- 1Joan and Sanford I. Weill, Department of Medicine, New York Presbyterian Hospital, Weill Cornell Medicine, New York, NY, United States
- 2Division of Cardiology, Department of Medicine, New York Presbyterian Hospital, Weill Cornell Medicine, New York, NY, United States
- 3Metabolic Health Center, Weill Cornell Medicine, New York, NY, United States
- 4Department of Pharmacology, Weill Cornell Medicine, New York, NY, United States
Obesity and atrial fibrillation have risen to epidemic levels worldwide and may continue to grow over the next decades. Emerging evidence suggests that obesity promotes atrial and ventricular arrhythmias. This has led to trials employing various strategies with the ultimate goal of decreasing the atrial arrhythmic burden in obese patients. The effectiveness of these interventions remains to be determined. Obesity is defined by the expansion of adipose mass, making adipocytes a prime candidate to mediate the pro-arrhythmogenic effects of obesity. The molecular mechanisms linking obesity and adipocytes to increased arrhythmogenicity in both the atria and ventricles remain poorly understood. In this focused review, we highlight areas of potential molecular interplay between adipocytes and cardiomyocytes. The effects of adipocytes may be direct, local or remote. Direct effect refers to adipocyte or fatty infiltration of the atrial and ventricular myocardium itself, possibly causing increased dispersion of normal myocardial electrical signals and fibrotic substrate of adipocytes that promote reentry or adipocytes serving as a direct source of aberrant signals. Local effects may originate from nearby adipose depots, specifically epicardial adipose tissue (EAT) and pericardial adipose tissue, which may play a role in the secretion of adipokines and chemokines that can incite inflammation given the direct contact and disrupt the conduction system. Adipocytes can also have a remote effect on the myocardium arising from their systemic secretion of adipokines, cytokines and metabolites. These factors may lead to mitochondrial dysfunction, oxidative stress, autophagy, mitophagy, autonomic dysfunction, and cardiomyocyte death to ultimately produce a pro-arrhythmogenic state. By better understanding the molecular mechanisms connecting dysfunctional adipocytes and arrhythmias, novel therapies may be developed to sever the link between obesity and arrhythmias.
Introduction
From 1980 to 2015, obesity rates at the population level have been steadily on the rise (Afshin et al., 2017). The association between obesity and hypertension, obstructive sleep apnea and diabetes is already well-known. Historically, fat has been an underappreciated organ. Much progress has been made in the last decades to elucidate the multiple functions of fat (Rosen and Spiegelman, 2014). These include endocrine functions such as control of nutritional intake (Halberg et al., 2008; Coelho et al., 2013), inflammatory functions including the production of cytokines and chemokines as well as electrical functions such as the presence of gap junctions (Linck et al., 1974). This growing knowledge along with the rise of obesity to epidemic proportions (Afshin et al., 2017) has led investigators to explore the relationship between obesity and cardiovascular disease. Specifically, of interest to us, is the relationship between obesity and arrhythmias. The links between obesity and atrial fibrillation specifically have been reviewed elsewhere (Lavie et al., 2017); however the molecular mechanisms of how fat may drive arrhythmias, both in the atria and ventricles have not been well-described. This state-of-the-art review will summarize the pathophysiologic molecular mechanisms by which obesity can lead to arrhythmia (Figure 1).
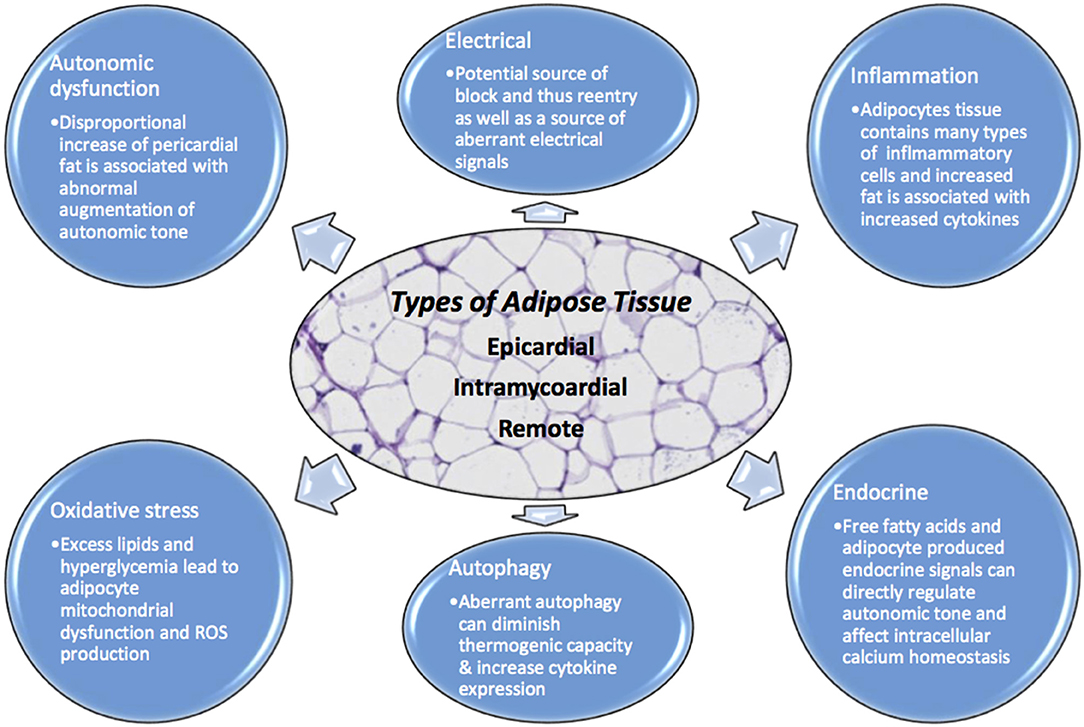
Figure 1. Adipocytes have numerous physiologic effects on myocytes including but not limited to endocrine, inflammatory, autonomic regulation, oxidative, autophagy, and electrical. When homeostasis is dysregulated due to excess adipocyte burden, no matter where the location, there is opportunity for arrhythmia. Excessive adipose tissue can be a source of increased ROS, aberrant autophagy, intracellular calcium dysregulation, autonomic tone augmentation, a cause of block and reentry among others.
Types of Fat and Arrhythmia Development
Local Adipose Tissue
The most studied fat depot linked to arrhythmia is epicardial adipose tissue (EAT). Firstly, the presence of some adipose tissue in and around the heart is physiologic. Fat around the heart can be divided into pericardial adipose tissue and visceral EAT. Pericardial fat is defined by fat surrounding the parietal pericardium while visceral EAT is defined by fat between the myocardium and visceral pericardium (Tam et al., 2016). EAT covers 80% of the heart and is present mostly along the coronary arteries, in the atrioventricular/intraventricular grooves, over the right ventricle (three or four times more than the left ventricle) especially along the right border, anterior surface and at the apex. Physiologic functions include buffering the coronary arteries against torsion and facilitating coronary artery remodeling. EAT has higher fatty acid synthesis and breakdown compared to many other fat depots (Marchington and Pond, 1990), which may serve to protect the heart against high levels of circulating lipids and as reserve in case of increased physiologic demand (i.e., exercise or infection; Iacobellis and Barbaro, 2008).
Although EAT is a type of visceral adipose tissue (VAT), it has unique characteristics such as smaller adipocytes (Bambace et al., 2014), different fatty acid composition, higher protein content and lower rates of glucose utilization (Iacobellis et al., 2005a; Iacobellis and Barbaro, 2008). The brown fat protein, uncoupling protein-1 (UCP-1), has been found to be expressed at high levels in EAT, suggesting a possible role in heart thermogenesis (Sacks et al., 2009). However, as with other homeostatic processes in the human body, there is a fine balance between physiologic quantity of EAT vs. excess. For instance, EAT accumulation may be part of a compensatory mechanism developed in response to chronic insults of the myocardial tissue, to support its higher demand-metabolic condition with free fatty acids, but this response can lead to increased inflammation and fibrosis to create a substrate for arrhythmias (Suffee et al., 2017).
Clinical imaging studies have demonstrated a strong direct correlation between epicardial adipose tissue (EAT) and abdominal visceral adiposity. EAT thickness has been reported to be associated with severity of coronary artery disease (CAD) (Jeong et al., 2007), atrial fibrillation (AF) recurrences after catheter ablation (Tsao et al., 2011), and adverse cardiovascular (CV) outcomes associated with AF (Chu et al., 2016). EAT and pericardial fat quantified by magnetic resonance imaging (MRI) is associated with the development of ventricular tachycardia (VT), ventricular fibrillation (VF) and long-term overall mortality in patients with systolic heart failure (HF; Wu et al., 2015). In an Asian population, pericardial fat thickness was also associated with increased frequency of premature ventricular contractions (Tam et al., 2016).
Multiple possible mechanisms could explain these findings. In the presence of obesity or metabolic syndrome, adipose tissue develops different characteristics resulting in changes such as “whitening” of the adipose tissue embedded within or surrounding the myocardial tissue. The adipocytes become larger in size with increased expression of inflammatory markers and decreased adiponectin levels (Bambace et al., 2014; Song et al., 2015; Zghaib et al., 2016). This pro-inflammatory environment likely leads to recruitment of inflammatory cells to perpetuate inflammation, leading to fibrosis (Cherian et al., 2012). Moreover, EAT shares the same coronary blood supply as the adjacent ventricular myocardium and has direct contact with it given the lack of fascia between adipocyte and myocardial layers (Guglielmi and Sbraccia, 2017). Therefore, EAT can have paracrine effects and directly infiltrate myocardium affecting cardiac function. Gap junctions have also been demonstrated within epicardial fat, which may lead to abnormal electrical coupling between the myocardium and EAT (Linck et al., 1974). For example, peri-atrial EAT has been found to express genes implicated in oxidative phosphorylation, muscular contraction, and calcium signaling in AF patients, which could potentially increase the translocation of cytosolic calcium into the lumen of the sarcoplasmic reticulum promoting excitation-contraction coupling and thus lead to disruptive electrophysiologic signals (Gaborit et al., 2015). Specific mechanisms associating EAT with development of arrhythmias are discussed below.
