- 1Department of Biochemistry, Medical, Pharmaceutical and Toxicological Chemistry, Krasnoyarsk State Medical University named after Prof. V.F. Voino-Yasenetsky, Krasnoyarsk, Russia
- 2Research Institute of Molecular Medicine and Pathobiochemistry, Krasnoyarsk State Medical University named after Prof. V.F. Voino-Yasenetsky, Krasnoyarsk, Russia
- 3Department of Pediatrics, Krasnoyarsk State Medical University named after Prof. V.F. Voino-Yasenetsky, Krasnoyarsk, Russia
- 4Department of Medical and Biological Physics, Krasnoyarsk State Medical University named after Prof. V.F. Voino-Yasenetsky, Krasnoyarsk, Russia
Adipose tissue is recognized as an important organ with metabolic, regulatory, and plastic roles. Adipose tissue-derived stem cells (ASCs) with self-renewal properties localize in the stromal vascular fraction (SVF) being present in a vascular niche, thereby, contributing to local regulation of angiogenesis and vessel remodeling. In the past decades, ASCs have attracted much attention from biologists and bioengineers, particularly, because of their multilineage differentiation potential, strong proliferation, and migration abilities in vitro and high resistance to oxidative stress and senescence. Current data suggest that the SVF serves as an important source of endothelial progenitors, endothelial cells, and pericytes, thereby, contributing to vessel remodeling and growth. In addition, ASCs demonstrate intriguing metabolic and interlineage plasticity, which makes them good candidates for creating regenerative therapeutic protocols, in vitro tissue models and microphysiological systems, and tissue-on-chip devices for diagnostic and regeneration-supporting purposes. This review covers recent achievements in understanding the metabolic activity within the SVF niches (lactate and NAD+ metabolism), which is critical for maintaining the pool of ASCs, and discloses their pro-angiogenic potential, particularly, in the complex therapy of cardiovascular and cerebrovascular diseases.
Adipose Tissue-Derived Stem Cells: Origin, Expression Patterns, and Niche Requirements
At present, adipose tissue is being recognized as an important organ with metabolic and regulatory roles and high regenerative potential. Adipose tissue responds to (patho)physiological stimuli such as fasting and meals (white adipose tissue) and hypothermia (brown adipose tissue) (Jokinen et al., 2017). The metabolic profile of mature adipocytes consists of reactions of lipogenesis, lipolysis, glycolysis, and oxidative phosphorylation and is controlled generally by the number and activity of mitochondria (Coelho et al., 2013).
Metabolically active brown adipose tissue is found as depots in newborns (in perivascular and periorgan visceral areas) as well as in adults (in cervical, supraclavicular, mediastinal, paravertebral, and suprarenal regions) (Silva et al., 2014). Moreover, adult perivascular adipose tissue (PVAT) remains the characteristic of both white and brown fat and its metabolic activity might be critical for controlling vascular tone, vessels remodeling, angiogenesis, and thermogenesis. Particularly, PVAT-derived adiponectin activates BKCa channels in smooth muscle cells (SMCs) resulting in vasodilation (Lynch et al., 2013). Aging-associated vascular dysfunction is coupled to aberrant browning of PVAT and local adenosine production (Kong et al., 2018), reduced PVAT NO production, and altered vasodilation as evident in the offspring of obese rats (Zaborska et al., 2016). Thus, it is not surprising that aberrant metabolic activity of adult PVAT results in deregulation of vasomotor mechanisms due to imbalanced production of vasoactive substances acting at neighboring endothelial and SMCs. It leads to the development of attenuated vasodilation as it was shown recently in aged spontaneous hypertensive rats (SHRs) with specific mitochondrial deficits. Particularly, Kong et al. (2018) found that there was significantly lowered expression of uncoupling protein 1 (UCP1) and peroxisome proliferator-activated receptor γ (PPARγ) in PVAT obtained from a 16-week-old SHR compared to an 8-week-old SHR, with the corresponding decline in the vasodilative effect and adenosine production in PVAT in the 16-week-old SHR. However, basal levels of UCP1 and PPARγ expression in PVAT of SHR were higher than in the control group, which was associated with smaller adipocyte size and lower lipid content (Kong et al., 2018).
