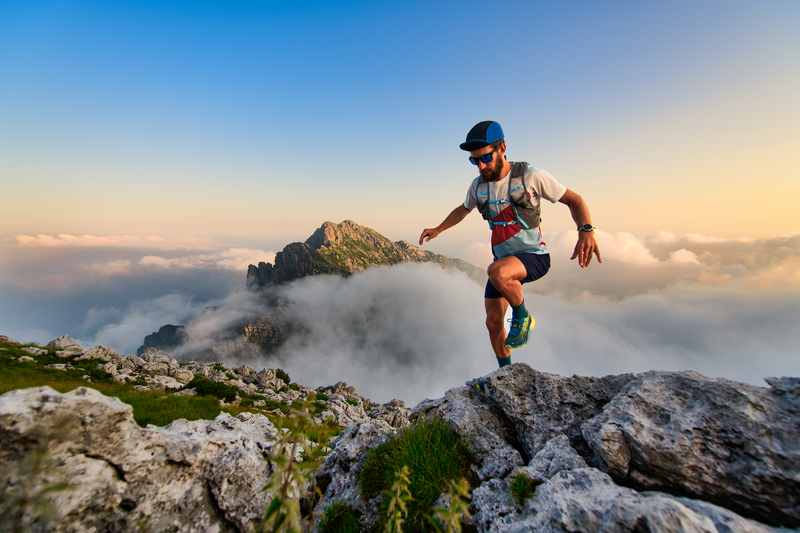
95% of researchers rate our articles as excellent or good
Learn more about the work of our research integrity team to safeguard the quality of each article we publish.
Find out more
ORIGINAL RESEARCH article
Front. Physiol. , 25 February 2019
Sec. Respiratory Physiology and Pathophysiology
Volume 10 - 2019 | https://doi.org/10.3389/fphys.2019.00160
Background: We aimed to investigate the effect of a priming ischemia-reperfusion (IR) model on the kinetics of pulmonary oxygen uptake (VO2) and cardiopulmonary parameters after high-intensity exercise. Our primary outcome was the overall VO2 kinetics and secondary outcomes were heart rate (HR) and O2 pulse kinetics. We hypothesized that the IR model would accelerate VO2 and cardiopulmonary kinetics during the exercise.
Methods: 10 recreationally active men (25.7 ± 4.7 years; 79.3 ± 10.8 kg; 177 ± 5 cm; 44.5 ± 6.2 mL kg−1 min−1) performed a maximal incremental ramp test and four constant load sessions at the midpoint between ventilatory threshold and VO2 max on separate days: two without IR (CON) and two with IR (IR). The IR model consisted of a thigh bi-lateral occlusion for 15 min at a pressure of 250 mmHg, followed by 3 min off, before high-intensity exercise bouts.
Results: There were no significant differences for any VO2 kinetics parameters (VO2 base 1.08 ± 0.08 vs. 1.12 ± 0.06 L min−1; P = 0.30; τ = 50.1 ± 7.0 vs. 47.9 ± 6.4 s; P = 0.47), as well as for HR (MRT180s 67.3 ± 6.0 vs. 71.3 ± 6.1 s; P = 0.54) and O2 pulse kinetics (MRT180s 40.9 ± 3.9 vs. 48.2 ± 5.6 s; P = 0.31) between IR and CON conditions, respectively.
Conclusion: We concluded that the priming IR model used in this study had no influence on VO2, HR, and O2 pulse kinetics during high-intensity cycling exercise.
Pulmonary oxygen uptake (VO2) kinetics plays a role in the oxidative metabolism during constant load exercise (Poole et al., 2008). Considering that the area above the curve is accepted as a representative surrogate of the required additional energy supplying – i.e., anaerobic energy sources (Margaria et al., 1963), any acute or chronic adjustments that could reduce the time to attain a steady state of oxygen uptake and improves the energy imbalance becomes relevant for aerobic exercise performance (Gastin, 2001) or health related outcomes (Poole and Jones, 2012).
Several methods have been used to acutely challenge the VO2 control system and to enhance VO2 kinetics, such as breathing hyperoxic gas (MacDonald et al., 1998, 2000); prior exercise (Gerbino et al., 1996; Burnley et al., 2001, 2002) a reactive hyperemia induced by ischemia-reperfusion (IR) (Walsh et al., 2002; Faisal et al., 2010) – Which have resulted in controversial findings. The IR model involves blocking arterial to the target site (usually through a cuff pressure device), for a given period (continuously or intermittently). Upon cuff release, blood flow is augmented well above resting values (Kooijman et al., 2008). The brief exposure of muscle tissue to a blood flow absence causes an immediate reduction of the local O2 saturation, followed by a reduced adenosine triphosphate (ATP) and phosphocreatine (PCr) intramuscular concentrations, and an increase in adenosine diphosphate (ADP) and inorganic phosphate (Pi) intramuscular concentrations (Boushel et al., 1998). On the very end, an increase in vascular dilation (Enko et al., 2011), a potential increase in local blood flow, O2 muscle saturation and cardiac function (Daly and Bondurant, 1962; Faisal et al., 2010) is expected, which may accelerate VO2 kinetics by improving O2 delivery and availability. Nonetheless, the blood flow restriction (e.g., increases in ADP, Pi, etc.,) may affect the VO2 time course through spin-off manifestations, likely improvements in the mitochondrial oxidation activity through the increases in ADP and Pi concentrations (Boushel et al., 1998; Walsh et al., 2002). Despite the physiological rationale, the IR model has received little attention to study VO2 kinetics at the onset of exercise.
