- 1College of Life Sciences, Fujian Agriculture and Forestry University, Fuzhou, China
- 2State Key Laboratory of Biocontrol, School of Life Sciences, Sun Yat-sen University, Guangzhou, China
- 3College of Crop Sciences, Fujian Agriculture and Forestry University, Fuzhou, China
Adipokinetic hormones (AKHs) are well known to mobilize lipids and carbohydrates for energy-consuming activities in insects. These neuropeptides exert their functions by interacting with AKH receptors (AKHRs) located on the plasma membrane of fat body cells, which regulates energy mobilization by stimulating lipolysis of triacylglycerols (TAG) to diacylglycerols (DAG) and conversion of glycogen into trehalose. Here, we investigated the roles of AKH/AKHR signaling system in trehalose metabolism and vitellogenesis during female reproduction in the brown planthopper, Nilaparvata lugens. Knockdown of AKHR expression by RNA interference (RNAi) resulted in a decrease of the circulating trehalose in hemolymph and significantly increased levels of two trehalases in fat bodies, indicating that the modulation of hemolymph trehalose levels by AKHR may be mediated by regulating trehalose degradation. In addition, adult females that had been injected with double-stranded RNA (dsRNA) for AKHR exhibited delayed oocyte maturation, prolonged pre-oviposition period, as well as decline in egg number and reduction in fecundity. Considering that these phenotypes resulting from AKHR silencing are similar to those of vitellogenin receptor (VgR) RNAi, we further analyzed a possible connection between AKHR and vitellogenesis. Knockdown of AKHR showed no effects on the Vg synthesis in fat bodies, whereas it significantly reduced the levels of VgR in ovaries. With RNAi-females, we observed an increase of Vg accumulation in hemolymph and a decrease of Vg deposition in ovaries. Moreover, the decrease in VgR expression and Vg incorporation by developing oocytes could be partially rescued by injection of trehalose into AKHR RNAi females. The present study has implicated trehalose in the AKH/AKHR signaling-mediated control of reproduction and provided new insight into mechanisms of AKH/AKHR regulation of trehalose metabolism in insect vitellogenesis, oocyte maturation and fecundity.
Introduction
Adipokinetic hormone (AKH) is a neuropeptide that is synthesized by the corpora cardiaca, stored within secretory vacuoles and secreted into hemolymph during energy-demanding conditions in insects (Gäde and Auerswald, 2003; Gáliková et al., 2015). The levels of hemolymph carbohydrate and lipid are tightly regulated by AKH, which is thought to be functionally analogous to mammalian glucagon (Lee and Park, 2004; Bharucha et al., 2008). AKH peptides are eight to ten amino acids in length, with the aromatic residues at position 4 and 8, a hydroxylated residue at position 5, a glycine residue at position 9, a pyroglutamated N-terminus and an amide blocked C-terminus. These highly conserved residues are essential for biological activity of AKH peptides (Gäde and Marco, 2013). To date, more than 60 different AKH forms have been identified in various insect species with similar structural characteristics (Gäde and Marco, 2013). AKH belongs to a class of structurally related neurohormones that interact with G protein coupled receptors (GPCRs), which regulates energy mobilization by stimulating lipolysis of triacylglycerols (TAG) to diacylglycerols (DAG) and glycogenolysis of glycogen to trehalose in the fat body (Grönke et al., 2007; Caers et al., 2012).
Adipokinetic hormones-stimulated energy mobilization relies on signaling via an adipokinetic hormone receptor (AKHR), which is composed of seven transmembrane-spanning alpha-helices, first identified from the fruit fly Drosophila melanogaster (Park et al., 2002) and the silkworm Bombyx mori (Staubli et al., 2002). To date, AKHRs have been identified or predicted from genome sequencing projects in several other insect species across many orders, including the cockroaches (Blattodea) Periplaneta americana (Hansen et al., 2006; Wicher et al., 2006) and Blattella americana (Huang et al., 2011), mosquitoes (Diptera) Anopheles gambiae (Kaufmann and Brown, 2006) and Aedes aegypti (Kaufmann et al., 2009), flies (Diptera) Glossina morsitans (Attardo et al., 2012), Sarcophaga crassipalpis (Bil et al., 2016) and Bactrocera dorsalis (Hou et al., 2017), wasps and bumblebees (Hymenoptera) Nasonia vitripennis (Hansen et al., 2010) and Bombus terrestris (Jedlička et al., 2016), beetle (Coleoptera) Tribolium castaneum (Li et al., 2008), moth (Lepidoptera) Manduca sexta (Ziegler et al., 2011), cricket (Orthoptera) Gryllus bimaculatus (Konuma et al., 2012), aphids (Hemiptera) Acyrthosiphon pisum (Li et al., 2013) and Pseudoregma bambucicola (Jedličková et al., 2015) and the triatomine hemipteran Rhodnius prolixus (Alves-Bezerra et al., 2016). AKH signaling is achieved by binding this peptide with AKHR located on the plasma membrane of fat body cells and then stimulating the intracellular production of Ca2+ and cyclic adenosine monophosphate (cAMP), and also activating protein kinase A (PKA) (Arrese et al., 1999; Van der Horst et al., 2001; Gäde and Auerswald, 2003).
Adipokinetic hormones/AKHR signaling possesses a wide variety of functions in different insect species (Dalibor, 2008). Of all the pleiotropic actions, the most crucial one is the regulation of energy mobilization to maintain hemolymph lipid and carbohydrate levels (Caers et al., 2012). Activation of AKH/AKHR signaling is usually a response to periods of starvation, stress or increased energy demand (Gäde and Auerswald, 2003; Van der Horst, 2003). In D. melanogaster, ablation of AKH-producing cells led to reduced levels of trehalose in hemolymph, inability to maintain glucose homeostasis and increased survival rates in response to starvation stress due to low levels of energy mobilization (Kim and Rulifson, 2004; Lee and Park, 2004; Isabel et al., 2005). Knockdown of AKHR in cricket G. bimaculatus by RNA interference (RNAi) resulted in decreased levels of DAG and trehalose in hemolymph, which significantly enhanced starvation resistance and feeding frequency (Konuma et al., 2012). Besides this direct energy-utilization function, AKH/AKHR signaling plays an important role in the regulation of reproduction. The fact that AKHR is structurally and functionally analogous to the vertebrate gonadotropin-releasing hormone (GnRH) receptor, coinciding with AKHR transcript expression in the fat body and ovary in some insect species, supports the idea that AKH/AKHR signaling-mediated nutrient metabolism might be critical for female reproduction (Lindemans et al., 2009). Recent studies have shown that knockdown of AKHR resulted in TAG accumulation and affected sexual courtship activity, fecundity and tethered-flight duration in B. dorsalis (Hou et al., 2017). In the tsetse fly, G. morsitans, for example, AKHR knockdown led to an inability to utilize lipid reserves that is required for milk production during female pregnancy and caused delayed oocyte development (Attardo et al., 2012). In the nematode Caenorhabditis elegans, AKH-GnRH silencing caused a delay of egg-laying and a reduction in the number of progeny produced (Lindemans et al., 2009). In the cricket G. bimaculatus, AKHs were found to be the triggers of catabolism in the fat body that energetic substrates to be incorporated into the developing oocytes (Lorenz and Gäde, 2009), and the energy homeostasis in fat body is critical for oogenesis in G. bimaculatus (Lorenz et al., 2004). However, the mechanisms underlying the control of insect female reproduction by AKH/AKHR signaling are poorly understood.
Production of eggs is one of the most energy-demanding events in the adult life of the female insects. During oogenesis, vitellogenin (Vg) is synthesized in the fat body, secreted into hemolymph and then incorporated into the developing oocytes by vitellogenin receptor (VgR)-mediated endocytosis (Tufail and Takeda, 2008, 2009). In addition to Vg, large amounts of carbohydrates and lipids have to be provided to meet the energetic demands of oocyte growth (Ziegler and Ibrahim, 2001; Thompson, 2003). It is obvious that the female reproductive processes require considerable amounts of energy-rich substrates, and AKH/AKHR signaling-mediated energy mobilization may be involved in the regulation of egg production. Several studies show that the role of AKHs in insect egg production is the inhibition of anabolic processes like vitellogenesis, protein and lipid synthesis in the fat body (Carlisle and Loughton, 1979; Gokuldas et al., 1988; Moshitzky and Applebaum, 1990; Dalibor, 2008). In adult crickets G. bimaculatus, AKH injection resulted in retarded oocyte maturation, decreased number of terminal oocytes and delayed egg-laying by interfering with the formation of energy reserves and the synthesis of Vg in the fat body that are mobilized to fuel egg production (Lorenz, 2003). It is also implied that AKH/AKHR signaling is critical for making circulating metabolites, such as trehalose and DAG, available in the hemolymph (Gäde and Auerswald, 2003). Since trehalose is the major circulating sugar that is used for oocyte growth (Thompson, 2003), and the involvement of trehalose in Vg synthesis in the fat body and Vg uptake by the developing oocytes has been confirmed in the migratory locust, Locusta migratoria and the cockroach, P. americana (Tanaka et al., 1998; Kono et al., 2001), it is presumed that AKH/AKHR signaling-dependent trehalose homeostasis operates to regulate vitellogenesis and oocyte development in female insects.
To test this hypothesis, RNAi experiments were performed to silence AKHR expression and the effects on vitellogenesis and trehalose metabolism were investigated in the brown planthopper Nilaparvata lugens. The involvement of trehalose in the regulation of vitellogenesis and oocyte development was also investigated. Our results indicate that AKH/AKHR signaling-mediated trehalose metabolism is important for Vg incorporation by developing oocytes and thereby facilitates female reproduction in N. lugens.
Materials and Methods
Insect Rearing Conditions
The population of N. lugens was originally sourced from adults collected from South China Agriculture University in September 2008 (Lu et al., 2015). Insects were maintained at 26 ± 1°C and 65% ± 5% relative humidity, and fed with fresh rice seedlings (Taichung Native 1) under a long-day lighting condition (16L: 8D). New females were selected and separated within 24 h post-adult emergence until they were used in experiments.
