- 1CAS Key Laboratory of Marine Ecology and Environmental Sciences, Institute of Oceanology, Chinese Academy of Sciences, Qingdao, China
- 2Laboratory for Marine Ecology and Environmental Sciences, Qingdao National Laboratory for Marine Science and Technology, Qingdao, China
- 3University of Chinese Academy of Sciences, Beijing, China
- 4Center for Ocean Mega-Science, Chinese Academy of Sciences, Qingdao, China
- 5Australian Institute of Marine Science, Townsville, QLD, Australia
Melatonin is a highly conserved hormone in evolutionary history. It occurs in numerous organisms and plays a role in the endocrine and immune systems. Locomotor behavior is a basic behavior in animals and is an important indicator of circadian rhythms, which are coordinated by the nervous and endocrine systems. To date, the effect of melatonin on locomotor behavior has been studied in vertebrates, including syrian hamsters, sparrows, rats, zebrafish, goldfish, and flatworms. However, there have been few studies of the effects of melatonin on locomotor behavior in marine invertebrates. The goals of present study were to show the existence of melatonin in the sea cucumber Apostichopus japonicus and to evaluate its effect on locomotor activity. In addition, muscle tissues from control and melatonin-treated sea cucumbers were tested using ultra performance liquid chromatography and quadrupole time-of-flight mass spectrometry (UPLC-Q-TOF-MS) to determine the changes of metabolic activity in muscle. Melatonin was present in the coelomic fluid of A. japonicus at a concentration of ∼135.0 ng/L. The total distance traveled and number steps taken over 9 h after melatonin administration decreased with increasing concentration of the melatonin dose. Mean and maximum velocity of movement and stride length and stride frequency also decreased, but their differences were not statistically significant. Overall, these results suggest that melatonin administration had a sedative effect on A. japonicus. The levels of 22 different metabolites were altered in the muscle tissues of melatonin-treated sea cucumbers. Serotonin, 9-cis retinoic acid, all-trans retinoic acid, flavin mononucleotide in muscles were downregulated after melatonin administration. Moreover, a high free fatty acid (FFA) concentration and a decrease in the adenosine 5′-triphosphate (ATP) concentration in the muscle tissues of the melatonin-treated group were detected as well. These results suggest that the sedative effect of melatonin involves some other metabolic pathways, and the reduced locomotor modulator—serotonin, inhibited fatty acid oxidation and disturbed oxidative phosphorylation are potential physiological mechanisms that result in the inhibitory effect of melatonin on locomotion in sea cucumbers.
Introduction
In 1958, melatonin was discovered from the pineal gland of cows (Lerner et al., 1958), and it subsequently was confirmed to be an indole neuroendocrine hormone (Reiter, 1995). This discovery triggered studies of the characteristics, physiology, medical applications, and behavioral functions of this special molecule. Melatonin is a highly conserved molecule in evolutionary history, and it occurs in organisms as different as plants and animals (Poeggeler, 1993). It is presumed to be a phylogenetically omnipresent hormone that primarily is involved in the regulation of circadian rhythm (Peres et al., 2014). Considering the extensive distribution of melatonin receptors in various tissues and organs of vertebrates, this hormone can play roles in diverse physiological processes (Zhdanova, 2005; Kulczykowska et al., 2006). In vertebrates, melatonin can be produced in the gastrointestinal system (Bubenik, 2002) and even the retina (Do Carmo Buonfiglio et al., 2011), but its main source of production is the pineal gland. In invertebrates, this hormone has been detected in numerous organs in insects (including the brain, palp, eyes, ovipositor, hind-leg, and ovary) and in the gonad of sea stars (Vivien-Roels and Pévet, 1993; Itoh et al., 1995; Peres et al., 2014). Melatonin also has been discovered in many other non-vertebrate organisms, including plants (Kolár and Machácková, 2005), dinoflagellates (Balzer and Hardeland, 1991), and fungi (Hardeland, 1999). However, the expression of melatonin in most invertebrates does not show obvious rhythmicity (Tanaka et al., 2007).
The effect of melatonin on locomotor behavior has been studied extensively in animals, especially in vertebrates. Melatonin has been shown to significantly reduce the locomotor activity in hamsters (Golombek et al., 1992) and have a sedative-like effect in cats, rats, and mice (Golombek et al., 1996). Locomotor inhibition by melatonin in the brain of rodents may be mediated by the central gamma aminobutyric acid (GABA) ergic system (Golombek et al., 1996). Similarly, it induces birds, fish and flatworms to fall asleep (Mintz et al., 1998; Zhdanova et al., 2008; Omond et al., 2017). Fewer studies of the effects of melatonin on locomotor behavior of invertebrates have been conducted. In a study of crickets, motor activity was reduced during the light-dark cycle as the melatonin concentration increased (Yamano et al., 2001). In another study of crayfish, melatonin had different effects on locomotor behavior depending on time of day of the melatonin injection: increased activity when treated during mid-photophase, no effect at late photophase, and decreased activity in early scotophase (Tilden et al., 2003a). Analogous results were reported for the fiddler crab Uca pugilator, which suggests that this feature is common in arthropods (Tilden et al., 2003b). Administration of exogenous melatonin also decreased locomotion rates in nematodes (Tanaka et al., 2007). However, the effect of melatonin on locomotor performance in other invertebrates, such as echinoderms, and how it might function remain unclear.
The sea cucumber Apostichopus japonicus is widely distributed along the shallow coast of northeastern Asia (Liao, 1980), and it is exploited as an important fishery resource in China, Japan, Korea, and Russia (Sloan, 1985). It also is a delicacy and a traditional medicine in China (Yang et al., 2015). As one of the most important benthic creatures in the marine environment in these areas, the activities of A. japonicus, including feeding, moving, and reproducing, may have a significant influence on the energy cycle of the marine ecosystem (Pan et al., 2015). The locomotor velocity of sea cucumbers is very slow (<3 mm/s) (Qiu et al., 2014; Pan et al., 2015). In addition, the coelomic cavity between the visceral mass and body wall is filled with coelomic fluid (Eliseikina and Magarlamov, 2002). Many types of coelomocytes are suspended in the coelomic fluid and may play important roles in the physiological processes of sea cucumbers (Eliseikina and Magarlamov, 2002). These features make sea cucumbers an ideal model system to investigate the behavioral endocrinology of marine invertebrates.
In living organisms, a complete and sophisticated regulation system and a complex metabolic network are involved in the production and regulation of the matter and energy that are necessary to support life. Most of the regulatory metabolites are small molecules that are present throughout the organism. Their concentrations are small, but their biological effects are powerful. These molecules are the physical basis for regulation of the neuroendocrine and immune systems in organisms. Metabolites are the final products that generated during living activities, and metabolomics analysis is study of these downstream molecules (Sharfstein et al., 2007). Metabolomics is an important tool for systems biology, as it provides the ability to measure the concentrations of endogenous small molecules in biofluids and characterize the quantitative changes of the metabolites that occur in specific physiological states of organisms (Nicholson et al., 1999; Sun et al., 2017). Ultraperformance liquid chromatography and quadrupole time-of-flight mass spectrometry (UPL-Q-TOF-MS) is a high resolution and sensitive metabolomics technology that can detect multiparametric metabolite profiles from biofluids or tissues rapidly and effectively (Wilson et al., 2005; O’Connor and Mortishire-Smith, 2006). This technology has been successfully applied to assess the metabolomic function of muscles in sea cucumbers between breeding and non-breeding stages (Ru et al., 2017), to study metabolic characteristics of intestinal fistula and obesity in humans (Yin et al., 2006; Kim et al., 2010), and to measure blood constituents in rats after treatment with traditional Chinese medicine (Wang et al., 2008, 2018).
The goals of the present study were to show the presence of melatonin in A. japonicus, evaluate its effect on locomotor behavior in this sea cucumber, and identify potential metabolic mechanisms involved in this effect. UPL-Q-TOF-MS metabolomic detection was performed on muscle tissues of sea cucumbers to identify the key metabolites and potential pathways that are involved in the effect of melatonin administration on locomotor activity. In order to verify the final effects of potentially changing signaling pathways on muscles, free fatty acid (FFA) and adenosine triphosphate (ATP) concentrations in the muscle tissue were measured as well.
Materials and Methods
Animals Rearing and Melatonin Detection
Sea cucumbers (A. japonicus) were obtained from the marine ranch of Laizhou Bay (37°18.382′N, 119°40.853′E) in China’s Bohai Sea. They were maintained in 1000 L aquariums containing aerated sand-filtered seawater (salinity 30‰, pH 8.0) and placed in a 12 h light (0700 h—1900 h)/12 h dark (1900 h—0700 h) condition for 2 weeks prior to use in the study. The water temperature and dissolved oxygen content were maintained at 16 ± 0.5°C and >6.0 mg/L, respectively. Sea cucumbers were fed an excess self-made diet (30% Sargassum powder, 70% sea mud) once a day at 0800 h. Before feeding, uneaten food and feces were siphoned carefully, then half of the tank water was exchanged to keep high water quality. Twenty four sea cucumbers (97.7 ± 14.8g) were selected to measure the concentration of melatonin in the coelomic fluid using a commercial melatonin ELISA kit (Zcibio, Shanghai, China). Before dissection, sea cucumbers were anesthetized using magnesium sulfate (0.4 M/L), which is considered to be an effective method with minimal negative effects on this species (Zhou et al., 2014). After each sea cucumber was dissected, 1 mL of coelomic fluid was pumped into a sterile tube, and tubes were stored in liquid nitrogen until use in the ELISA test. These samples were divided into eight groups (C1–C8) randomly and each three samples in one group were mixed together as one sample to determine the melatonin concentration based on the premise that an unknown amount of melatonin competes with a fixed amount of enzyme-labeled antigen for the binding sites of antibodies coated on the wells of the assay plate. Standards with precise concentrations were used in this detection procedure. Buffers, incubation times, shaking processes, and temperatures were used or set according to the kit instructions. Finally, absorbance was measured using a multifunctional microplate reader at 450 nm (Varioskan Flash, Thermo Scientific, Vantaa, Finland).