Few studies were conducted with contradictory and inconclusive findings, like an impairment (Faisal et al., 2010), no effect (Kido et al., 2015) or an improvement (Walsh et al., 2002) in the pulmonary VO2 kinetics. At high intensity exercise, VO2 kinetics appears to be sensitive to the fundamental component amplitude (Ap) (Walsh et al., 2002; Wilkerson et al., 2006), slow component amplitude (As) (MacDonald et al., 1998; Burnley et al., 2002; Walsh et al., 2002), time-constant of fundamental component (τp) (MacDonald et al., 1998) and the “overall” kinetics (Walsh et al., 2002; Tordi et al., 2003; Nascimento et al., 2015). Also, VO2 kinetics is dependent on systemic and peripheral mechanisms, such as increased activity of oxidative enzymes, muscle blood flow and improved O2 distribution in the exercise muscles (Burnley et al., 2002; Wilkerson et al., 2006; Faisal et al., 2010; Poole and Jones, 2012). Notwithstanding, there is a question that still provokes further investigation for the physiologists: is the VO2 kinetics at the onset of exercise limited by muscle O2 delivery or by an oxidative enzyme inertia? In that scenario the reactive hyperemia by occluding target members, then augmenting muscle blood flow for several minutes prior to a subsequent exercise bout (Faisal et al., 2010), with less pronounced changes in the metabolic environment (i.e., peripheral mechanisms) seems an innovative approach.
Finally, it has been suggested that O2 delivery does not limit the phase II of VO2 kinetics in healthy subjects during a high intensity exercise (Poole et al., 2008), at least in some instances. However, at some “tipping point,” an O2 limitation could occur and VO2 kinetics can be modified through alterations in O2 availability. Specifically, high-intensity approaches the tipping point, where VO2 kinetics is O2-delivery dependent and may potentially be more susceptible to the influence of blood-muscle O2 influx (Poole and Jones, 2012). Thus, this study aims to investigate the effect of a priming IR protocol prior to high-intensity exercise on pulmonary VO2 kinetics and cardiac parameters (heart rate and O2 pulse). We hypothesized that a prior IR stimulus would accelerate VO2 and cardiac related kinetics at the onset of high-intensity cycling.
Ten recreationally active men (age 25 ± 5 years; body mass 79.3 ± 11 kg; height 177 ± 5 cm; VO2 max 44.5 ± 6.2 mL kg−1 min−1) volunteered to participate in this study. Participants were classified as physically active by the American College of Sports Medicine (ACSM) criteria (cardiorespiratory exercise training for ≥30 min⋅days−1 on ≥5 days⋅week−1 for a total of ≥150 min⋅week−1) (Garber et al., 2011). Some of them were involved in further recreational sport activities but not in any systematic physical exercise program. Participants were informed about risks and possible discomfort related to the protocol study. After that, a written informed consent was given before starting the study.
The subjects were instructed to avoid any intake of caffeine for 3 h, or alcohol and strenuous exercise in the 24 h preceding the test sessions. They were also instructed to arrive at the laboratory in a rested and fully hydrated state, and to eat the last meal up to 2 h prior to the experiment. All tests were conducted at the same time of day in controlled environmental laboratory conditions (19–22°C; 50–60% RH), to minimize the effects of diurnal biological variation on the results. All procedures were performed in accordance to the Declaration of Helsinki statement and were reviewed and approved by the Institutional Research Board (Ethics Committee for Research in Human Beings, Universidade Federal de Santa Catarina, IRB number 51045315.7.0000.0121).
The subjects visited the laboratory on five occasions. In the first visit, participants underwent a maximal incremental cycling test to determine intensity parameters for the experimental protocol (Visit 1); the other four experimental visits were randomly assigned, and separated by at least 48 h.
The experimental trials consisted of 3 min of baseline cycling at 20 W plus 8 min at Δ50% intensity. Gas exchange was collected throughout exercise bouts to analyze VO2, and O2 pulse kinetics. Heart rate (HR) was measured during the entire exercise bouts as well. In two of the four trials, cycling exercise was preceded by 15 min bilateral thigh blood flow occlusion, whereas two trials without IR were conducted as control sessions (Figure 1).