Reverse Transcription PCR (RT-PCR) and Real-Time Quantitative PCR (qPCR)
Total RNA was extracted from ten whole adult females or specific tissues dissected from thirty females using Trizol reagent (Invitrogen, California, CA, United States). DNase I (Promega, Madison, WI, United States) was used for removing genomic DNA contamination. Five μg of RNA for each sample was used for cDNA synthesis using the GoScript Reverse System (Promega) with random primers and oligo (dT) in a total volume of 20 μL. Specific primers used for RT-PCR and qPCR were listed in Table 1 or from previously reported research (Tang et al., 2017; Zhang et al., 2017). For RT-PCR, partial cDNA fragments of NlAKHR, Nlβ-actin (EU179850) and NlTUB (alpha 2-tubulin, FJ810204) were amplified in a Veriti PCR thermal cycler (Applied Biosystems, Foster City, CA, United States) using the GoTaq Master Mix (Promega) under the following conditions: one cycle for 2 min at 95°C, followed by 30 cycles of 30 s at 95°C, 30 s at 60°C and 45 s at 72°C. PCR products were subjected to 1.5% agarose gel electrophoresis and stained with ethidium bromide. qPCR was performed in a StepOnePlusTM Real-Time PCR system (Applied Biosystems) with the UltraSYBR Mixture (CWBIO, Beijing, China) using an amplification program as follow: initial incubation at 95°C for 10 min, followed by 40 cycles of 95°C for 10 s, 60°C for 15 s, and 72°C for 20 s. All qPCR assays were performed in triplicate, and relative levels of mRNAs were normalized using two internal controls (Nlβ-actin and NlTUB) (Yuan et al., 2014) and calculated with 2-ΔΔCT method (Livak and Schmittgen, 2001).
RNA Interference (RNAi) of AKHR and Bioassays
Double-stranded RNA (dsRNA) was synthesized by T7 RiboMAXTM Express RNAi System (Promega) using specific primers linked with T7 promoter sequences at 5′ends for NlAKHR (591 bp) or green fluorescent protein gene (GFP, ACY56286, 542 bp) (Table 1) (Chen et al., 2010). After in vitro transcription reaction, DNase I and RNase (Promega) were added to remove genomic DNA and single-stranded RNA (ssRNA) contamination. Products were subjected to 1.5% agarose gel electrophoresis to confirm dsRNA integrity, and dsRNA concentration was measured spectrophotometrically at 260 nm using a Nanodrop2000C (Thermo Fisher Scientific, West Palm Beach, FL, United States). Newly emerged female adults (within 24 h after emergence) were anesthetized by carbon dioxide and 23 nL of diethyl pyrocarbonate (DEPC)-treated water (DW) containing 100 ng of dsRNA against the NlAKHR sequence or a control GFP was injected into each female. The dsRNA was injected into the conjunctive between prothorax and mesothorax using a Nanoject II microinjection device (Drummond Scientific, Broomall, PA, United States) (Liu et al., 2010). Knockdown efficiencies of target genes were determined by RT-PCR and qPCR on the 3rd and 6th day after dsRNA injection as described above.
Biological performance parameters after dsRNA injection were measured using the method of Ge et al. (2015) with slight modification. Briefly, one injected female was mated with two males and reared on fresh rice seedlings for oviposition. The number of laid eggs was recorded daily using a microscope, and the pre-oviposition period was counted as the time from adult female emergence to the onset of egg-laying. For fecundity analysis, the parents were removed 15 days later and the numbers of hatched offspring were counted. Ovaries were dissected on day 6 post-injection and photographed with a SMZ18 stereomicroscope (Nikon, Tokyo, Japan). At least 12 females were used per treatment and three independent biological replicates were performed.
NlAKH Treatment and Trehalose Content Determination
NlAKH peptide (pQVNFSPNW-NH2) was chemically synthesized (GenScript Biotech Inc., Nanjing, China) and dissolved in dimethyl sulfoxide (DMSO) as described previously (Lu et al., 2018b). Three-day-old females were immobilized by a short exposure to carbon dioxide and then injected with either NlAKH or the same volume of DMSO (control). The injected females were left for 90 min and then a 5 μL hemolymph was collected using centrifugation methods of Konuma et al. (2012) and Xu et al. (2013) with moderate modifications.
Hemolymph (2 μL) was mixed with 250 μL of 0.25 M Na2CO3 buffer solution, and then incubated at 95°C for 10 min to inactivate endogenous enzymes. After cooling, 150 μL of 1 M acetic acid and 600 μL of 0.25 M sodium acetate (pH 5.2) were added, and centrifuged at 12000 × g at 24°C for 10 min. The supernatant (100 μL) was mixed with 1 μL of porcine kidney trehalase (Sigma Aldrich, St. Louis, MO, United States) overnight at 37°C to catalyze the conversion of trehalose into glucose. A 30 μL aliquot of this solution was further mixed with 100 μL of a glucose reagent solution (Sigma-Aldrich) and then incubated at 37°C for 30 min. Glucose concentration was measured at 340 nm using a Nanodrop 2000C spectrophotometer (Thermo Fisher Scientific), and calculated by comparison of enzymatic-reacted glucose standard curve before treatment with trehalase. Trehalose concentration was determined by comparison of enzymatic-reacted trehalose standard curve after treatment with trehalase.
Trehalose Injection and Western Blot Analysis
Newly emerged females, injected with dsAKHR, were reared on rice plant seedlings for 2 days. They were subsequently used for trehalose injection using the methods described by a previous report with moderate modifications (Kikuta et al., 2012). Briefly, 23 nL of either 2 M trehalose or ultrapure water as a negative control was injected into the conjunction between prothorax and mesothorax of N. lugens females by using a Nanoject II microinjection device.
Protein isolation and western blot were performed using the method of Lu et al. (2018a) with moderate modifications. Briefly, total protein was isolated from ten whole adult females or specific tissues dissected from thirty females using a Tissue Protein Extraction Kit (CWBIO, Beijing, China) according to the manufacturer’s instructions. The protein was treated with protease inhibitor cocktail and 30 μg of protein was loaded in each lane of a 10% SDS-PAGE. Blots were blocked with 5% (w/v) milk in Tris-buffered saline (TBS). The following primary antibodies were used: rabbit anti-Vg (dil. 1:5000), rabbit anti-VgR (dil. 1:1000) and rabbit anti-β-actin (dil. 1:2000) (Lu et al., 2015). Primary antibodies were further combined with goat anti-rabbit horseradish peroxidase (HRP)-linked secondary antibody (dil. 1:5000). Signals were detected with Super Signal West Pico system (Pierce, Rockford, IL, United States). Images were acquired with a GBOX-Chemi XT4 imaging system (Syngene, Cambridge, United Kingdom).
Statistical Analyses
Student’s t-test was used for the comparison of two different conditions and one-way analysis of variance (ANOVA) followed by Duncan’s multiple comparison was used for the comparison among more than two different conditions. All statistical analyses were performed with GraphPad Prism 7.0 software (GraphPad Software, San Diego, CA, United States) and data are presented as means ± SE (standard error). Differences were considered significant at P < 0.05.
Results
Knockdown Efficiency of NlAKHR by RNAi
To confirm the role of AKHR in trehalose metabolism and female reproduction, we silenced the expression of NlAKHR by dsRNA injection. Newly emerged females were chosen for the dsRNA injection and knockdown efficiencies were determined 24 h and 48 h later. dsRNA treatment of females resulted in reduction of NlAKHR transcripts in the whole body by at least 60% in comparison to DEPC-treated water-injected and dsGFP-injected controls (Figure 1A). In the fat body of dsAKHR-injected females, the transcript abundance of NlAKHR was significantly decreased with 77.1 and 86.1% lower than those in dsGFP-injected controls at 24 and 48 h after treatment, respectively (Figure 1B).
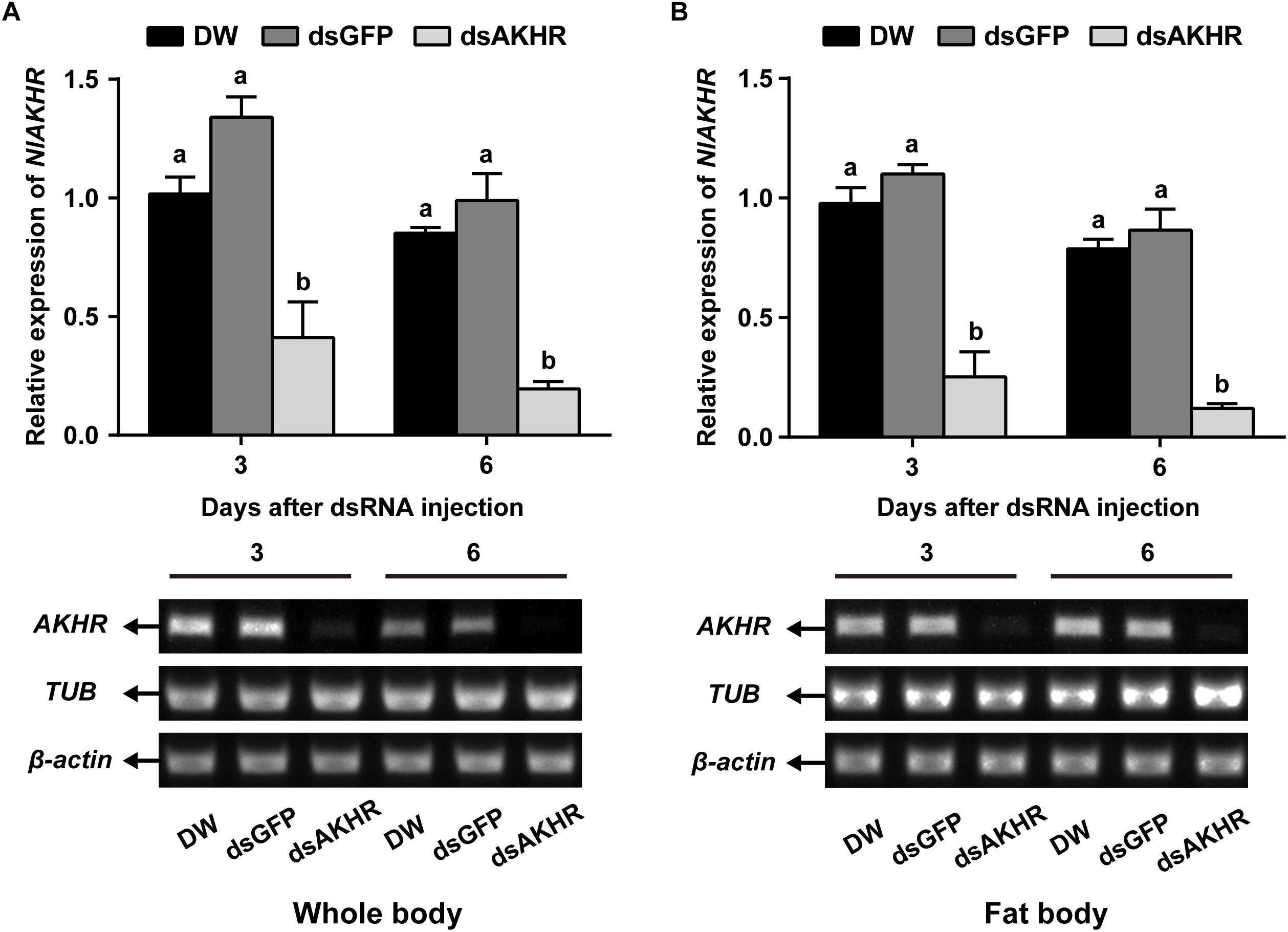
Figure 1. Knockdown of NlAKHR after double-stranded RNA (dsRNA) injection in Nilaparvata lugens. Newly emerged females were injected with DEPC-treated water (DW) or dsRNA for NlAKHR or GFP (control) genes. Whole body (A) and fat body (B) samples were obtained on the 3rd and 6th day after injection, respectively. Differences between NlAKHR expression levels were determined by RT-PCR and qPCR analyses. The results are represented as means ± SE from three independent experiments. Different lowercase letters above the columns represent significant difference at P < 0.05 using one-way ANOVA followed by Duncan’s multiple comparison.