Melatonin Administration and Locomotor Behavior Tests
After ELISA test, other 48 sea cucumbers were weighed and divided randomly into four groups (98.4 ± 16.2g, n = 12 sea cucumber/group) representing the three melatonin concentrations and the control. Melatonin (Solarbio Life Sciences, Beijing, China) was dissolved in 5% ethanol and diluted with sterile sand-filtered seawater to reach the required concentrations (10, 100, and 1000 μM). Sterile sand-filtered seawater with an equivalent amount of ethanol was used as the control. These experimental concentrations were set by reference to the relative study of melatonin affection on locomotor and feeding behavior (Pinillos et al., 2001; Azpeleta et al., 2010). The experiments were conducted in aquariums (50 cm × 50 cm × 50 cm), and the water line was maintained at 30 cm. A white acrylic board was placed at the bottom of each aquarium to make the sea cucumber easier to detect by the analysis software. An infrared camera (Hikvision, Hangzhou, China) fixed by plastic frames was installed above each aquarium. The photoperiod was identical to the condition of previous 2 weeks temporary cultivation. To maintain a soft and uniform light during the experiment, softboxes were placed around the aquariums, and all experimental facilities were enclosed by a black-out cloth. Before the locomotor behavior recording, sea cucumber individuals were placed in the test tank for at least 24 h to become familiar with the experimental environment. For the experiment, sea cucumbers were injected with 0.1% (v/w) (Kato et al., 2009) of a given melatonin concentration or the control via peripheral injection at 2200 h, so the zeitgeber time is 15 (ZT15) in our study. There are two reasons for why the injection made at this time point: (1). Sea cucumbers are very sensitive to lights (Dong et al., 2010; Sun et al., 2018), and their physiological status and activities are totally adjusted to dark at 3 h after turning to dark. (2). Previous study demonstrated that 2200 h is the beginning time of feeding peak (Sun et al., 2015), and the period of locomotor peak (0300–0600 h) of our preliminary study was almost the same as feeding peak (0200–0600 h). This design allowed an increase in circulating melatonin during the peak of activity in sea cucumber. The injections were performed with a 1 mL ICO syringe (JIANSHI®, Luohe, China) and a 0.3 mm Microlance needle, and they were done under dim red light conditions (Pinillos et al., 2001).
Locomotor activities were recorded by the infrared camera of Hikvision (DS-2CD3310D-I, 4MM, Hangzhou, China) for 12 h, and videos were analyzed using Ethovision (version 10.1) software (Noldus Inc., Netherlands). To evaluate the effect of melatonin on locomotor performance of the sea cucumbers, the following behaviors before and after melatonin injection were analyzed and counted: total distance traveled, cumulative duration of movement, mean and maximum velocity, total steps taken, mean and maximum stride (mean and maximum length of steps), and stride frequency (number of steps taken per minutes).
Muscle Tissue Collection, UPLC-Q-TOF-MS Detection
The locomotor activity data were used to identify the time point and melatonin treating concentration at which a significant change in locomotor behavior occurred, and 24 sea cucumbers were collected at 7 h after administration and divided into 8 specimens averagely to acquire the tissues and measure the metabolites present in control (CON) and 1000 μM melatonin-treated (MEL) groups respectively. Sea cucumbers were anesthetized using magnesium sulfate (0.4 M/L). For each specimen, ∼2 g of longitudinal muscle tissue was carefully sheared off from the inner layer of the body wall and washed with ultrapure water. The samples were placed in a pipe and stored in a −80°C freezer prior to metabolomic analysis.
Before the extraction of metabolites, the samples from the −80°C freezer were stored for 30 min at −20°C and then thawed in a refrigerator at 4°C. For each specimen, 25 mg of muscle tissue were added to an Eppendorf tube containing 800 μl of ice-cold methanol and two small steel balls. All samples were ground using a TissueLyser (Tiangen, Beijing, China) at 50 Hz for 4 min. After grinding, the steel balls were removed, the samples were precipitated overnight at −20°C, and then they were centrifuged at 30000 × g for 20 min at 4°C. Finally, 550 μl of supernatant were collected from each sample and transferred to a new Eppendorf tube for subsequent UPLC-Q-TOF-MS analysis.
All samples were acquired by the LC-MS system following the manufacturer’s instructions. First, all chromatographic separations were performed using the UPLC system (Waters Corp., Manchester, United Kingdom). An ACQUITY UPLC BEH C18 column (100 mm × 2.1 mm, 1.7 μm, Waters) was used for the reversed phase separation. The column oven was maintained at 50°C. The flow rate was 0.4 mL/min, and the mobile phase consisted of solvent A (water + 0.1% formic acid) and solvent B (acetonitrile + 0.1% formic acid). Gradient elution conditions were set as follows: 0 – 2 min, 100% phase A; 2–11 min, 0–100% B; 11–13 min, 100% B; and 13–15 min, 0–100% A. The injection volume for each sample was 10 μl.
A high-resolution tandem mass spectrometer Xevo G2 XS QTOF (Waters) was used to detect metabolites eluted form the column. The Q-TOF was operated in both positive and negative ion modes. For positive ion mode, the capillary and sampling cone voltages were set at 3 kV and 40 V, respectively. For negative ion mode, the capillary and sampling cone voltages were set at 2 kV and 40 V, respectively. The MS data were acquired in Centroid MSE mode. The TOF mass range was 50–1200 Da, and the scan time was 0.2 s. For the MS/MS detection, all precursors were fragmented using 20 – 40 eV, and the scan time was 0.2 s. During the acquisition, the LE signal was acquired every 3 s to calibrate the mass accuracy. To evaluate the stability of the LC-MS during the whole acquisition, a quality control sample (pool of all samples) was acquired after every 10 samples.
ATP and FFA Tests
The ATP levels in muscle tissues were measured using a luciferase-based enhanced ATP assay kit (S0027, Beyotime Biotechnology, Shanghai, China). In brief, the muscle tissues were fully dispersed, ground using a homogenate machine (BRS-200, BUNKIN, Anhui, China), and incubated in lysis buffer for 10 min. The mixtures were centrifuged for 5 min at 4°C and 12,000 × g, and each supernatant was collected in a new tube. Subsequently, 100 mL of detection solution were added to a 96-well plate and incubated for 5 min at room temperature. Next, 20 mL of each supernatant were added to the plate and mixed quickly with the detection solution. Luminescence was detected using the Varioskan Flash multifunctional microplate reader within 30 min. The concentration of ATP was calculated according to an ATP standard curve, and results were expressed as nmol/mg. Besides, a FFA assay kit was used to measure the FFA concentration in muscle tissues according to the kit instructions. FFA concentrations were calculated according to an FFA standard curve (y = 0.0038x + 0.0055, R2 = 0.994), and results were expressed as nmol/g.
Statistical Analysis
Behavioral data acquired from video analysis and the ATP and FFA concentrations in muscle tissues were analyzed using one-way analysis of variance followed by Tukey’s post hoc multiple comparison tests. SPSS 20.0 software was used to conduct the analyses. A probability level of p < 0.05 was considered to be statistically significant.
The raw UPLC-Q-TOF-MS data were imported into Progenesis QI software to generate a data matrix that included retention time (RT), mass-to-charge ratio (m/z) values, and peak intensity. The main parameters were at default settings. After eliminating low-weight ions (Relative Standard Deviation > 30%), the quality control-based robust LOESS signal correction method was used to correct the data. To identify the key metabolites that differed between the CON and MEL groups, principal component analysis (PCA), partial least squares discriminant analysis (PLS-DA), fold-change (FC) analysis, and the t-test were performed on the UPLC-MS data. PCA is a descending dimension method in which multiple variables are transformed into a few key variables that can mirror the original overall variable information. PLS-DA is a multivariate statistical analysis method that is widely used in the analysis of metabolomics data. As a supervised analysis method, PLS-DA can provide an extremely comprehensive reflection of the distinction among the experimental samples (Boulesteix and Strimmer, 2007). In this model, parameter R2 denotes the explanation capacity and Q2 represents the predictive rate of the model. The variable importance of projection (VIP) values were calculated in the model. Potential metabolic biomarkers satisfy three conditions (VIP > 1, FC > 1.2/ < 0.8333, q < 0.05), and those that met these criteria were chosen as key metabolites with significant changes. Ultimately, significant metabolites were searched in the Kyoto Encyclopedia of Genes and Genomes biochemical databases to annotate related metabolic pathways.
Results
Existence of Melatonin in Coelomic Fluid of A. japonicus
Melatonin was present in the coelomic fluid of normal A. japonicus at concentrations ranging from 120.01 to 165.38 ng/L (Table 1). The average concentration was 135.68 ng/L.
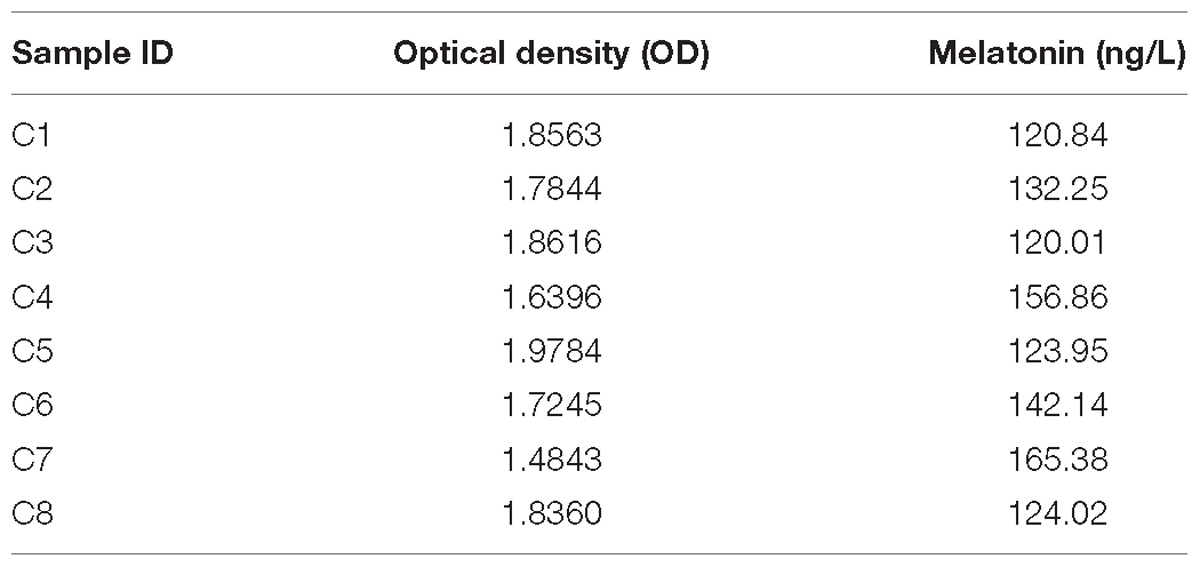
Table 1. Concentrations of melatonin in the coelomic fluid of A. japonicus based on the method of ELISA test.
Effect of Melatonin on Locomotor Behavior of A. japonicus
Figure 1 shows the locomotor performance (distance moved, cumulative duration of moving, and mean and maximum velocity) of A. japonicus in the experiment. The total distance moved by sea cucumber during the 9 h after melatonin administration significantly decreased with increasing melatonin dose, ranging from 22.53 ± 4.35 m in the control group to 9.93 ± 2.57 m in the 1000 μM melatonin group (Figure 1A; F3,12 = 12.828, p < 0.01). The distances moved within each hour interval before and after melatonin administration also decreased with increased melatonin concentration, and the maximum difference between control and treatments occurred at 7 h after injection (Figure 1B). Moreover, in the 100 and 1000 μM treatment groups, no typical peak point was observed after melatonin injection (Figure 1B). These results illustrate a declining trend in motility after melatonin administration, which explains the decreased cumulative duration of locomotion overall (Figure 1C; F3,12 = 13.101, p < 0.01). However, the velocity of motor behavior was low in A. japonicus. Although the mean and maximum velocities decreased to some extent with increased melatonin dose, the decreases were not statistically significant (Figure 1D; F3,12 = 7.865, p > 0.05).