Figure 1. Illustrative figure of ischemia-reperfusion (IR) experimental design. For CON conditions, the experiment initiated with the 3-min 20 W cycling.
To determine physiological indexes for exercise sessions, we conducted the maximal incremental ramp test on a cycle ergometer (Lode Excalibur, Groningen, Netherlands). Participants exercised for 4 min at a constant load of 20 W to stabilize gas measurements and then the exercise intensity increased systematically by 1 W every 2 s (rate of 30 W min−1) until volitional exhaustion.
Oxygen uptake was measured breath-by-breath using a gas analyzer (Quark PFT Ergo, COSMED, Rome, Italy). The gas analyzer was calibrated immediately before each test using ambient air and certificated alpha standard gasses containing 16.0% of oxygen and 5.0% of carbon dioxide. The turbine flowmeter was calibrated with a syringe of three liters (Quark PFT Ergo, COSMED, Rome, Italy). HR was continuously recorded by a HR monitor integrated into the gas analyzer system. Earlobe capillary blood lactate samples (25 μL) were taken before and immediately after the incremental exercise from the ear lobe and analyzed by an electrochemical method (YSI 2700 STAT, Yellow Springs, OH, United States).
Maximal oxygen uptake was defined by two criteria, depending on the presence of a VO2 plateau. The VO2 plateau was defined as any 60 s period in which the VO2 stabilized without increases higher than 150 mL min−1. In the absence of a VO2 plateau, we considered the maximum oxygen uptake as VO2 max, by the maximal observed value in a 15 s interval (Day et al., 2003). Maximal power output (Wpeak), maximal heart rate (HRmax) and maximal lactate were considered as the highest values observed during exercise. Gas exchange threshold (GET) was calculated using the V-slope method (Beaver et al., 1986). The increase in VO2 ventilatory equivalent (VE/VO2) with no further increases in VCO2 ventilatory equivalent (VE/VCO2) was verified through visual inspection and used as a secondary criterion.
The Δ50% exercise intensity was calculated by defining GET-VO2 and VO2 max, and then adding 50% of the difference between them to GET-VO2. After this, we made an interpolation using the linear regression model with the equation of a VO2/intensity relationship (Souza et al., 2016), in order to determine the Δ50% workload.
Each testing session consisted of a square-wave transition of 3 min at 20 W followed by 8 min at Δ50% intensity. Earlobe capillary blood lactate samples were collected 30 s before the baseline ([Lac]rest), 30 s before exercise ([Lac]pre) and immediately after the exercise ([Lac]post). O2 pulse was determined as the fraction between VO2 and HR (Whipp et al., 1996), both interpolated by 1 s intervals after matching the data of the two identical sessions corresponding to IR and CON conditions.
Oxygen uptake and HR were recorded breath-by-breath during the entire exercise sessions (Quark PFT Ergo, COSMED, Rome, Italy) and were exported as raw data for filtering and analysis (OriginPro 7.0, United States). For VO2, signals suggesting sighs and coughs were initially removed from each test. According to Lamarra et al. (1987), occasional breath values were excluded from analysis using three standard deviations from the local mean as the criterion. Breath-by-breath data were linearly interpolated by 1 s intervals and then time-aligned to the start of exercise. After this procedure, both data series of each condition were matched and averaged in order to provide a unique profile for each exercise condition. The last filtering procedure was applied to reduce interpolated data to a 5 s stationary mean (Rossiter et al., 2005). The cardiopulmonary phase (i.e., first 20 s of exercise) was not included in the analysis (Rossiter et al., 2005).
For modeling the VO2 profile, a non-linear regression technique was applied (Rossiter et al., 2002), where phase II and a slow component were fitted separately. The phase II was modeled from the end of the cardio-dynamic phase to the beginning of the slow component phase. The slow component phase was determined by four criterias: (1) the narrowest confidence interval for τ; (2) breakpoint and the systematic rising of phase II amplitude and time-constant with a decrease in TD; (3) breakpoint and a systematic rising of fitting R2; (4) visual inspection of residual plot, considering the end-phase by the farthest point from zero. The model was constrained in the VO2 baseline (Barstow et al., 1996) to identify key parameters according to the Eq. 1:
Equation 1. Mono-exponential model for VO2 kinetics.
where VO2 (t) represents VO2 values for a given time t; VO2 base is the average VO2 of the last minute of 20 W load period; Ap is the amplitude of phase II; τp is the phase II time-constant; and TD is the phase II time delay.
The slow component amplitude was determined by the difference between the VO2 end and the sum of the VO2 baseline and Ap, according to Eq. 2:
Equation 2. VO2 slow component calculation.
Where, As is the slow component amplitude; VO2 end is the average VO2 value over the last 15 s; and Ap is the phase II amplitude.