Inflammation and EAT
It is well-known that obesity is accompanied by a state of chronic low-grade inflammation. Adipose tissue contains different cell types other than adipocytes such as preadipocytes, macrophages, stromal cells, endothelial cells, and lymphocytes. The immune cells present within the adipose tissue can release a variety of chemokines, cytokines, and other factors contributing to pathogenic inflammation (Wong et al., 2017). In patients with CAD, EAT has been shown to have increased levels of inflammatory cytokines (Mazurek et al., 2003; Baker et al., 2009; Hirata et al., 2011a; Zhou et al., 2011; Greulich et al., 2012; Vianello et al., 2016) along with augmented number of pro-inflammatory M1 macrophages (Hirata et al., 2011a; Vianello et al., 2016) and T cells (Hirata et al., 2011b). Adiponectin expression in EAT (a potent anti-inflammatory adipokine) seems to be lower in patients with CAD compared to non-CAD patients (Iacobellis et al., 2005b; Zhou et al., 2011).
This greater inflammatory environment can provide substrate for arrhythmia development. In fact, increased EAT levels of adiponectin are related to decreased risk of developing post-operative AF in patients undergoing cardiac surgery (Kourliouros et al., 2011). Specifically, peri-atrial EAT seems to play an important role in the development of AF. Patients with permanent AF have increased EAT volume and serum inflammation biomarkers compared to paroxysmal AF (Nagashima et al., 2012). This correlates with the fact that inflammatory activity of left peri-atrial, atrioventricular groove, and left main artery EAT is greater than in subcutaneous or visceral thoracic tissue from patients with AF compared to controls (Mazurek et al., 2014). Consistently, peri-atrial EAT volume estimated by computer tomography is a predictor of new-onset AF in patients with CAD (Nakanishi et al., 2012). Inflammation of EAT around the LA, determined by higher density in computed tomography images, has been related to the presence of paroxysmal AF (Kusayama et al., 2016).
Recently, it has become evident that different adipose tissue depots, have distinct pro-inflammatory properties (Packer, 2018). The secretory profile pattern of EAT seems to be different than that of pericardial or subcutaneous adipose tissues (SAT) even in the same patient (Greulich et al., 2012). Specifically, EAT samples from patients with CAD have evidence of increased pro-inflammatory markers [NF-κB, IKKβ, and JNK, TNF-α, angiotensinogen, monocyte chemotactic protein (MCP-1), IL-1β, IL-6, sIL-6R] compared to SAT from the same patient (Mazurek et al., 2003; Baker et al., 2009; Hirata et al., 2011a; Zhou et al., 2011; Greulich et al., 2012; Vianello et al., 2016). Samples of EAT from patients paired for cardiovascular, CAD and AF risk factors show that the transcriptomic signature of peri-ventricular EAT appears to be more linked to inflammation in comparison to peri-atrial and peri-coronary EAT (Gaborit et al., 2015). Thus, adipose tissue function is widely heterogeneous, differing both in distinct locations in the body and various regions within the heart.
The potential harmful and pro-fibrotic effects of EAT are suggested by secretome analyses, identifying adipocytokines such as activin A, a member of the transforming growth factor- β (TGF-β) superfamily, which may promote atrial fibrosis (Venteclef et al., 2015). Additionally, rat cardiomyocytes treated with conditioned media obtained from human EAT from patients with diabetes vs. patients without diabetes demonstrate reductions in contractile dysfunction, decreased insulin-mediated Akt-Ser473-phosphorylation (activation of cell survival pathway) and elevations in SMAD2 phosphorylation/activation (a TGF-β pathway protein implicated in cardiomyocyte fibrosis) demonstrating how EAT can affect cardiomyocyte function and remodeling (Greulich et al., 2012). The same study also showed reductions in cytosolic Ca2+ fluxes and expression of SERCA2a in rat cardiomyocytes treated with conditioned media from diabetic patients, implicating altered calcium homeostasis that could lead to arrhythmias.
Oxidative Stress and EAT
Excessive production of mitochondrial reactive oxygen species (ROS) in adipose tissue is known to be an early instigator of adipose tissue inflammation (Yeop Han et al., 2010; Han, 2016). Increased lipid levels and hyperglycemia can lead to adipocyte mitochondrial dysfunction and ROS production, and this phenomenon has been associated with insulin resistance (Furukawa et al., 2004; Houstis et al., 2006; Yeop Han et al., 2010). This is evident by the fact that obese patients with type 2 diabetes mellitus seem to have increased production of ROS and decreased activity of anti-oxidant enzymes in SAT compared to controls (Chattopadhyay et al., 2015).
Few studies have looked into oxidative stress in EAT in the setting of obesity. As mentioned before, EAT seems to have a thermogenic function given its high expression of UCP-1 (Sacks et al., 2009). Cultured adipocytes isolated from EAT show that the thermogenic phenotype is negatively correlated with the oxidative stress-related parameters at the gene expression level (Chechi et al., 2017), corresponding to the whitening of EAT seen in patients with cardiovascular diseases.
In patients with cardiovascular diseases, there is increased ROS production in EAT compared to SAT, as well as lower catalase levels (Salgado-Somoza et al., 2010). Also, the SAT and EAT from the same patient differ in the post-translational modifications of proteins involved in oxidative stress such as protein disulfide isomerase A1 (PDIA1), glutathione S-transferase P1 (GSTP1), and phosphoglycerate mutase (PGAM1) (Salgado-Somoza et al., 2010). There are transgenic and knockout models of arrhythmias, such as transgenic mice with cardiac-specific overexpression of peroxisome proliferator-activated receptor gamma-1 (PPARγ1) which induces spontaneous ventricular arrhythmias (Morrow et al., 2011). However, ROS production can have beneficial properties as well. In CAD patients cardiomyocytes release products of oxidation that likely activate PPARγ-dependent upregulation of adiponectin expression in EAT, which can act as a defense mechanism (Antonopoulos et al., 2016).
Oxidative stress may play a role in the development of arrhythmias. For instance, in AF, oxidative stress has been correlated to early electrophysiological remodeling (Carnes et al., 2001). AF patients have been described to have higher levels of circulating oxidative stress markers (Neuman et al., 2007), and have upregulation of gene expression associated to oxidative stress (Kim et al., 2003). Proteins involved in myocyte contractility such as myofibrillar creatine kinase (MM-CK), have decreased activity in AF patients related to oxidative injury (Mihm et al., 2001). Anti-oxidant agents may have a therapeutic role (Carnes et al., 2001; Ozaydin et al., 2008). No studies have been done correlating oxidative stress in EAT and AF.
It is important to note that even though ROS are usually seen as deleterious. Under normal conditions, mitochondrial ROS production is increased during brown adipose tissue thermogenesis. In this case, ROS may function as signaling molecules that activate UCP-1 and abrogation of ROS production can lead to induction of hypothermia (Chouchani et al., 2016). Given that EAT has been considered brown adipose tissue with thermogenic properties, it is possible that under non-pathologic conditions, ROS are involved in EAT signaling for thermogenesis regulation as well as adiponectin expression. However, in the setting of obesity, uncontrolled ROS production can elicit inflammation.
Autophagy and EAT
Autophagy is a homeostatic process that recycles intracellular components through lysosomal degradation (Mizushima et al., 2008). The majority of the studies have shown that in the setting of obesity, autophagy may be upregulated in adipocytes, but downregulated in the liver, heart, and pancreas (Zhang et al., 2018). There is contradicting evidence regarding the beneficial and deleterious effects of autophagy in obesity and cardiovascular diseases. This is due in part to the fact that results from studies of autophagy regulation may vary depending on the model used, the organ and specific cell evaluated and the chronicity of the stimuli (Pabon et al., 2016; Zhang et al., 2018). Most of the evidence suggests there is decreased autophagic activity in obesity-related metabolic cardiomyopathy (Xu et al., 2013; An et al., 2017; Hu and Zhang, 2017). Aberrant autophagy activation in adipocytes leads to defective browning of the adipose tissue diminishing its thermogenic capacity (Okla et al., 2015), as well as increasing pro-inflammatory cytokine expression (Jansen et al., 2012). Under high fat diet (HFD) conditions, murine adipocytes have increased autophagic activity, leading to degradation of the anti-inflammatory adipokine adiponectin (Zhou and Liu, 2010), suggesting a role of adipocyte-autophagy contributing to the low grade inflammatory state in obesity. Few studies have looked into autophagy activity in EAT. In one study comparing EAT and SAT samples from heart failure patients, EAT was found to have higher expression of genes related to autophagy and mitophagy (selective autophagy of the mitochondria), as well as protein expression related to autophagy (Beclin-1 and microtubule-associated proteins 1A/1B light chain 3B (LC3B-II)) (Burgeiro et al., 2018).
There is limited data regarding the relationship between autophagy and arrhythmia pathogenesis. Autophagy has been shown to be increased in cardiomyocytes in a canine model of AF (tachypacing; Yuan et al., 2014; Wiersma et al., 2017). Furthermore, autophagy seems to be activated by increased endoplasmic reticulum (ER) stress in the setting of AF and inhibition of ER stress can prevent autophagic activity conferring protection for atrial remodeling associated with AF (Wiersma et al., 2017).
In humans, patients with persistent AF have increased autophagosome formation in atrial myocardium determined by electron microscopy and higher levels of LC3B-II compared to patients with normal sinus rhythm (Wiersma et al., 2017) which correlates with previous findings of increased LC3BII/I ratio (marker of autophagic activity) in samples from atrium from AF patients (Yuan et al., 2014). Contrary to these results, in a study using post-operative AF patients atrial samples, there was reduced levels of LC3B-II possibly suggesting impaired autophagic activity (Garcia et al., 2012). Also, higher expression of Beclin-1 and LC3BII has been reported in myocardial muscle of mouse hearts with ventricular fibrillation after ischemia reperfusion injury, compared to non-fibrillated hearts (Meyer et al., 2013). The interpretation of these findings is difficult given that these could be secondary to increased autophagic flux leading to consumption of LC3B-II or decreased autophagy protein expression. Future studies determining autophagic flux in EAT in the setting of obesity are needed.