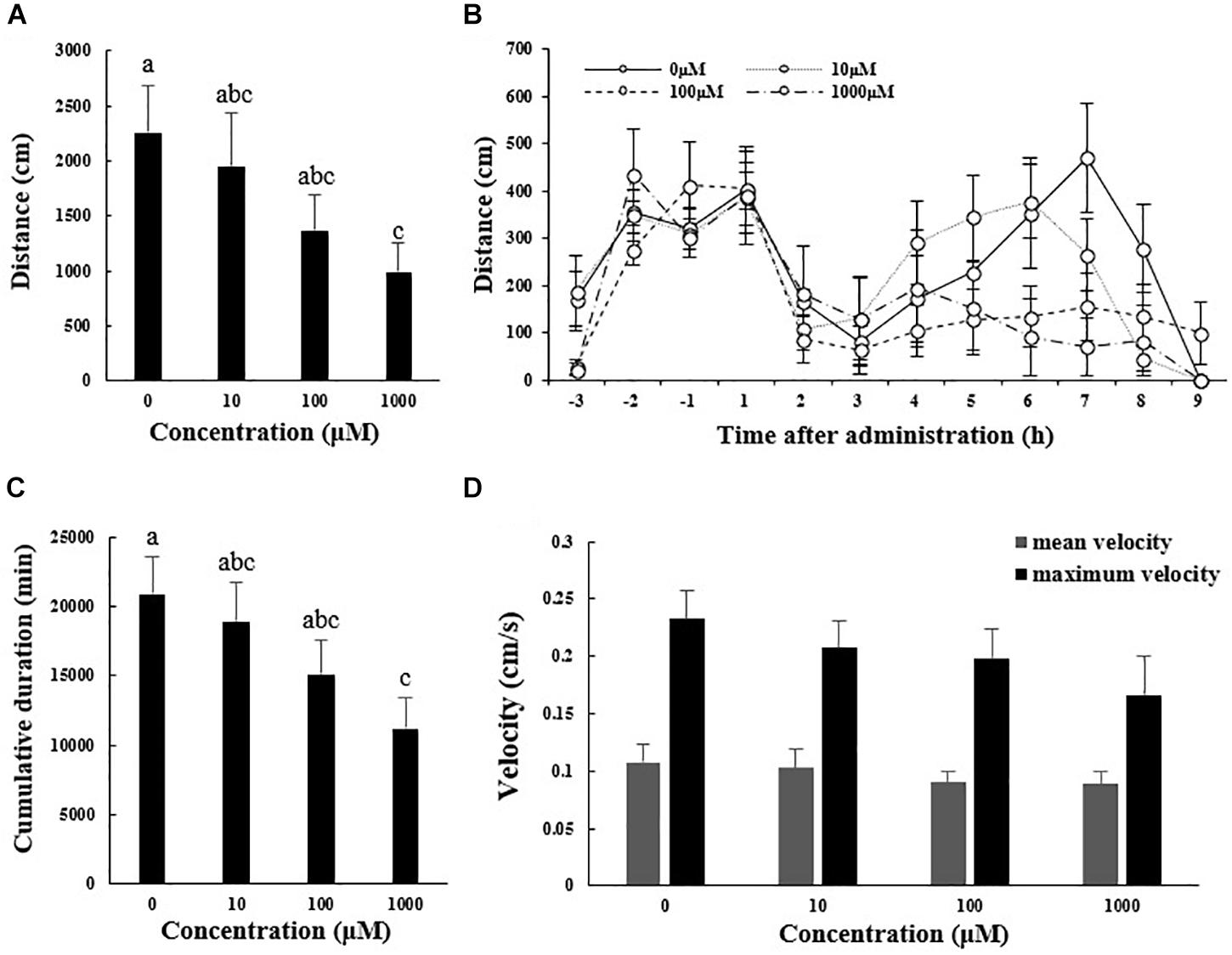
Figure 1. Total distance traveled (A), cumulative duration of movement (C), and mean and maximum velocity (D) for A. japonicus in the different treatment groups 9 h after intraperitoneal injection with melatonin or the control and distances moved within each hour interval before and after injection with melatonin or the control (B). Results are shown as the mean ± SEM. Different letters indicate significant differences (N = 12, p < 0.05).
Figure 2 shows results for other measures of locomotor performance (number of steps taken, mean stride, and stride frequency). The total number of steps taken during 9 h after melatonin administration decreased from 465.17 ± 78.49 in the control group to 233.17 ± 62.14 m in the 1000 μM melatonin group (Figure 2A; F3,12 = 10.919, p < 0.01). The number of steps taken within each hour interval before and after melatonin injection showed a curve similar to that for distance traveled (Figure 2B). Mean stride and stride frequency decreased with increasing melatonin dose, but the differences were not statistically significant (Figures 2C,D).
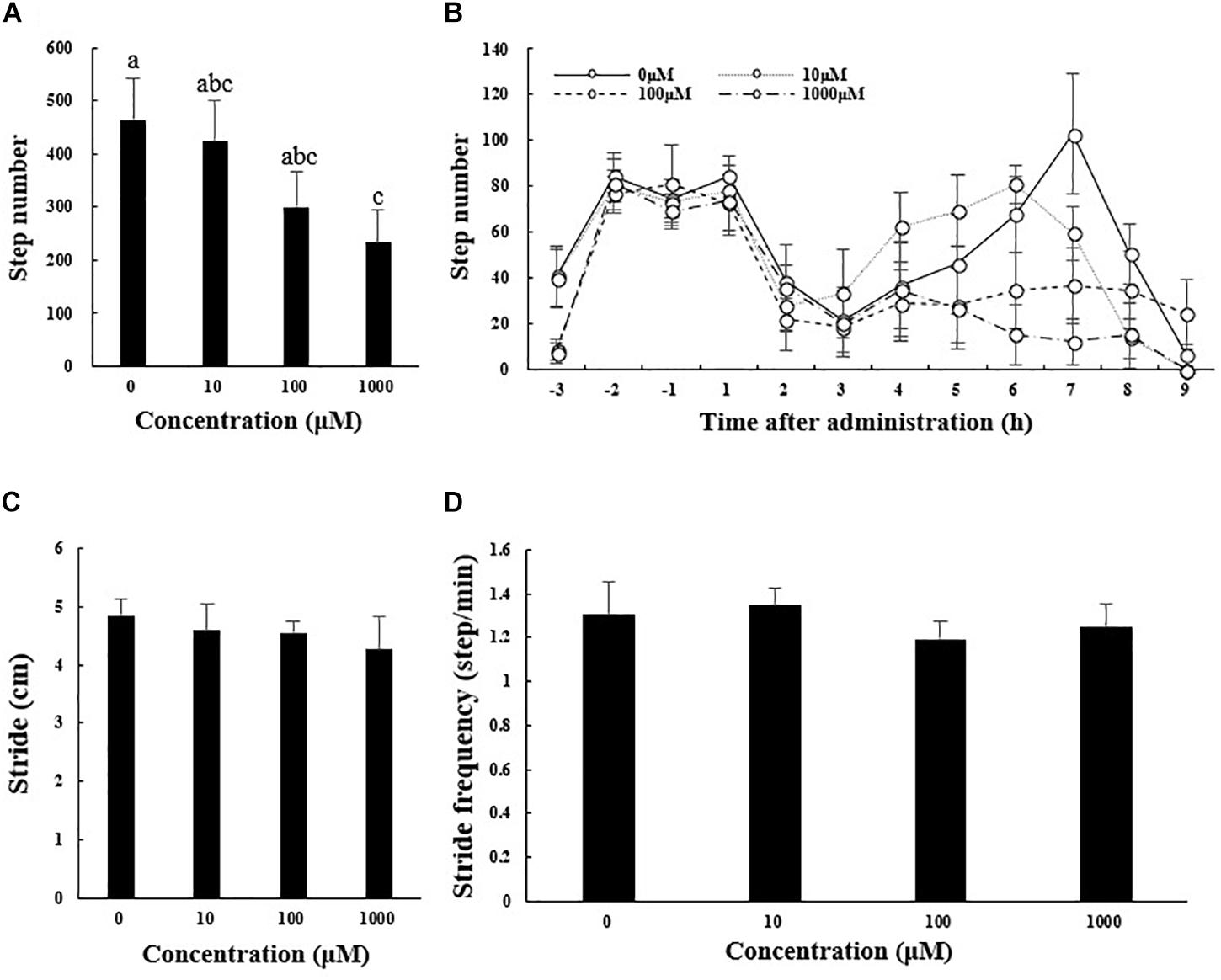
Figure 2. Total number of steps taken (A), stride length (C), and stride frequency (D) of A. japonicus in the different treatment groups 9 h after intraperitoneal injection with melatonin or the control and the number of steps taken within each hour interval before and after injection with melatonin or the control (B). Results are shown as the mean ± SEM. Different letters indicate significant differences (N = 12, p < 0.05).
Effect of Melatonin on Muscle Physiology of A. japonicus
The abscissa and ordinate in the PLS-DA plot represent the first principal component (PC1) and the second principal component (PC2), respectively (Figure 3). The percentages in parentheses indicate the proportion of the variance in the raw data explained by a given principle component. Thus, for the positive ion mode, 5.50 and 18.37% of the variance in the data could be explained by PC1 and PC2, respectively. In the negative ion mode, the values were 7.73% for PC1 and 20.44% for PC2. Each dot represents one sample. The CON (red) and MEL (blue) groups are clearly separated from each other in both ion modes. In addition, the heat maps of overall differential metabolites from CON and MEL groups in positive (A) and negative (B) mode are shown in Figure 4. These two figures indicate differences in the metabolites in the muscles of these two groups.