The mean response time (MRT), which describes an overall pattern of VO2 kinetics for the exercise bout, was calculated by a mono-exponential model from the beginning to the end of the exercise, excluding the time delay (Barstow and Molé, 1991), according to Eq. 3. The same equation was used to fit HR and O2 pulse kinetics at the onset of the exercise (Wilkerson et al., 2006), with the end-point fixed at 180 s in order to augment fitting quality (i.e., better r2), since the bi-exponential pattern of HR and O2 pulse were not detected for all of the subjects:
Equation 3. Mean-response time (overall kinetics) for VO2, HR and O2 pulse.
For IR, a 15 min bilateral thigh occlusion was introduced by the application of 250 mmHg pressure simultaneously in each thigh of the participants while they rested in a supine position (Ger-Ar, São Paulo, Brazil). Occlusion cuffs, chosen to be 20% wider than the upper leg diameter, were placed at the inguinal leg site. After 15 min of inflated cuffs, they were released, and the exercise load started following 3 min of 20 W cycling. Capillary blood lactate was taken from the ear lobe at rest and every 5 min until the end of the occlusion period ([Lac]rest, [Lac]5, [Lac]10, and [Lac]15). The occlusion time, mode and the time between cuff releasing and the beginning of exercise were determined based on a time-window where the blood flow remained augmented against the baseline conditions (Hampson and Piantadosi, 1988; Paganelli et al., 1989; Faisal et al., 2010). The period of time between the subject’s displacement from the bed to the cycle ergometer, as well as the data acquisition procedures (cuff displacement, face mask, HR strap adjustment, and sitting) were also controlled, in order to ensure trial reliability.
Descriptive data is presented as mean ± standard deviation. Firstly, we tested the normality of the distribution by the Shapiro-Wilk test (n < 50). For variance analysis, sphericity assumptions were tested by the Mauchly’s test, and corrections were made by the Greenhouse-Geisser factor. For VO2, HR, and O2 pulse kinetics parameter comparison, the two-tailed Student t-test for paired samples was used. Log transformations were made when necessary. For lactate concentration analysis between-conditions, the two-way ANOVA for repeated measures (condition vs. time) was conducted. For comparisons of lactate concentrations during thigh occlusion (in supine position), we used a one-way ANOVA for repeated measures. The Bonferroni’s post hoc analysis was used to estimate point-by-point differences after ANOVA. The sample size was calculated for a moderate effect size (ES = 0.5), with an α level of 0.05 and a statistical power of 80%, resulting in 10 subjects. VO2, HR and O2 pulse data were loaded into the Origin 8.0 software and the statistical analysis was completed using the SPSS 22.0 for Windows® and the GraphPad Prism for Mac® (Prism 7.0, United States). An α level of 0.05 was set to claim for statistical significance.
Table 1 shows the physiological indexes derived from the maximal incremental test.
Table 2 shows VO2 kinetics parameters obtained during the 8 min square wave exercise with or without previous limb IR protocol. In Figure 2, the overall exercise VO2 pattern is illustrated by averaging all the subject’s 5 s data points. VO2 measured at the end of square-wave trials were 3.38 ± 0.11 L min−1 and 3.41 ± 0.09 L min−1 (P = 0.39) for CON and IR conditions, respectively, overestimating predicted VO2 about 22.9% ± 0.3% and 24.1 ± 0.2%. There were no differences between the conditions for any VO2 kinetics parameters (VO2 base, Ap, As, VO2 end, τp, TDp e MRT). In addition, there were no interactional effects between the condition and time for the capillary blood lactate concentrations (P = 0.16), as well as no effect of conditions (CON vs. IR) (P = 0.20) for [Lac]rest (0.86 ± 0.32 and 1.16 ± 0.38 mmol L−1), [Lac]pre (1.04 ± 0.35 and 1.16 ± 0.44 mmol L−1) and [Lac]final (10.26 ± 1.62 and 9.43 ± 1.46 mmol L−1); and for [Lac] during bilateral thigh blood flow occlusion (P = 0.64; Figure 3).
Figure 2. VO2 during high-intensity exercise in IR and CON conditions. Points are averaged in 5 s means along the exercise period (8 min). Each point is representative of the mean ± SD of all 10 studied subjects. Mean VO2 baseline for CON: 1.08 ± 0.08; mean VO2 baseline for IR: 1.12 ± 0.06.
Figure 3. Capillary blood lactate concentrations during thigh blood flow restriction and after release. [Lac]base refers to the [Lac] immediately before the Δ50% exercise with individual observations. Data are expressed as mean ± SD.
Table 3 shows HR and O2 pulse kinetics results. MRT and the amplitude values are related to the time-fixed first 180 s of the exercise. There were no differences for any analyzed variables.