Autonomic Dysfunction and EAT
The autonomic nervous system (ANS) has ganglionated plexi (GPs) within the heart located in the epicardial fat pads that can modulate cardiac autonomic nerve input (Zhou et al., 2014). Vagal stimulation is modulated through multiple cardiac GPs before reaching the sinus and atrio-ventricular (AV) nodes (Zhou et al., 2014). Autonomic dysfunction leading to atrial fibrillation has already been well-described. The parasympathetic system seems to contribute significantly to AF in young patients with otherwise normal hearts (Coumel, 1996). Firstly, there is significant vagal innervation of the atrial muscle sleeves extending into the pulmonary veins (Zipes and Knope, 1972). Vagal tone is mediated via acetylcholine which binds to the muscarinic receptor. This in turn can directly activate the G-protein-gated atrial K+ channel (IKACh) leading to hyperpolarization and indirectly inhibition of cyclic adenosine monophosphate (cAMP; Harvey and Belevych, 2003). This results in shortening of the atrial action potential duration with increased spatial heterogeneity (Rosenshtraukh et al., 1989) allowing for atrial fibrillation. Furthermore, GPs can be located adjacent to areas of targeted for pulmonary vein isolation (PVI). PVI can result in vagal denervation of the left atrium (Yang et al., 2011). Complex fractional atrial electrograms are commonly located at sites of GPs and ablation of complex fractionated atrial electrograms has been associated with AF ablation success in some studies although this has been controversial (Nademanee et al., 2004). Conversely, the sympathetic system also can incite atrial fibrillation as well as ventricular arrhythmias by directly triggering β-adrenergic mediated increasing of intracellular Ca2+ (Francis, 1988). Given GPs are located in the epicardial fat pads, EAT may play a strong role in regulating autonomic tone. It is possible that in the setting of obesity, EAT autonomic signals become dysregulated and allow for arrhythmia. Disproportional increase of pericardial fat may be associated with abnormal augmentation of autonomic tone, which might lead to increased VT/VF and mortality (Wu et al., 2015).
Intramyocardial Fat
There have been numerous links made between intramyocardial fat and arrhythmias. Fibrofatty replacement of myocytes is one of the hallmarks of arrhythmogenic right ventricular cardiomyopathy (ARVC) and is thought to constitute the nidus for re-entry ventricular arrhythmias (Bauce et al., 2005). The characteristic adiposis associated with ARVC seems to be part of a non-specific repair process similar to the one that takes place after ischemia (Samanta et al., 2016). It is thought that after myocardial infarction (MI), patients are at risk of developing ventricular arrhythmias due to scar tissue formation. Yet, not all patients develop VT after MI despite the presence of intramyocardial collagen (Pouliopoulos et al., 2013). This may be explained in part by the greater electrical resistance of adipose tissue compared to myocardium or collagen, which may promote reentry (Rudy et al., 1979; Fallert et al., 1993; Pouliopoulos et al., 2013). This is also supported by animal studies showing that intramyocardial adipose tissue can impede myocardial conduction and attenuate both electrogram amplitude and slope to a greater extent than collagen (Pouliopoulos et al., 2013). However, conflicting findings have been shown in an in-silico computational model, where adipose tissue was found to be less arrhythmogenic than fibrotic tissue in terms of percentage of non-conducting tissue needed to induce the arrhythmias (De Coster et al., 2018). Other mechanisms for arrhythmogenesis should also be considered, such as the presence of gap junctions in adipose tissue, that as mentioned before may lead to aberrant signal propagation (Linck et al., 1974).
Intramyocardial fat has also been associated with supraventricular arrhythmias (Haemers et al., 2017). These fibro-fatty infiltrations in human atrial biopsy samples have also been associated with increased inflammation given findings of small lymphoid aggregates composed mainly of CD8+ cytotoxic effector T cells (Haemers et al., 2017) which have been reported to have a pathogenic role in adipose tissue inflammation in the setting of obesity (Nishimura et al., 2009; Pouliopoulos et al., 2013; Haemers et al., 2017).
The relationship between EAT and the myocardium is likely to be bidirectional. In an animal model of AF using rapid atrial pacing, there was an increase in expression of genes that favor adipocyte differentiation and infiltration to the right atrium, leading to increases in adipose tissue mass (including EAT) and lipid accumulation (Chilukoti et al., 2015). A recent study also found that progenitor cells located in the epicardium can undergo epithelial-to-mesenchymal transition process and give origin to adult EAT adipocytes, and this process is driven by atrial natriuretic peptide (ANP) secreted by the atrial myocardium (Suffee et al., 2017). Recently, NLRP3 inflammasome activation in atrial cardiomyocytes was shown to promote AF, likely due to increased calcium release from the ER and increased production of pro-inflammatory cytokines (Yao et al., 2018). This is relevant given that in the setting of obesity the NLRP3 inflammasome is activated (Haneklaus and O'Neill, 2015). So it is possible that inflammasome activation can contribute to arrhythmia pathogenesis.
Remote Adipose Tissue
Remote fat cells have numerous endocrine effects. The interplay between the endocrine function of fat and the heart has not been fully elucidated. However, there are a couple of examples highlighting how increased fat burden can lead to arrhythmia. Obesity is characterized by a change in cardiac metabolism (Lopaschuk et al., 2007). Under normal conditions free fatty acids are the major source of energy for the heart given its high demand. In the setting of obesity, there is increased circulating free fatty acids, which may lead to fatty acid infiltration of myocardial tissue, increases in fatty acid oxidation, insulin resistance, and glucose intolerance, all of which decreases cardiac metabolic efficiency (Lopaschuk et al., 2007). Insulin resistance provokes systemic hyperglycemia which can result in myocardial injury (glucotoxicity; Ebong et al., 2014). Classically, obesity is also associated with low grade chronic inflammation. Visceral fat has higher levels of pro-inflammatory cytokines compared to EAT in CAD patients, leading some to think that part of the inflammatory effects may be systemic, arising from sources such as visceral fat and not just epicardial or local fat (Cheng et al., 2008).
Adipocytes secrete leptin, a peptide hormone which regulates sympathetic outflow. In a canine MI model of left anterior descending artery occlusion, leptin injection into the left stellate ganglion was associated with increased ventricular arrhythmia incidence and decreased action potential duration and duration dispersion compared to controls (Yu et al., 2018). Adipocyte fatty acid-binding protein (FABP4), which is predominantly expressed in adipose tissues, is known to depress shortening amplitude as well as intracellular systolic peak Ca2+ in a dose-dependent manner in isolated rat cardiomyocytes. Both the calcium dysregulation effect as well as the cardio-inhibitory effect leading to heart failure can lead to atrial/ventricular arrhythmias (Lamounier-Zepter et al., 2009). Also, increased leptin levels can lead to increased aldosterone secretion, systemic inflammation, endothelial dysfunction, increased vascular stiffness, hypertension, and cardiac hypertrophy, all of which could contribute to the pathogenesis of AF (Huby et al., 2015). Obesity-related adrenergic activation in particular may allow for more triggered arrhythmia. Overexpression of angiotensin I receptor and angiotensin converting enzyme related carboxypeptidase (ACE2) can lead to AV block and spontaneous ventricular tachycardia (Verheule et al., 2004).
In summary, remote adipose tissue can cause arrhythmia via a multitude of mechanisms from total body adipose tissue load related hemodynamic changes, neurohormonal mediated cardiac structural remodeling, adrenergic system activation and chronic low grade inflammation. Figure 2 reviews the different possible mechanisms implicated in arrhythmogenesis previously described.
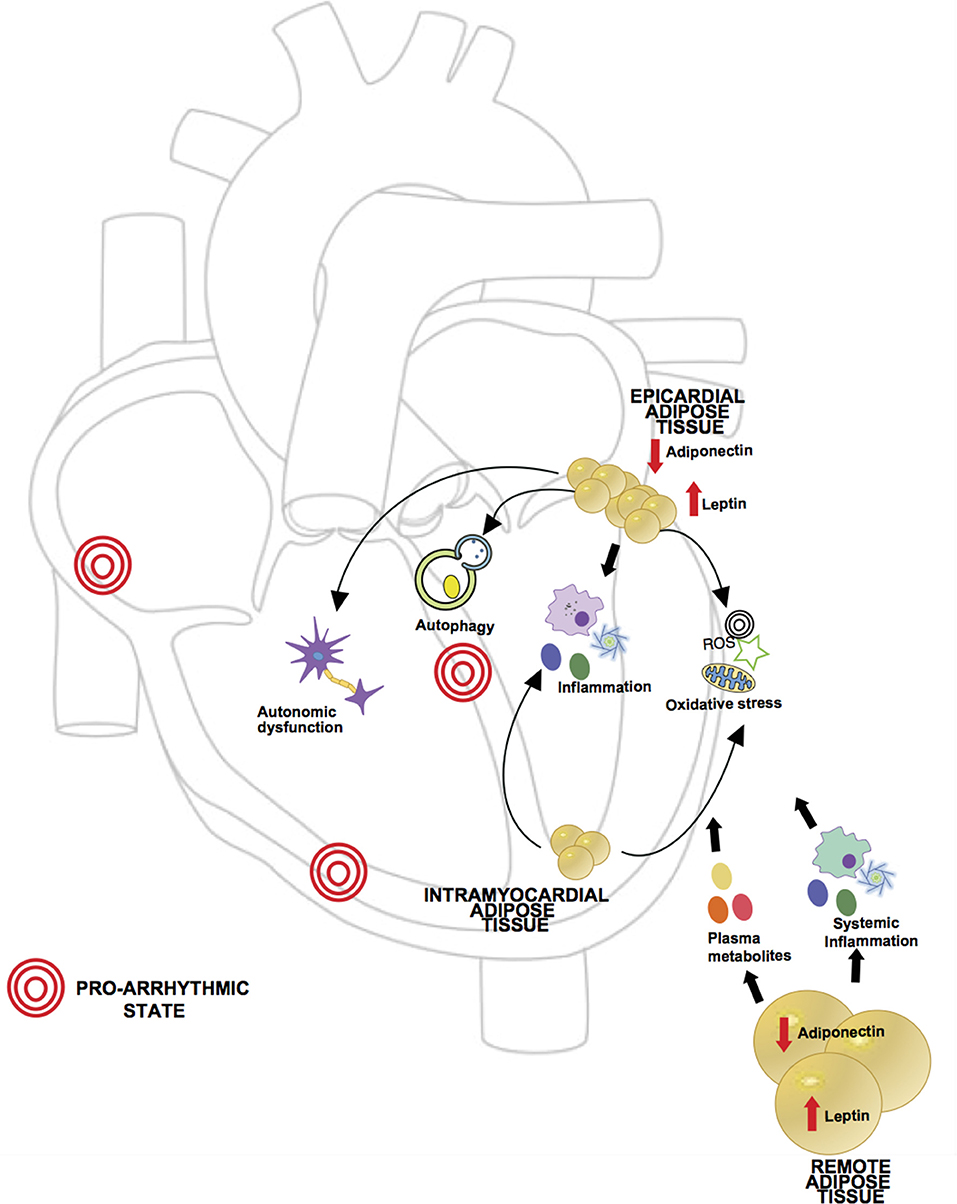
Figure 2. Proposed mechanisms of obesity in pathogenesis of arrhythmia. Obesity is characterized by a dysregulated state of adipose tissue, leading to an increase in pro-inflammatory adipokines (i.e., leptin) and decrease in anti-inflammatory adipokines (i.e., adiponectin), creating a chronic inflammatory state which has been related to arrhythmia development. Levels of circulatory metabolites are also increased such as free fatty acids and glucose leading to cardiac glucotoxicity and increased fatty acid oxidation. Given the anatomic location, local adipose tissue can exert direct effects in myocardial function. In obesity, epicardial adipose tissue (EAT) has been shown to have increased macrophage infiltration and higher levels of pro-inflammatory and pro-fibrotic cytokines. Excess lipids and hyperglycemia can induce mitochondrial dysfunction in adipocytes causing excessive production of reactive oxygen species (ROS). In setting of obesity aberrant autophagy activation in adipocytes can lead to degradation of adiponectin, contributing to the pro-inflammatory state. Also, ganglionated plexi of the autonomic nervous system are located in epicardial fat pads and in setting of obesity there may be an abnormal augmentation of autonomic tone. Intramyocardial fat affecting atrial tissue has also been described in association with supraventricular arrhythmias, likely through oxidative stress and perpetuation of inflammation.