NlAKHR Knockdown Impairs Female Reproduction
The pre-oviposition period is the time form adult female emergence to the onset of egg-laying. As shown in Figure 2A, the pre-oviposition period of the NlAKHR-silenced females was significantly prolonged by 2.3 days compared to the dsGFP-injected females. No difference was observed in the pre-oviposition periods after injection of DEPC-treated water (5.8 days) or dsGFP (6.2 days). Knockdown of NlAKHR resulted in a 26.5% reduction in the number of laid eggs compared to the dsGFP-injected controls (Figure 2B). Analysis of female fecundity revealed that females injected with dsNlAKHR produced less offspring than those treated with DEPC-treated water or dsGFP. As shown in Figure 2C, DEPC-treated water-injected and dsGFP-injected controls were capable of producing 394.1 and 331.3 offspring per female, respectively. However, silencing of NlAKHR lowered the production to 216.9 offspring per female. To ascertain the impact of NlAKHR knockdown on the reproductive system of females, we dissected the silenced females on the 6th day post-injection and found that less vitellin (Vn) was deposited in their oocytes in contrast to the fully developed oocytes from control females (Figure 2D).
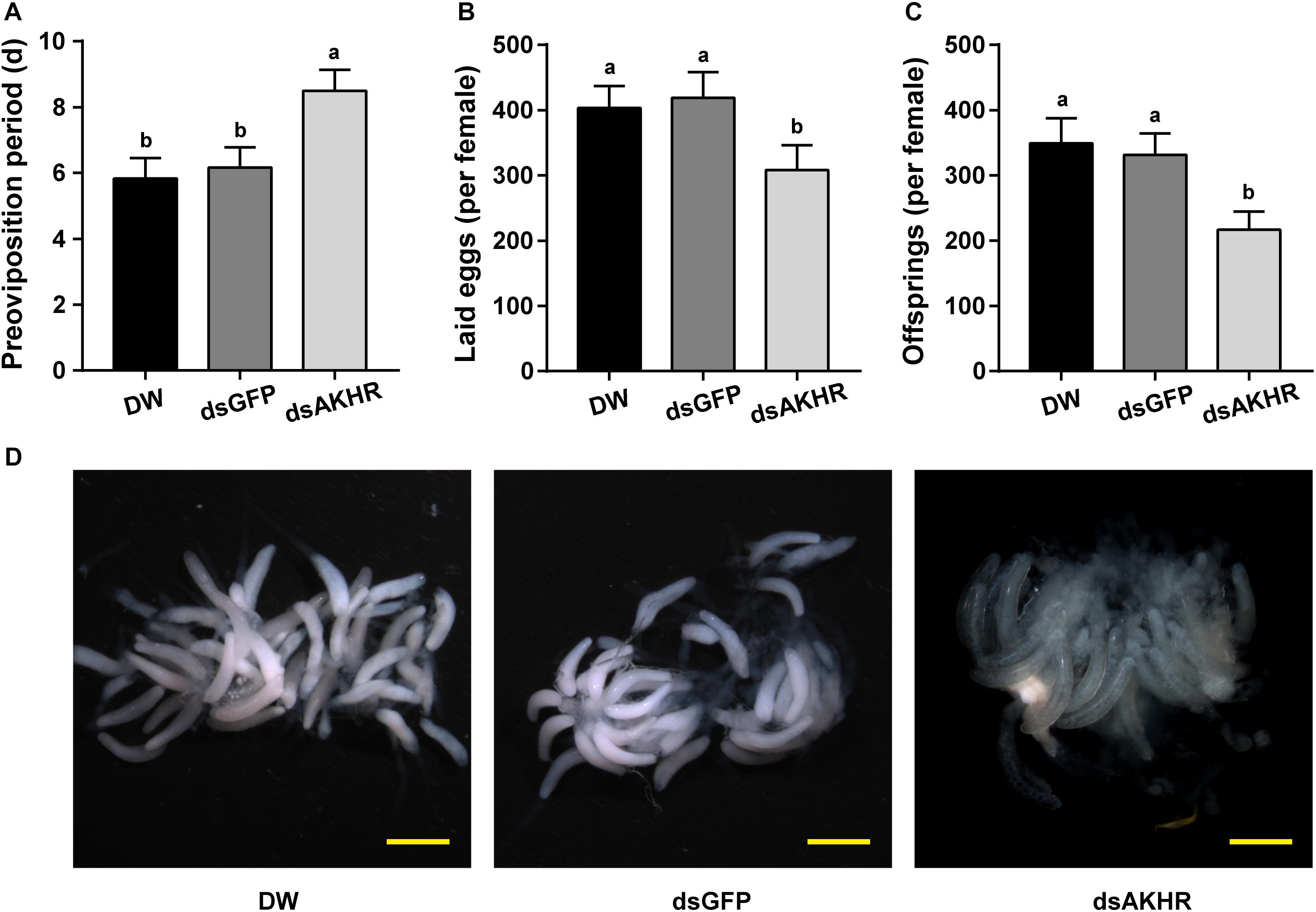
Figure 2. Knockdown of NlAKHR decreases fecundity and leads to abnormal morphology of the ovary. Newly emerged females were injected with DEPC-treated water (DW) or dsRNA for NlAKHR or GFP (control) genes. Oviposition and fecundity were monitored after dsRNA injection. (A) Pre-oviposition periods were counted and (B) the eggs laid were collected after oviposition using microscopy (n = 12). (C) Total offsprings produced by silenced and control females (n = 12). The results are represented as means ± SE from three independent experiments. Different lowercase letters above the columns represent significant difference at P < 0.05 using one-way ANOVA followed by Duncan’s multiple comparison. (D) Representative images of ovaries from control and silenced females observed under a stereomicroscope. Scale bar, 500 μm.
NlAKHR Knockdown Reduces Hemolymph Trehalose Levels and Affects the Expression of Trehalose-Relate Genes
Injection of synthetic NlAKH significantly elevated hemolymph trehalose levels by 1.4- and 1.5-fold when compared to the levels in the DMSO-injected females and untreated controls, respectively (Figure 3A). In contrast, dsAKHR-treated females resulted in reduction of the hemolymph trehalose levels by at least 65% in comparison to DEPC-treated water-injected and dsGFP-injected controls (Figure 3B). Because silencing of NlAKHR affects the hemolymph trehalose contents, we hypothesized that the NlAKHR knockdown would affect the transcription of genes related to trehalose metabolism. Transcription of the trehalase 1-1 (NlTRE1-1) gene in the fat body of NlAKHR-silenced females on the 3rd day after injection was twofold higher than that in dsGFP-injected controls. In addition, compared to dsGFP-injected females, the gene expression levels of trehalase 1–2 (NlTRE1-2) increased significantly 2.1- and 2.6-fold on the 3rd and 6th day after dsAKHR injection, respectively. However, no statistically significant effect was observed on the mRNA levels of trehalase 2 (NlTRE2), trehalose-6-phosphate synthase (NlTPS) and trehalose transporter (NlTRET) following the knockdown of NlAKHR (Figure 3C). In order to test if the eggs produce trehalase and trehalose transporter to metabolize trehalose for the production of cellular energy, we analyzed the tissue-specific expression patterns of TREs and TRET using qRT-PCR. The lowest expression levels of these four genes (NlTRE1-1, NlTRE1-2, NlTRE2, and NlTRET) were found in ovary (Supplementary Figure S1), suggesting that eggs produce trehalase and trehalose transporter to metabolize trehalose with relative low rates. Our previous study suggested that Brummer (Bmm) lipase regulates vitellogenesis partially through juvenile hormone (JH) pathway (Lu et al., 2018c). Therefore, we also analyzed whether AKHR signaling works through JH to regulate vitellogenesis. However, as shown in Supplementary Figure S2, knockdown of NlAKHR showed no effect on the expression of JH pathway-related genes.