The main aim of this study was to investigate the impact of a prior bilateral thigh IR model on pulmonary VO2 kinetics during a cycling exercise at Δ50% intensity. The secondary aim was to investigate central control of the O2 pathway via the kinetic responses or HR and estimations of stroke volume (via O2 pulse) at the onset of exercise. Our major finding was that the proposed IR protocol did not modify VO2 kinetics during high-intensity exercise, neither HR and O2 pulse, rejecting our hypothesis that a previous blood flow occlusion protocol would accelerate pulmonary VO2 kinetics in a high intensity exercise. Our data also suggest that the IR model did not accelerate any central variables kinetics at the onset of the exercise. To the best of our knowledge, this is the first study to report the effects of IR on HR and O2 pulse kinetics during a high intensity cycling exercise.
In light of the previous studies in the literature related to IR model, the absence of effects is in accordance to the study of Kido et al. (2015), that did not find any acceleration of pulmonary VO2 kinetics during a severe intensity exercise when preceded by a similar protocol (ischemic preconditioning – 3 × 5 min bilateral occlusion, with 300 mmHg of pressure, ending within 5 min before exercise), especially at the onset of the exercise (τp) and also for “overall” kinetics (MRT). Faisal et al. (2010) found a diminished muscle VO2 when the exercise began following 3 min of recovery after 15 min of forearm ischemia during an upper arm heavy exercise model, although an enhancement of forearm blood flow was observed. On the other hand, the findings of Walsh et al. (2002) suggests that “overall” pulmonary VO2 kinetics could be accelerated by previous thigh IR lasting 5 to 10 min using about 250 mmHg of pressure. However, the major difference between the Walsh et al. (2002) study to ours/others was the timing between the end of a blood flow occlusion and the beginning of exercise.
Whilst it has been suggested that the local muscular hyperemia could be accessed by up to 2–3 min after the cuff release (Mullen et al., 2001; Faisal et al., 2010), we consider this potential as a feature to explain possible differences. In our study, we chose a 3 min time frame until the beginning of the exercise based on a study of Faisal et al. (2010). In addition, the baseline exercise before the Δ50% exercise, which is recommended and often used for pulmonary VO2 kinetics experimental assessments (Rossiter et al., 2002; Poole and Jones, 2012), may have influenced muscle blood flow levels before Δ50% exercise as well.
A classic study of Daly and Bondurant (1962) provided accurate measurements of HR, cardiac output and stroke volume after 15 min of a 250 mmHg forearm blood flow occlusion, suggesting a sudden rise of those variables in the first 15 s of cuff releasing. Thus, the central parameter results, such as HR and stroke volume (O2 pulse), need to be considered when evaluating VO2 kinetics. Also, it is noteworthy to highlight that we did not find any effects of IR model on [Lac] during the occlusion period, neither any differences in [Lac] rest values and [Lac]pre. Blood lactate has been suggested as a properly mediator of O2 transport enhancement by vascular tissue dilation (Krustrup et al., 2001; Tordi et al., 2003), which could partially explain the VO2 kinetics acceleration after priming exercise conditions (Wilkerson et al., 2004; Nascimento et al., 2016). We also observed a concomitant absence of change in [Lac] and central hemodynamic parameters following the IT intervention. This may have occurred due to a higher than expected O2 delivery and availability during thigh occlusion, as well as a better mitochondrial oxidation caused by higher levels of ADP, Pi, and Cr, which would attenuate any changes in [Lac].
We considered that our findings need to be interpreted in light of its limitations. Here we point out some future directions for research that investigators could further consider.
When defining our protocol, we considered three major factors: (1) the pressure cuff level; (2) the time period for occlusion; (3) and the time between the end of occlusion and the beginning of the exercise. In regard to the first, a 250 mmHg level was chosen because of its efficiency to completely occlude the femoral artery blood flow (Sharma et al., 2014) and because it has been successfully by others (Salvador et al., 2015). For the same reason, the occlusion time was chosen – i.e., 15 min of bilateral thigh IR, as it had been shown to trigger reactive hyperemia (Kooijman et al., 2008) and necessary metabolic disturbances to maximize (Sharma et al., 2014). Finally, the time between the end of the IR model and the beginning of the exercise was selected for two main reasons: firstly, a physiological rationale to augment the local blood flow, supported by previous experimental results (Faisal et al., 2010); and secondly a logistic reason that is related to the necessary time to strictly adhere to the entire necessary methodological procedures for the VO2 kinetics assessments in cycle ergometry, such as the participants transposition from the supine to the upright position, moving to the ergometer, cuff deflation and equipment removal.
Our results reinforce the absence of the central limitation in the onset-exercise pulmonary VO2 kinetics, and, strengthen the evidence on this side of the discussion. Therefore, we recommend that further research limited to this aspect is unlikely to change the direction of the evidence by its robustness. However, investigators still interested in using IR to study the onset-exercise pulmonary or muscular VO2 kinetics, and should consider a reduction in the time to the beginning of the exercise and to control the confounding factors necessary to reduce muscle inertia and rest muscle tension (Hughson and Morrissey, 1983) such as the 3 min of a baseline exercise.