Dietary Fat
Independent of obesity-induced cardiac structural changes mentioned earlier (i.e., biventricular enlargement/hypertrophy, diastolic/systolic dysfunction), increased dietary fat burden alone can lead to arrhythmia. For example, even a short period of HFD in gerbils can lead to radical inflammation and apoptosis causing cardiac remodeling (Sahraoui et al., 2016). Rats fed a high fat diet had an increased incidence of malignant ventricular tachyarrhythmias (Liptak et al., 2017) in the setting of induced ischemia. Furthermore, atria from rats fed high fat diet showed upregulation of transforming growth factor-β1 and matrix metalloproteinase-2 (atrial fibrosis pathway proteins), gap junction remodeling with alterations and distribution of connexin 40 (Cx40) and Cx43, broadened interstitial space, and myocyte disarray, and increased apoptotic cell death (Meng et al., 2017). In guinea pigs, HFD produces alteration of atrial cell electrophysiology favilitating the development of reentrant arrhythmias (Aromolaran et al., 2016).
In humans, hypercholesterolemia has been associated with QT prolongation, offering yet another mechanism for potential arrhythmia (el-Gamal et al., 1995). When studying the effect of palmitic, stearic, and oleic free fatty acids on sheep atrial myocytes, it was found that stearic acid disrupts t-tubular and induces inhibition of ICa−L and ITO ionic currents in sheep atrial myocytes. This could result in abbreviation (ICa−L) or a prolongation (ITO) of atrial myocyte action potential duration and thus play role in arrhythmogenesis (O'Connell et al., 2015). This also highlights that not all dietary lipid is the same. In pig ventricular myocytes, for example, dietary fish oil prevents calcium overload and reduces incidence of norepinephrine induced triggered activity (Berecki et al., 2007). Clinical trials in humans looking at the utility of fish oil in the prevention of arrhythmia in various cohorts, however have been contradictory. In the Alpha-Omega trial, low-dose EPA-DHA, or ALA did not reduce the rate of major cardiovascular events, but secondary analysis of the data showed a reduction in arrhythmias in the fish oil groups (Kromhout et al., 2010). In the SOFA trial, omega-3 poly unsaturated fatty acids from fish oil did not significantly protect against ventricular arrhythmia in patients with implantable cardioverter defibrillators (Brouwer et al., 2006).
High fat diet (HFD) has also been studied in AF. In a study using a sheep model of HFD-induced obesity, there was infiltration of the posterior left atrium by EAT associated with reduced endocardial voltage in this region compared to lean sheep (Mahajan et al., 2015). HFD was associated with biatrial endocardial remodeling, conduction abnormalities, increased expression of TGF- β1 in the left atrium and interstitial atrial fibrosis leading to increase frequency and duration of AF (Mahajan et al., 2015). In mice, HFD-induced obesity has been shown to be related to increased LA fibrosis and higher vulnerability of developing AF (AF was able to be induced in 50% of animals that were treated with HFD vs. 0% in the control group; Kondo et al., 2018). Further, studies are needed to observe the impact of diet modifications and weight loss in animals in reversing alterations in electrophysiology.
Therapeutics
In the case of obesity, a single mechanism likely does not account for the link between adipose tissue and arrhythmia. Rather, it is the activation of multiple pathways and effects of adipose tissue that make the myocardium more prone to arrhythmia. Treating this requires a multidisciplinary approach with upstream incorporation of risk factor modification (RFM) and lifestyle modifications. In a study of 1425 consecutive patients offered weight management, those with >10% weight loss had 6 fold increased likelihood of arrhythmia free survival over 5 years compared to those with less weight loss (Pathak et al., 2017). Similar data has been reported in an AF ablation cohort. In 281 consecutive patients undergoing AF ablation, patients who opted for RFM had greater reductions in weight, blood pressure and lipid profiles. In both single procedure and multiple procedure drug unassisted cases, patients opting for RFM had significant arrhythmia free survival compared to controls. On multivariate analysis, RFM was an independent predictor of arrhythmia free survival (Pathak et al., 2014). An even more provocative finding is that when RFM is implemented rigorously, achieved via face to face consultation for behavioral change as it relates to weight management and physical activity), RFM turned out to be a very cost effective measure with an increase of 0.193 quality-adjusted life years in those patients with symptomatic AF who opted for RFM compared to those who opted out. This is a cost saving of $12,094 (Pathak et al., 2017). How this relates to ventricular arrhythmia, remains to be seen but RFM should be a cornerstone in the treatment of arrhythmia, especially atrial fibrillation.
Future Directions
The relationship between obesity and the development of arrhythmias is exciting, but further work to clarify the mechanisms and possible therapeutic interventions is needed. Identification of the proarrhythmogenic mechanisms elicited by obesity will involve basic, translational and clinical research. Clinical arrhythmias may be multifactorial in nature. Thus, teasing apart the mechanisms that drive an arrhythmia in any individual patient will likely require further work.
One of the most interesting areas is the process of the whitening of EAT. It is possible that in setting of lipid overload, ROS production becomes uncontrollable leading to inflammation and whitening of EAT (Dozio et al., 2014). However, given EAT's thermogenic function where ROS production is high at baseline, there may be a transition point at which ROS production may become excessive having pathogenic effects on the heart. Targeting EAT activity and ROS production could be leveraged to treat or prevent arrhythmias.
As discussed previously, EAT affects the adjacent myocardium by both paracrine mechanisms as well as by direct infiltration. However, mechanisms of how EAT infiltrates the myocardium remains poorly understood. It seems like one of the most common characteristics found in the studies mentioned above are the inflammatory properties of the secretome from EAT in the setting of obesity that leads to myocardial fibrosis. Atrial fibrosis is a feature of AF, and activin A, a member of the TFG-β superfamily has been associated with the development of atrial fibrosis (Venteclef et al., 2015). Importantly, activin A has been shown to be upregulated by angiotensin-II in cardiomyocytes and atrial fibroblasts in non-obese mice (Wang et al., 2017). EAT from CAD patients has been shown to have higher expression of angiotensinogen than control patients. Thus, it is possible that EAT activation of the renin-angiotensin system may be a key player in the stimulation of atrial fibrosis as a substrate for AF.
Another key player to target would be TGF- β 1. As mentioned earlier, sheep models fed a HFD have higher TGF- β 1 levels in their atrium. In vitro, human atrial myofibroblast tissue treated with TGF- β 1 showed increased collagen Ia2 and fibronectin synthesis (both markers of active fibrosis) as well as increased autophagy activation possibly leading to atrial fibrosis..Thus, exploring how fat affects TGF- β 1 and potential mechanisms to inhibit this pathway may offer further treatment options.
Increased ROS production and inflammation can lead to ER stress. SERCA proteins are calcium pumps that transport Ca2+ from the cytoplasm into the ER (Kang et al., 2016). SERCA dysfunction leading to decreased or increased cytoplasmic calcium levels, has been related to ER stress and arrhythmia (Erkasap, 2007; Kang et al., 2016). Determining the factors involved in the development of ER stress and the regulation of expression of SERCA proteins in myocardium and adipose tissue in the setting of obesity may be key. These findings underline the need to better understand the pathogenic effects of obesity in arrhythmogenesis and the role of inflammation, oxidative stress, ER stress and autophagy, as well as the potential therapeutic interventions that can prevent electrophysiological alterations.
Research Models
Small animal models (such as mice or rats) are valuable for the study of cardiac physiology given the lower maintenance cost, easier handling, cost effectiveness and possibility for genetic manipulation (Milani-Nejad and Janssen, 2014). Techniques for assessing cardiac function and electrophysiology measures are well-established for rodents. Despite being the most common in vivo model used, differences between murine models and human patients can make the generalizability of the data obtained from murine experiments on action potentials, cardiac contraction, cardiomyocytes characteristics and protein expression difficult (Nerbonne, 2004; Milani-Nejad and Janssen, 2014). Specifically, for the study of arrhythmias, baseline heart rates for rodents are greater than humans, which leads to a rapid repolarization and lack of plateau phase in the action potentials of ventricular myocytes observed in large animals (Nerbonne, 2004). Guinea-pigs have a more similar action potential wave given similarities in ionic currents contributing to repolarization (Bosch et al., 1998). Rabbits have also been used for the study of cardiac diseases given that rabbit myocardium shares more similarities with the human myocardium than small rodents (Milani-Nejad and Janssen, 2014); but the action potential is still faster than humans (Szél et al., 2011). Larger animal models share more similarities with human physiology, but their maintenance and costs can be challenging. Moreover, the contractile and relaxation kinetics in larger animal models remain slightly faster than humans (Milani-Nejad and Janssen, 2014), and the ion channel distribution in the different species is unknown (Schram et al., 2002; Milani-Nejad and Janssen, 2014).