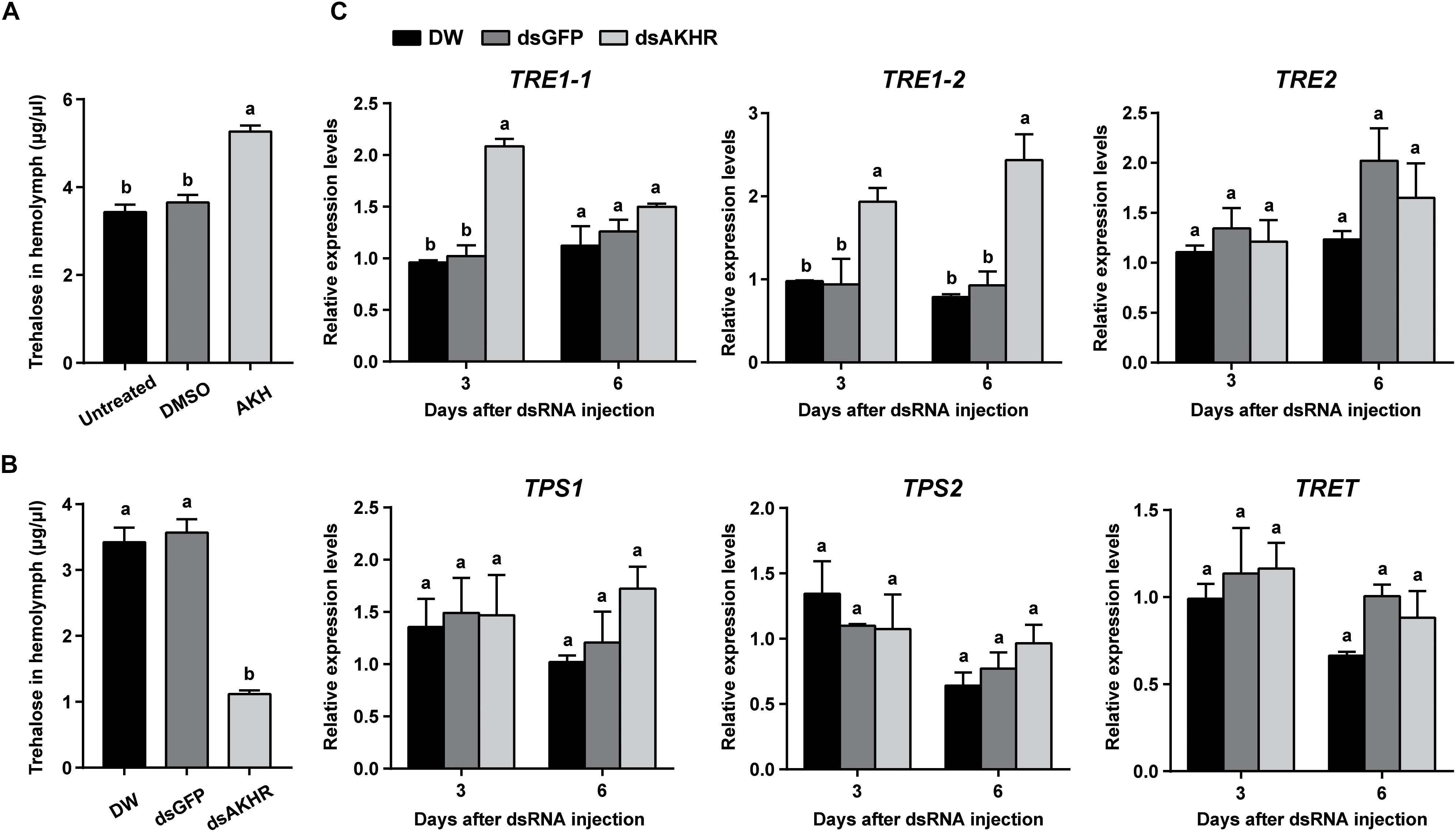
Figure 3. NlAKHR knockdown decreases hemolymph trehalose levels and affects the expression of trehalose metabolism-related genes. Three-day-old females were injected with 20 pmol of NlAKH or the same volume of DMSO (control). (A) Hemolymph was collected 90 min later and trehalose levels were determined. Newly emerged females were injected with DEPC-treated water (DW) or dsRNA for NlAKHR or GFP (control) genes. (B) Hemolymph trehalose levels were determined on the 3rd day after dsRNA injection. (C) Relative expression levels of trehalose metabolism-related genes were determined by qPCR on the 3rd and 6th day after dsRNA injection. The results are represented as means ± SE from three independent experiments. Different lowercase letters above the columns represent significant difference at P < 0.05 using one-way ANOVA followed by Duncan’s multiple comparison.
NlAKHR Knockdown Suppresses Vitellogenin Uptake by Oocytes
Because NlAKHR knockdown resulted in less Vn deposition in the ovary, we predicted that this receptor would exert a major role in vitellogenesis. To test this hypothesis, we first determined the biosynthesis of Vg in whole body after NlAKHR knockdown. When compared to controls, NlAKHR-deficient females did not exhibit any significant changes in NlVg mRNA levels and NlVg protein abundances in the whole body (Figure 4A). However, knockdown of NlAKHR resulted in reduced NlVg protein levels in the ovary (Figure 4B), whereas caused an accumulation of NlVg in the hemolymph (Figure 4C). It seems like that suppressing NlAKHR leads to reduced fecundity, likely due to the inability of oocytes to uptake NlVg from hemolymph. Therefore, we further investigated the expression of Vg receptor (NlVgR) after knockdown of NlAKHR. In the ovary of dsAKHR-injected females, the transcripts of NlVgR were significantly decreased with 16.9 and 30.1% lower than those in dsGFP-injected controls on the 3rd and 6th day after dsRNA injection, respectively. Moreover, the amounts of NlVgR protein in the ovary of NlAKHR-deficient females were significantly reduced compared with those in controls (Figure 4D).
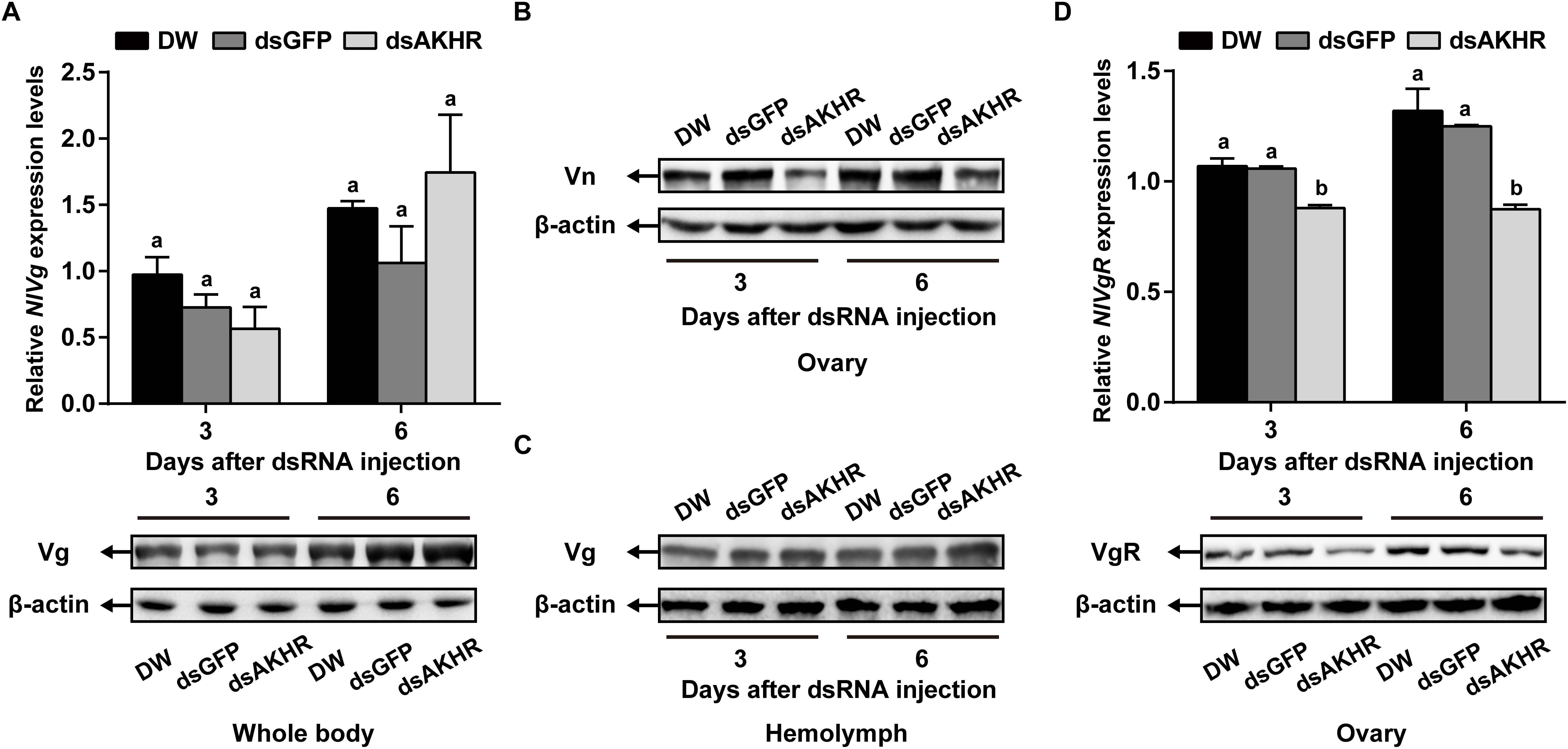
Figure 4. Effects of NlAKHR knockdown on the NlVg biosynthesis and transportation. Newly emerged females were injected with DEPC-treated water (DW) or dsRNA for NlAKHR or GFP (control) genes. (A) NlVg mRNA levels and NlVg protein abundance in the whole body were determined on the 3rd and 6th day after dsRNA injection. (B) The amounts of vitellin (Vn) in the ovary (B) and Vg in the hemolymph (C) were determined by western blot on the 3rd and 6th day after injection. (D) The ovary was obtained on the 3rd and 6th day after dsRNA injection. NlVgR mRNA levels were detected by qPCR and NlVgR protein amounts were determined by western blot. The results are represented as means ± SE from three independent experiments. Differences between cDNA levels were determined by qPCR and analyzed using one-way ANOVA followed by Duncan’s multiple comparison. Different lowercase letters above the columns represent significant difference at P < 0.05.
Trehalose Injection for NlAKHR-Deficient Females
Our data suggested that NlAKHR contributes substantially to hemolymph trehalose content and NlVg distribution, we asked whether trehalose injection would alter the uptake of NlVg by oocytes. Injection of trehalose significantly induced NlVgR gene expression by 1.6-fold in the ovary when compared to the levels in the same tissue dissected from ultrapure water-injected controls at 48 h after injection. Analysis of protein content revealed that females injected with trehalose also had higher NlVgR protein amounts in the ovary compared to those treated with ultrapure water (Figure 5A). Significant variations in NlVg protein abundances were not detected in the whole body of trehalose-injected females when compared to those in ultrapure water-injected controls (Figure 5B). However, trehalose injection caused an accumulation of NlVg in the ovary (Figure 5C) where it resulted in reduced hemolymph NlVg levels (Figure 5D).