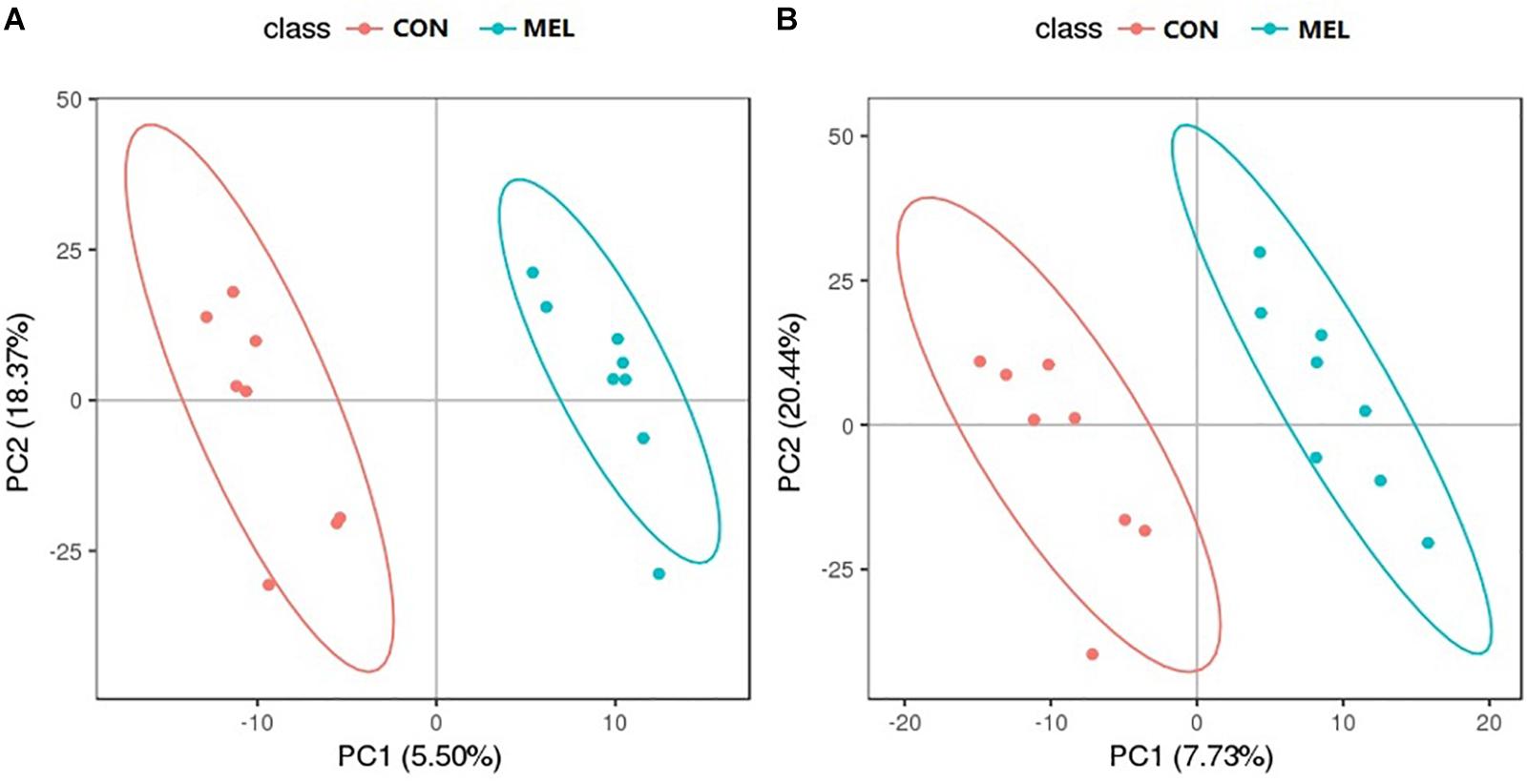
Figure 3. The partial least square discriminant analysis (PLS-DA) scores plot of muscle metabolites from the control (CON) and melatonin treated (MEL) groups in the positive ion (A) and negative ion (B) scan modes. The abscissa and ordinate represent the first principal component (PC1) and the second principal component (PC2) respectively.
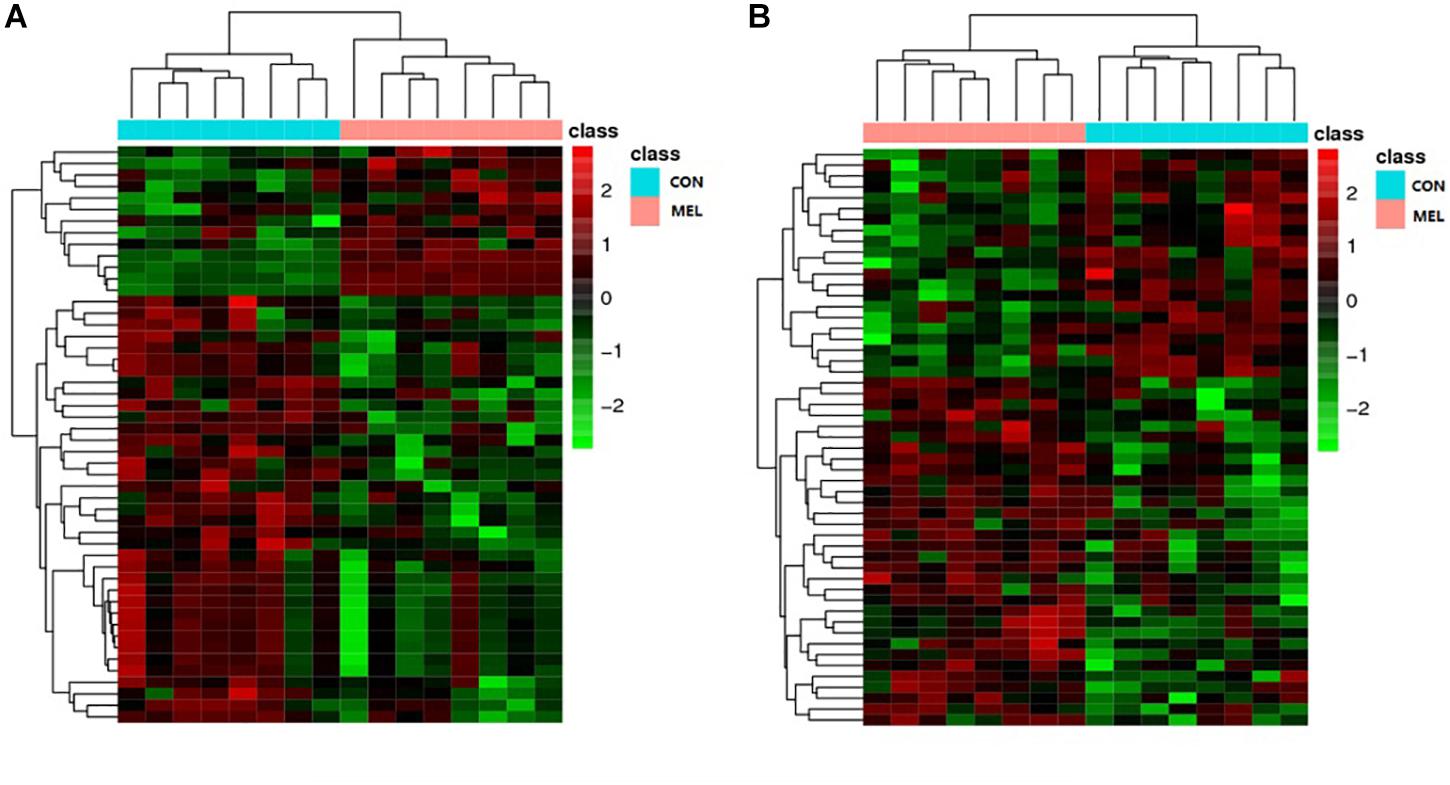
Figure 4. The heat maps of overall differential metabolites from CON and MEL groups in positive (A) and negative (B) mode. Each line represents a differential metabolite and each cross represents a muscle sample. Different colors represent different higher abundance intensity (mean value acquired from all detected samples of the same group).
The t-test (p < 0.05) and PLS-DA model (VIP > 2.0) results together identified 22 metabolites from the positive and negative ion modes that differed between the CON and MEL groups (Table 2). These metabolites included (3S,6E)-nerolidol, serotonin, melatonin, flavin mononucleotide, retinoic acid, indole, and cinnamaldehyde. (3S,6E)-nerolidol and melatonin were significantly upregulated in the MEL group, whereas the other metabolites were downregulated. These metabolites are mainly involved in the peroxisome proliferator-activated receptor (PPAR) signaling pathway, oxidative phosphorylation, gap junction, arachidonic acid metabolism, protein digestion and absorption, and other metabolic pathways.
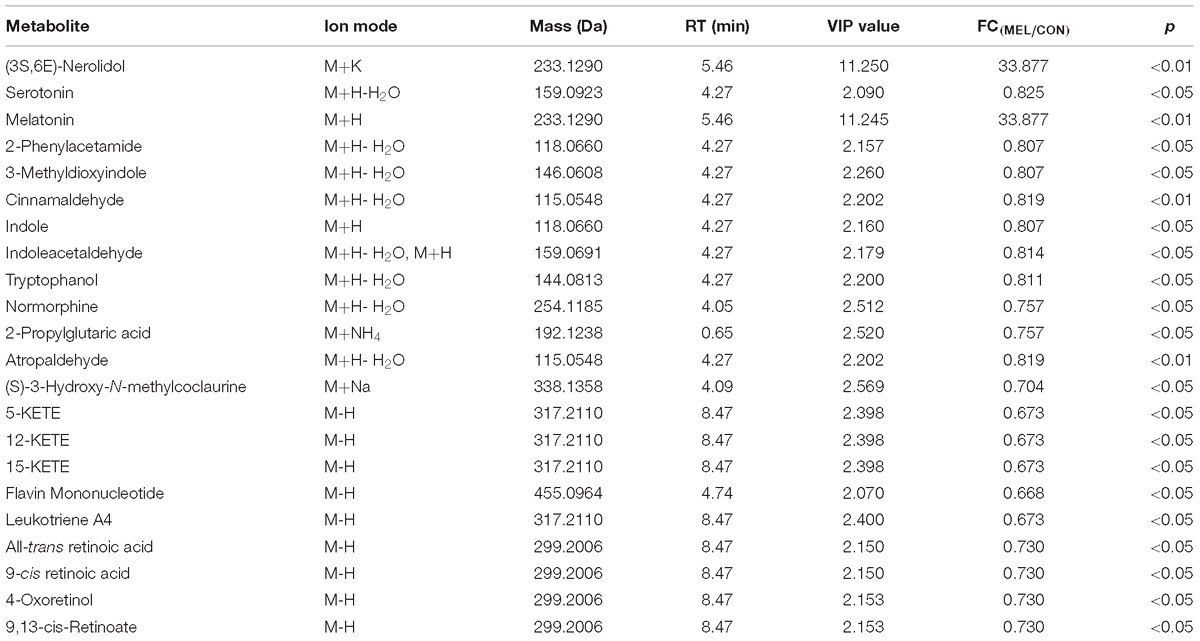
Table 2. Muscle metabolites with concentrations that differed significantly between the MEL and CON groups.
FFA and ATP Content in the Muscle of A. japonicus
To investigate the condition of the energy supply in muscles of sea cucumbers in the CON and MEL groups, the concentrations of FFA and ATP in muscle tissue were measured (Table 3). The concentration of ATP in the muscles of sea cucumbers after melatonin administration was significantly lower than that in the control group (p < 0.05), whereas the FFA concentration in the MEL group was higher than that in the CON group, although the difference was not statistically significant.