We found that VO2, HR, and O2 pulse kinetics during a high-intensity cycling exercise was not accelerated when preceded by a 15 min bilateral thigh blood flow occlusion and 3 min off. These results have important implications in possible strategies to accelerate VO2 kinetics and improve metabolic efficiency. Considering there were no effects of IR when preceded by the off-period between the blood flow release and the exercise starting, further research should use other methods to investigate whether VO2 kinetics are limited by oxygen delivery or oxidative enzyme inertia.
IRB: Comite de Ética em Pesquisa com Seres Humanos – Universidade Federal de Santa Catarina Procedures: we first submitted to IRB’s appreciation our project with rationale, methods, risks, and benefits. Together, the Consent Form was approved and, before any enrollment with this study, the subjects needed to assign it. In the consent form, information regarding risk, benefits, study’s rationale and relevance and their wrights to drop out of the study whenever they want was made available to them. All signed forms are still stored in the project’s folder.
All authors designed the study and interpreted the data. LH and PS collected and analyzed the data. LH wrote the first draft. PS, RdL, and LG wrote the final draft.
LH and PS received Ph.D. funding support by the CAPES Foundation. LG is granted by the CNPq Council.
The authors declare that the research was conducted in the absence of any commercial or financial relationships that could be construed as a potential conflict of interest.
We want to thank the Exercise Physiology Laboratory staff (LAEF/UFSC) for supporting data collection, Dr. Eurico Wilhelm for supporting the reporting development.
Barstow, T. J., Jones, A. M., Nguyen, P. H., and Casaburi, R. (1996). Influence of muscle fiber type and pedal frequency on oxygen uptake kinetics of heavy exercise. J. Appl. Physiol. 81, 1642–1650. doi: 10.1152/jappl.1996.81.4.1642
Barstow, T. J., and Molé, P. A. (1991). Linear and nonlinear characteristics of oxygen uptake kinetics during heavy exercise. J. Appl. Physiol. 71, 2099–2106. doi: 10.1152/jappl.1991.71.6.2099
Beaver, W. L., Wasserman, K., and Whipp, B. J. (1986). A new method for detecting anaerobic threshold by gas exchange. J. Appl. Physiol. 60, 2020–2027. doi: 10.1152/jappl.1986.60.6.2020
Boushel, R., Pott, F., Madsen, P., Radegran, G., Nowak, M., Quistorff, B., et al. (1998). Muscle metabolism from near infrared spectroscopy during rhythmic handgrip in humans. Eur. J. Appl. Physiol. Occup. Physiol. 79, 41–48. doi: 10.1007/s004210050471
Burnley, M., Doust, J. H., Carter, H., and Jones, A. M. (2001). Effects of prior exercise and recovery duration on oxygen uptake kinetics during heavy exercise in humans. Exp. Physiol. 86, 417–425. doi: 10.1113/eph8602122
Burnley, M., Doust, J. H., and Jones, A. M. (2002). Effects of prior heavy exercise, prior sprint exercise and passive warming on oxygen uptake kinetics during heavy exercise in humans. Eur. J. Appl. Physiol. 87, 424–432. doi: 10.1007/s00421-002-0647-8
Daly, W. J., and Bondurant, S. (1962). Effects of oxygen breathing on the heart rate, blood pressure, and cardiac index of normal men–resting, with reactive hyperemia, and after atropine. J. Clin. Invest. 41, 126–132. doi: 10.1172/JCI104454
Day, J. R., Rossiter, H. B., Coats, E. M., Skasick, A., and Whipp, B. J. (2003). The maximally attainable VO2 during exercise in humans: the peak vs. maximum issue. J. Appl. Physiol. 95, 1901–1907. doi: 10.1152/japplphysiol.00024.2003
Enko, K., Nakamura, K., Yunoki, K., Miyoshi, T., Akagi, S., Yoshida, M., et al. (2011). Intermittent arm ischemia induces vasodilatation of the contralateral upper limb. J. Physiol. Sci. 61, 507–513. doi: 10.1007/s12576-011-0172-9
Faisal, A., Dyson, K. S., and Hughson, R. L. (2010). Prolonged ischaemia impairs muscle blood flow and oxygen uptake dynamics during subsequent heavy exercise. J. Physiol. 588, 3785–3797. doi: 10.1113/jphysiol.2010.188698