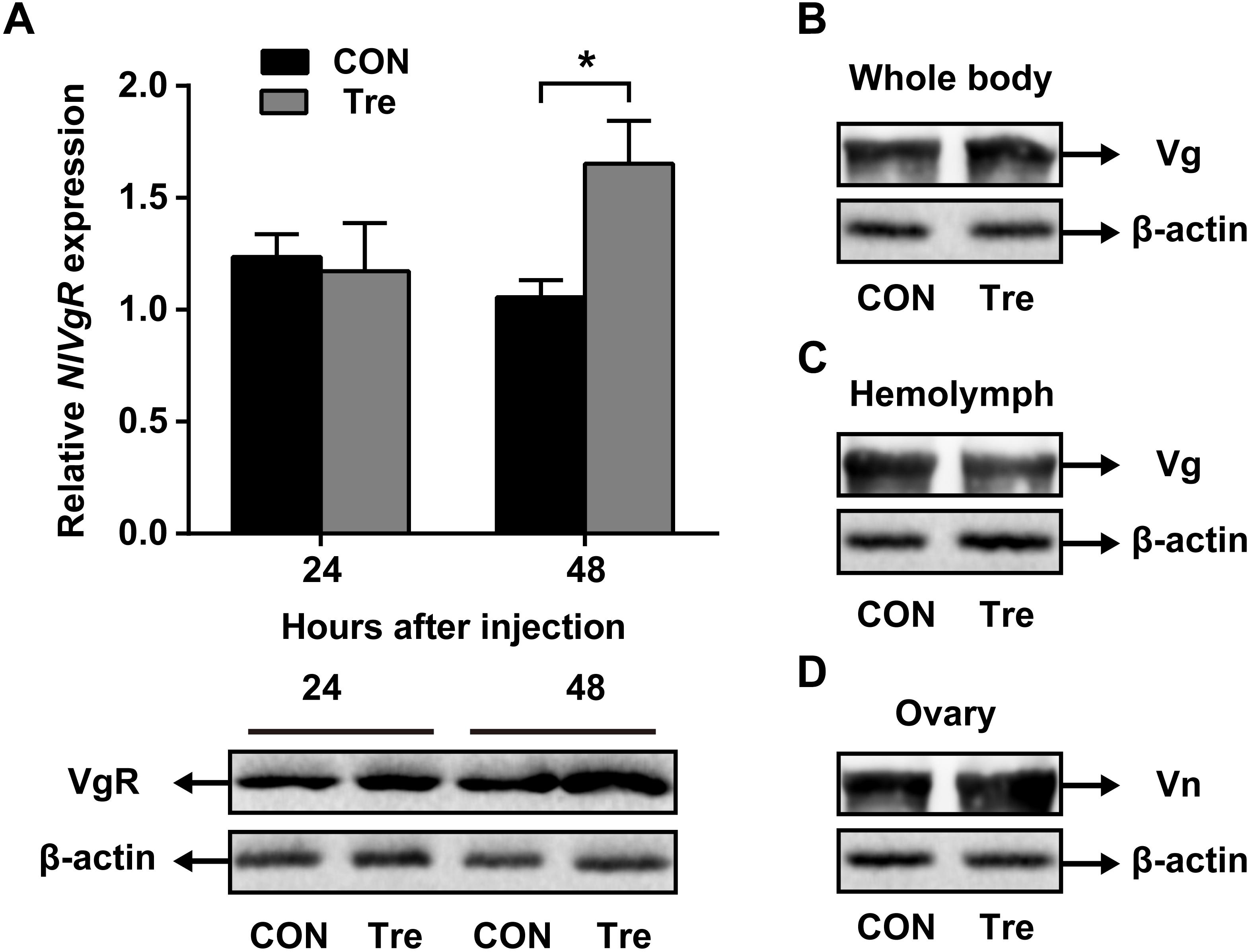
Figure 5. Effects of trehalose injection on the NlVg biosynthesis and transportation after NlAKHR knockdown. Newly emerged females were injected with dsAKHR and reared for 2 days, at which point the females were injected with either trehalose (Tre) or ultrapure water (control, CON). (A) The ovary was dissected 24 and 48 h after trehalose injection. Differences between NlVgR mRNA levels were determined by qPCR and NlVgR protein amounts were measured by western blot. NlVg protein levels in the whole body (B) and hemolymph (C) and Vn in the ovary (D) were determined 48 h after trehalose injection. Results are means ± SE from three independent experiments and asterisk represents significant difference at P < 0.05 by Student’s t-test.
NlAKH Injection Suppresses Vitellogenesis
Injection of NlAKH into N. lugens females significantly reduced NlVg mRNA expression levels by 61.11 and 97.19% with 10 and 20 pmol dosages, respectively, when compared to the levels in the fat body of DMSO-injected controls (Figure 6A). Western blot analyses showed that NlVg protein abundances were also decreased in the NlAKH-injected females. To investigate the impact of NlAKH injection on the Vg incorporation by oocytes, we further analyzed the expression levels of VgR in NlAKH-treated females. As shown in Figure 6B, the mRNA levels of NlVgR were significantly decreased by 42.76 and 71.63% after 10 and 20 pmol treatments with the synthetic NlAKH peptide, respectively. A significant reduction of NlVgR protein abundance was also observed after injection of NlAKH.
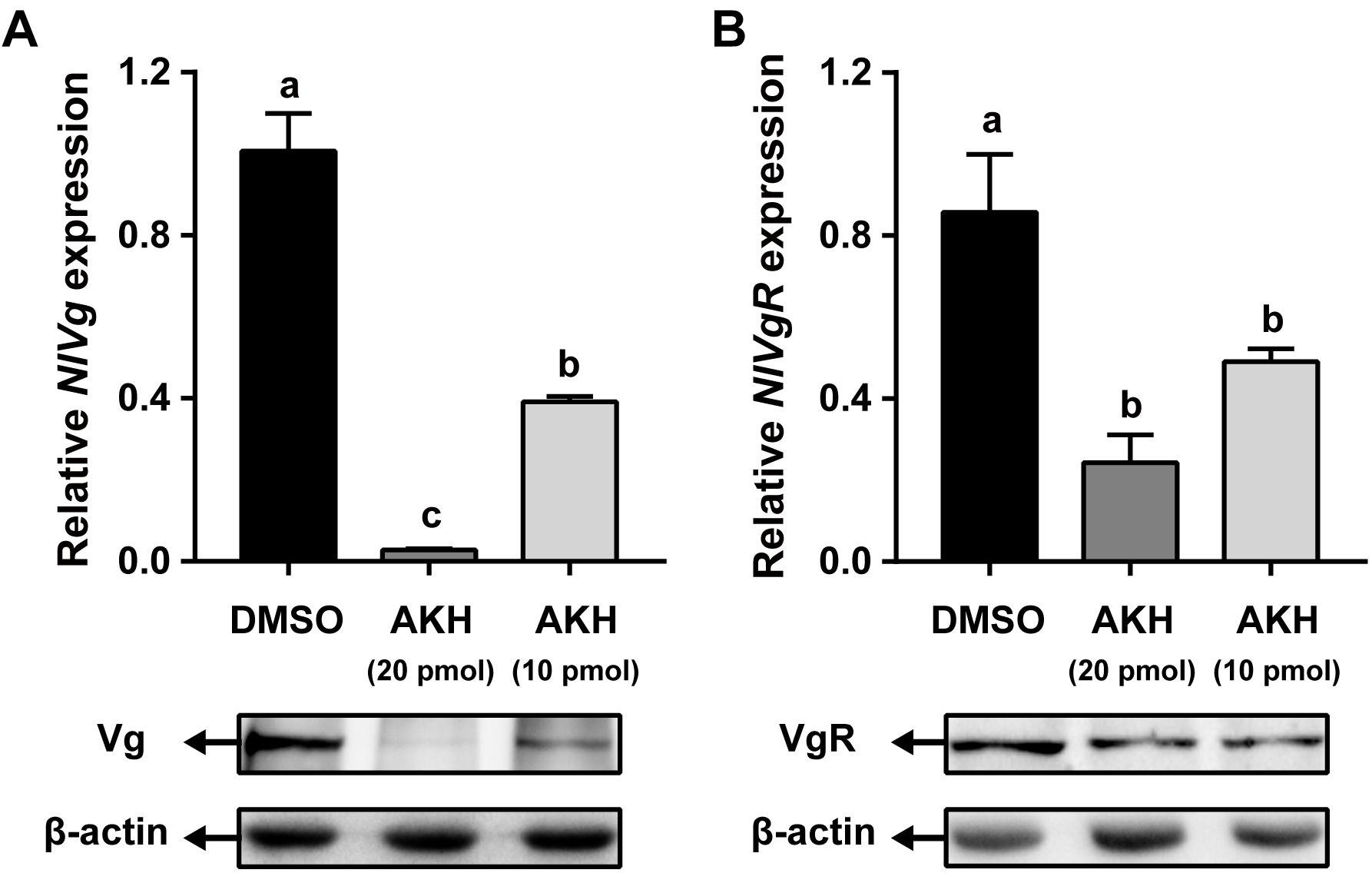
Figure 6. Effects of AKH injection on the expression of NlVg and NlVgR. Newly emerged females were injected daily with NlAKH or the same volume of DMSO (control). Transcript levels and protein abundance of NlVg (A) in the fat body and NlVgR (B) in the ovary were determined on the 3rd day. Each sample represents the means ± SE from three independent experiments. Differences between cDNA levels were determined by qPCR and analyzed using one-way ANOVA followed by Duncan’s multiple comparison. Different lowercase letters above the columns represent significant difference at P < 0.05.
Discussion
Previously, we have identified Bmm lipase systems to be critical regulators of lipolysis and JH-mediated vitellogenesis during female reproduction in N. lugens (Lu et al., 2018c; Zhou et al., 2018a,b). Here, we showed that AKH/AKHR signaling pathway, another lipolytic/glycogenolytic system, possesses a functional role in reproduction. Our results demonstrated that both Bmm lipase and AKH/AKHR signaling pathway are important for the maintenance of hemolymph metabolic homeostasis by regulating lipid or carbohydrate metabolism during female vitellogenesis. Interestingly, trehalose, not JH, serves as the mediator of AKHR regulation of Vg incorporation by developing oocytes. The most significant finding of this study is establishing a regulatory link between AKHR-mediated trehalose metabolism and vitellogenesis during energy provision in N. lugens female reproduction.
