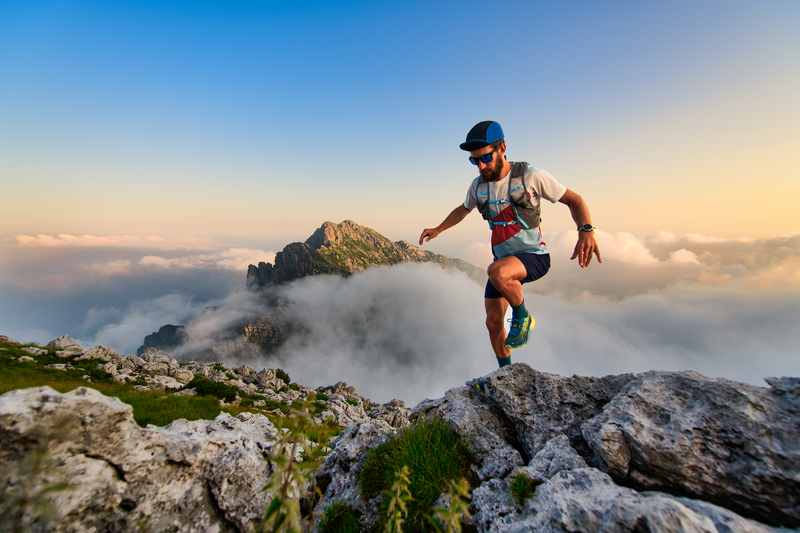
94% of researchers rate our articles as excellent or good
Learn more about the work of our research integrity team to safeguard the quality of each article we publish.
Find out more
ORIGINAL RESEARCH article
Front. Physiol. , 21 November 2018
Sec. Exercise Physiology
Volume 9 - 2018 | https://doi.org/10.3389/fphys.2018.01657
Exercise has been shown to induce cerebrovascular adaptations. However, the underlying temporal dynamics are poorly understood, and regional variation in the vascular response to exercise has been observed in the large cerebral arteries. Here, we sought to measure the cerebrovascular effects of a single 20-min session of moderate-intensity exercise in the one hour period immediately following exercise cessation. We employed transcranial Doppler (TCD) ultrasonography to measure cerebral blood flow velocity (CBFV) in the middle cerebral artery (MCAv) and posterior cerebral artery (PCAv) before, during, and following exercise. Additionally, we simultaneously measured cerebral blood flow (CBF) in the internal carotid artery (ICA) and vertebral artery (VA) before and up to one hour following exercise cessation using Duplex ultrasound. A hypercapnia challenge was used before and after exercise to examine exercise-induced changes in cerebrovascular reactivity (CVR). We found that MCAv and PCAv were significantly elevated during exercise (p = 4.81 × 10-5 and 2.40 × 10-4, respectively). A general linear model revealed that these changes were largely explained by the partial pressure of end-tidal CO2 and not a direct vascular effect of exercise. After exercise cessation, there was no effect of exercise on CBFV or CVR in the intracranial or extracranial arteries (all p > 0.05). Taken together, these data confirm that CBF is rapidly and uniformly regulated following exercise cessation in healthy young males.
There is robust evidence that exercise is beneficial to vascular health. Randomized controlled trials indicate that exercise is as effective as drug interventions for reducing coronary heart disease mortality, treatment of heart failure and prevention of diabetes, and is more beneficial than drug treatment in stroke rehabilitation (Naci and Ioannidis, 2013). However, the underpinning mechanisms associated with this low-cost and accessible therapeutic intervention are not well understood.
Long-term exercise has been shown to induce angiogenesis, neurogenesis, and synaptic plasticity in the human and rodent brain (Isaacs et al., 1992; Cotman and Berchtold, 2002; van Praag et al., 2005; Black et al., 2008) which manifests as increased cerebral perfusion and metabolism (Nishijima and Soya, 2006; Kinni et al., 2011). Improving our understanding of how exercise promotes such cerebral vascular adaptations is crucial, given the increasingly recognized importance of vascular contributions to cognitive impairment and dementia (Gorelick et al., 2011) and the potential to optimize exercise to target the aging and diseased brain. The demand acute exercise places on the cerebrovasculature may be relevant to chronic adaptations as the acute demand may be a stimulus for adaptation and may reveal individual differences in the propensity for chronic adaptations.
The ability of the cerebral vasculature to adapt to changing metabolic demands depends on how readily it can alter its resistance, i.e., CVR. CBF is modulated by the PaCO2, which has a major influence on arteriolar diameter (Thomas et al., 1989; Ide and Secher, 2000; Ratanakorn et al., 2001). During exercise, both CBF and CVR to CO2 are increased (Rasmussen et al., 2006; Ainslie et al., 2008; Ogoh et al., 2008). Changes in CBF are intensity dependent, with CBFV in the MCA increasing linearly with exercise intensity until approximately 60–70% of maximal aerobic power, or VO2 max (Ide and Secher, 2000; Ogoh and Ainslie, 2009), before returning to baseline resting levels. Performing high-intensity exercise results in hyperventilation-induced hypocapnia, which subsequently causes cerebral vasoconstriction (Ogoh and Ainslie, 2009) and the observed return of CBFV to baseline values. Recent evidence suggests there is regional variation in the blood flow response during exercise (Sato et al., 2011; Smith et al., 2016, 2017; Washio et al., 2017). Similar to findings in the MCA, Sato et al. (2011) found that CBF in the ICA increased from rest to exercise at 60% of maximal aerobic power yet returned toward resting levels at 80% of maximal aerobic power. Crucially, the change in ICA CBF during graded exercise (40, 60, and 80% aerobic power) was correlated with the partial pressure of end-tidal CO2 (PETCO2) (Sato et al., 2011). In contrast, in the external carotid, common carotid and VA, CBF increased proportionally with workload (Ainslie et al., 2008), suggesting a non-uniform effect of exercise across vascular territories.