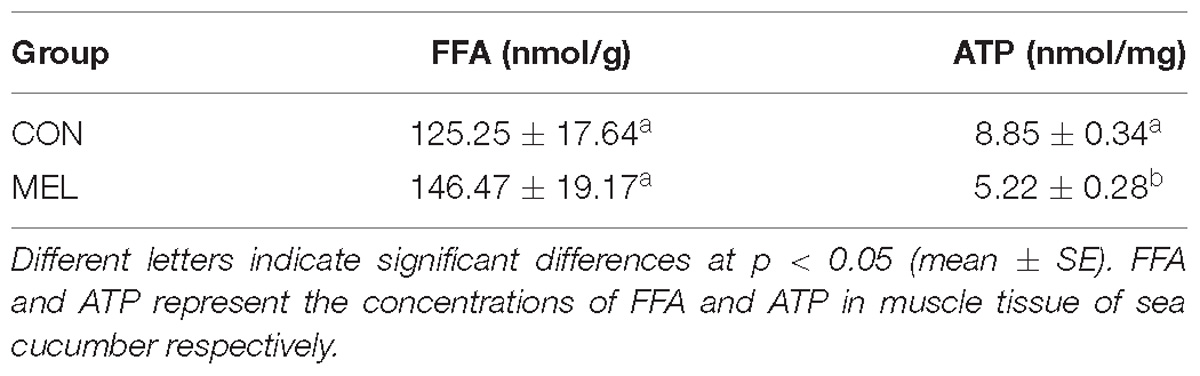
Table 3. Concentrations of FFA and ATP in the muscle tissue of sea cucumbers in the MEL and CON groups.
Discussion
Existence of Melatonin in A. japonicus
Our study demonstrated the existence of melatonin in the coelomic fluid of A. japonicus. Many types of coelomocytes are suspended in the coelomic fluid, and they are involved in immunity, trephocytic activities, and nutrient transport (Eliseikina and Magarlamov, 2002). Considering the function of coelomic fluid in the biological system of the sea cucumber, it is reasonable to speculate that melatonin could be present in many other tissues or organs, such as the intestine, gonad, and body wall. To the best of our knowledge, only a few studies have investigated melatonin in echinoderms, despite their important phylogenetic and ecological status. Endogenous melatonin was detected in the gonad of the sea star Echinaster brasiliensis, and its production peaked at night (Peres et al., 2014). The enzymes arylalkylamine N-acetyltransferase and tryptophan hydroxylase were proposed as possible regulators of the production of melatonin in this species (Peres et al., 2014). In addition, three melatonin receptor sequences were identified from the genome of the sea urchin Strongylocentrotus purpuratus (D’Aniello et al., 2015). Therefore, we hypothesize that melatonin is a common molecule in echinoderms, which further supports the premise that melatonin is a phylogenetically ubiquitous molecule in organisms with a deep evolutionary origin (Poeggeler et al., 1991).
Effect of Melatonin on Locomotor Performance of A. japonicus
Our results strongly support the premise that melatonin is involved in regulation of locomotor activities in A. japonicus, particularly locomotor endurance. The decrease of total distance traveled, total steps taken, and cumulative duration of movement with increasing dosage of melatonin suggests that melatonin can greatly reduce the endurance of movement in this species. The effect of melatonin on locomotor activity depends on the location of administration, time of administration, and circadian rhythms (diurnal or nocturnal) (López-Olmeda et al., 2006; Azpeleta et al., 2010). Melatonin decreased the locomotor endurance in scotophase and eliminated the locomotor activity cycles in the crayfish Procambarus clarkii (Tilden et al., 2003a) and the fiddler crab Uca pugilator (Tilden et al., 2003b). Although the locomotor circadian rhythm of sea cucumbers is still unclear, considering the administration time point, our results are consistent with results of studies of other invertebrates. Decreased locomotion has also been widely observed after exogenous application of melatonin in vertebrate species, including fishes (Zhdanova et al., 2001; López-Olmeda et al., 2006; Azpeleta et al., 2010), reptiles (Hyde and Underwood, 2000), birds (Murakami et al., 2001), mammals (Zhdanova, 2005), and platyhelminthes (Omond et al., 2017). These results all support the idea that the administration of melatonin caused sleep-like behavior in diverse species.
In addition, the average and maximum velocity, mean stride length, and stride frequency of A. japonicus in the control group were higher than those in the treatment group, indicating that melatonin can decrease the movement efficiency of this species. This result coincides with a previous result reported for an invertebrate, the nematode Caenorhabditis elegans. The locomotion rates of C. elegans significantly decreased after administration of exogenous melatonin (Tanaka et al., 2007). By means of genetics and mutant strains, melatonin acted as a direct neuromodulator by regulating locomotor activity in C. elegans (Tanaka et al., 2007).
In summary, results of the present study indicate that melatonin diminished locomotor behavior—more precisely, locomotor endurance and efficiency—in A. japonicus. This finding provides evidence for the effect of melatonin on the locomotor behavior of sea cucumbers.
Potential Mechanisms Involved in the Decrease of Locomotor Performance of A. japonicus After Melatonin Administration
To date, most studies of the effect of melatonin on locomotor behavior have focused on the alteration of behavior, and little attention has been paid to the potential physiological changes involved in locomotor influence by melatonin. In present study, we selected the time point at which we observed maximum differentiation in locomotor performance between control and melatonin-treated sea cucumbers (7 h after melatonin administration) and measured metabolite levels in organisms each group. Treated sea cucumbers and control sea cucumbers had different muscle metabolite profiles, and it was clear that a combination rather than a single metabolic pathway resulted in the loss of locomotor performance. In addition to higher levels of melatonin in treated animals, which probably was due to the exogenous melatonin administration, serotonin, flavin mononucleotide, 9-cis-retinoic acid, and all-tans retinoic acid were downregulated. These metabolites are categorized into four main pathways: metabolic, gap junction, oxidative phosphorylation, and PPAR signaling. These metabolites and the pathways in which they participate suggest a potential mechanism by which melatonin caused the decreased locomotor performance in A. japonicus.
Serotonin is an indole derivate that is widely distributed in the tissues of animals and plants (Nässel, 1988; Murch et al., 2001). It is speculated to be one of the most critical, but poorly understood transmitters involved in many brain functions, such as mood, eating, and sleep, as well as depression and some other psychiatric disorders (Uchida and Cohen, 2017). In echinoderms, serotonin is demonstrated to be contained in the oocytes, zygotes, blastomeres and adults of starfishes (Squires et al., 2010). Previous studies have also reported the distribution and concentrations of serotonin and serotonin-like compounds in the embryos of sea urchins (Renaud et al., 1983; Morikawa et al., 2001; Shmukler and Tosti, 2001; Buznikov et al., 2005). Furthermore, serotonin has mainly been identified biochemically in the central nervous system of many invertebrates (Welsh and Moorhead, 1960; Fujii and Takeda, 1988), where it is a neurotransmitter that acts as a messenger in the “communication” of the nervous system (Nässel, 1988). A number of studies revealed that serotonin stimulates or triggers the locomotor behavior of animals, including rats (Cabaj et al., 2017), fish (Byrne, 1970), mollusks (Pavlova, 2001), and marine trematodes (Tolstenkov et al., 2017). At the cellular and behavioral levels, serotonin is considered to be a powerful modulator rather than a mediator that takes part in vertebrate motor function (Jacobs and Fornal, 1997; Feraboli-Lohnherr et al., 1999). In C. elegans, the motor circuits in chemical synapses, gap junctions, and neuromuscular junctions are mediated by serotonin and dopamine, and this is the pathway that C. elegans uses to modulate motor behavior (Sawin et al., 2000; Cao et al., 2005; Chase and Koelle, 2007). Consumption of serotonin by the central nervous system resulted in increased locomotor activity in a number of mammals, possibly due to the direct effect of serotonin on the locomotor system (Jacobs and Fornal, 1997). In contrast, systematic melatonin administration restrained the release of serotonin in the brain and led to a reduction of locomotor performance in rats (Chuang and Lin, 1994). This effect of melatonin on motor behavior agrees with our results for A. japonicus. In addition, the study of locomotion in snails Helix lucorum revealed that the length of pedal waves (stride) increased after serotonin injection (Pavlova, 2001). Although the difference was not significant, the stride length in the melatonin-treated group of A. japonicus decreased with increasing melatonin dose, which would occur as a result of reduced serotonin level. In our study, metabolomic results revealed a significant reduction of serotonin in muscles after melatonin administration. Based on the depressed locomotor performance of sea cucumbers in the melatonin-treated group and the known modulatory role of serotonin in the locomotor system, we suggest that downregulation of serotonin was an important contributor to the decreased locomotor performance observed in melatonin-treated sea cucumbers.
There is an interrelationship between melatonin and serotonin systems (Gaffori and Van Ree, 1985) and serotonin is involved in the synthesis of melatonin (Sprenger et al., 1999). The levels of serotonin are high in the daytime and decreased at night, which is contrary to the rhythm of melatonin (Ganguly et al., 2002). Treating with melatonin caused a dose-dependent reducing of serotonin release (Chuang et al., 1993). Injection of serotonin antagonists (methysergide and cyproheptadine) gave rise to decreased locomotor activity as was found after administration of melatonin (Gaffori and Van Ree, 1985). The melatonin production pathway in starfish E. brasiliensis is likely to be the same as previously described in vertebrates by enzymatic assays (Peres et al., 2014). The increase of arylalkylamine N-acetyltransferase (AANAT) activity reduces the nocturnal serotonin levels and results in an increase in the intracellular concentration of N-acetylserotonin (NAS), which will convert to melatonin by hydroxyindole-O-methyltransferase (HIOMT) (Ganguly et al., 2002). In addition, AANAT activity can be finely modulated and decreases sharply when sympathetic stimulation is ceased by treating with adrenergic antagonists or nocturnal photostimulation (Deguchi and Axelrod, 1972; Klein and Weller, 1972; Parfitt et al., 1976). Hence, the depression of melatonin on serotonin is likely to be mediated by AANAT activity. However, melatonin is highly pleiotropic (Hardeland et al., 2006), which can also be demonstrated by our results of metabolomic data, and even the gene of AANAT was not blasted from the genome of A. japonicus, which suggest a special relationship between melatonin and serotonin in this species. Therefore, internal mechanisms for the decrease of serotonin after melatonin administration still need further studies, especially in A. japonicus.