Garber, C. E., Blissmer, B., Deschenes, M. R., Franklin, B. A., Lamonte, M. J., Lee, I. M., et al. (2011). Quantity and quality of exercise for developing and maintaining cardiorespiratory, musculoskeletal, and neuromotor fitness in apparently healthy adults: guidance for prescribing exercise. Med. Sci. Sports Exerc. 43, 1334–1359. doi: 10.1249/MSS.0b013e318213fefb
Gastin, P. B. (2001). Energy system interaction and relative contribution during maximal exercise. Sports Med. 31, 725–741. doi: 10.2165/00007256-200131100-00003
Gerbino, A., Ward, S. A., and Whipp, B. J. (1996). Effects of prior exercise on pulmonary gas-exchange kinetics during high-intensity exercise in humans. J. Appl. Physiol. 80, 99–107. doi: 10.1152/jappl.1996.80.1.99
Hampson, N. B., and Piantadosi, C. A. (1988). Near infrared monitoring of human skeletal muscle oxygenation during forearm ischemia. J. Appl. Physiol. 64, 2449–2457. doi: 10.1152/jappl.1988.64.6.2449
Hughson, R. L., and Morrissey, M. A. (1983). Delayed kinetics of VO2 in the transition from prior exercise. Evidence for O2 transport limitation of VO2 kinetics: a review. Int. J. Sports Med. 4, 31–39. doi: 10.1055/s-2008-1026013
Kido, K., Suga, T., Tanaka, D., Honjo, T., Homma, T., Fujita, S., et al. (2015). Ischemic preconditioning accelerates muscle deoxygenation dynamics and enhances exercise endurance during the work-to-work test. Physiol. Rep. 3:e12395. doi: 10.14814/phy2.12395
Kooijman, M., Thijssen, D. H. J., de Groot, P. C. E., Bleeker, M. W. P., van Kuppevelt, H. J. M., Green, D. J., et al. (2008). Flow-mediated dilatation in the superficial femoral artery is nitric oxide mediated in humans. J. Physiol. 586, 1137–1145. doi: 10.1113/jphysiol.2007.145722
Krustrup, P., Gonzalez-Alonso, J., Quirstorff, B., and Bangsbo, J. (2001). Muscle heat production and anaerobic energy turnover during repeated intense dynamic exercise in humans. J. Physiol. 536, 947–956. doi: 10.1111/j.1469-7793.2001.00947.x
Lamarra, N., Whipp, B. J., Ward, S. A., and Wasserman, K. (1987). Effect of interbreath fluctuations on characterizing exercise gas exchange kinetics. J. Appl. Physiol. 62, 2003–2012. doi: 10.1152/jappl.1987.62.5.2003
MacDonald, M. J., Shoemaker, J. K., Tschakovsky, M. E., and Hughson, R. L. (1998). Alveolar oxygen uptake and femoral artery blood flow dynamics in upright and supping leg exercise in humans. J. Appl. Physiol. 85, 1622–1628. doi: 10.1152/jappl.1998.85.5.1622
MacDonald, M. J., Tarnopolsky, M. A., and Hughson, R. L. (2000). Effect of hyperoxia and hypoxia on leg blood flow and pulmonary and leg oxygen uptake ate the onset of kicking exercise. Can. J. Physiol. Pharmacol. 78, 67–74. doi: 10.1139/cjpp-78-1-67
Margaria, R., Cerretelli, P., Di Prampero, P. E., Massari, C., and Torelli, G. (1963). Kinetics and mechanism of oxygen debt contraction in man. J. Appl. Physiol. 18, 371–377. doi: 10.1152/jappl.1963.18.2.371
Mullen, M. J., Kharbanda, R. K., Cross, J., Donald, A. E., Taylor, M., Vallance, P., et al. (2001). Heterogeneous nature of flow-mediated dilatation in human conduit arteries in vivo: relevance to endothelial dysfunction in hypercholesterolemia. Circ. Res. 88, 145–151. doi: 10.1161/01.RES.88.2.145
Nascimento, P. C., de Aguiar, R. A., Teixeira, A. S., de Souza, K. M., de Lucas, R. D., Denadai, B. S., et al. (2016). Are the oxygen uptake and heart rate off-kinetics influenced by the intensity of prior exercise? Respir. Physiol. Neurobiol. 230, 60–67. doi: 10.1016/j.resp.2016.05.007
Nascimento, P. C., de Lucas, R. D., Souza, K. M., De Aguiar, R. A., Denadai, B. S., and Guglielmo, L. G. A. (2015). The effect of prior exercise intensity on oxygen uptake kinetics during high-intensity running exercise in trained subjects. Eur. J. Appl. Physiol. 115, 147–156. doi: 10.1007/s00421-014-3000-0
Paganelli, W., Pendergast, D. R., Koness, J., and Cerretelli, P. (1989). The effect of decreased muscle energy stores on the VO2 kinetics at the onset of exercise. Eur. J. Appl. Physiol. Occup. Physiol. 59, 312–316.