Current understanding of cerebrovascular function in the period immediately following exercise cessation is limited, with previous studies showing equivocal results depending on the duration, intensity, and mode of exercise. For example, during the early recovery period directly after exercise, when blood pressure and HR are decreasing rapidly toward baseline levels, conflicting evidence exists for the stability of cerebral autoregulation (e.g., Koch et al., 2005; Ogoh et al., 2005, 2007; Murrell et al., 2007, 2009; Bailey et al., 2011) – the process of maintaining adequate and stable CBF during changes in blood pressure. Koch et al. (2005) observed alterations in cerebral autoregulation (decreased signal coherence and gain between blood pressure and CBFV) along with increased expired CO2 immediately following exhaustive resistance exercise. In contrast, cerebral autoregulation at the mean phase of the MCA velocity profile was found to be stable during the first 10 min following cessation of mild, moderate and heavy cycling (Ogoh et al., 2007). Beyond this early recovery phase, Willie et al. (2013) found CBFV was maintained in the MCA at 10-, 30-, and 60-min after aerobic exercise cessation, despite the presence of hypotension and exercise-induced hypocapnia. A lack of change in dynamic cerebral autoregulation change suggests that disturbances in vascular mechanisms are transient and recover within 10 min in the MCA, specifically. No studies to date have examined exercise recovery in cerebral arteries other than the MCA, despite evidence for regional variability during exercise (Sato et al., 2011).
In the recovery period following exercise, changes in CO2 have been shown up to 60-min following exercise cessation (Willie et al., 2013). Thus, it is necessary to understand how exercise-induced changes in arterial CO2 impact the cerebral vasculature during the post-exercise recovery period. There is evidence that CVR is affected by long-term exercise. Using TCD sonography, CVR was increased in the MCA following 12-weeks of exercise training in both young and older adults (Murrell et al., 2013), whilst in a separate TCD study of older adults, CVR was related to aerobic fitness in older adults (Barnes et al., 2013). In contrast and paradoxically, a blunted CVR response was observed in aged lifelong endurance runners compared to sedentary age-matched controls using magnetic resonance imaging. A proposed explanation for this blunting effect is the increased lifetime exposure to high levels of CO2 (i.e., exercise-induced hypercapnia) resulted in desensitization of the cerebrovasculature to CO2 stimulation. CVR has not been examined acutely during the post-exercise recovery phase.
In this study, we therefore sought to examine the immediate effects of a single session of moderate-intensity exercise on cerebrovascular function. To explore the hypothesis that regional variability in CBF would be observed post-exercise, we simultaneously measured CBFV in the MCA and PCA using transcranial Doppler ultrasonography (TCD) and blood flow in the ICA and vertebral arteries (VA) using Duplex ultrasound for up to 65 min after exercise cessation. To measure the dynamic vessel properties, CVR to hypercapnia was assessed based on the hypothesis that CVR would be increased during the post-exercise recovery period compared to baseline.
The study design is detailed in Figure 1.
FIGURE 1. Study Design. Measures were recorded up to 65-min after exercise cessation. X-US, Doppler ultrasound to assess extracranial arteries; TCD, transcranial Doppler; X-US +CO2, Doppler ultrasound to assess cerebrovascular reactivity in the extracranial arteries with a hypercapnia challenge. Baseline ultrasound (X-US) was recorded for 5 continuous minutes after a minimum of 15-min supine rest. Cycling was performed on an upright ergometer.
Healthy male participants (n = 18; 26 ± 6.2 years old, range = 18–37 years) were recruited; demographic features are shown in Table 1. The inclusion criteria were: 18–55 years old, non-smoking, non-hypertensive and free from known neurological, respiratory and cardiovascular diseases. Participants were screened for contraindications to exercise and respiratory gas modulation. All participants were asked to refrain from vigorous physical activity, caffeine, and alcohol consumption for at least 12 h prior to experimentation. All subjects gave written informed consent in accordance with the Declaration of Helsinki. The protocol was prior approved by the clinical research ethics board at the University of British Columbia. Participants were instructed to lie supine for at least 15 min prior to beginning the study protocol.
Participants underwent 20-min of moderate-intensity aerobic cycling on an upright ergometer (Lode Ergometer, Lode, Groningen, Netherlands). To ensure a moderate-intensity aerobic intervention, a prescribed intensity of 50–70% of the maximal heart rate reserve (HRR) was determined using the Karvonen formula (American College of Sports Medicine et al., 2012) with maximum HR calculated as (220-age).
Participants first rested on the ergometer for two-minutes before commencing a two-minute warm up at 25 watts. Following this, the resistance was adjusted to target the HRR, and was manually adjusted at two-minute intervals to ensure HR was maintained with the HRR zone. At two-minute intervals, whole blood capillary lactate concentration was collected from the left earlobe, blood pressure was measured and self-report ratings of perceived exertion were recorded using a 10-point Borg scale (Borg, 1974).
Cerebral blood flow velocity in the right MCA and left PCA (P1 segment) were measured using a 2-MHz pulsed TCD ultrasound system (PMD150B, Spencer Technologies, Redmond, WA, United States) using insonation techniques described in Willie et al. (2012), and identical to previous studies (Skow et al., 2013; Tymko et al., 2015). Mean CBFV was calculated from the envelope of the velocity tracings for both MCA and PCA.
Continuous diameter and blood flow recordings in the right ICA and left VA were obtained using a 10 MHz multifrequency linear array probe attached to a high-resolution ultrasound machine (Terason 3000, Teratech, Burlington, MA, United States) and using insonation techniques described in Thomas et al. (2015). Simultaneous measurements of blood vessel diameter and CBFV were recorded over a minimum of ten cardiac cycles with care taken to ensure probe position was stable. The sample volume was positioned in the center of the vessel and adjusted to cover the width of the vessel diameter.