Adipogenesis in the white adipose tissue requires proliferation and differentiation of adipose-derived mesenchymal stem cells (ADMSCs) that localize along blood capillaries (Fu et al., 2014). Adipose tissue-derived stem cells (ASCs) (ADMSCs) attract much attention from biologists and bioengineers, particularly because of the potential of ASCs to differentiate into a huge number of cells (i.e., cells of osteogenic, chondrogenic, white adipogenic, and brown adipogenic lineages, cardiomyocytes, cardiac pacemakerlike cells, SMCs, endothelial cells, neurons, etc.) (Zavan et al., 2010; Nagata et al., 2016; Sun et al., 2018). Attention should be paid to the observations that the clonogenic ability of ASCs seems to be reduced significantly in obesity and metabolic syndrome (Silva et al., 2015; Oliva-Olivera et al., 2017). Thus, the clonogenic potential of ASCs is affected by systemic and local factors, i.e., by microenvironmental alterations caused by deregulated metabolism in the adipose tissue or by systemic alterations caused by cytokines, toxins, and hormones.
In addition to ASCs/ADMSCs, other cell populations contribute to adipogenesis: adipose progenitors and preadipocytes. As it was summarized by Berry et al. (2015) from several in vivo studies, early adipocyte progenitors (APs) express CD24, CD29, CD34, Sca-1, and PDGFR2 and are negative in the expression of CD45 and CD31, whereas preadipocytes do not express CD24.
The ASCs with self-renewal properties localize in the stromal vascular fraction (SVF) being present in a vascular niche, demonstrate region-specific expression profile, and express mural cell markers such as platelet-derived growth factor receptor (PDGFRβ), neural/glial antigen NG2 as well as CD24 and PPARγ (Berry et al., 2013; Rezai Rad et al., 2017; Tran et al., 2018). Being cultured in vitro or assessed in vivo, ASCs display a spindle-shaped morphology, lack the intracellular lipid droplets in contrast to mature adipocytes, and express mesenchymal markers, i.e., CD90, CD105, CD73, CD44, and CD166 (Sullivan et al., 2015; Frese et al., 2016). Slight expression of other proteins, i.e., β-III-tubulin, VEGF, PDGFRβ, etc., was registered in ASCs (Zemelko et al., 2014; Mildmay-White and Khan, 2017). In sum, a recent systematic review revealed that the following markers are attributed to ASCs phenotype: CD90, CD44, CD29, CD105, CD13, CD73, CD166, CD10, CD49e, and CD59 (positive markers), while CD31, CD45, CD14, CD11b, CD34, CD19, CD56, and CD146 (negative markers) (Mildmay-White and Khan, 2017). However, attention should be paid while selecting an appropriate marker for studies in humans and rodents. As an example, mouse ASCs have been found to be positive for mesenchymal markers CD90 and CD105, Nanog, SSEA-1, CD106, and VEGFR-1 and negative for hematopoietic markers CD34 and CD45 (Luna et al., 2014), whereas human ASCs may demonstrate quite a different expression of CD90 and CD105, which correlates with their differentiation potential (Baer, 2014). The situation is complicated further by the different levels of expression of markers in ASCs isolated from various regions, i.e., from subcutaneous and visceral fat (Ong et al., 2014).
White and brown ASCs are different in the origin and lineage characteristics, particularly, white adipose stem cells originate from Myf5 (myogenic regulatory factor) negative progenitors, whereas brown adipose stem cells originate from myogenic lineage and express Myf5 (Algire et al., 2013). In general, adipose progenitors develop in close relation to vasculature; express PPARγ, stem cell antigen-1 (SCA-1), CD34, smooth-muscle actin (SMA), PDGFRβ, and chondroitin sulfate proteoglycan 4 (NG2), and VE-cadherin; and may, probably, have endothelial (but not hematopoietic) origin (Algire et al., 2013; Onogi et al., 2017). As an example, the expression profile of perivascular ASCs contains (in addition to mesenchymal markers) some markers of endothelial cells (i.e., platelet endothelial cell adhesion molecule CD31/PECAM-1, vascular cell adhesion molecule CD106/VCAM-1, melanoma cell adhesion molecule CD146/MCAM, and activated leukocyte cell adhesion molecule CD166/ALCAM) and perivascular cells (i.e., 3G5 ganglioside expressed in pericytes) (Zannettino et al., 2008). Presumably, such controversies in results of assessment of expression patterns in ASCs could be caused by different protocols applied for cell purification and characterization.