Specific rat models which available for study would include those with mutations in the calcium channel SCN5A in mice which is associated with Brugada syndrome (Milan and MacRae, 2005) and mice with human NaV1.5 variant with a mutation in the anesthetic-binding site that produces an incomplete Na+ channel inactivation (F1759A-NaV1.5), who develop spontaneous AF induction (Wan et al., 2016). How these arrhythmia prone models respond to obesity may offer further insight into the link between arrhythmia and obesity.
Possible human clinical areas of research would be rigorous quantification of different types of adipose tissue. This would allow risk stratification for hard clinical outcomes such as predicting new AF development as well as recurrence after ablation, prediction of ventricular arrhythmias after MI, risk stratification for need for implantable cardioverter defibrillator in various disease where risk is not clear (e.g., asymptomatic, gene positive, phenotype negative hypertrophic cardiomyopathy patients).
Recently human induced pluripotent stem cell derived cardiomyocytes from disease patients have been shown to be a promising method to study cardiac arrhythmias, especially in arrhythmias related to genetic mutations (Garg et al., 2018). Pluripotent stem cell derived cardiomyocytes can be used to study channelopathies by modeling genetic syndromes in an in vitro dish (Garg et al., 2018). Even though the relevance of pluripotent stem cells in arrhythmias with multifactorial etiologies (including metabolic syndrome) is limited, endothelial cells derived from HFD mice exhibit signs of endothelial dysfunction compared to regular diet mice (Gu et al., 2015). Further studies are needed to delucidate if pluripotent stem cell derived cardiomyocytes from obese animals or patients can be used to model arrhythmias.
Conclusion
There has been an epidemic level rise in obesity. Emerging evidence suggests that obesity promotes atrial and ventricular arrhythmias. However, the function of adipose tissues is multifaceted and its interplay with the heart is even more complex. Future studies examining the connection between adipose tissues and arrhythmias are urgently needed. Numerous adipose tissue depots, such as intramyocardial, local and/or remote may play integral roles in the regulation of inflammation, oxidative stress, autophagy, autonomic tone, mitophagy, propagation of aberrant signals, creation of potential re-entry circuits and cardiomyocyte death. Upon elucidation of the mechanisms by which arrhythmias are incited, it may be possible to identify high value therapeutic targets.
Author Contributions
MP and KM are co-first authors. MP, KM, JC, and JL contributed and reviewed and approved the final manuscript. All co-authors contributed and reviewed and approved the final manuscript.
Conflict of Interest Statement
The authors declare that the research was conducted in the absence of any commercial or financial relationships that could be construed as a potential conflict of interest.
References
Afshin, A., Forouzanfar, M. H., Reitsma, M. B., Sur, P., Estep, K., et al. (2017). Health effects of overweight and obesity in 195 countries over 25 Years. N. Engl. J. Med. 377, 13–27. doi: 10.1056/NEJMoa1614362
An, M., Ryu, D. R., Won Park, J., Ha Choi, J., Park, E. M., Eun Lee, K., et al. (2017). ULK1 prevents cardiac dysfunction in obesity through autophagy-meditated regulation of lipid metabolism. Cardiovasc. Res. 113, 1137–1147. doi: 10.1093/cvr/cvx064
Antonopoulos, A. S., Margaritis, M., Verheule, S., Recalde, A., Sanna, F., Herdman, L., et al. (2016). Mutual regulation of epicardial adipose tissue and myocardial redox state by PPAR-gamma/Adiponectin signalling. Circ. Res. 118, 842–855. doi: 10.1161/CIRCRESAHA.115.307856
Aromolaran, A. S., Colecraft, H. M., and Boutjdir, M. (2016). High-fat diet-dependent modulation of the delayed rectifier K(+) current in adult guinea pig atrial myocytes. Biochem. Biophys. Res. Commun. 474, 554–559. doi: 10.1016/j.bbrc.2016.04.113
Baker, A. R., Harte, A. L., Howell, N., Pritlove, D. C., Ranasinghe, A. M., da Silva, N. F., et al. (2009). Epicardial adipose tissue as a source of nuclear factor-kappaB and c-Jun N-terminal kinase mediated inflammation in patients with coronary artery disease. J. Clin. Endocrinol. Metab. 94, 261–267. doi: 10.1210/jc.2007-2579
Bambace, C., Sepe, A., Zoico, E., Telesca, M., Olioso, D., Venturi, S., et al. (2014). Inflammatory profile in subcutaneous and epicardial adipose tissue in men with and without diabetes. Heart Vessels. 29, 42–48. doi: 10.1007/s00380-012-0315-9
Bauce, B., Basso, C., Rampazzo, A., Beffagna, G., Daliento, L., Frigo, G., et al. (2005). Clinical profile of four families with arrhythmogenic right ventricular cardiomyopathy caused by dominant desmoplakin mutations. Eur. Heart J. 26, 1666–1675. doi: 10.1093/eurheartj/ehi341
Berecki, G., Den Ruijter, H. M., Verkerk, A. O., Schumacher, C. A., Baartscheer, A., Bakker, D., et al. (2007). Dietary fish oil reduces the incidence of triggered arrhythmias in pig ventricular myocytes. Heart Rhythm. 4, 1452–1460. doi: 10.1016/j.hrthm.2007.07.015
Bosch, R. F., Gaspo, R., Busch, A. E., Lang, H. J., Li, G. R., and Nattel, S. (1998). Effects of the chromanol 293B, a selective blocker of the slow, component of the delayed rectifier K+ current, on repolarization in human and guinea pig ventricular myocytes. Cardiovasc. Res. 38, 441–450. doi: 10.1016/S0008-6363(98)00021-2
Brouwer, I. A., Zock, P. L., Camm, A. J., Böcker, D., Hauer, R. N., Wever, E. F., et al. (2006). Effect of fish oil on ventricular tachyarrhythmia and death in patients with implantable cardioverter defibrillators: the Study on Omega-3 Fatty Acids and Ventricular Arrhythmia (SOFA) randomized trial. JAMA 295, 2613–2619. doi: 10.1001/jama.295.22.2613
Burgeiro, A., Fonseca, A. C., Espinoza, D., Carvalho, L., Lourenço, N., Antunes, M., et al. (2018). Proteostasis in epicardial versus subcutaneous adipose tissue in heart failure subjects with and without diabetes. Biochim. Biophys. Acta 1864(6 Pt A), 2183–2198. doi: 10.1016/j.bbadis.2018.03.025
Carnes, C. A., Chung, M. K., Nakayama, T., Nakayama, H., Baliga, R. S., Piao, S., et al. (2001). Ascorbate attenuates atrial pacing-induced peroxynitrite formation and electrical remodeling and decreases the incidence of postoperative atrial fibrillation. Circ. Res. 89, E32–E38. doi: 10.1161/hh1801.097644
Chattopadhyay, M., Khemka, V. K., Chatterjee, G., Ganguly, A., Mukhopadhyay, S., and Chakrabarti, S. (2015). Enhanced ROS production and oxidative damage in subcutaneous white adipose tissue mitochondria in obese and type 2 diabetes subjects. Mol. Cell. Biochem. 399, 95–103. doi: 10.1007/s11010-014-2236-7
Chechi, K., Voisine, P., Mathieu, P., Laplante, M., Bonnet, S., Picard, F., et al. (2017). Functional characterization of the Ucp1-associated oxidative phenotype of human epicardial adipose tissue. Sci. Rep. 7:15566. doi: 10.1038/s41598-017-15501-7
Cheng, K. H., Chu, C. S., Lee, K. T., Lin, T. H., Hsieh, C. C., Chiu, C. C., et al. (2008). Adipocytokines and proinflammatory mediators from abdominal and epicardial adipose tissue in patients with coronary artery disease. Int. J. Obes. 32, 268–274. doi: 10.1038/sj.ijo.0803726
Cherian, S., Lopaschuk, G. D., and Carvalho, E. (2012). Cellular cross-talk between epicardial adipose tissue and myocardium in relation to the pathogenesis of cardiovascular disease. Am. J. Physiol. Endocrinol. Metab. 303, E937–E949. doi: 10.1152/ajpendo.00061.2012
Chilukoti, R. K., Giese, A., Malenke, W., Homuth, G., Bukowska, A., Goette, A., et al. (2015). Atrial fibrillation and rapid acute pacing regulate adipocyte/adipositas-related gene expression in the atria. Int. J. Cardiol. 187, 604–613. doi: 10.1016/j.ijcard.2015.03.072
Chouchani, E. T., Kazak, L., Jedrychowski, M. P., Lu, G. Z., Erickson, B. K., Szpyt, J., et al. (2016). Mitochondrial ROS regulate thermogenic energy expenditure and sulfenylation of UCP1. Nature 532, 112–116. doi: 10.1038/nature17399
Chu, C. Y., Lee, W. H., Hsu, P. C., Lee, M. K., Lee, H. H., Chiu, C. A., et al. (2016). Association of increased epicardial adipose tissue thickness with adverse cardiovascular outcomes in patients with atrial fibrillation. Medicine 95:e2874. doi: 10.1097/MD.0000000000002874
Coelho, M., Oliveira, T., and Fernandes, R. (2013). Biochemistry of adipose tissue: an endocrine organ. Arch. Med. Sci. 9, 191–200. doi: 10.5114/aoms.2013.33181
Coumel, P. (1996). Autonomic influences in atrial tachyarrhythmias. J. Cardiovasc. Electrophysiol. 7, 999–1007. doi: 10.1111/j.1540-8167.1996.tb00474.x
De Coster, T., Claus, P., Kazbanov, I. V., Haemers, P., Willems, R., Sipido, K. R., et al. (2018). Arrhythmogenicity of fibro-fatty infiltrations. Sci. Rep. 8:2050. doi: 10.1038/s41598-018-20450-w
Dozio, E., Vianello, E., Briganti, S., Fink, B., Malavazos, A. E., Scognamiglio, E. T., et al. (2014). Increased reactive oxygen species production in epicardial adipose tissues from coronary artery disease patients is associated with brown-to-white adipocyte trans-differentiation. Int. J. Cardiol. 174, 413–414. doi: 10.1016/j.ijcard.2014.04.045
Ebong, I. A., Goff, D. C. Jr., Rodriguez, C. J., Chen, H., and Bertoni, A. G. (2014). Mechanisms of heart failure in obesity. Obes. Res. Clin. Pract. 8, e540–e548. doi: 10.1016/j.orcp.2013.12.005
el-Gamal, A., Gallagher, D., Nawras, A., Gandhi, P., Gomez, J., Allison, D. B., et al. (1995). Effects of obesity on QT, RR, and QTc intervals. Am. J. Cardiol. 75, 956–959. doi: 10.1016/S0002-9149(99)80700-0
Erkasap, N. (2007). SERCA in genesis of arrhythmias: what we already know and what is new? Anadolu Kardiyol. Derg. 7(Suppl. 1), 43–46.