All extracranial vascular images were stored for offline analysis using a custom-designed edge-detection and wall-tracking software described in depth elsewhere (Black et al., 2008; Willie et al., 2012). Mean blood flow was determined as half the time averaged maximum velocity multiplied by the cross-sectional lumen area, in line with Willie et al. (Evans, 1985; Willie et al., 2012; Thomas et al., 2015).
Extracranial blood velocity was not measured during exercise due to motion during upright cycling.
Cardiorespiratory measurements were sampled at 200 Hz using an analog-to-digital converter (Powerlab; AD Instruments; Colorado Springs, CO, United States) and analyzed offline (ADI LabChart version 7.1; AD Instruments, Colorado Springs, CO, United States). Participants breathed through a mouthpiece with nose clip, and respiratory gasses were recorded by a calibrated gas analyzer (ADI ML206; ADInstruments), with daily calibration to known gas concentrations performed prior to each test. PETCO2 was calculated using peak analysis and was corrected for the daily atmospheric pressure.
Heart rate was measured by a three-lead electrocardiogram (ECG; ADI bioamp ML132; AD Instruments). Beat-to-beat mean arterial blood pressure (MAP), cardiac output and stroke volume were measured using finger photoplethysmography (Finometer Pro, Finapres Medical Systems, Amsterdam, NL), which was calibrated using the return-to-flow function prior to baseline data collection. Blood pressure accuracy was confirmed with manual sphygmomanometer.
End-tidal gasses were controlled using a dynamic end-tidal forcing system previously described by Tymko et al. (2015). PETO2, PETCO2, inspiratory and expiratory tidal volume, breathing frequency and minute ventilation were determined for each breath in real time using custom software (Labview 13.0, National Instruments, Austin, TX, United States). To determine baseline end-tidal values, participants breathed room air until a steady-state was achieved (defined as ± 1 mmHg PETCO2 and respiratory gasses being controlled and stable for at least three consecutive breaths).
Dynamic end-tidal forcing was utilized to maintain PETCO2 and PETO2 at the baseline value on an individual basis for a minimum of one minute, during which, imaging of the extracranial arteries was conducted. Upon completion of the baseline stage, PETO2 remained unchanged while PETCO2 was elevated to +7 mmHg PETCO2. After reaching steady-state PETCO2, imaging of the extracranial arteries was conducted for two minutes. CBFV in the right MCA and left PCA were recorded continuously as described above.
Middle cerebral artery, PCAv and PETCO2 were recorded continuously and statistically analyzed using a two-level approach in MATLAB R2012a (MathWorks, Natick, MA, United States). Time series were subjected to robust outlier detection, followed by temporal smoothing. A general linear model (GLM) was first fit to the individual subject data time series with the change in posture (supine to upright during transition to cycle ergometer) and PETCO2 included in the model. Next, a one-sample t-test was performed on the regression coefficients, to assess the independent effect of (a) exercise on MCAv and PCAv, and (b) the effect of PETCO2 on MCAv and PCAv.
For the extracranial arteries, 30-s averaging of the beat-by-beat data (velocity, diameter) was conducted and blood flow was calculated. Statistical analyses were performed on the data averaged across each recording period (Figure 1). Absolute values were assessed using a one-way repeated-measures analysis of variance (ANOVA; 8 levels: baseline, 8-, 15-, 20-, 40-, 45-, 60-, and 65-min post exercise) followed by pairwise post hoc comparisons, with a Bonferroni-adjustment for multiple comparisons. Relative change was assessed with a one-sample t-test and corrected for multiple comparisons using the false discovery rate at p < 0.05 (Benjamini and Hochberg, 1995). The assumption of sphericity was tested and where violated, the Greenhouse-Geisser adjusted values are reported.
Cerebrovascular reactivity was calculated in both absolute and relative terms (normalized to steady-state baseline) as the slopes of the linear regression of CBFV on PETCO2 and % Δ CBFV on PETCO2, respectively, quantified on an individual basis. A one-way repeated-measures ANOVA was used to measure the effect of exercise. For relative measures of CVR, regional specificity was assessed for the intracranial and extracranial arteries separately (e.g., MCA and PCA as a within-subject variable, and ICA and VA as a within-subject variable) due to different acquisition methods.
Results across the time series of the intervention are shown in Figure 2. Participants worked at an average 58.4 ± 1.2% of their maximal HR reserve. Average ratings of perceived exertion were 4 ± 0 in the legs, and 3 ± 0 for breathing, indicating “moderate” to “somewhat hard” exertion. Average blood lactate levels were 3.07 ± 0.57 mmoL (resting levels = 1.00 ± 0.1 mmoL). On average, participants cycled at a workload of 144 ± 9 watts, at a speed of 77 ± 2 revolutions per minute. MAP, HR and PETCO2 were significantly elevated throughout the exercise intervention compared to the upright rest period on the ergometer (see Table 2 and Supplementary Figure S1).
FIGURE 2. Change from baseline in MCA Vmean (red line), PCA Vmean (green line) and end-tidal CO2 (PETCO2, purple line), before, during and after 20-min of moderate-intensity aerobic exercise. Black arrow indicates start of a hypercapnia (HC) challenge. Dotted vertical lines indicates discontinuity in the data, gray vertical columns indicate where the participant transferred from supine position to/from the cycle ergometer. Black horizontal line represents supine baseline. Data shown are mean ± S.E.M.