Some authors suggest that both endothelial cells and pericytes are precursors of adipose progenitors as confirmed by the scrupulous VE-cadherin promoter-driven lineage-tracing experiments in rodents and experiments with cultured human adipose tissue: endothelial cells of developing white and brown adipose tissue capillaries are a source of adipocyte precursors; thereby, adipocytes and endothelial cells are plastic enough to undergo interconversion (Tran et al., 2012).
Perivascular location of ASCs might suggest their contribution to a well-known endocrine and paracrine activity of adipose tissue surrounding medium- and large-sized vessels in the context of the so-called adipose-vascular coupling (Gollasch and Dubrovska, 2004; Gollasch, 2017; Gollasch et al., 2018). The PVAT is in direct contact with the vessel adventitia, which is considered as a progenitor cell niche within the vessel wall inhabited by a huge number of stem cells and progenitors, i.e., endothelial progenitor cells (EPCs), progenitors for SMCs, mesenchymal stem cells (MSCs), mesangial cells coexpressing both endothelial and myogenic markers, and organ- and tissue-specific progenitors, i.e., neural stem cells in neural tissue (Ergun et al., 2011; Majesky et al., 2011). Thus, it is not surprising that ASCs reside in this particular area as a part of the MSCs population (adventitial vasculogenic zone). It is important to note that PVAT (particularly in the thoracic, but not in the abdominal area) are originated from Myf5- precursors and may not share myogenic characteristics typical for “classic” brown ASCs (Hildebrand et al., 2018).
The ASCs with multilineage differentiation potential have been isolated successfully from metabolically active brown adipose tissue (Silva et al., 2014). In contrast to white ASCs, visceral (i.e., mediastinal) brown adipose tissue-derived ASCs (BADSCs) demonstrate the highest expression of transmembrane protein 26 (TMEM26) and CD137 that also can be considered as markers of beige adipose tissue (white adipose tissue is enriched in brown UCP1-expressing thermogenically competent adipocytes) (Silva et al., 2014). Therefore, BADSCs might be defined as a population of stem cells with promising applications either in tissue engineering or in therapeutic protocols (Yang et al., 2017; Chen et al., 2018).
The clonogenic activity of ASCs is supported by the microenvironment established within the SVF by fibroblasts, mature brown adipocytes and preadipocytes, pericytes, immune cells (macrophages, lymphocytes), and endothelial cells/EPCs (Chazenbalk et al., 2011; Kim et al., 2014). Strictly speaking, adipogenic niches are required for adipogenesis, i.e., in the conditions of brown adipogenesis induction (beta-adrenergic stimulation, hypothermia) or in white adipogenesis induction (high-fat feeding) (Lee et al., 2012, 2013; Lee Y.H. et al., 2015). It is interesting that stimulation of adipogenesis is associated usually with remodeling of the niche-resembling establishment of the pro-inflammatory microenvironment (activation of local macrophages) (Lee et al., 2013).
Establishment of the clonogenic niche in the perivascular adipose fraction provides a microenvironment enriched with factors contributing to the regulation of stemness and self-renewal of ASCs. As an example, stimulation of PPARγ in ASCs results in activation of PDGFRβ and VEGFR, further leading to extensive local vascular sprouting and increased vessel niche affinity for ASCs (Jiang et al., 2017). Thus, the local concentration of PPARγ ligands (fatty acids derivatives including nitroalkene fatty acids) might be one of the critical factors for controlling the occupancy of ASCs within the perivascular niche. Expression of CD73 in ASCs suggests involvement of adenosine-mediated mechanisms in the regulation of behavior of stem cells. In this scenario, CD39 expressed by stromal cells or SVF regulatory T-cells may produce adenosine monophosphate (AMP) from adenosine triphosphate (ATP), whereas CD73 expressed by ASCs can convert AMP to adenosine (de Oliveira Bravo et al., 2016; Donninelli et al., 2017). Presence of CD203+ macrophages in the adipose tissue and, particularly, in the SVF niche (Faris et al., 2012; Silva et al., 2017) leads to the proposal of an alternative mechanism. Particularly, adenosine might be produced in the SVF niche due to conversion of NAD+ into ADP-ribose by means of the activity of CD38/NAD+-glycohydrolase expressed in stem cells, followed by ADP-ribose metabolism to AMP due to activity of CD203a expressed by macrophages. Then, the CD73-mediated reaction might result in adenosine production as it was proposed before for activated immune cells (Horenstein et al., 2013). Regardless of the way of production, adenosine is a well-known regulator of development of stem cells (Carroll et al., 2012; Jing et al., 2015). Mesenchymal stem cells as well as adipocytes in white and brown adipose tissue express adenosine receptors (Gharibi et al., 2011; Tozzi and Novak, 2017); therefore, local production of adenosine may control the development of ASCs. Therefore, it is very reasonable that modulation of adenosine receptors regulates adipogenesis: adenosine activates brown adipose tissue adipocytes and brown like beige adipocytes (Gnad et al., 2014), promotes differentiation, and blocks lipolysis in adipocytes (Eisenstein and Ravid, 2014).