Fallert, M. A., Mirotznik, M. S., Downing, S. W., Savage, E. B., Foster, K. R., Josephson, M. E., et al. (1993). Myocardial electrical impedance mapping of ischemic sheep hearts and healing aneurysms. Circulation 87, 199–207. doi: 10.1161/01.CIR.87.1.199
Francis, G. S. (1988). Should asymptomatic ventricular arrhythmias in patients with congestive heart failure be treated with antiarrhythmic drugs? J. Am. Coll. Cardiol. 12, 274–283. doi: 10.1016/0735-1097(88)90388-9
Furukawa, S., Fujita, T., Shimabukuro, M., Iwaki, M., Yamada, Y., Nakajima, Y., et al. (2004). Increased oxidative stress in obesity and its impact on metabolic syndrome. J. Clin. Invest. 114, 1752–1761. doi: 10.1172/JCI21625
Gaborit, B., Venteclef, N., Ancel, P., Pelloux, V., Gariboldi, V., Leprince, P., et al. (2015). Human epicardial adipose tissue has a specific transcriptomic signature depending on its anatomical peri-atrial, peri-ventricular, or peri-coronary location. Cardiovasc. Res. 108, 62–73. doi: 10.1093/cvr/cvv208
Garcia, L., Verdejo, H. E., Kuzmicic, J., Zalaquett, R., Gonzalez, S., Lavandero, S., et al. (2012). Impaired cardiac autophagy in patients developing postoperative atrial fibrillation. J. Thorac. Cardiovasc. Surg. 143, 451–459. doi: 10.1016/j.jtcvs.2011.07.056
Garg, P., Garg, V., Shrestha, R., Sanguinetti, M. C., Kamp, T. J., and Wu, J. C. (2018). Human induced pluripotent stem cell-derived cardiomyocytes as models for cardiac channelopathies: a primer for non-electrophysiologists. Circ. Res. 123, 224–243. doi: 10.1161/CIRCRESAHA.118.311209
Greulich, S., Maxhera, B., Vandenplas, G., de Wiza, D. H., Smiris, K., Mueller, H., et al. (2012). Secretory products from epicardial adipose tissue of patients with type 2 diabetes mellitus induce cardiomyocyte dysfunction. Circulation 126, 2324–2334. doi: 10.1161/CIRCULATIONAHA.111.039586
Gu, M., Mordwinkin, N. M., Kooreman, N. G., Lee, J., Wu, H., Hu, S., et al. (2015). Pravastatin reverses obesity-induced dysfunction of induced pluripotent stem cell-derived endothelial cells via a nitric oxide-dependent mechanism. Eur. Heart J. 36, 806–816. doi: 10.1093/eurheartj/ehu411
Guglielmi, V., and Sbraccia, P. (2017). Epicardial adipose tissue: at the heart of the obesity complications. Acta Diabetol. 54, 805–812. doi: 10.1007/s00592-017-1020-z
Haemers, P., Hamdi, H., Guedj, K., Suffee, N., Farahmand, P., Popovic, N., et al. (2017). Atrial fibrillation is associated with the fibrotic remodelling of adipose tissue in the subepicardium of human and sheep atria. Eur. Heart J. 38, 53–61. doi: 10.1093/eurheartj/ehv625
Halberg, N., Wernstedt-Asterholm, I., and Scherer, P. E. (2008). The adipocyte as an endocrine cell. Endocrinol. Metab. Clin. North Am. 37, 753–68, x–xi. doi: 10.1016/j.ecl.2008.07.002
Han, C. Y. (2016). Roles of reactive oxygen species on insulin resistance in adipose tissue. Diabetes Metab. J. 40, 272–279. doi: 10.4093/dmj.2016.40.4.272
Haneklaus, M., and O'Neill, L. A. (2015). NLRP3 at the interface of metabolism and inflammation. Immunol. Rev. 265, 53–62. doi: 10.1111/imr.12285
Harvey, R. D., and Belevych, A. E. (2003). Muscarinic regulation of cardiac ion channels. Br. J. Pharmacol. 139, 1074–1084. doi: 10.1038/sj.bjp.0705338
Hirata, Y., Kurobe, H., Akaike, M., Chikugo, F., Hori, T., Bando, Y., et al. (2011b). Enhanced inflammation in epicardial fat in patients with coronary artery disease. Int Heart J. 52, 139–142. doi: 10.1536/ihj.52.139
Hirata, Y., Tabata, M., Kurobe, H., Motoki, T., Akaike, M., Nishio, C., et al. (2011a). Coronary atherosclerosis is associated with macrophage polarization in epicardial adipose tissue. J. Am. Coll. Cardiol. 58, 248–255. doi: 10.1016/j.jacc.2011.01.048
Houstis, N., Rosen, E. D., and Lander, E. S. (2006). Reactive oxygen species have a causal role in multiple forms of insulin resistance. Nature 440, 944–948. doi: 10.1038/nature04634
Hu, N., and Zhang, Y. (2017). TLR4 knockout attenuated high fat diet-induced cardiac dysfunction via NF-kappaB/JNK-dependent activation of autophagy. Biochim. Biophys. Acta 1863, 2001–2011. doi: 10.1016/j.bbadis.2017.01.010
Huby, A. C., Antonova, G., Groenendyk, J., Gomez-Sanchez, C. E., Bollag, W. B., Filosa, J. A., et al. (2015). Adipocyte-derived hormone leptin is a direct regulator of aldosterone secretion, which promotes endothelial dysfunction and cardiac fibrosis. Circulation 132, 2134–2145. doi: 10.1161/CIRCULATIONAHA.115.018226
Iacobellis, G., and Barbaro, G. (2008). The double role of epicardial adipose tissue as pro- and anti-inflammatory organ. Horm. Metab. Res. 40, 442–445. doi: 10.1055/s-2008-1062724
Iacobellis, G., Corradi, D., and Sharma, A. M. (2005a). Epicardial adipose tissue: anatomic, biomolecular and clinical relationships with the heart. Nat. Clin. Pract. Cardiovasc. Med. 2, 536–543. doi: 10.1038/ncpcardio0319
Iacobellis, G., Pistilli, D., Gucciardo, M., Leonetti, F., Miraldi, F., Brancaccio, G., et al. (2005b). Adiponectin expression in human epicardial adipose tissue in vivo is lower in patients with coronary artery disease. Cytokine 29, 251–255. doi: 10.1016/j.cyto.2004.11.002
Jansen, H. J., van Essen, P., Koenen, T., Joosten, L. A., Netea, M. G., Tack, C. J., et al. (2012). Autophagy activity is up-regulated in adipose tissue of obese individuals and modulates proinflammatory cytokine expression. Endocrinology 153, 5866–5874. doi: 10.1210/en.2012-1625
Jeong, J. W., Jeong, M. H., Yun, K. H., Oh, S. K., Park, E. M., Kim, Y. K., et al. (2007). Echocardiographic epicardial fat thickness and coronary artery disease. Circ. J. 71, 536–539. doi: 10.1253/circj.71.536
Kang, S., Dahl, R., Hsieh, W., Shin, A., Zsebo, K. M., Buettner, C., et al. (2016). Small molecular allosteric activator of the sarco/endoplasmic reticulum Ca2+-ATPase (SERCA) attenuates diabetes and metabolic disorders. J. Biol. Chem. 291, 5185–5198. doi: 10.1074/jbc.M115.705012
Kim, Y. H., Lim, D. S., Lee, J. H., Shim, W. J., Ro, Y. M., Park, G. H., et al. (2003). Gene expression profiling of oxidative stress on atrial fibrillation in humans. Exp. Mol. Med. 35, 336–349. doi: 10.1038/emm.2003.45
Kondo, H., Abe, I., Gotoh, K., Fukui, A., Takanari, H., Ishii, Y., et al. (2018). Interleukin 10 treatment ameliorates high-fat diet-induced inflammatory atrial remodeling and fibrillation. Circ. Arrhythm. Electrophysiol. 11:e006040. doi: 10.1161/CIRCEP.117.006040
Kourliouros, A., Karastergiou, K., Nowell, J., Gukop, P., Tavakkoli Hosseini, M., Valencia, O., et al. (2011). Protective effect of epicardial adiponectin on atrial fibrillation following cardiac surgery. Eur. J. Cardiothorac. Surg. 39, 228–232. doi: 10.1016/j.ejcts.2010.05.006
Kromhout, D., Giltay, E. J., and Geleijnse, J. M. (2010). n-3 fatty acids and cardiovascular events after myocardial infarction. N. Engl. J. Med. 363, 2015–2026. doi: 10.1056/NEJMoa1003603
Kusayama, T., Furusho, H., Kashiwagi, H., Kato, T., Murai, H., Usui, S., et al. (2016). Inflammation of left atrial epicardial adipose tissue is associated with paroxysmal atrial fibrillation. J. Cardiol. 68, 406–411. doi: 10.1016/j.jjcc.2015.11.005
Lamounier-Zepter, V., Look, C., Alvarez, J., Christ, T., Ravens, U., Schunck, W. H., et al. (2009). Adipocyte fatty acid-binding protein suppresses cardiomyocyte contraction: a new link between obesity and heart disease. Circ. Res. 105, 326–334. doi: 10.1161/CIRCRESAHA.109.200501
Lavie, C. J., Pandey, A., Lau, D. H., Alpert, M. A., and Sanders, P. (2017). Obesity and atrial fibrillation prevalence, pathogenesis, and prognosis: effects of weight loss and exercise. J. Am. Coll. Cardiol. 70, 2022–2035. doi: 10.1016/j.jacc.2017.09.002
Linck, G., Stoeckel, M. E., Porte, A., and Petrovic, A. (1974). [Demonstration of gap junctions or nexus by lanthanum in the brown fat of the European hamster (Cricetus cricetus)]. C R Acad. Sci. Hebd. Seances Acad. Sci. D. 278, 87–89.