Table 2 shows the cardiorespiratory response during and after moderate-intensity exercise, averaged across each recording period. HR following exercise cessation was significantly elevated compared to supine baseline (Greenhouse-Geisser corrected F2.6,45.0= 10.37, p < 0.001, ε = 0.38), with post hoc tests showing HR remained significantly elevated for 45-min after exercise cessation (p < 0.05 Bonferroni-adjusted). Whereas MAP was significantly elevated during exercise, in the post exercise period (measured from 8-min post exercise cessation), MAP was not significantly changed compared to supine baseline (F7,91 = 0.44, p > 0.05). Compared to supine rest, PETCO2 was significantly reduced during the post-exercise period (Greenhouse-Geisser corrected F2.1,32.9 = 4.80, p = 0.014, ε = 0.29), with post hoc tests showing participants were initially hypocapnic following exercise cessation (p = 0.012), and returned to resting levels of PETCO2 15-min after exercise cessation (p = 0.079).
Figure 2 shows the absolute change in MCAV, PCAV, and PETCO2 from baseline, including the hypercapnia challenge. On average across the 20-min exercise period, MCAV and PCAV were elevated by 17.2 ± 4.9% and 20.7 ± 4.4%, respectively, compared to the upright rest period. A t-test performed on the regression coefficients found that before accounting for PETCO2, exercise had a significant effect on MCAV (t16 = 5.50, p = 4.81 × 10-5, 95% CI [3.00 6.76]) and PCAV (t16 = 4.70, p = 2.40 × 10-4, 95% CI [1.37 3.62]). After including PETCO2 in the regression model and accounting for postural changes, PETCO2 was found to have a significant effect on MCAV (t16 = 14.94, p = 8.14 × 10-11, 95% CI = [1.66 2.21]) and PCAV (t16 = 10.27, p = 1.89 × 10-8, 95% CI = [0.77 1.17]), whilst the effect of exercise on CBFV was no longer significant (MCA t16 = -0.49, p = 0.632, 95% CI [-2.51 1.57]; PCA t 16 = -0.39, p = 0.702, 95% CI [-1.20 0.83]).
When comparing the post-exercise period to baseline, there was no effect of exercise on MCAV before (t16 = 1.60, p = 0.13, 95% CI = [-7.38 1.04]), or after (t16 = -0.24, p = 0.82, 95% CI = [-4.95 3.88]) including PETCO2 in the model. Similarly, there was no effect of exercise on PCAV before (t16 = 0.44, p = 0.67, 95% CI = [-3.00 4.57]), or after (t16 = 1.21, p = 0.24, 95% CI = [-1.63 5.99]) including PETCO2 in the model.
After accounting for postural change, PETCO2 was found to account for 38.5 ± 16.9% of the variability in MCAv and 24.7 ± 16.9% of the variability in PCAv overall.
Absolute flow and CBFV values are shown in Table 2 for the extracranial feeding arteries (ICA, VA). There was a significant effect of time on absolute ICA velocity (F7,105 = 2.16, p = 0.043) with post hoc comparisons showing a significant difference between ICA velocity at 8 min post-exercise and 65-min post exercise (p = 0.048), however, this difference was not significant after accounting for the change in PETCO2 (p = 0.069). Similarly, there was a main effect of time of absolute ICA blood flow (F7,105 = 2.67, p = 0.014); however, there were no pairwise differences after correcting for multiple comparisons (all p > 0.05). There was no effect of time on absolute VA velocity (p = 0.84) and no effect of time on relative ICA or VA flow (all p > 0.05) and on ICA and VA diameter (p > 0.05).
During hypercapnia, both the absolute and relative rate of change in MCAv per mmHg PETCO2 were not significantly different post-exercise (2.33 ± 0.17 cm/s, 3.65 ± 0.25% per mmHg, respectively) compared to baseline (2.44 ± 0.18 cm/s, 3.79 ± 0.27% per mmHg, respectively, all p > 0.05). Similarly, there was no statistical difference in CVR to CO2 for PCAv before (1.34 ± 0.12 cm/s, 3.41 ± 0.28% per mmHg) and after exercise (1.57 ± 0.16 cm/s, 3.92 ± 0.33% per mmHg, p > 0.05). The absolute and relative change in blood flow were also not significantly different pre- and post-exercise in the ICA (Baseline = 11.57 ± 3.64 ml/min, 4.60 ± 1.45% per mmHg; post-exercise = 7.51 ± 1.22 ml/min, 4.03 ± 0.88% per mmHg, p = 0.26 and 0.67, respectively) and in the VA (Baseline = 4.23 ± 0.89 ml/min, 5.47 ± 1.11%; post-exercise = 4.36 ± 1.59 ml/min, 5.33 ± 1.58%, p = 0.94 and 0.94, respectively) per mmHg PETCO2.
In terms of regional differences in CO2 reactivity, there was no difference in relative CVR between the MCA and PCA (p = 0.81) and no interaction between vessel (MCA vs. PCA) and exercise effects (p = 0.23). Similarly, there was no difference in relative CVR for the ICA and VA (p = 0.88) and no interaction between vessel (ICA vs. VA) and exercise effects (p = 0.54).
This is the first known study to investigate cerebrovascular function in the middle and posterior cerebral arteries (MCAV and PCAV, respectively) and in the extracranial cerebral arteries (ICA and VA) during the post-exercise recovery period. Based on evidence for regional variability in the vascular response during exercise (Sato et al., 2011), we hypothesized that regional variability in CBF would be observed post-exercise. We found that during exercise, significant changes in MCAV and PCAV were observed before changes in PETCO2 were accounted for. However, after accounting for changes in PETCO2 we instead found that CBFV in the MCA, PCA, ICA, and VA were stable compared to baseline, with changes in CBF being transient and returning to baseline rapidly and uniformly after exercise cessation in all measured vessels. Similar results have been reported previously in the MCA (Willie et al., 2013). Critically, PETCO2 was measured and included in the statistical analyses as a regressor in this study, which allowed us to show for the first time that observed changes in CBFV during exercise were completely accounted for by exercise-induced changes in PETCO2; despite an initial period of hypocapnia post exercise, CBF and CBFV remained stable compared to baseline.