Poole, D. C., Barstow, T. J., McDonough, P., and Jones, A. M. (2008). Control of oxygen uptake during exercise. Med. Sci. Sports Exerc. 40, 462–474. doi: 10.1249/MSS.0b013e31815ef29b
Poole, D. C., and Jones, A. M. (2012). Oxygen uptake kinetics. Compr. Physiol. 2, 933–996. doi: 10.1002/cphy.c100072
Rossiter, H., Howe, F., and Ward, S. (2005). “Intramuscular phosphate and pulmonary VO2 kinetics during exercise,” in Oxygen Uptake Kinetics in Sport, Exercise and Medicine, eds D. Poole and A. M. Jones (London: Routledge), 154–184.
Rossiter, H. B., Ward, S. A., Howe, F. A., Kowalchuk, J. M., Griffiths, J. R., and Whipp, B. J. (2002). Dynamics of intramuscular 31P-MRS Pi peak splitting and the slow components of PCr and O2 uptake during exercise. J. Appl. Physiol. 93, 2059–2069. doi: 10.1152/japplphysiol.00446.2002
Salvador, A. F., De Aguiar, R. A., Lisboa, F. D., Pereira, K. L., Cruz, R. S., and Caputo, F. (2015). Ischemic preconditioning and exercise performance: a systematic review and meta-analysis. Int. J. Sports Physiol. Perform. 11, 4–14. doi: 10.1123/ijspp.2015-0204
Sharma, V., Cunniffe, B., Verma, A. P., Cardinale, M., and Yellon, D. (2014). Characterization of acute ischemia-related physiological responses associated with remote ischemic preconditioning: a randomized controlled, crossover human study. Physiol. Rep. 2:e12200. doi: 10.14814/phy2.12200
Souza, K. M., De Lucas, R. D., Helal, L., Guglielmo, L. G. A., Greco, C. C., and Denadai, B. S. (2016). Agreement analysis between critical power and intensity corresponding to 50% Δ in cycling exercise. Rev. Bras. Cineantropom. Desempenho Hum. 18, 197–206. doi: 10.5007/1980-0037.2016v18n2p197
Tordi, N., Perrey, S., Harvey, A., and Hughson, R. L. (2003). Oxygen uptake kinetics during two bouts of heavy cycling separated by fatiguing sprint exercise in humans. J. Appl. Physiol. 94, 533–541. doi: 10.1152/japplphysiol.00532.2002
Walsh, M. L., Takahashi, A., Endo, M., Miura, A., and Fukuba, Y. (2002). Effects of ischaemia on subsequent exercise-induced oxygen uptake kinetics in healthy adult humans. Exp. Physiol. 87, 227–235. doi: 10.1113/eph8702262
Whipp, B. J., Higgenbotham, M. B., and Cobb, F. C. (1996). Estimating exercise stroke volume from asymptotic oxygen pulse in humans. J. Appl. Physiol. 81, 2674–2679. doi: 10.1152/jappl.1996.81.6.2674
Wilkerson, D. P., Berger, N. J., and Jones, A. M. (2006). Influence of hyperoxia on pulmonary O2 uptake kinetics following the onset of exercise in humans. Respir. Physiol. Neurobiol. 153, 92–106. doi: 10.1016/j.resp.2005.09.006
Keywords: oxygen uptake kinetics, ischemia-reperfusion, cardiopulmonary test, physical exercise, exercise physiology
Citation: Helal L, do Nascimento Salvador PC, de Lucas RD and Guglielmo LGA (2019) Thigh Ischemia-Reperfusion Model Does Not Accelerate Pulmonary VO2 Kinetics at High Intensity Cycling Exercise. Front. Physiol. 10:160. doi: 10.3389/fphys.2019.00160
Received: 20 April 2018; Accepted: 08 February 2019;
Published: 25 February 2019.
Edited by:
Keith Russell Brunt, Dalhousie University, CanadaReviewed by:
Joanna E. MacLean, University of Alberta, CanadaCopyright © 2019 Helal, do Nascimento Salvador, de Lucas and Guglielmo. This is an open-access article distributed under the terms of the Creative Commons Attribution License (CC BY). The use, distribution or reproduction in other forums is permitted, provided the original author(s) and the copyright owner(s) are credited and that the original publication in this journal is cited, in accordance with accepted academic practice. No use, distribution or reproduction is permitted which does not comply with these terms.
*Correspondence: Lucas Helal, bHVjYXMuaGVsYWxAdWZyZ3MuYnI= orcid.org/0000-0002-6900-7185
Disclaimer: All claims expressed in this article are solely those of the authors and do not necessarily represent those of their affiliated organizations, or those of the publisher, the editors and the reviewers. Any product that may be evaluated in this article or claim that may be made by its manufacturer is not guaranteed or endorsed by the publisher.
Research integrity at Frontiers
Learn more about the work of our research integrity team to safeguard the quality of each article we publish.