Liptak, B., Knezl, V., and Gasparova, Z. (2017). Metabolic disturbances induce malignant heart arrhythmias in rats. Bratisl. Lek. Listy. 118, 539–543. doi: 10.4149/BLL_2017_103
Lopaschuk, G. D., Folmes, C. D., and Stanley, W. C. (2007). Cardiac energy metabolism in obesity. Circ. Res. 101, 335–347. doi: 10.1161/CIRCRESAHA.107.150417
Mahajan, R., Lau, D. H., Brooks, A. G., Shipp, N. J., Manavis, J., Wood, J. P., et al. (2015). Electrophysiological, electroanatomical, and structural remodeling of the atria as consequences of sustained obesity. J. Am. Coll. Cardiol. 66, 1–11. doi: 10.1016/j.jacc.2015.04.058
Marchington, J. M., and Pond, C. M. (1990). Site-specific properties of pericardial and epicardial adipose tissue: the effects of insulin and high-fat feeding on lipogenesis and the incorporation of fatty acids in vitro. Int. J. Obes. 14, 1013–1022.
Mazurek, T., Kiliszek, M., Kobylecka, M., Skubisz-Głuchowska, J., Kochman, J., Filipiak, K., et al. (2014). Relation of proinflammatory activity of epicardial adipose tissue to the occurrence of atrial fibrillation. Am. J. Cardiol. 113, 1505–1508. doi: 10.1016/j.amjcard.2014.02.005
Mazurek, T., Zhang, L., Zalewski, A., Mannion, J. D., Diehl, J. T., Arafat, H., et al. (2003). Human epicardial adipose tissue is a source of inflammatory mediators. Circulation 108, 2460–2466. doi: 10.1161/01.CIR.0000099542.57313.C5
Meng, T., Cheng, G., Wei, Y., Ma, S., Jiang, Y., Wu, J., et al. (2017). Exposure to a chronic high-fat diet promotes atrial structure and gap junction remodeling in rats. Int. J. Mol. Med. 40, 217–225. doi: 10.3892/ijmm.2017.2982
Meyer, G., Czompa, A., Reboul, C., Csepanyi, E., Czegledi, A., Bak, I., et al. (2013). The cellular autophagy markers Beclin-1 and LC3B-II are increased during reperfusion in fibrillated mouse hearts. Curr. Pharm. Des. 19, 6912–6918. doi: 10.2174/138161281939131127122510
Mihm, M. J., Yu, F., Carnes, C. A., Reiser, P. J., McCarthy, P. M., Van Wagoner, D. R., et al. (2001). Impaired myofibrillar energetics and oxidative injury during human atrial fibrillation. Circulation 104, 174–180. doi: 10.1161/01.CIR.104.2.174
Milan, D. J., and MacRae, C. A. (2005). Animal models for arrhythmias. Cardiovasc. Res. 67, 426–437. doi: 10.1016/j.cardiores.2005.06.012
Milani-Nejad, N., and Janssen, P. M. (2014). Small and large animal models in cardiac contraction research: advantages and disadvantages. Pharmacol. Ther. 141, 235–249. doi: 10.1016/j.pharmthera.2013.10.007
Mizushima, N., Levine, B., Cuervo, A. M., and Klionsky, D. J. (2008). Autophagy fights disease through cellular self-digestion. Nature 451, 1069–1075. doi: 10.1038/nature06639
Morrow, J. P., Katchman, A., Son, N. H., Trent, C. M., Khan, R., Shiomi, T., et al. (2011). Mice with cardiac overexpression of peroxisome proliferator-activated receptor gamma have impaired repolarization and spontaneous fatal ventricular arrhythmias. Circulation 124, 2812–2821. doi: 10.1161/CIRCULATIONAHA.111.056309
Nademanee, K., McKenzie, J., Kosar, E., Schwab, M., Sunsaneewitayakul, B., Vasavakul, T., et al. (2004). A new approach for catheter ablation of atrial fibrillation: mapping of the electrophysiologic substrate. J. Am. Coll. Cardiol. 43, 2044–2053. doi: 10.1016/j.jacc.2003.12.054
Nagashima, K., Okumura, Y., Watanabe, I., Nakai, T., Ohkubo, K., Kofune, M., et al. (2012). Does location of epicardial adipose tissue correspond to endocardial high dominant frequency or complex fractionated atrial electrogram sites during atrial fibrillation? Circ. Arrhythm. Electrophysiol. 5, 676–683. doi: 10.1161/CIRCEP.112.971200
Nakanishi, K., Fukuda, S., Tanaka, A., Otsuka, K., Sakamoto, M., Taguchi, H., et al. (2012). Peri-atrial epicardial adipose tissue is associated with new-onset nonvalvular atrial fibrillation. Circ. J. 76, 2748–2754. doi: 10.1253/circj.CJ-12-0637
Nerbonne, J. M. (2004). Studying cardiac arrhythmias in the mouse–a reasonable model for probing mechanisms? Trends Cardiovasc. Med. 14, 83–93. doi: 10.1016/j.tcm.2003.12.006
Neuman, R. B., Bloom, H. L., Shukrullah, I., Darrow, L. A., Kleinbaum, D., Jones, D. P., et al. (2007). Oxidative stress markers are associated with persistent atrial fibrillation. Clin. Chem. 53, 1652–1657. doi: 10.1373/clinchem.2006.083923
Nishimura, S., Manabe, I., Nagasaki, M., Eto, K., Yamashita, H., Ohsugi, M., et al. (2009). CD8+ effector T cells contribute to macrophage recruitment and adipose tissue inflammation in obesity. Nat. Med. 15, 914–920. doi: 10.1038/nm.1964
O'Connell, R. P., Musa, H., Gomez, M. S., Avula, U. M., Herron, T. J., Kalifa, J., et al. (2015). Free fatty acid effects on the atrial myocardium: membrane ionic currents are remodeled by the disruption of T-tubular architecture. PLoS ONE 10:e0133052. doi: 10.1371/journal.pone.0133052
Okla, M., Wang, W., Kang, I., Pashaj, A., Carr, T., and Chung, S. (2015). Activation of toll-like receptor 4 (TLR4) attenuates adaptive thermogenesis via endoplasmic reticulum stress. J. Biol. Chem. 290, 26476–26490. doi: 10.1074/jbc.M115.677724
Ozaydin, M., Peker, O., Erdogan, D., Kapan, S., Turker, Y., Varol, E., et al. (2008). N-acetylcysteine for the prevention of postoperative atrial fibrillation: a prospective, randomized, placebo-controlled pilot study. Eur. Heart J. 29, 625–631. doi: 10.1093/eurheartj/ehn011
Pabon, M. A., Ma, K. C., and Choi, A. M. (2016). Autophagy and obesity-related lung disease. Am. J. Respi.r Cell Mol. Biol. 54, 636–646. doi: 10.1165/rcmb.2016-0045PS
Packer, M. (2018). Epicardial adipose tissue may mediate deleterious effects of obesity and inflammation on the myocardium. J. Am. Coll. Cardiol. 71, 2360–2372. doi: 10.1016/j.jacc.2018.03.509
Pathak, R. K., Evans, M., Middeldorp, M. E., Mahajan, R., Mehta, A. B., Meredith, M., et al. (2017). Cost-effectiveness and clinical effectiveness of the risk factor management clinic in atrial fibrillation: the CENT study. JACC Clin. Electrophysiol. 3, 436–447. doi: 10.1016/j.jacep.2016.12.015
Pathak, R. K., Middeldorp, M. E., Lau, D. H., Mehta, A. B., Mahajan, R., Twomey, D., et al. (2014). Aggressive risk factor reduction study for atrial fibrillation and implications for the outcome of ablation: the ARREST-AF cohort study. J. Am. Coll. Cardiol. 64, 2222–2231. doi: 10.1016/j.jacc.2014.09.028
Pouliopoulos, J., Chik, W. W., Kanthan, A., Sivagangabalan, G., Barry, M. A., Fahmy, P. N., et al. (2013). Intramyocardial adiposity after myocardial infarction: new implications of a substrate for ventricular tachycardia. Circulation 128, 2296–2308. doi: 10.1161/CIRCULATIONAHA.113.002238
Rosen, E. D., and Spiegelman, B. M. (2014). What we talk about when we talk about fat. Cell 156, 20–44. doi: 10.1016/j.cell.2013.12.012
Rosenshtraukh, L. V., Zaitsev, A. V., Fast, V. G., Pertsov, A. M., and Krinsky, V. I. (1989). Vagally induced block and delayed conduction as a mechanism for circus movement tachycardia in frog atria. Circ. Res. 64, 213–226. doi: 10.1161/01.RES.64.2.213
Rudy, Y., Plonsey, R., and Liebman, J. (1979). The effects of variations in conductivity and geometrical parameters on the electrocardiogram, using an eccentric spheres model. Circ. Res. 44, 104–111. doi: 10.1161/01.RES.44.1.104
Sacks, H. S., Fain, J. N., Holman, B., Cheema, P., Chary, A., Parks, F., et al. (2009). Uncoupling protein-1 and related messenger ribonucleic acids in human epicardial and other adipose tissues: epicardial fat functioning as brown fat. J. Clin. Endocrinol. Metab. 94, 3611–3615. doi: 10.1210/jc.2009-0571
Sahraoui, A., Dewachter, C., de Medina, G., Naeije, R., Aouichat Bouguerra, S., and Dewachter, L. (2016). Myocardial structural and biological anomalies induced by high fat diet in psammomys obesus gerbils. PLoS ONE 11:e0148117. doi: 10.1371/journal.pone.0148117
Salgado-Somoza, A., Teijeira-Fernández, E., Fernández, A. L., González-Juanatey, J. R., and Eiras, S. (2010). Proteomic analysis of epicardial and subcutaneous adipose tissue reveals differences in proteins involved in oxidative stress. Am. J. Physiol. Heart Circ. Physiol. 299, H202–H209. doi: 10.1152/ajpheart.00120.2010