We directly manipulated CO2 levels through the use of a hypercapnic challenge to measure CVR, which has not previously been examined acutely during the post-exercise recovery phase. We hypothesized that CVR to hypercapnia would be increased post-exercise compared to baseline, based on evidence of increased CVR during exercise (Ogoh et al., 2008) and following exercise training (Murrell et al., 2013). In contrast to our hypothesis, we found that CVR in the large cerebral vessels was stable during the post-exercise recovery period. The lack of a post-exercise effect was observed under normotensive conditions after 20-min of moderate intensity aerobic cycling. The duration of the exercise session was selected to be a feasible time period for future therapeutic studies with clinical and aged groups; however, this duration and intensity may account for the results observed. Previous work using TCD to measure the effect of exercise-induced syncope after four hours of prolonged exercise reported changes in MCA velocity and CVR (Murrell et al., 2013), whereas 40-min of similar-intensity exercise was also found to produce no alterations in cerebral autoregulation in the MCA at 10, 30-, and 60-min after exercise cessation under pharmacologically-induced hypotensive conditions (10 mmHg in blood pressure) (Willie et al., 2013). Taken together, this suggests that the duration of exercise influences the post-exercise recovery response. For shorter exercise durations and in the absence of syncope, disturbances in cerebral autoregulation (Koch et al., 2005) are transient and recover by post 10 min in large arteries.
The results of the current study are notably restricted to a cohort of healthy normotensive adults aged under 40 years old, whilst the effect of age on exercise-related cerebrovascular function remains equivocal to date. During exercise, age effects in mean CBF velocity in the MCA have been reported (Heckmann et al., 2003; Marsden et al., 2012; Fisher et al., 2013; Flück et al., 2014) along with enhanced cerebral vasoconstriction at moderate-to-high exercise workloads specifically in older groups (Ogoh et al., 2011; Fisher et al., 2013). In contrast, other studies have suggested a more marked response in young cohorts, for example, during maximal exercise, older adults were found to have a blunted hyperventilation response resulting in less hypocapnia-induced cerebral vasoconstriction (Marsden et al., 2012), whilst cerebral autoregulatory capacity was found to be retained but delayed in response to ergometer stress in healthy normotensive elderly subjects compared with healthy young subjects (Heckmann et al., 2003). In contrast, the chronic, or long-term effects of exercise appear to be less affected by age, with a training-induced elevation in CVR observed in both young and older cohorts (Murrell et al., 2013), along with a consistent elevation in MCAv in in endurance-trained men compared to sedentary men irrespective of age (Ainslie et al., 2008). Methodologically, the validity of the equation commonly used to estimate maximal HR and as a basis for prescribing exercise programs has been shown to underestimate maximal HR in older adults (Tanaka et al., 2001), which may contribute to the difficulty in making comparisons across the lifespan and is an important consideration when evaluating observed age effects.
A strength of this study is the combination of Duplex ultrasound with TCD sonography to examine both CBF velocity and diameter in the large cerebral arteries with real-time resolution. Most previous exercise studies have solely relied on TCD methods (Murrell et al., 2013; Willie et al., 2013), however, TCD only gives a reliable index of CBF if the diameter of the insonated artery is constant. Exercise alters PaCO2 and increases arterial pressure by sympathetic activation, and previous work has shown that CBF velocity derived from TCD underestimates CBF during modest hypercapnia and hypocapnia (Coverdale et al., 2014). Moreover, a recent study employing magnetic resonance imaging at high spatial resolution showed a 2% decrease in MCA cross-sectional area during rhythmic handgrip exercise (Verbree et al., 2017), suggesting that diameter constancy cannot be assumed during exercise, and thus represents a confound for TCD exercise studies. Here, we measured diameter in the ICA and VA simultaneously at baseline and during the post-exercise recovery period, and found that along with CBF velocity, diameter did not change during the post-exercise recovery period.
In order to reduce between-subject variability in autonomic regulation (Rossy and Thayer, 1998; Kuo et al., 1999; Lutfi and Sukkar, 2011), only male participants were recruited in the current study. Whereas there is no evidence to date to suggest sex differences occur in the cerebrovascular response to exercise, sex differences have been shown in resting cerebral haemodynamics (Tarumi et al., 2014) and in cerebrovascular CO2 reactivity (Kastrup et al., 1998), and studies with the statistical power required to examine sex differences are warranted.
A methodological consideration in this study is the prescription of moderate-intensity exercise with a range of 50–70% of the maximal HRR which may have introduced unnecessary inter-subject variability in the results. An additional consideration is the use of end-tidal CO2 (PETCO2) as a statistical regressor across the time course. PETCO2 was used as a non-invasive estimate of PaCO2 to avoid the invasive procedure of sampling arterial blood. However, whereas PETCO2 is a good index of PaCO2 during resting conditions, PETCO2 becomes higher than PaCO2 when metabolic CO2 production and ventilation are increased during exercise and therefore PaCO2 may be overestimated during the exercising period (Dubois et al., 1952; Jones et al., 1966, 1979). Given that the primary focus of this study was on changes occurring during the post-exercise recovery period rather than during exercise, and that results obtained during exercise replicate previous findings (Ogoh and Ainslie, 2009), this methodological issue is not likely to affect the interpretation of the results.
Cardiorespiratory recovery following moderate-intensity exercise does not map onto cerebrovascular recovery. For the first time, we show that CBF in the large cerebral arteries recovers rapidly and uniformly after cessation from moderate intensity exercise and remains stable during the post-exercise recovery period. Similarly, we found no change in CVR approximately 25-min after exercise cessation. Future work is required to ascertain if cerebrovascular recovery is similarly efficient in aged populations, which is of particular relevance given the importance of vascular contributions to dementia and the potential for exercise as a therapeutic for cognitive aging.
The raw data supporting the conclusions of this manuscript will be made available by the authors, without undue reservation, to any qualified researcher.