During insect oogenesis, the developing oocytes accumulate huge amounts of energy reserves from the hemolymph such as lipids, carbohydrates and proteins, and efficient mobilization of energy reserves in the fat body is important for adult insects (Tufail and Takeda, 2008). Evidence that female reproduction is regulated by AKH/AKHR signaling pathway has been reported previously. The AKH peptide serves as stress-responsive neurohormone by inhibiting the synthesis of energy storage and stimulating energy mobilization. The observed reduction of lipid synthesis is due to an inhibitory effect of AKH on the enzymes involved in the synthesis of fatty acid and fat (Lorenz, 2001). It is also proposed that AKH activates the TAG lipase in the fat body and thereby regulates the degradation of stored lipids (Lorenz, 2003). Since the ovary’s ability to synthesize lipids is limited, the formation and mobilization of lipid reserves in the fat body are essential for egg maturation (Hoof et al., 2005; Parraperalbo and Culi, 2011). Thus, the inhibitory effect of AKH injection on egg production is mainly attributed to the inhibition of lipid synthesis in the fat body (Lorenz, 2003). We observed that synthesized AKH interfered with the production of eggs by inhibiting vitellogenesis in the fat body. In particular, the amounts of synthesized Vg in the fat body and VgR in the oocytes were dramatically decreased by AKH-injection. Clearly, AKH exerts its function via inhibiting vitellogenesis and thereby interferes with egg production, as has been reported in G. bimaculatus (Lorenz, 2003; Lorenz and Gäde, 2009) and L. migratoria (Carlisle and Loughton, 1979; Asher et al., 1984). In this study, the injected dose of AKH (20 pmol per injection) is rather high, leading to a high concentration of AKH in the hemolymph. The amount of Vg deposited in the oocyte was reduced by AKH-injection. This can be explained by two different actions of AKH. First, the inhibitory effect of AKH on the Vg expression in the fat body is the major reason for the impaired oocyte Vg deposition in AKH-injected females. Second, the AKH-treatment has exerted a strong inhibitory effect on the capability of the oocyte to uptake Vg from hemolymph via VgR-mediated incorporation. Although it is possible that AKH injection may also affect JH or ecdysteroid titres in the hemolymph, it seems more likely that AKH acts by interfering with the synthesis of Vg in the fat body and the incorporation of Vg by the oocyte.
Functionally, AKH exerts its function in energy metabolism to maintain hemolymph lipid and carbohydrate levels by binding peptide to AKHR. In D. melanogaster, AKHR deficiency caused large amounts of TAG and glycogen to accumulate in the fat body (Grönke et al., 2007). In addition, an important role of AKHR in female reproduction has been reported. In the tsetse fly, G. morsitans, knockdown of AKHR or Bmm transcript levels caused an inability to utilize lipid reserves during pregnancy, resulting in delayed oocyte development and a severe reduction in female fecundity (Attardo et al., 2012). It is suggested that the reduced level of lipolysis in the fat body is the main cause of impaired egg production in AKHR-silenced insects. Consistent with these observations, we demonstrate here that AKHR regulates female reproduction in N. lugens. Our previous study suggested that Bmm lipase regulates vitellogenesis partially through JH pathway (Lu et al., 2018c). However, no significant variations in the expression levels of JH-pathway genes were detected after NlAKHR knockdown (Supplementary Figure S2), indicating that AKHR may work through other ways, not JH, to regulate female reproduction in N. lugens. Interestingly, we observed that knockdown of NlAKHR resulted in reduced levels of trehalose in the hemolymph and Vg deposited in the oocytes. Furthermore, we have established a regulatory link between AKHR-mediated trehalose metabolism and the vitellogenesis in egg maturation.
Trehalose is the major insect blood sugar with relatively high levels (5–100 mM) in the hemolymph to provide energy to target cells (Becker et al., 1996; Thompson, 2003). The physiological role of trehalose as a hemolymph sugar during insect reproductive processes has been reported previously. In B. germanica, a parallel relationship between hemolymph trehalose levels and ovarian maturation was observed, suggesting that trehalose supplies the energy demand required by the reproductive cycle (Huang and Lee, 2011). Moreover, injection with a highly specific trahalase inhibitor, validoxylamine A (VAA) suppressed Vg synthesis in the fat body and its uptake by the maturating oocytes, as has been demonstrated in several insect species (Tanaka et al., 1998; Kono et al., 1999). In the cockroach P. americana, inhibition of the trehalase activity resulted in a reduced Vg accumulation in the ovary, which suggests that the uptake of Vg by developing oocytes is an energy-demanding process (Kono et al., 2001). It is therefore presumed that hemolymph trehalose can be used as an energy fuel for the processes of vitellogenesis and oocyte maturation. Here, our RNAi assay showed that knockdown of NlAKHR caused a retarded oocyte maturation (Figure 2D) and a reduction of hemolymph trehalose (Figure 3B). Most likely, the inhibitory effect of NlAKHR knockdown on the trehalose-mediated Vg uptake by oocytes is the major reason for the retarded egg production of N. lugens. The hemolymph trehalose levels in insects appears to be regulated by the action of two kinds of enzymes, trehalose-6-phosphate synthase (TPS, an enzyme for trehalose synthesis) and trehalase (TRE, an enzyme for the conversion of trehalose to glucose) (Becker et al., 1996; Elbein et al., 2003; Shukla et al., 2015). Our results demonstrated that knockdown of NlAKHR significantly decreased the hemolymph trehalose abundance and increased the expression levels of two TRE genes in N. lugens (Figure 3C), suggesting that the modulation of hemolymph trehalose levels by AKHR may be mediated by regulating TRE-dependent trehalose degradation. More importantly, the decrease in VgR expression and Vg incorporation by developing oocytes could be partially rescued by injection of trehalose into NlAKHR-RNAi females (Figure 5). Together, we proposed that AKH/AKHR signaling-mediated maintenance of trehalose levels in the hemolymph is closely associated with Vg uptake and maturation of oocytes.
Knockdown of NlAKHR prolonged the pre-oviposition periods in female adults of N. lugens, which indicates that AKH/AKHR signaling is also involved in the control of oviposition. The myotropic effect of AKHs on muscle contraction has been reported in several insect species (Kodrík et al., 2000). In B. germanica, inhibition of hypertrehalosemic hormone (HTH), a neuropeptide that belongs to the AKH family, decreased the hemolymph trehalose levels and caused a delay in time of ootheca production (Huang and Lee, 2011). It is hypothesized that Bg-HTH increases muscle contraction of the oviduct to release mature eggs during oviposition. Recently, the insect AKHs have been regarded to be structurally and functionally analogous to the vertebrate GnRH (Lindemans et al., 2009), and AKHRs have been proposed to be evolutionarily related to GnRH receptors (Park et al., 2002; Staubli et al., 2002). A similar role of GnRH in oviposition has been demonstrated in nematode C. elegans, where AKH-GnRH silencing caused a delay in egg-laying and a reduction in fecundity (Lindemans et al., 2009). Furthermore, GnRH peptide stimulates contraction of oviduct to lay eggs in Octopus vulgaris (Iwakoshi et al., 2010), and GnRH is important for the rapid release of gamete in Ciona intestinalis (Terakado, 2001). These results suggest that insect AKHs are functional in regulating energy utilization and oviposition. In addition, the hemolymph trehalose levels correlated well to oviposition (Huang and Lee, 2011), which indicates that trehalose may be used as an important energy fuel for the process of oviposition. More studies are needed to clarify whether the decreased levels of hemolymph trehalose are functionally related to the delayed oviposition in NlAKHR-silenced females.
Conclusion
Our results have implicated that AKHR to be a critical regulator of trehalose metabolism in the regulation of Vg incorporation during oocyte maturation in female N. lugens.
Author Contributions
KL, QZ, and RZ designed the research and wrote the manuscript. YW, XZ, XC, WL, YC, and YL performed the experiments and analyzed the data. JZ, KY, and YS revised the manuscript. All authors listed and approved the manuscript for publication.
Funding
This work was supported by the National Natural Science Foundation of China (31772159, 31601634, 31470477, 31670414, and 31770474), the Natural Science Foundation of Fujian Province (2017J01428), the Fujian Provincial Excellent Youth Science Foundation (2017J06010), the Fujian Provincial Distinguished Youth Innovative Talent Project (2016), the One Hundred Talents Program of Fujian Province of China (2015-08), and the Distinguished Youth Talent Program of Fujian Agriculture and Forestry University (xjq201722).
Conflict of Interest Statement
The authors declare that the research was conducted in the absence of any commercial or financial relationships that could be construed as a potential conflict of interest.
Supplementary Material
The Supplementary Material for this article can be found online at: https://www.frontiersin.org/articles/10.3389/fphys.2018.01904/full#supplementary-material
References
Alves-Bezerra, M., Paula, I. F. D., Medina, J. M., Silva-Oliveira, G., Medeiros, J. S., Gäde, G., et al. (2016). Adipokinetic hormone receptor gene identification and its role in triacylglycerol metabolism in the blood-sucking insect Rhodnius prolixus. Insect Biochem. Mol. Biol. 69, 51–60. doi: 10.1016/j.ibmb.2015.06.013
Arrese, E. L., Flowers, M. T., Gazard, J. L., and Wells, M. A. (1999). Calcium and cAMP are second messengers in the adipokinetic hormone-induced lipolysis of triacylglycerols in Manduca sexta fat body. J. Lipid Res. 40, 556–564.