Samanta, R., Pouliopoulos, J., Thiagalingam, A., and Kovoor, P. (2016). Role of adipose tissue in the pathogenesis of cardiac arrhythmias. Heart Rhythm. 13, 311–320. doi: 10.1016/j.hrthm.2015.08.016
Schram, G., Pourrier, M., Melnyk, P., and Nattel, S. (2002). Differential distribution of cardiac ion channel expression as a basis for regional specialization in electrical function. Circ. Res. 90, 939–950. doi: 10.1161/01.RES.0000018627.89528.6F
Song, D. K., Hong, Y. S., Lee, H., Oh, J. Y., Sung, Y. A., and Kim, Y. (2015). Increased epicardial adipose tissue thickness in type 2 diabetes mellitus and obesity. Diabetes Metab. J. 39, 405–413. doi: 10.4093/dmj.2015.39.5.405
Suffee, N., Moore-Morris, T., Farahmand, P., Rücker-Martin, C., Dilanian, G., Fradet, M., et al. (2017). Atrial natriuretic peptide regulates adipose tissue accumulation in adult atria. Proc. Natl. Acad. Sci. U.S.A. 114, E771–E80. doi: 10.1073/pnas.1610968114
Szél, T., Koncz, I., Jost, N., Baczkó, I., Husti, Z., Virág L, L., et al. (2011). Class I/B antiarrhythmic property of ranolazine, a novel antianginal agent, in dog and human cardiac preparations. Eur. J. Pharmacol. 662, 31–39. doi: 10.1016/j.ejphar.2011.04.042
Tam, W. C., Lin, Y. K., Chan, W. P., Huang, J. H., Hsieh, M. H., Chen, S. A., et al. (2016). Pericardial fat is associated with the risk of ventricular arrhythmia in asian patients. Circ. J. 80, 1726–1733. doi: 10.1253/circj.CJ-16-0047
Tsao, H. M., Hu, W. C., Wu, M. H., Tai, C. T., Lin, Y. J., Chang, S. L., et al. (2011). Quantitative analysis of quantity and distribution of epicardial adipose tissue surrounding the left atrium in patients with atrial fibrillation and effect of recurrence after ablation. Am. J. Cardiol. 107, 1498–1503. doi: 10.1016/j.amjcard.2011.01.027
Venteclef, N., Guglielmi, V., Balse, E., Gaborit, B., Cotillard, A., Atassi, F., et al. (2015). Human epicardial adipose tissue induces fibrosis of the atrial myocardium through the secretion of adipo-fibrokines. Eur. Heart J. 36, 795–805a. doi: 10.1093/eurheartj/eht099
Verheule, S., Sato, T., Everett, T., Engle, S. K., Otten, D., Rubart-von der Lohe, M., et al. (2004). Increased vulnerability to atrial fibrillation in transgenic mice with selective atrial fibrosis caused by overexpression of TGF-beta1. Circ. Res. 94, 1458–1465. doi: 10.1161/01.RES.0000129579.59664.9d
Vianello, E., Dozio, E., Arnaboldi, F., Marazzi, M. G., Martinelli, C., Lamont, J., et al. (2016). Epicardial adipocyte hypertrophy: association with M1-polarization and toll-like receptor pathways in coronary artery disease patients. Nutr. Metab. Cardiovasc. Dis. 26, 246–253. doi: 10.1016/j.numecd.2015.12.005
Wan, E., Abrams, J., Weinberg, R. L., Katchman, A. N., Bayne, J., Zakharov, S. I., et al. (2016). Aberrant sodium influx causes cardiomyopathy and atrial fibrillation in mice. J. Clin. Invest. 126, 112–122. doi: 10.1172/JCI84669
Wang, Q., Yu, Y., Zhang, P., Chen, Y., Li, C., Chen, J., et al. (2017). The crucial role of activin A/ALK4 pathway in the pathogenesis of Ang-II-induced atrial fibrosis and vulnerability to atrial fibrillation. Basic Res. Cardiol. 112:47. doi: 10.1007/s00395-017-0634-1
Wiersma, M., Meijering, R. A. M., Qi, X. Y., Zhang, D., Liu, T., Hoogstra-Berends, F., et al. (2017). Endoplasmic reticulum stress is associated with autophagy and cardiomyocyte remodeling in experimental and human atrial fibrillation. J. Am. Heart Assoc. 6:10. doi: 10.1161/JAHA.117.006458
Wong, C. X., Ganesan, A. N., and Selvanayagam, J. B. (2017). Epicardial fat and atrial fibrillation: current evidence, potential mechanisms, clinical implications, and future directions. Eur. Heart J. 38, 1294–1302. doi: 10.1093/eurheartj/ehw045
Wu, C. K., Tsai, H. Y., Su, M. Y., Wu, Y. F., Hwang, J. J., Tseng, W. Y., et al. (2015). Pericardial fat is associated with ventricular tachyarrhythmia and mortality in patients with systolic heart failure. Atherosclerosis 241, 607–614. doi: 10.1016/j.atherosclerosis.2015.05.025
Xu, X., Hua, Y., Nair, S., Zhang, Y., and Ren, J. (2013). Akt2 knockout preserves cardiac function in high-fat diet-induced obesity by rescuing cardiac autophagosome maturation. J. Mol. Cell Biol. 5, 61–63. doi: 10.1093/jmcb/mjs055
Yang, R., Sikka, G., Larson, J., Watts, V. L., Niu, X., Ellis, C. L., et al. (2011). Restoring leptin signaling reduces hyperlipidemia and improves vascular stiffness induced by chronic intermittent hypoxia. Am. J. Physiol. Heart Circ. Physiol. 300, H1467–H1476. doi: 10.1152/ajpheart.00604.2009
Yao, C., Veleva, T., Scott, L. Jr., Cao, S., Li, L., Chen, G., et al. (2018). Enhanced cardiomyocyte NLRP3 inflammasome signaling promotes atrial fibrillation. Circulation 138, 2227–2242. doi: 10.1161/CIRCULATIONAHA.118.035202
Yeop Han, C., Kargi, A. Y., Omer, M., Chan, C. K., Wabitsch, M., O'Brien, K. D., et al. (2010). Differential effect of saturated and unsaturated free fatty acids on the generation of monocyte adhesion and chemotactic factors by adipocytes: dissociation of adipocyte hypertrophy from inflammation. Diabetes 59, 386–396. doi: 10.2337/db09-0925
Yu, L., Wang, Y., Zhou, X., Huang, B., Wang, M., Li, X., et al. (2018). Leptin injection into the left stellate ganglion augments ischemia-related ventricular arrhythmias via sympathetic nerve activation. Heart Rhythm. 15, 597–606. doi: 10.1016/j.hrthm.2017.12.003
Yuan, Y., Zhao, J., Yan, S., Wang, D., Zhang, S., Yun, F., et al. (2014). Autophagy: a potential novel mechanistic contributor to atrial fibrillation. Int. J. Cardiol. 172, 492–494. doi: 10.1016/j.ijcard.2014.01.027
Zghaib, T., Ipek, E. G., Zahid, S., Balouch, M. A., Misra, S., Ashikaga, H., et al. (2016). Association of left atrial epicardial adipose tissue with electrogram bipolar voltage and fractionation: electrophysiologic substrates for atrial fibrillation. Heart Rhythm. 13, 2333–2339. doi: 10.1016/j.hrthm.2016.08.030
Zhang, Y., Sowers, J. R., and Ren, J. (2018). Targeting autophagy in obesity: from pathophysiology to management. Nat. Rev. Endocrinol. 14, 356–376. doi: 10.1038/s41574-018-0009-1
Zhou, L., and Liu, F. (2010). Autophagy: roles in obesity-induced ER stress and adiponectin downregulation in adipocytes. Autophagy 6, 1196–1197. doi: 10.4161/auto.6.8.13478
Zhou, Q., Zhang, L., Wang, K., Xu, X., Ji, M., Zhang, F., et al. (2014). Effect of interconnection between cervical vagus trunk, epicardial fat pad on sinus node function, and atrial fibrillation. Pacing Clin. Electrophysiol. 37, 356–363. doi: 10.1111/pace.12265
Zhou, Y., Wei, Y., Wang, L., Wang, X., Du, X., Sun, Z., et al. (2011). Decreased adiponectin and increased inflammation expression in epicardial adipose tissue in coronary artery disease. Cardiovasc. Diabetol. 10:2. doi: 10.1186/1475-2840-10-2
Keywords: arrhythmia, obesity, adipocyte, fat, atrial fibrillation, epicardial adipose tissue, intramyocardial adipose
Citation: Pabon MA, Manocha K, Cheung JW and Lo JC (2018) Linking Arrhythmias and Adipocytes: Insights, Mechanisms, and Future Directions. Front. Physiol. 9:1752. doi: 10.3389/fphys.2018.01752
Received: 10 August 2018; Accepted: 20 November 2018;
Published: 05 December 2018.
Edited by:
Carol Ann Remme, University of Amsterdam, NetherlandsReviewed by:
Marina Cerrone, School of Medicine, New York University, United StatesAdemuyiwa S. Aromolaran, Veterans Affairs New York Harbor Healthcare System, United States
Copyright © 2018 Pabon, Manocha, Cheung and Lo. This is an open-access article distributed under the terms of the Creative Commons Attribution License (CC BY). The use, distribution or reproduction in other forums is permitted, provided the original author(s) and the copyright owner(s) are credited and that the original publication in this journal is cited, in accordance with accepted academic practice. No use, distribution or reproduction is permitted which does not comply with these terms.
*Correspondence: James C. Lo, amxvQG1lZC5jb3JuZWxsLmVkdQ==