JS, AH, DN-F, MT, KM, and PA contributed toward the research project organization and execution, and reviewed the manuscript. JS, KM, and PA additionally contributed toward the research project conception, statistical analysis design and review. JW contributed toward the statistical analysis design, execution and along with manuscript review. KW contributed toward the research execution.
This work was supported by the Wellcome Trust (Cardiff University ISSF: 105613/Z/14/Z) and the Waterloo Foundation. KM was funded through a Wellcome Trust Senior Research Fellowship [WT200804].
The authors declare that the research was conducted in the absence of any commercial or financial relationships that could be construed as a potential conflict of interest.
The Supplementary Material for this article can be found online at: https://www.frontiersin.org/articles/10.3389/fphys.2018.01657/full#supplementary-material
CBF, cerebral blood flow; CBFV, cerebral blood flow velocity; CVR, cerebrovascular reactivity; HR, heart rate; ICA, internal carotid artery; MAP, mean arterial pressure; MCA, middle cerebral artery; PaCO2, Partial pressure of arterial carbon dioxide; PCA, posterior cerebral artery; PCO2, partial pressure of carbon dioxide; PEtCO2, partial pressure of end-tidal respiratory carbon dioxide; TCD, transcranial doppler; VA, vertebral artery.
Ainslie, P. N., Cotter, J. D., George, K. P., Lucas, S., Murrell, C., Shave, R., et al. (2008). Elevation in cerebral blood flow velocity with aerobic fitness throughout healthy human ageing. J. Physiol. 586, 4005–4010. doi: 10.1113/jphysiol.2008.158279
American College of Sports Medicine Tharrett, S. J., and Peterson, J. A. (2012). ACSM’s Health/Fitness Facility Standards and Guidelines. Champaign, IL: Human Kinetics.
Bailey, D. M., Evans, K. A., McEneny, J., Young, I. S., Hullin, D. A., James, P. E., et al. (2011). Exercise-induced oxidative-nitrosative stress is associated with impaired dynamic cerebral autoregulation and blood-brain barrier leakage. Exp. Physiol. 96, 1196–1207. doi: 10.1113/expphysiol.2011.060178
Barnes, J. N., Taylor, J. L., Kluck, B. N., Johnson, C. P., and Joyner, M. J. (2013). Cerebrovascular reactivity is associated with maximal aerobic capacity in healthy older adults. J. Appl. Physiol. 114, 1383–1387. doi: 10.1152/japplphysiol.01258.2012
Benjamini, Y., and Hochberg, Y. (1995). Controlling the false discovery rate: a practical and powerful approach to multiple testing. J. R. Stat. Soc. Ser. B 57, 289–300.
Black, M. A., Cable, N. T., Thijssen, D. H. J., and Green, D. J. (2008). Importance of measuring the time course of flow-mediated dilatation in humans. Hypertension 51, 203–210. doi: 10.1161/HYPERTENSIONAHA.107.101014
Borg, G. A. (1974). Perceived exertion. Exerc. Sport Sci. Rev. 2, 131–153. doi: 10.1249/00003677-197400020-00006
Cotman, C. W., and Berchtold, N. C. (2002). Exercise: a behavioral intervention to enhance brain health and plasticity. Trends Neurosci. 25, 295–301. doi: 10.1016/S0166-2236(02)02143-4
Coverdale, N. S., Gati, J. S., Opalevych, O., Perrotta, A., and Shoemaker, J. K. (2014). Cerebral blood flow velocity underestimates cerebral blood flow during modest hypercapnia and hypocapnia. J. Appl. Physiol. 117, 1090–1096. doi: 10.1152/japplphysiol.00285.2014
Dubois, A. B., Britt, A. G., and Fenn, W. O. (1952). Alveolar CO2 during the respiratory cycle. J. Appl. Physiol. 4, 535–548. doi: 10.1152/jappl.1952.4.7.535
Evans, D. H. (1985). On the measurement of the mean velocity of blood flow over the cardiac cycle using Doppler ultrasound. Ultrasound Med. Biol. 11, 735–741. doi: 10.1016/0301-5629(85)90107-3
Fisher, J. P., Hartwich, D., Seifert, T., Olesen, N. D., McNulty, C. L., Nielsen, H. B., et al. (2013). Cerebral perfusion, oxygenation and metabolism during exercise in young and elderly individuals. J. Physiol. 591, 1859–1870. doi: 10.1113/jphysiol.2012.244905
Flück, D., Braz, I. D., Keiser, S., Hüppin, F., Haider, T., Hilty, M. P., et al. (2014). Age, aerobic fitness, and cerebral perfusion during exercise: role of carbon dioxide. Am. J. Physiol. Heart Circ. Physiol. 307, H515–H523. doi: 10.1152/ajpheart.00177.2014
Gorelick, P. B., Scuteri, A., Black, S. E., Decarli, C., Greenberg, S. M., Iadecola, C., et al. (2011). Vascular contributions to cognitive impairment and dementia: a statement for healthcare professionals from the American heart association/American stroke association. Stroke 42, 2672–2713. doi: 10.1161/STR.0b013e3182299496
Heckmann, J. G., Brown, C. M., Cheregi, M., Hilz, M. J., and Neundörfer, B. (2003). Delayed cerebrovascular autoregulatory response to ergometer exercise in normotensive elderly humans. Cerebrovasc. Dis. 16, 423–429. doi: 10.1159/000072567
Ide, K., and Secher, N. H. (2000). Cerebral blood flow and metabolism during exercise. Prog. Neurobiol. 61, 397–414. doi: 10.1016/S0301-0082(99)00057-X
Isaacs, K. R., Anderson, B. J., Alcantara, A. A., Black, J. E., and Greenough, W. T. (1992). Exercise and the brain: angiogenesis in the adult rat cerebellum after vigorous physical activity and motor skill learning. J. Cereb. Blood Flow Metab. 12, 110–119. doi: 10.1038/jcbfm.1992.14
Jones, N. L., McHardy, G. J., Naimark, A., and Campbell, E. J. (1966). Physiological dead space and alveolar-arterial gas pressure differences during exercise. Clin. Sci. 31, 19–29.