Asher, C., Moshitzky, P., Ramachandran, J., and Applebaum, S. W. (1984). The effects of synthetic locust adipokinetic hormone on dispersed locust fat body cell preparations: cAMP induction, lipid mobilization, and inhibition of protein synthesis. Gen. Comp. Endocrinol. 55, 167–173. doi: 10.1016/0016-6480(84)90098-4
Attardo, G. M., Benoit, J. B., Michalkova, V., Yang, G., Roller, L., Bohova, J., et al. (2012). Analysis of lipolysis underlying lactation in the tsetse fly, Glossina morsitans. Insect Biochem. Mol. Biol. 42, 360–370. doi: 10.1016/j.ibmb.2012.01.007
Becker, A., Schlöder, P., Steele, J. E., and Wegener, G. (1996). The regulation of trehalose metabolism in insects. Experientia 52, 433–439. doi: 10.1007/BF01919312
Bharucha, K. N., Tarr, P., and Zipursky, S. L. (2008). A glucagon-like endocrine pathway in Drosophila modulates both lipid and carbohydrate homeostasis. J. Exp. Biol. 211, 3103–3110. doi: 10.1242/jeb.016451
Bil, M., Timmermans, I., Verlinden, H., and Huybrechts, R. (2016). Characterization of the adipokinetic hormone receptor of the anautogenous flesh fly, Sarcophaga crassipalpis. J. Insect Physiol. 89, 52–59. doi: 10.1016/j.jinsphys.2016.04.001
Caers, J., Verlinden, H., Zels, S., Vandersmissen, H. P., Vuerinckx, K., and Schoofs, L. (2012). More than two decades of research on insect neuropeptide GPCRs: an overview. Front. Endocrinol. 3:151. doi: 10.3389/fendo.2012.00151
Carlisle, J. A., and Loughton, B. G. (1979). Adipokinetic hormone inhibits protein synthesis in Locusta. Nature 282, 420–421. doi: 10.1038/282420a0
Chen, J., Zhang, D., Yao, Q., Zhang, J., Dong, X., Tian, H., et al. (2010). Feeding-based RNA interference of a trehalose phosphate synthase gene in the brown planthopper, Nilaparvata lugens. Insect Mol. Biol. 19, 777–786. doi: 10.1111/j.1365-2583.2010.01038.x
Dalibor, K. (2008). Adipokinetic hormone functions that are not associated with insect flight. Physiol. Entomol. 33, 171–180. doi: 10.1111/j.1365-3032.2008.00625.x
Elbein, A. D., Pan, Y. T., Pastuszak, I., and Carroll, D. (2003). New insights on trehalose: a multifunctional molecule. Glycobiology 13, 17R–27R. doi: 10.1093/glycob/cwg047
Gäde, G., and Auerswald, L. (2003). Mode of action of neuropeptides from the adipokinetic hormone family. Gen. Comp. Endocrinol. 132, 10–20. doi: 10.1016/S0016-6480(03)00159-X
Gäde, G., and Marco, H. G. (2013). “AKH-RPCH peptides,” in Handbook of Biologically Active Peptides, ed. A. J. Kastin (San Diego, CA: Elsevier), 185–190. doi: 10.1016/B978-0-12-385095-9.00028-2
Gáliková, M., Diesner, M., Klepsatel, P., Hehlert, P., Xu, Y., Bickmeyer, I., et al. (2015). Energy homeostasis control in Drosophila adipokinetic hormone mutants. Genetics 201, 665–683. doi: 10.1534/genetics.115.178897
Ge, L. Q., Jiang, Y. P., Xia, T., Song, Q. S., Stanley, D., Peng, K., et al. (2015). Silencing a sugar transporter gene reduces growth and fecundity in the brown planthopper, Nilaparvata lugens (Stål) (Hemiptera: Delphacidae). Sci. Rep. 5:12194. doi: 10.1038/srep12194
Gokuldas, M., Hunt, P. A., and Candy, D. J. (1988). The inhibition of lipid synthesis in vitro in the locust, Schistocerca gregaria, by factors from the corpora cardiaca. Physiol. Entomol. 13, 43–48. doi: 10.1111/j.1365-3032.1988.tb00907.x
Grönke, S., Müller, G., Hirsch, J., Fellert, S., Andreou, A., Haase, T., et al. (2007). Dual lipolytic control of body fat storage and mobilization in Drosophila. PLoS Biol. 5:e137. doi: 10.1371/journal.pbio.0050137
Hansen, K. K., Hauser, F., Cazzamali, G., Williamson, M., and Grimmelikhuijzen, C. J. (2006). Cloning and characterization of the adipokinetic hormone receptor from the cockroach Periplaneta americana. Biochem. Biophys. Res. Commun. 343, 638–643. doi: 10.1016/j.bbrc.2006.03.012
Hansen, K. K., Stafflinger, E., Schneider, M., Hauser, F., Cazzamali, G., Williamson, M., et al. (2010). Discovery of a novel insect neuropeptide signaling system closely related to the insect adipokinetic hormone and corazonin hormonal systems. J. Biol. Chem. 285, 10736–10747. doi: 10.1074/jbc.M109.045369
Hoof, D. V., Rodenburg, K. W., and Van der Horst, D. J. (2005). Receptor-mediated endocytosis and intracellular trafficking of lipoproteins and transferrin in insect cells. Insect Biochem. Mol. Biol. 35, 117–128. doi: 10.1016/j.ibmb.2004.09.009
Hou, Q. L., Chen, E. H., Jiang, H. B., Wei, D. D., Gui, S. H., Wang, J. J., et al. (2017). Adipokinetic hormone receptor gene identification and its role in triacylglycerol mobilization and sexual behavior in the oriental fruit fly (Bactrocera dorsalis). Insect Biochem. Mol. Biol. 90, 1–13. doi: 10.1016/j.ibmb.2017.09.006
Huang, J. H., and Lee, H. J. (2011). RNA interference unveils functions of the hypertrehalosemic hormone on cyclic fluctuation of hemolymph trehalose and oviposition in the virgin female Blattella germanica. J. Insect Physiol. 57, 858–864. doi: 10.1016/j.jinsphys.2011.03.012
Huang, J. H., Xavier, B., and How-Jing, L. (2011). Functional characterization of hypertrehalosemic hormone receptor in relation to hemolymph trehalose and to oxidative stress in the cockroach Blattella germanica. Front. Endocrinol. 2:114. doi: 10.3389/fendo.2011.00114
Isabel, G., Martin, J. R., Chidami, S., Veenstra, J. A., and Rosay, P. (2005). AKH-producing neuroendocrine cell ablation decreases trehalose and induces behavioral changes in Drosophila. Am. J. Physiol. Regul. Integr. Comp. Physiol. 288, R531–R538. doi: 10.1152/ajpregu.00158.2004
Iwakoshi, U. E., Ukena, K. K. K., Kanda, A., Tsutsui, K., and Minakata, H. (2010). Expression and distribution of octopus gonadotropin-releasing hormone in the central nervous system and peripheral organs of the octopus (Octopus vulgaris) by in situ hybridization and immunohistochemistry. J. Comp. Neurol. 477, 310–323. doi: 10.1002/cne.20260
Jedlička, P., Ernst, U. R., Votavová, A., Hanus, R., and Valterová, I. (2016). Gene expression dynamics in major endocrine regulatory pathways along the transition from solitary to social life in a bumblebee, Bombus terrestris. Front. Physiol. 7:574. doi: 10.3389/fphys.2016.00574
Jedličková, V., Jedlička, P., and Lee, H. J. (2015). Characterization and expression analysis of adipokinetic hormone and its receptor in eusocial aphid Pseudoregma bambucicola. Gen. Comp. Endocrinol. 223, 38–46. doi: 10.1016/j.ygcen.2015.09.032
Kaufmann, C., and Brown, M. R. (2006). Adipokinetic hormones in the African malaria mosquito, Anopheles gambiae: identification and expression of genes for two peptides and a putative receptor. Insect Biochem. Mol. Biol. 36, 466–481. doi: 10.1016/j.ibmb.2006.03.009
Kaufmann, C., Merzendorfer, H., and Gäde, G. (2009). The adipokinetic hormone system in Culicinae (Diptera: Culicidae): molecular identification and characterization of two adipokinetic hormone (AKH) precursors from Aedes aegypti and Culex pipiens and two putative AKH receptor variants from A. aegypti. Insect Biochem. Mol. Biol. 39, 770–781. doi: 10.1016/j.ibmb.2009.09.002
Kikuta, S., Hagiwara-Komoda, Y., Noda, H., and Kikawada, T. (2012). A novel member of the trehalose transporter family functions as an H+-dependent trehalose transporter in the reabsorption of trehalose in Malpighian tubules. Front. Physiol. 3:290. doi: 10.3389/fphys.2012.00290
Kim, S. K., and Rulifson, E. J. (2004). Conserved mechanisms of glucose sensing and regulation by Drosophila corpora cardiaca cells. Nature 431, 316–320. doi: 10.1038/nature02897
Kodrík, D., Socha, R., Simek, P., Zemek, R., and Goldsworthy, G. J. (2000). A new member of the AKH/RPCH family that stimulates locomotory activity in the firebug, Pyrrhocoris apterus (Heteroptera). Insect Biochem. Mol. Biol. 30, 489–498. doi: 10.1016/S0965-1748(00)00025-4
Kono, Y., Takahashi, M., Matsushita, K., Nishina, M., and Kameda, Y. (1999). Effect of a trehalase inhibitor, validoxylamine A, on the oocyte development and ootheca formation in Blattella germanica and Periplaneta fuliginosa. Med. Entomol. Zool. 50, 33–39. doi: 10.7601/mez.50.33-1
Kono, Y., Takahashi, M., Matsushita, K., Nishina, M., and Kameda, Y. (2001). Inhibition of oocyte development by a trehalase inhibitor, validoxylamine A, in Periplaneta americana. Med. Entomol. Zool. 52, 23–30. doi: 10.7601/mez.52.23-1
Konuma, T., Morooka, N., Nagasawa, H., and Nagata, S. (2012). Knockdown of the adipokinetic hormone receptor increases feeding frequency in the two-spotted cricket Gryllus bimaculatus. Endocrinology 153, 3111–3122. doi: 10.1210/en.2011-1533
Lee, G., and Park, J. H. (2004). Hemolymph sugar homeostasis and starvation-induced hyperactivity affected by genetic manipulations of the adipokinetic hormone-encoding gene in Drosophila melanogaster. Genetics 167, 311–323. doi: 10.1534/genetics.167.1.311
Li, B., Predel, R., Neupert, S., Hauser, F., Tanaka, Y., Cazzamali, G., et al. (2008). Genomics, transcriptomics, and peptidomics of neuropeptides and protein hormones in the red flour beetle Tribolium castaneum. Genome Res. 18, 113–122. doi: 10.1101/gr.6714008