9-cis-retinoic acid (9cRA) and all-trans retinoic acid (atRA) are all bioactive retinoids that belong to vitamin A metabolites (Heine et al., 2018). 9cRA is generated from atRA by spontaneous isomerization in vivo (Yu et al., 2017; Heine et al., 2018) and they can regulate metabolism by activating two specific nuclear receptors——retinoic acid receptor (RAR) and retinoic X receptor (RXR) (Egea et al., 2000; Ziouzenkova and Plutzky, 2008; Abed et al., 2017). Interestingly, RARs can bind both atRA and 9cRA, whereas RXRs exclusively binds 9cRA (Heyman et al., 1992; Egea et al., 2000; Abed et al., 2017). Retinoic acid signaling is relayed through these two nuclear receptors (Viera-Vera and García-Arrarás, 2018). For a long time, this signaling was regarded as a chordate innovation, the presence and function of it in invertebrates are therefore rarely studied (Gutierrez-Mazariegos et al., 2014). In echinoderms, RAR have ever been identified in sea urchins. Besides, the sequences of RAR and RXR as well as their isoforms are recently identified and characterized in the sea cucumber Holothuria glaberrima, and both retinoid receptors are expressed in all sampled tissues including muscles (Viera-Vera and García-Arrarás, 2018). Moreover, retinoic acid signaling is also existed in the starfish Patiria pectinifera, and serves as a regulator in metamorphosis process (Yamakawa et al., 2018). As an obligate heterodimeric partner for other nuclear receptors, including PPARs (α, δ/β, and γ), which are ligand-activated transcription factors that regulate gene expression (Ziouzenkova et al., 2002), RXR helps coordinate energy balance (Ziouzenkova and Plutzky, 2008). PPARα heterodimerizes with RXRβ, and they cooperate to activate the acyl-CoA oxidase gene promoter (Keller et al., 1993). Due to the rate-limiting enzyme of the peroxisomal β-oxidation pathway of fatty acids is encoded by the acyl-CoA oxidase gene, PPARs and RXRs play an important role in lipid metabolism (Dreyer et al., 1992; Tugwood et al., 1992; Keller et al., 1993). Since the presence of PPARs and RXRs in echinoderms (Pagliarani et al., 2012; Viera-Vera and García-Arrarás, 2018; Yamakawa et al., 2018), this process of lipid metabolism regulation is likely to be existed in sea cucumbers. According to our result, 9cRA and atRA were downregulated in the muscles of melatonin-treated sea cucumbers. The reduced 9cRA and atRA levels may decreased the formation of ligand/receptor complex of PPARs and RXR, downregulated their ability to activate the acyl-CoA oxidase gene, and thereby inhibited the peroxisomal β-oxidation pathway of fatty acids. In addition, the relatively high levels of FFAs may also indicate insufficient fatty acid consumption in the melatonin-treated groups. Because of the energy supply function of fatty acids, inadequate energy supply for muscles to contract for locomotion is predicted in sea cucumbers after melatonin administration.
Flavin mononucleotide (riboflavin-5-phospate, FMN) is an important organic cofactor in living cells and plays a vital role in biological systems (Yan et al., 2018). To be more precise, FMN is a coenzyme for flavoprotein, which can pass electrons and hydrogen atoms and is involved in the mitochondrial oxidative phosphorylation process in animals (Zheng et al., 1990; Sakai and Takahashi, 1996). It is well-know that the mitochondrial oxidative phosphorylation system is the final biochemical pathway that takes part in the production of ATP——the energy substrate for muscle contraction (Mommaerts, 1969; Smeitink et al., 2001). Actually, mitochondria generate most of the energy in cells needed for animal activity primarily through the oxidative phosphorylation process, in which electrons are passed along a series of respiratory enzyme complexes that are located in the inner mitochondrial membrane (Gibson and Hastings, 1962; Saraste, 1999). The energy produced by electron transfer is devoted to pumping protons across membrane, and then the electrochemical gradient produced from this process enables another complex, ATP synthase, to synthesize the energy carrier ATP (Saraste, 1999). In the melatonin-treated group of sea cucumbers in our study, FMN was significantly downregulated, and this would have had a negative effect on electron transfer, inhibited protons from crossing the membrane, and reduced the production of ATP in muscle. Locomotor behavior of sea cucumbers involves a relatively simple alternating pattern of contraction and extension of the body wall, which is under the control of muscle (Qiu et al., 2014). While ATP plays a fundamental role in supplying energy for muscle contraction (Saraste, 1999). We therefore suggest that this effect combined with the actual decrease of ATP in the muscles of sea cucumbers in the melatonin-treated group decreased the ATP supply for muscle contraction by oxidative phosphorylation and resulted in insufficient energy to support locomotor activity. Consequently, the hypodynamic muscles along the body wall of the sea cucumbers led to the significant decrease in the locomotor ability of treated sea cucumbers compared to control animals.
Conclusion
In this study, we detected melatonin in the coelomic fluid of A. japonicus and showed that melatonin had a sedative effect on this species. Locomotor endurance, including movement distance, number of steps taken, and cumulative duration of movement, significantly decreased with increasing dose of melatonin. Locomotor efficiency (e.g., velocity, stride length, and stride frequency) also decreased to some extent with increasing dose of melatonin. The metabolomic results combined with FFA and ATP data indicated that there are a number of potential physiological mechanisms that can explain the inhibitory effect of melatonin on locomotor endurance and efficiency of A. japonicus. These include reduction of the locomotor modulator — serotonin, inhibited fatty acid oxidation and disturbed oxidative phosphorylation.
Author Contributions
KD and LZ designed and performed the research. TZ and HY contributed new reagents and analytic tools. KD analyzed the data and wrote the manuscript. LZ and HY supervised the research. RB revised this manuscript.
Funding
This research was financially supported by the National Key Research and Development Plan (2016YFE0101500) (Sino-Australian Centre for Healthy Coasts), the National Natural Science Foundation of China (41676136), the Funding of Youth Innovation Promotion Association, CAS (2015163), the Creative Team Project of the Laboratory for Marine Ecology and Environmental Science, Qingdao National Laboratory for Marine Science and Technology (No. LMEES-CTSP-2018-1), Taishan Scholars Program.
Conflict of Interest Statement
The authors declare that the research was conducted in the absence of any commercial or financial relationships that could be construed as a potential conflict of interest.
Acknowledgments
We would like to thank Dr. Yang Pan and Ruiting Gu for help with behavioral video and data analysis. We are grateful to Qiming Feng for help with behavioral recordance of sea cucumber.
References
Abed, M., Alzoubi, K., Bhuayn, A. A. M., and Lang, F. (2017). Stimulation of phospholipid scrambling of the erythrocyte membrane by 9-Cis-retinoic acid. Cell Physiol. Biochem. 41, 543–554. doi: 10.1159/000457014
Azpeleta, C., Martínez-álvarez, R. M., Delgado, M. J., Isorna, E., and Pedro, N. D. (2010). Melatonin reduces locomotor activity and circulating cortisol in goldfish. Horm. Behav. 57, 323–329. doi: 10.1016/j.yhbeh.2010.01.001
Balzer, I., and Hardeland, R. (1991). Photoperiodism and effects of indoleamines in a unicellular alga, Gonyaulax polyedra. Science 253, 795–797. doi: 10.1126/science.1876838
Boulesteix, A. L., and Strimmer, K. (2007). Life Science & Mathematical & Physical Science. Oxford J. 8, 32–44.
Bubenik, G. A. (2002). Gastrointestinal melatonin: localization, function, and clinical relevance. Digest. Dis. Sci. 47, 2336–2348. doi: 10.1023/A:1020107915919
Buznikov, G. A., Peterson, R. E., Nikitina, L. A., Bezuglov, V. V., and Lauder, J. M. (2005). The pre-nervous serotonergic system of developing sea urchin embryos and larvae: pharmacologic and immunocytochemical evidence. Neurochem. Res. 30, 825–837. doi: 10.1007/s11064-005-6876-6
Byrne, J. E. (1970). Locomotor activity responses in juvenile sockeye salmon, Oncorhynchus nerka, to melatonin and serotonin. Can. J. Zool. 48, 1425–1427. doi: 10.1139/z70-241
Cabaj, A. M., Majczyński, H., Couto, E., Gardiner, P. F., Stecina, K., Sławinska, U., et al. (2017). Serotonin controls initiation of locomotion and afferent modulation of coordination via 5-HT7 receptors in adult rats. J. Physiol. 595, 301–320. doi: 10.1113/JP272271
Cao, S., Gelwix, C. C., Caldwell, K. A., and Caldwell, G. A. (2005). Torsin-mediated protection from cellular stress in the dopaminergic neurons of Caenorhabditis elegans. J. Neurosci. 25, 3801–3812. doi: 10.1523/JNEUROSCI.5157-04.2005
Chase, D. L., and Koelle, M. R. (2007). Biogenic amine neurotransmitters in C. elegans. WormBook 20, 1–15.
Chuang, J., and Lin, M. T. (1994). Pharmacological effects of melatonin treatment on both locomotor activity and brain serotonin release in rats. J. Pineal Res. 17, 11–16. doi: 10.1111/j.1600-079X.1994.tb00107.x
Chuang, J. I., Chen, S. S., and Lin, M. T. (1993). Melatonin decreases brain serotonin release, arterial pressure and heart rate in rats. Pharmacology 47, 91–97. doi: 10.1159/000139083
D’Aniello, S., Delroisse, J., Valero-Gracia, A., Lowe, E. K., Byrne, M., Cannon, J. T., et al. (2015). Opsin evolution in the Ambulacraria. Mar. Genomics 24, 177–183. doi: 10.1016/j.margen.2015.10.001
Deguchi, T., and Axelrod, J. (1972). Sensitive assay for serotonin N-acetyltransferase activity in rat pineal. Anal. Biochem. 50, 174–179. doi: 10.1016/0003-2697(72)90496-4
Do Carmo Buonfiglio, D., Peliciari-Garcia, R. A., Do Amaral, F. G., Peres, R., Araujo Nogueira, T. C., Afeche, S. C., et al. (2011). Early-stage retinal melatonin synthesis impairment in streptozotocin-induced diabetic wistar rats. Invest. Ophthalmol. Vis. Sci. 52, 7416–7422. doi: 10.1167/iovs.10-6756
Dong, G., Dong, S., Wang, F., and Tian, X.-L. (2010). Effects of light intensity on daily activity rhythm of juvenile sea cucumber, Apostichopus japonicus (Selenka). Aquac. Res. 41, 1640–1647. doi: 10.1111/j.1365-2109.2010.02534.x
Dreyer, C., Krey, G., Keller, H., Givel, F., Helftenbein, G., and Wahli, W. (1992). Control of the peroxisomal β-oxidation pathway by a novel family of nuclear hormone receptors. Cell 68, 879–887. doi: 10.1016/0092-8674(92)90031-7
Egea, P. F., Mitschler, A., Rochel, N., Ruff, M., Chambon, P., and Moras, D. (2000). Crystal structure of the human RXRα ligand-binding domain bound to its natural ligand: 9-cis retinoic acid. EMBO J. 19, 2592–2601. doi: 10.1093/emboj/19.11.2592