Jones, N. L., Robertson, D. G., Kane, J. W., and Grobertson, D. (1979). Difference between end-tidal and arterial PC02 in exercise. J. Appl. Physiol. Respir. Environ. Exerc. Physiol. 47, 954–960. doi: 10.1152/jappl.1979.47.5.954
Kastrup, A., Dichgans, J., Niemeier, M., and Schabet, M. (1998). Changes of cerebrovascular CO2 reactivity during normal aging. Stroke 29, 1311–1314. doi: 10.1161/01.STR.29.7.1311
Kinni, H., Guo, M., Ding, J. Y., Konakondla, S., Dornbos, D. III, Tran, R., et al. (2011). Cerebral metabolism after forced or voluntary physical exercise. Brain Res. 1388, 48–55. doi: 10.1016/j.brainres.2011.02.076
Koch, A., Ivers, M., Gehrt, A., Schnoor, P., Rump, A., and Rieckert, H. (2005). Cerebral autoregulation is temporarily disturbed in the early recovery phase after dynamic resistance exercise. Clin. Auton. Res. 15, 83–91. doi: 10.1007/s10286-005-0249-8
Kuo, T. B., Lin, T., Yang, C. C., Li, C. L., Chen, C. F., and Chou, P. (1999). Effect of aging on gender differences in neural control of heart rate. Am. J. Physiol. 277, H2233–H2239. doi: 10.1152/ajpheart.1999.277.6.H2233
Lutfi, M. F., and Sukkar, M. Y. (2011). The effect of gender on heart rate variability in asthmatic and normal healthy adults. Int. J. Health Sci. 5, 146–154.
Marsden, K. R., Haykowsky, M. J., Smirl, J. D., Jones, H., Nelson, M. D., Altamirano-Diaz, L. A., et al. (2012). Aging blunts hyperventilation-induced hypocapnia and reduction in cerebral blood flow velocity during maximal exercise. Age 34, 725–735. doi: 10.1007/s11357-011-9258-9
Murrell, C., Cotter, J. D., George, K., Shave, R., Wilson, L., Thomas, K., et al. (2009). Influence of age on syncope following prolonged exercise: differential responses but similar orthostatic intolerance. J. Physiol. 587, 5959–5969. doi: 10.1113/jphysiol.2009.179549
Murrell, C., Wilson, L., Cotter, J. D., Lucas, S., Ogoh, S., George, K., et al. (2007). Alterations in autonomic function and cerebral hemodynamics to orthostatic challenge following a mountain marathon. J. Appl. Physiol. 103, 88–96. doi: 10.1152/japplphysiol.01396.2006
Murrell, C. J., Cotter, J. D., Thomas, K. N., Lucas, S. J., Williams, M. J., and Ainslie, P. N. (2013). Cerebral blood flow and cerebrovascular reactivity at rest and during sub-maximal exercise: effect of age and 12-week exercise training. Age 35, 905–920. doi: 10.1007/s11357-012-9414-x
Naci, H., and Ioannidis, J. P. A. (2013). Comparative effectiveness of exercise and drug interventions on mortality outcomes: metaepidemiological study. BMJ 347:f5577. doi: 10.1136/bmj.f5577
Nishijima, T., and Soya, H. (2006). Evidence of functional hyperemia in the rat hippocampus during mild treadmill running. Neurosci. Res. 54, 186–191. doi: 10.1016/j.neures.2005.11.005
Ogoh, S., and Ainslie, P. N. (2009). Regulatory mechanisms of cerebral blood flow during exercise. Exerc. Sport Sci. Rev. 37, 123–129. doi: 10.1097/JES.0b013e3181aa64d7
Ogoh, S., Dalsgaard, M. K., Yoshiga, C. C., Dawson, E. A., Keller, D. M., Raven, P. B., et al. (2005). Dynamic cerebral autoregulation during exhaustive exercise in humans. Am. J. Physiol. Circ. Physiol. 288, H1461–H1467. doi: 10.1152/ajpheart.00948.2004
Ogoh, S., Fisher, J. P., Purkayastha, S., Dawson, E. A., Fadel, P. J., White, M. J., et al. (2007). Regulation of middle cerebral artery blood velocity during recovery from dynamic exercise in humans. J. Appl. Physiol. 102, 713–721. doi: 10.1152/japplphysiol.00801.2006
Ogoh, S., Fisher, J. P., Young, C. N., and Fadel, P. J. (2011). Impact of age on critical closing pressure of the cerebral circulation during dynamic exercise in humans. Exp. Physiol. 96, 417–425. doi: 10.1113/expphysiol.2010.055871
Ogoh, S., Hayashi, N., Inagaki, M., Ainslie, P. N., and Miyamoto, T. (2008). Interaction between the ventilatory and cerebrovascular responses to hypo- and hypercapnia at rest and during exercise. J. Physiol. 586, 4327–4338. doi: 10.1113/jphysiol.2008.157073
Rasmussen, P., Stie, H., Nielsen, B., and Nybo, L. (2006). Enhanced cerebral CO2 reactivity during strenuous exercise in man. Eur. J. Appl. Physiol. 96, 299–304. doi: 10.1007/s00421-005-0079-3
Ratanakorn, D., Greenberg, J. P., Meads, D. B., and Tegeler, C. H. (2001). Middle cerebral artery flow velocity correlates with common carotid artery volume flow rate after CO2 inhalation. J. Neuroimaging 11, 401–405. doi: 10.1111/j.1552-6569.2001.tb00069.x
Rossy, L. A., and Thayer, J. F. (1998). Fitness and gender-related differences in heart period variability. Psychosom. Med. 60, 773–781. doi: 10.1097/00006842-199811000-00022
Sato, K., Ogoh, S., Hirasawa, A., Oue, A., and Sadamoto, T. (2011). The distribution of blood flow in the carotid and vertebral arteries during dynamic exercise in humans. J. Physiol. 589, 2847–2856. doi: 10.1113/jphysiol.2010.204461
Skow, R. J., MacKay, C. M., Tymko, M. M., Willie, C. K., Smith, K. J., Ainslie, P. N., et al. (2013). Differential cerebrovascular CO2 reactivity in anterior and posterior cerebral circulations. Respir. Physiol. Neurobiol. 189, 76–86. doi: 10.1016/j.resp.2013.05.036
Smith, K. J., Hoiland, R. L., Grove, R., McKirdy, H., Naylor, L., Ainslie, P. N., et al. (2017). Matched increases in cerebral artery shear stress, irrespective of stimulus, induce similar changes in extra-cranial arterial diameter in humans. J. Cereb. Blood Flow Metab. doi: 10.1177/0271678X17739220 [Epub ahead of print].