Li, C., Yun, X., Hu, X., Zhang, Y., Sang, M., Liu, X., et al. (2013). Identification of G protein-coupled receptors in the pea aphid, Acyrthosiphon pisum. Genomics 102, 345–354. doi: 10.1016/j.ygeno.2013.06.003
Lindemans, M., Liu, F., Janssen, T., Husson, S. J., Mertens, I., Gäde, G., et al. (2009). Adipokinetic hormone signaling through the gonadotropin-releasing hormone receptor modulates egg-laying in Caenorhabditis elegans. Proc. Natl. Acad. Sci. U.S.A. 106, 1642–1647. doi: 10.1073/pnas.0809881106
Liu, S., Ding, Z., Zhang, C., Yang, B., and Liu, Z. (2010). Gene knockdown by intro-thoracic injection of double-stranded RNA in the brown planthopper, Nilaparvata lugens. Insect Biochem. Mol. Biol. 40, 666–671. doi: 10.1016/j.ibmb.2010.06.007
Livak, K. J., and Schmittgen, T. D. (2001). Analysis of relative gene expression data using real-time quantitative PCR and the 2-ΔΔCT method. Methods 25, 402–408. doi: 10.1006/meth.2001.1262
Lorenz, M. W. (2001). Synthesis of lipids in the fat body of Gryllus bimaculatus: age-dependency and regulation by adipokinetic hormone. Arch. Insect Biochem. Physiol. 47, 198–214. doi: 10.1002/arch.1052
Lorenz, M. W. (2003). Adipokinetic hormone inhibits the formation of energy stores and egg production in the cricket Gryllus bimaculatus. Comp. Biochem. Physiol. B Biochem. Mol. Biol. 136, 197–206. doi: 10.1016/S1096-4959(03)00227-6
Lorenz, M. W., and Gäde, G. (2009). Hormonal regulation of energy metabolism in insects as a driving force for performance. Integr. Comp. Biol. 49, 380–392. doi: 10.1093/icb/icp019
Lorenz, M. W., Zemek, R., Kodrik, D., and Socha, R. (2004). Lipid mobilization and locomotor stimulation in Gryllus bimaculatus by topically applied adipokinetic hormone. Physiol. Entomol. 29, 146–151. doi: 10.1111/j.1365-3032.2004.00378.x
Lu, K., Chen, X., Li, Y., Li, W., and Zhou, Q. (2018a). Lipophorin receptor regulates Nilaparvata lugens fecundity by promoting lipid accumulation and vitellogenin biosynthesis. Comp. Biochem. Physiol. A Mol. Integr. Physiol. 219–220, 28–37. doi: 10.1016/j.cbpa.2018.02.008
Lu, K., Zhang, X., Chen, X., Li, Y., Li, W. R., Cheng, Y. B., et al. (2018b). Adipokinetic hormone receptor mediates lipid mobilization to regulate starvation resistance in the brown planthopper, Nilaparvata lugens. Front. Physiol. 9:1730. doi: 10.3389/fphys.2018.01730
Lu, K., Zhou, J., Chen, X., Li, W., Li, Y., Cheng, Y., et al. (2018c). Deficiency of brummer impaires lipid mobilization and JH-mediated vitellogenesis in the brown planthopper, Nilaparvata lugens. Front. Physiol. 9:1535. doi: 10.3389/fphys.2018.01535
Lu, K., Shu, Y., Zhou, J., Zhang, X., Zhang, X., Chen, M., et al. (2015). Molecular characterization and RNA interference analysis of vitellogenin receptor from Nilaparvata lugens (Stal). J. Insect Physiol. 73, 20–29. doi: 10.1016/j.jinsphys.2015.01.007
Moshitzky, P., and Applebaum, S. W. (1990). The role of adipokinetic hormone in the control of vitellogenesis in locusts. Insect Biochem. 20, 319–323. doi: 10.1016/0020-1790(90)90050-5
Park, Y., Kim, Y. J., and Adams, M. E. (2002). Identification of G protein-coupled receptors for Drosophila PRXamide peptides, CCAP, corazonin, and AKH supports a theory of ligand-receptor coevolution. Proc. Natl. Acad. Sci. U.S.A. 99, 11423–11428. doi: 10.1073/pnas.162276199
Parraperalbo, E., and Culi, J. (2011). Drosophila lipophorin receptors mediate the uptake of neutral lipids in oocytes and imaginal disc cells by an endocytosis-independent mechanism. PLoS Genet. 7:e1001297. doi: 10.1371/journal.pgen.1001297
Shukla, E., Thorat, L. J., Nath, B. B., and Gaikwad, S. M. (2015). Insect trehalase: physiological significance and potential applications. Glycobiology 25, 357–367. doi: 10.1093/glycob/cwu125
Staubli, F., Jorgensen, T. J., Cazzamali, G., Williamson, M., Lenz, C., Sondergaard, L., et al. (2002). Molecular identification of the insect adipokinetic hormone receptors. Proc. Natl. Acad. Sci. U.S.A. 99, 3446–3451. doi: 10.1073/pnas.052556499
Tanaka, S., Okuda, T., Hasegawa, E., and Kono, Y. (1998). Suppression of oocyte development by a trehalase inhibitor, validoxylamine A, through inhibition of juvenile hormone biosynthesis and vitellogenesis in the migratory locust, Locusta migratoria L. Entomol. Sci. 1, 313–320.
Tang, B., Yang, M., Shen, Q., Xu, Y., Wang, H., and Wang, S. (2017). Suppressing the activity of trehalase with validamycin disrupts the trehalose and chitin biosynthesis pathways in the rice brown planthopper, Nilaparvata lugens. Pestic. Biochem. Physiol. 137, 81–90. doi: 10.1016/j.pestbp.2016.10.003
Terakado, K. (2001). Induction of gamete release by gonadotropin-releasing hormone in a protochordate, Ciona intestinalis. Gen. Comp. Endocrinol. 124, 277–284. doi: 10.1006/gcen.2001.7728
Thompson, S. N. (2003). Trehalose – the insect ‘blood’ sugar. Adv. Insect Physiol. 31, 205–285. doi: 10.1016/S0065-2806(03)31004-5
Tufail, M., and Takeda, M. (2008). Molecular characteristics of insect vitellogenins. J. Insect Physiol. 54, 1447–1458. doi: 10.1016/j.jinsphys.2008.08.007
Tufail, M., and Takeda, M. (2009). Insect vitellogenin/lipophorin receptors: molecular structures, role in oogenesis, and regulatory mechanisms. J. Insect Physiol. 55, 87–103. doi: 10.1016/j.jinsphys.2008.11.007
Van der Horst, D. J. (2003). Insect adipokinetic hormones: release and integration of flight energy metabolism. Comp. Biochem. Physiol. B Biochem. Mol. Biol. 136, 217–226. doi: 10.1016/S1096-4959(03)00151-9
Van der Horst, D. J., Marrewijk, W. J., and Diederen, J. H. (2001). Adipokinetic hormones of insect: release, signal transduction, and responses. Int. Rev. Cytol. 211, 179–240. doi: 10.1016/S0074-7696(01)11019-3
Wicher, D., Agricola, H. J., Söhler, S., Gundel, M., Heinemann, S. H., Wollweber, L., et al. (2006). Differential receptor activation by cockroach adipokinetic hormones produces differential effects on ion currents, neuronal activity, and locomotion. J. Neurophysiol. 95, 2314–2325. doi: 10.1152/jn.01007.2005
Xu, J., Sheng, Z., and Palli, S. R. (2013). Juvenile hormone and insulin regulate trehalose homeostasis in the red flour beetle, Tribolium castaneum. PLoS Genet. 9:e1003535. doi: 10.1371/journal.pgen.1003535
Yuan, M., Lu, Y., Zhu, X., Wan, H., Muhammad, S., Zhan, S., et al. (2014). Selection and evaluation of potential reference genes for gene expression analysis in the brown planthopper, Nilaparvata lugens (Hemiptera: Delphacidae) using reverse-transcription quantitative PCR. PLoS One 9:e86503. doi: 10.1371/journal.pone.0086503
Zhang, L., Qiu, L. Y., Yang, H. L., Wang, H. J., Zhou, M., Wang, S. G., et al. (2017). Study on the effect of wing bud chitin metabolism and its developmental network genes in the brown planthopper, Nilaparvata lugens, by knockdown of TRE gene. Front. Physiol. 8:750. doi: 10.3389/fphys.2017.00750
Zhou, J., Chen, X., Yan, J., You, K., Yuan, Z., Zhou, Q., et al. (2018a). Brummer-dependent lipid mobilization regulates starvation resistance in Nilaparvata lugens. Arch. Insect Biochem. Physiol. 99:e21481. doi: 10.1002/arch.21481
Zhou, J., Yan, J., You, K., Chen, X., Yuan, Z., Zhou, Q., et al. (2018b). Characterization of a Nilaparvata lugens (Stål) brummer gene and analysis of its role in lipid metabolism. Arch. Insect Biochem. Physiol. 97:e21442. doi: 10.1002/arch.21442
Ziegler, R., and Ibrahim, M. M. (2001). Formation of lipid reserves in fat body and eggs of the yellow fever mosquito, Aedes aegypti. J. Insect Physiol. 47, 623–627. doi: 10.1016/S0022-1910(00)00158-X
Keywords: adipokinetic hormone receptor, trehalose, vitellogenin, vitellogenin receptor, fecundity, Nilaparvata lugens
Citation: Lu K, Wang Y, Chen X, Zhang X, Li W, Cheng Y, Li Y, Zhou J, You K, Song Y, Zhou Q and Zeng R (2019) Adipokinetic Hormone Receptor Mediates Trehalose Homeostasis to Promote Vitellogenin Uptake by Oocytes in Nilaparvata lugens. Front. Physiol. 9:1904. doi: 10.3389/fphys.2018.01904
Received: 18 October 2018; Accepted: 18 December 2018;
Published: 08 January 2019.
Edited by:
Fernando Ariel Genta, Fundação Oswaldo Cruz (Fiocruz), BrazilReviewed by:
Jae Park, The University of Tennessee, Knoxville, United StatesRaman Chandrasekar, Kansas State University, United States
Haijian Huang, Nanjing Agricultural University, China
Copyright © 2019 Lu, Wang, Chen, Zhang, Li, Cheng, Li, Zhou, You, Song, Zhou and Zeng. This is an open-access article distributed under the terms of the Creative Commons Attribution License (CC BY). The use, distribution or reproduction in other forums is permitted, provided the original author(s) and the copyright owner(s) are credited and that the original publication in this journal is cited, in accordance with accepted academic practice. No use, distribution or reproduction is permitted which does not comply with these terms.
*Correspondence: Qiang Zhou, bHNzemhvdUBtYWlsLnN5c3UuZWR1LmNu Rensen Zeng, cnN6ZW5nQHNjYXUuZWR1LmNu