Eliseikina, M. G., and Magarlamov, T. Y. (2002). Coelomocyte morphology in the holothurians Apostichopus japonicus (Aspidochirota, Stichopodidae) and Cucumaria japonica (Dendrochirota, Cucumariidae). Russ. J. Mar. Biol. 28, 197–202. doi: 10.1023/A:1016801521216
Feraboli-Lohnherr, D., Barthe, J. Y., and Orsal, D. (1999). Serotonin-induced activation of the network for locomotion in adult spinal rats. J. Neurosci. Res. 55, 87–98. doi: 10.1002/(SICI)1097-4547(19990101)55:1<87::AID-JNR10>3.0.CO;2-#
Fujii, K., and Takeda, N. (1988). Phylogenetic detection of serotonin immunoreactive cells in the central nervous system of invertebrates. Comp. Biochem. Phys. C 89, 233–239. doi: 10.1016/0742-8413(88)90217-4
Gaffori, O., and Van Ree, J. M. (1985). Serotonin and antidepressant drugs antagonize melatonin-induced behavioural changes after injection into the nucleus accumbens of rats. Neuropharmacology 24, 237–244. doi: 10.1016/0028-3908(85)90080-2
Ganguly, S., Coon, S. L., and Klein, D. C. (2002). Control of melatonin synthesis in the mammalian pineal gland: the critical role of serotonin acetylation. Cell Tissue Res. 309, 127–137. doi: 10.1007/s00441-002-0579-y
Gibson, Q. H., and Hastings, J. W. (1962). The oxidation of reduced flavin mononucleotide by molecular oxygen. Biochem. J. 83, 368–377. doi: 10.1042/bj0830368
Golombek, D. A., Fernández Duque, D., De Brito Sánchez, M. G., Burin, L., and Cardinali, D. P. (1992). Time-dependent anticonvulsant activity of melatonin in hamsters. Eur. J. Pharmacol. 210, 253–258. doi: 10.1016/0014-2999(92)90412-W
Golombek, D. A., Pévet, P., and Cardinali, D. P. (1996). Melatonin effects on behavior: possible mediation by the central GABAergic system. Neurosci. Biobehav. Rev. 20, 403–412. doi: 10.1016/0149-7634(95)00052-6
Gutierrez-Mazariegos, J., Nadendla, E. K., Lima, D., Pierzchalski, K., Jones, J. W., Kane, M., et al. (2014). A mollusk retinoic acid receptor (RAR) ortholog sheds light on the evolution of ligand binding. Endocrinology 155, 4275–4286. doi: 10.1210/en.2014-1181
Hardeland, R. (1999). Melatonin and 5-methoxytryptamine in non-metazoans. Reprod. Nutr. Dev. 39, 399–408. doi: 10.1051/rnd:19990311
Hardeland, R., Pandi-Perumal, S. R., and Cardinali, D. P. (2006). Melatonin. Int. J. Biochem. Cell B. 38, 313–316. doi: 10.1016/j.biocel.2005.08.020
Heine, G., Hollstein, T., Treptow, S., Radbruch, A., and Worm, M. (2018). 9-cis retinoic acid modulates the type I allergic immune response. J. Allergy Clin. Immun. 141, 650–658. doi: 10.1016/j.jaci.2017.03.046
Heyman, R. A., Mangelsdorf, D. J., Dyck, J. A., Stein, R. B., Eichele, G., Evans, R. M., et al. (1992). 9-cis retinoic acid is a high affinity ligand for the retinoid X receptor. Cell 68, 397–406. doi: 10.1016/0092-8674(92)90479-V
Hyde, L. L., and Underwood, H. (2000). Effects of melatonin administration on the circadian activity rhythm of the lizard Anolis carolinensis. Physiol. Behav. 71, 183–192. doi: 10.1016/S0031-9384(00)00340-1
Itoh, M. T., Hattori, A., Sumi, Y., and Suzuki, T. (1995). Day-night changes in melatonin levels in different organs of the cricket (Gryllus bimaculatus). J. Pineal Res. 18, 165–169. doi: 10.1111/j.1600-079X.1995.tb00156.x
Jacobs, B. L., and Fornal, C. A. (1997). Serotonin and motor activity. Curr. Opin. Neurobiol. 7, 820–825. doi: 10.1016/S0959-4388(97)80141-9
Kato, S., Tsurumaru, S., Taga, M., Yamane, T., Shibata, Y., Ohno, K., et al. (2009). Neuronal peptides induce oocyte maturation and gamete spawning of sea cucumber, Apostichopus japonicus. Dev. Biol. 326, 169–176. doi: 10.1016/j.ydbio.2008.11.003
Keller, H., Dreyer, C., Medin, J., Mahfoudi, A., Ozato, K., and Wahli, W. (1993). Fatty acids and retinoids control lipid metabolism through activation of peroxisome proliferator-activated receptor-retinoid X receptor heterodimers. Proc. Natl. Acad. Sci. U.S.A. 90, 2160–2164. doi: 10.1073/pnas.90.6.2160
Kim, J. Y., Park, J. Y., Kim, O. Y., Ham, B. M., Kim, H. J., and Kwon, D. Y. (2010). Metabolic profiling of plasma in overweight/obese and lean men using ultra performance liquid chromatography and Q-TOF mass spectrometry (UPLC/Q-TOF MS). J. Proteome Res. 9, 4368–4375. doi: 10.1021/pr100101p
Klein, D. C., and Weller, J. L. (1972). Rapid light-induced decrease in pineal serotonin N-acetyltransferase activity. Science 177, 532–533. doi: 10.1126/science.177.4048.532
Kolár, J., and Machácková, I. (2005). Melatonin in higher plants: occurrence and possible functions. J. Pineal Res. 39, 333–341. doi: 10.1111/j.1600-079X.2005.00276.x
Kulczykowska, E., Kalamarz, H., Warne, J. M., and Balment, R. J. (2006). Day–night specific binding of 2-[125I]Iodomelatonin and melatonin content in gill, small intestine and kidney of three fish species. J. Comp. Physiol. B 176, 277–285. doi: 10.1007/s00360-005-0049-4
Lerner, A. B., Case, J. D., Takahashi, Y., Lee, T. H., and Mori, W. (1958). Isolation of melatonin, the pineal gland factor that lightens melanocytes1. J. Am. Chem. Soc. 80, 2587–2587. doi: 10.1021/ja01543a060
Liao, Y. (1980). “The aspidochirote holothurians of China with erection of a new genus,” in Echinoderms: Present and Past. ed. M. Jangous (Rotterdam: AA Balkema), 115–120.
López-Olmeda, J. F., Madrid, J. A., and Sánchez-Vázquez, F. J. (2006). Melatonin effects on food intake and activity rhythms in two fish species with different activity patterns: diurnal (goldfish) and nocturnal (tench). Comp. Biochem. Phys. A 144, 180–187. doi: 10.1016/j.cbpa.2006.02.031
Mintz, E. M., Phillips, N. H., and Berger, R. J. (1998). Daytime melatonin infusions induce sleep in pigeons without altering subsequent amounts of nocturnal sleep. Neurosci. Lett. 258, 61–64. doi: 10.1016/S0304-3940(98)00849-0
Mommaerts, W. F. (1969). Energetics of muscular contraction. Physiol. Rev. 49, 427–508. doi: 10.1152/physrev.1969.49.3.427
Morikawa, K., Tsuneki, K., and Ito, K. (2001). Expression patterns of HNK-1 carbohydrate and serotonin in sea urchin, amphioxus, and lamprey, with reference to the possible evolutionary origin of the neural crest. Zoology 104, 81–90. doi: 10.1078/0944-2006-00011
Murakami, N., Kawano, T., Nakahara, K., Nasu, T., and Shiota, K. (2001). Effect of melatonin on circadian rhythm, locomotor activity and body temperature in the intact house sparrow, Japanese quail and owl. Brain Res. 889, 220–224. doi: 10.1016/S0006-8993(00)03205-4
Murch, S. J., Campbell, S. S. B., and Saxena, P. K. (2001). The role of serotonin and melatonin in plant morphogenesis: regulation of auxin-induced root organogenesis in in vitro-cultured explants of St. John’s wort (Hypericum perforatum L.). In Vitro Cell. Dev. Biol. Plant 37, 786–793. doi: 10.1007/s11627-001-0130-y
Nässel, D. R. (1988). Serotonin and serotonin-immunoreactive neurons in the nervous system of insects. Prog. Neurobiol. 30, 1–85. doi: 10.1016/0301-0082(88)90002-0
Nicholson, J. K., Lindon, J. C., and Holmes, E. (1999). ’Metabonomics’: understanding the metabolic responses of living systems to pathophysiological stimuli via multivariate statistical analysis of biological NMR spectroscopic data. Xenobiotica 29, 1181–1189. doi: 10.1080/004982599238047
O’Connor, D., and Mortishire-Smith, R. (2006). High-throughput bioanalysis with simultaneous acquisition of metabolic route data using ultra performance liquid chromatography coupled with time-of-flight mass spectrometry. Anal. Bioanal. Chem. 385, 114–121. doi: 10.1007/s00216-006-0353-1
Omond, S., Ly, L. M. T., Beaton, R., Storm, J. J., Hale, M. W., and Lesku, J. A. (2017). Inactivity is nycthemeral, endogenously generated, homeostatically regulated, and melatonin modulated in a free-living platyhelminth flatworm. Sleep 40:zsx124. doi: 10.1093/sleep/zsx124
Pagliarani, A., Trombetti, F., and Ventrella, V. (2012). Biochemical and Biological Effects of Organotins. Emirate of Sharjah: Bentham Science Publishers. doi: 10.2174/97816080526531120101
Pan, Y., Zhang, L., Lin, C., Sun, J., Kan, R., and Yang, H. (2015). Influence of flow velocity on motor behavior of sea cucumber Apostichopus japonicus. Physiol. Behav. 144, 52–59. doi: 10.1016/j.physbeh.2015.02.046
Parfitt, A., Weller, J. L., and Klein, D. C. (1976). Beta adrenergic-blockers decrease adrenergically stimulated N-acetyltransferase activity in pineal glands in organ culture. Neuropharmacology 15, 353–358. doi: 10.1016/0028-3908(76)90083-6
Pavlova, G. A. (2001). Effects of serotonin, dopamine and ergometrine on locomotion in the pulmonate mollusc Helix lucorum. J. Exp. Biol. 204, 1625–1633.
Peres, R., Amaral, F. G., Marques, A. C., and Neto, J. C. (2014). Melatonin production in the sea star Echinaster brasiliensis (Echinodermata). Biol. Bull. 226, 146–151. doi: 10.1086/BBLv226n2p146
Pinillos, M. L., Pedro, N. D., Alonso-Gómez, A. L., Alonso-Bedate, M., and Delgado, M. J. (2001). Food intake inhibition by melatonin in goldfish (Carassius auratus). Physiol. Behav. 72, 629–634. doi: 10.1016/S0031-9384(00)00399-1
Poeggeler, B. (1993). Introduction. Melatonin and the light-dark zeitgeber in vertebrates, invertebrates and unicellular organisms. Cell Mol. Life Sci. 49, 611–613. doi: 10.1007/BF01923940
Poeggeler, B., Balzer, I., Hardeland, R., and Lerchl, A. (1991). Pineal hormone melatonin oscillates also in the dinoflagellate Gonyaulax polyedra. Naturwissenschaften 78, 268–269. doi: 10.1007/BF01134354
Qiu, T., Zhang, L., Zhang, T., and Yang, H. (2014). Effects of mud substrate and water current on the behavioral characteristics and growth of the sea cucumber Apostichopus japonicus, in the Yuehu lagoon of northern China. Aquacult. Int. 22, 423–433. doi: 10.1007/s10499-013-9650-9
Reiter, R. J. (1995). Functional pleiotropy of the neurohormone melatonin: antioxidant protection and neuroendocrine regulation. Front. Neuroendocrin. 16:383–415. doi: 10.1006/frne.1995.1014
Renaud, F., Parisi, E., Capasso, A., and Prisco, P. D. (1983). On the role of serotonin and 5-methoxy-tryptamine in the regulation of cell division in sea urchin eggs. Dev. Biol. 98, 37–46. doi: 10.1016/0012-1606(83)90333-0
Ru, X., Zhang, L., Liu, S., and Yang, H. (2017). Reproduction affects locomotor behaviour and muscle physiology in the sea cucumber, Apostichopus japonicus. Anim. Behav. 133, 223–228. doi: 10.1016/j.anbehav.2017.09.024
Sakai, M., and Takahashi, H. (1996). One-electron photoreduction of flavin mononucleotide: time-resolved resonance Raman and absorption study. J. Mol. Struct. 379, 9–18. doi: 10.1016/0022-2860(95)09176-9
Saraste, M. (1999). Oxidative phosphorylation at the fin de siècle. Science 283, 1488–1493. doi: 10.1126/science.283.5407.1488
Sawin, E. R., Ranganathan, R., and Horvitz, H. R. (2000). C. elegans locomotory rate is modulated by the environment through a dopaminergic pathway and by experience through a serotonergic pathway. Neuron 26, 619–631. doi: 10.1016/S0896-6273(00)81199-X
Shmukler, I. B., and Tosti, E. (2001). Serotoninergic processes in cells of early embryos of the sea urchin Paracentrotus lividus. Rossiiskii fiziologicheskii zhurnal imeni IM Sechenova 87, 1557–1564.