Smith, K. J., Wildfong, K. W., Hoiland, R. L., Harper, M., Lewis, N. C., Pool, A., et al. (2016). Role of CO2 in the cerebral hyperemic response to incremental normoxic and hyperoxic exercise. J. Appl. Physiol. 120, 843–854. doi: 10.1152/japplphysiol.00490.2015
Tanaka, H., Monahan, K. D., and Seals, D. R. (2001). Age-predicted maximal heart rate revisited. J. Am. Coll. Cardiol. 37, 153–156. doi: 10.1016/S0735-1097(00)01054-8
Tarumi, T., Ayaz Khan, M., Liu, J., Tseng, B. Y., Parker, R., Riley, J., et al. (2014). Cerebral hemodynamics in normal aging: central artery stiffness, wave reflection, and pressure pulsatility. J. Cereb. Blood Flow Metab. 3444, 971–978. doi: 10.1038/jcbfm.2014.44
Thomas, K. N., Lewis, N. C. S., Hill, B. G., and Ainslie, P. N. (2015). Technical recommendations for the use of carotid duplex ultrasound for the assessment of extracranial blood flow. Am. J. Physiol. Regul. Integr. Comp. Physiol. 309, R707–R720. doi: 10.1152/ajpregu.00211.2015
Thomas, S. N., Schroeder, T., Secher, N. H., and Mitchell, J. H. (1989). Cerebral blood flow during submaximal and maximal dynamic exercise in humans. J. Appl. Physiol. 67, 744–748. doi: 10.1152/jappl.1989.67.2.744
Tymko, M. M., Ainslie, P. N., MacLeod, D. B., Willie, C. K., and Foster, G. E. (2015). End tidal-to-arterial CO2 and O2 gas gradients at low- and high-altitude during dynamic end-tidal forcing. Am. J. Physiol. Regul. Integr. Comp. Physiol. 308, R895–R906. doi: 10.1152/ajpregu.00425.2014
van Praag, H., Shubert, T., Zhao, C., and Gage, F. H. (2005). Exercise enhances learning and hippocampal neurogenesis in aged mice. J. Neurosci. 25, 8680–8685. doi: 10.1523/JNEUROSCI.1731-05.2005
Verbree, J., Bronzwaer, A., van Buchem, M. A., Daemen, M., van Lieshout, J. J., and van Osch, M. (2017). Middle cerebral artery diameter changes during rhythmic handgrip exercise in humans. J. Cereb. Blood Flow Metab. 37, 2921–2927. doi: 10.1177/0271678X16679419
Washio, T., Sasaki, H., and Ogoh, S. (2017). Transcranial Doppler-determined change in posterior cerebral artery blood flow velocity does not reflect vertebral artery blood flow during exercise. Am. J. Physiol. Hear. Circ. Physiol. 312, H827–H831. doi: 10.1152/ajpheart.00676.2016
Willie, C. K., Ainslie, P. N., Taylor, C. E., Eves, N. D., and Tzeng, Y.-C. (2013). Maintained cerebrovascular function during post-exercise hypotension. Eur. J. Appl. Physiol. 113, 1597–1604. doi: 10.1007/s00421-012-2578-3
Keywords: PETCO2, cerebrovascular reactivity, cerebral blood flow, exercise, haemodynamics, cerebral plasticity
Citation: Steventon JJ, Hansen AB, Whittaker JR, Wildfong KW, Nowak-Flück D, Tymko MM, Murphy K and Ainslie PN (2018) Cerebrovascular Function in the Large Arteries Is Maintained Following Moderate Intensity Exercise. Front. Physiol. 9:1657. doi: 10.3389/fphys.2018.01657
Received: 02 August 2018; Accepted: 02 November 2018;
Published: 21 November 2018.
Edited by:
Hideaki Soya, University of Tsukuba, JapanReviewed by:
David Andrew Low, Liverpool John Moores University, United KingdomCopyright © 2018 Steventon, Hansen, Whittaker, Wildfong, Nowak-Flück, Tymko, Murphy and Ainslie. This is an open-access article distributed under the terms of the Creative Commons Attribution License (CC BY). The use, distribution or reproduction in other forums is permitted, provided the original author(s) and the copyright owner(s) are credited and that the original publication in this journal is cited, in accordance with accepted academic practice. No use, distribution or reproduction is permitted which does not comply with these terms.
*Correspondence: Jessica J. Steventon, c3RldmVudG9uampAY2FyZGlmZi5hYy51aw==
Disclaimer: All claims expressed in this article are solely those of the authors and do not necessarily represent those of their affiliated organizations, or those of the publisher, the editors and the reviewers. Any product that may be evaluated in this article or claim that may be made by its manufacturer is not guaranteed or endorsed by the publisher.
Research integrity at Frontiers
Learn more about the work of our research integrity team to safeguard the quality of each article we publish.