. R. Soc. Lond. B Biol. Sci. 281:20140990. doi: 10.1098/rspb.2014.0990
Valencia, A., Alves, A. P., and Siegfried, B. D. (2013). Molecular cloning and functional characterization of an endogenous endoglucanase belonging to GHF45 from the western corn rootworm, Diabrotica virgifera virgifera. Gene 513, 260–267. doi: 10.1016/j.gene.2012.10.046
Vogel, H., Badapanda, C., Knorr, E., and Vilcinskas, A. (2014). RNA-sequencing analysis reveals abundant developmental stage-specific and immunity-related genes in the pollen beetle Meligethes aeneus. Insect Mol. Biol. 23, 98–112. doi: 10.1111/imb.12067
Walton, J. D. (1994). Deconstructing the cell wall. Plant Physiol. 104, 1113–1118. doi: 10.1104/pp.104.4.1113
Watanabe, H., and Tokuda, G. (2010). Cellulolytic systems in insects. Annu. Rev. Entomol. 55, 609–632. doi: 10.1146/annurev-ento-112408-085319
Zhu-Salzman, K., and Zeng, R. S. (2015). Insect response to plant defensive protease inhibitors. Annu. Rev. Entomol. 60, 233–252. doi: 10.1146/annurev-ento-010814-020816
Keywords: herbivorous insect, beetle, plant cell wall degrading enzymes, pectin, GH28, polygalacturonase, pseudoenzyme, RNAi
Citation: Kirsch R, Kunert G, Vogel H and Pauchet Y (2019) Pectin Digestion in Herbivorous Beetles: Impact of Pseudoenzymes Exceeds That of Their Active Counterparts. Front. Physiol. 10:685. doi: 10.3389/fphys.2019.00685
Received: 08 November 2018; Accepted: 16 May 2019;
Published: 29 May 2019.
Edited by:
Jin-Jun Wang, Southwest University, ChinaReviewed by:
Vu Nguyen Thanh, Food Industries Research Institute, VietnamBernard Henrissat, Centre National de la Recherche Scientifique (CNRS), France
Copyright © 2019 Kirsch, Kunert, Vogel and Pauchet. This is an open-access article distributed under the terms of the Creative Commons Attribution License (CC BY). The use, distribution or reproduction in other forums is permitted, provided the original author(s) and the copyright owner(s) are credited and that the original publication in this journal is cited, in accordance with accepted academic practice. No use, distribution or reproduction is permitted which does not comply with these terms.
*Correspondence: Roy Kirsch, cmtpcnNjaEBpY2UubXBnLmRl; Yannick Pauchet, eXBhdWNoZXRAaWNlLm1wZy5kZQ==