Smeitink, J., Van Den Heuvel, L., and DiMauro, S. (2001). The genetics and pathology of oxidative phosphorylation. Nat. Rev. Genet. 2, 342–352. doi: 10.1038/35072063
Sprenger, J., Hardeland, R., Fuhrberg, B., and Han, S. Z. (1999). Melatonin and other 5-methoxylated indoles in yeast: presence in high concentrations and dependence on tryptophan availability. Cytologia 64, 209–213. doi: 10.1508/cytologia.64.209
Squires, L. N., Rubakhin, S. S., Wadhams, A. A., Talbot, K. N., Nakano, H., Moroz, L. L., et al. (2010). Serotonin and its metabolism in basal deuterostomes: insights from Strongylocentrotus purpuratus and Xenoturbella bocki. J. Exp. Biol. 213, 2647–2654. doi: 10.1242/jeb.042374
Sun, J., Zhang, L., Pan, Y., Lin, C., Wang, F., Kan, R., et al. (2015). Feeding behavior and digestive physiology in sea cucumber Apostichopus japonicus. Physiol. Behav. 139, 336–343. doi: 10.1016/j.physbeh.2014.11.051
Sun, J., Zhang, L., Pan, Y., Lin, C., Wang, F., and Yang, H. (2018). Effect of water temperature on diel feeding, locomotion behaviour and digestive physiology in the sea cucumber Apostichopus japonicus. J. Exp. Biol. 221:jeb177451. doi: 10.1242/jeb.177451
Sun, L., Sun, J., Xu, Q., Li, X., Zhang, L., and Yang, H. (2017). Metabolic responses to intestine regeneration in sea cucumbers Apostichopus japonicus. Comp. Biochem. Phys. D 22, 32–38. doi: 10.1016/j.cbd.2017.02.003
Tanaka, D., Furusawa, K., Kameyama, K., Okamoto, H., and Doi, M. (2007). Melatonin signaling regulates locomotion behavior and homeostatic states through distinct receptor pathways in Caenorhabditis elegans. Neuropharmacology 53, 157–168. doi: 10.1016/j.neuropharm.2007.04.017
Tilden, A. R., Brauch, R., Ball, R., Janze, A. M., Ghaffari, A. H., Sweeney, C. T., et al. (2003a). Modulatory effects of melatonin on behavior, hemolymph metabolites, and neurotransmitter release in crayfish. Brain Res. 992, 252–262.
Tilden, A. R., Shanahan, J. K., Khilji, Z. S., Owen, J. G., Sterio, T. W., and Thurston, K. T. (2003b). Melatonin and locomotor activity in the fiddler crab Uca pugilator. J. Exp. Zool. Part A 297, 80–87.
Tolstenkov, O. O., Prokofiev, V. V., Pleskacheva, M. V., Gustafsson, M. K. S., and Zhukovskaya, M. I. (2017). Age and serotonin effects on locomotion in marine trematode cercariae. J. Evol. Biochem. Physiol. 53, 135–142. doi: 10.1134/S1234567817020069
Tugwood, J. D., Issemann, I., Anderson, R. G., Bundell, K. R., McPheat, W. L., and Green, S. (1992). The mouse peroxisome proliferator activated receptor recognizes a response element in the 5’ flanking sequence of the rat acyl CoA oxidase gene. EMBO J. 11, 433–439. doi: 10.1002/j.1460-2075.1992.tb05072.x
Uchida, N., and Cohen, J. Y. (2017). Serotonin: slow motion. eLife 6:e24792. doi: 10.7554/eLife.24792
Viera-Vera, J., and García-Arrarás, J. E. (2018). Molecular characterization and gene expression patterns of retinoid receptors, in normal and regenerating tissues of the sea cucumber, Holothuria glaberrima. Gene 654, 23–35. doi: 10.1016/j.gene.2018.01.102
Vivien-Roels, B., and Pévet, P. (1993). Melatonin: presence and formation in invertebrates. Experientia 49, 642–647. doi: 10.1007/BF01923945
Wang, X., Li, D., Jiang, M., Pei, Z., and Xu, L. (2018). K nearest neighbor algorithm coupled with metabonomics to study the therapeutic mechanism of sendeng-4 in adjuvant-induced rheumatoid arthritis rat. Evid. Based Complement. Alternat. Med. 2018:2484912. doi: 10.1155/2018/2484912
Wang, X., Sun, W., Sun, H., Lv, H., Wu, Z., Wang, P., et al. (2008). Analysis of the constituents in the rat plasma after oral administration of Yin Chen Hao Tang by UPLC/Q-TOF-MS/MS. J. Pharm. Biomed. Anal. 46, 477–490. doi: 10.1016/j.jpba.2007.11.014
Welsh, J. H., and Moorhead, M. (1960). The quantitative distribution of 5-hydroxy-tryptamine in the invertebrates, especially in their nervous systems. J. Neurochem. 6, 146–169. doi: 10.1111/j.1471-4159.1960.tb13460.x
Wilson, I. D., Nicholson, J. K., Castro-Perez, J., Granger, J. H., Johnson, K. A., Smith, B. W., et al. (2005). High resolution “ultra performance” liquid chromatography coupled to oa-TOF mass spectrometry as a tool for differential metabolic pathway profiling in functional genomic studies. J. Proteome Res. 4, 591–598. doi: 10.1021/pr049769r
Yamakawa, S., Morino, Y., Honda, M., and Wada, H. (2018). The role of retinoic acid signaling in starfish metamorphosis. EvoDevo 9:10. doi: 10.1186/s13227-018-0098-x
Yamano, H., Watari, Y., Arai, T., and Takeda, M. (2001). Melatonin in drinking water influences a circadian rhythm of locomotor activity in the house cricket, Acheta domesticus. J. Insect. Physiol. 47, 943–949. doi: 10.1016/S0022-1910(01)00067-1
Yan, L., Fu, L., Li, M., Bai, X., and Jin, L. (2018). Fabrication of dual-stimuli responsive films assembled by flavin mononucleotide and layered double hydroxides. Chem. Commun. 54, 12590–12593. doi: 10.1039/c8cc06459h
Yang, H., Hamel, J. F., and Mercier, A. (2015). The Sea Cucumber Apostichopus Japonicus: History, Biology and Aquaculture, Vol. 39. Cambridge, MA: Academic Press.
Yin, P., Zhao, X., Li, Q., Wang, J., Li, J., and Xu, G. (2006). Metabonomics study of intestinal fistulas based on ultraperformance liquid chromatography coupled with Q-TOF mass spectrometry (UPLC/Q-TOF MS). J. Proteome Res. 5, 2135–2143. doi: 10.1021/pr060256p
Yu, S. J., Airavaara, M., Wu, K. J., Harvey, B. K., Liu, H. S., Yang, Y., et al. (2017). 9-cis retinoic acid induces neurorepair in stroke brain. Sci. Rep. 7:4512. doi: 10.1038/s41598-017-04048-2
Zhdanova, I. V. (2005). Melatonin as a hypnotic: pro. Sleep Med. Rev. 9, 51–65. doi: 10.1016/j.smrv.2004.04.003
Zhdanova, I. V., Wang, S. Y., Leclair, O. U., and Danilova, N. P. (2001). Melatonin promotes sleep-like state in zebrafish. Brain Res. 903, 263–268. doi: 10.1016/S0006-8993(01)02444-1
Zhdanova, I. V., Yu, L., Lopez-Patino, M., Shang, E., Kishi, S., and Guelin, E. (2008). Aging of the circadian system in zebrafish and the effects of melatonin on sleep and cognitive performance. Brain Res. Bull. 75, 433–441. doi: 10.1016/j.brainresbull.2007.10.053
Zheng, X., Shoffner, J. M., Voljavec, A. S., and Wallace, D. C. (1990). Evaluation of procedures for assaying oxidative phosphorylation enzyme activities in mitochondrial myopathy muscle biopsies. Biochim. Biophys. Acta 1019, 1–10. doi: 10.1016/0005-2728(90)90118-N
Zhou, H., Song, J., Chang, Y., Cheng, L., and Ning, J. (2014). A comparative study of the anesthetic effect of different anesthetic on juvenile sea cucumber. Chin. Agric. Sci. Bull. 30, 95–99.
Ziouzenkova, O., Perrey, S., Marx, N., Bacqueville, D., and Plutzky, J. (2002). Peroxisome proliferator-activated receptors. Curr. Atheroscler. Rep. 4, 59–64. doi: 10.1007/s11883-002-0063-x
Keywords: melatonin, sea cucumber, locomotor behavior, physiological mechanism, fatty acid oxidation, oxidative phosphorylation
Citation: Ding K, Zhang L, Zhang T, Yang H and Brinkman R (2019) The Effect of Melatonin on Locomotor Behavior and Muscle Physiology in the Sea Cucumber Apostichopus japonicus. Front. Physiol. 10:221. doi: 10.3389/fphys.2019.00221
Received: 27 September 2018; Accepted: 21 February 2019;
Published: 19 March 2019.
Edited by:
Elzbieta M. Pyza, Jagiellonian University, PolandReviewed by:
Gustavo Bueno Rivas, Texas A&M University, United StatesLuiz Eduardo Maia Nery, Fundação Universidade Federal do Rio Grande, Brazil
John Lesku, La Trobe University, Australia
Copyright © 2019 Ding, Zhang, Zhang, Yang and Brinkman. This is an open-access article distributed under the terms of the Creative Commons Attribution License (CC BY). The use, distribution or reproduction in other forums is permitted, provided the original author(s) and the copyright owner(s) are credited and that the original publication in this journal is cited, in accordance with accepted academic practice. No use, distribution or reproduction is permitted which does not comply with these terms.
*Correspondence: Libin Zhang, zhanglibin@qdio.ac.cn