Adipogenesis and production of adipose-derived regulatory factors are compromised in chronic vascular dementia, neurodegeneration, and cardiovascular diseases (Zhou and Qin, 2012; Ishii and Iadecola, 2016). Adipose-derived factors contribute to progression of vascular dementia and Alzheimer’s disease (Ishii and Iadecola, 2016). High salt intake provokes adipogenesis and local inflammation (Park et al., 2017). Aberrant epicardial adipogenesis contributes to pathogenesis of cardiac arrhythmias (Yamaguchi et al., 2015) with the distribution of adipose tissue being changed. Also, there is a negative correlation between adipose tissue-produced leptin levels and amyloid-beta concentrations in cerebrospinal fluid in females with Alzheimer’s disease (Diehl-Wiesenecker et al., 2015). Thus, it is very reasonable that adipose stem cells or their derivatives have been tested in humans and animal models as a regenerating therapeutic tool. However, it is too early to conclude on promising results in preclinical animal studies or clinical trials for myocardial infarction (Lee H.W. et al., 2015; Kim et al., 2016; Bobi et al., 2017; Joo et al., 2017), ischemic cardiomyopathy (Suzuki et al., 2015), stroke (Gutiérrez-Fernández et al., 2013; Grudzenski et al., 2017), and Alzheimer’s disease (Lee et al., 2018). In light of these introductory remarks, it is apparent that deciphering the novel regulatory mechanisms of the self-renewal and differentiation of ASCs would promote application of advanced and safe protocols for regenerating therapy. The next chapters will cover some recent achievements in understanding the metabolic activity within the SVF niches, which is critical for maintaining the pool of ASCs, and disclosures of their regenerative, particularly, pro-angiogenic potential.
Metabolism of Lactate and NAD+ in Adipose Clonogenic Niches
Mobilization of fatty acids from adipocytes and production of lactate serve as a major mechanism of energy supply, and uncoupling of oxidation and phosphorylation in adipocyte mitochondria supports thermogenesis (Berry et al., 2013). Recently, it has become clear that glycolytic production of lactate and its transport via monocarboxylate transporters (MCTs) control metabolic activity of brown fat cells underlying thermogenesis. Prolonged optogenetic activation of ChR2-expressing sympathetic neurons innervating brown adipose tissue resulted in thermogenic responses and extensive GLUT1-mediated glucose uptake needed for lactate production and MCT1-mediated lactate transport and its utilization as an energy substrate (Jeong et al., 2018).
How do such metabolic and regulatory activities of the adipose tissue affect the behavior of ASCs? The ASCs, as all other cells with multilineage differentiation characteristics, greatly depend on glycolysis whose metabolites are required for maintaining the pool of stem cells and preventing their inappropriate (uncontrolled) recruitment (Burgess et al., 2014). Stem cells themselves as well as neighboring cells serve as a source of lactate. Glycolysis in adipose cells is required absolutely for their functional activity and differs in the adipose tissue obtained from various anatomical locations (Ferng et al., 2016). Glycolytic activity of brown fat cells depends on transcriptional activation of HIF-1, expression of glucose transporters (GLUT) expression, and glycolytic enzymes (Basse et al., 2017). As an example, activation of PDGFRβ is required for effective glucose uptake in the adipose tissue (Onogi et al., 2017). It was observed that adipogenic induction is associated usually with transition from glycolytic mechanism to mitochondrial respiration (Drehmer et al., 2016; Zheng et al., 2018).
Proteomic studies reveal that expression of glycolytic enzymes in mature adipocytes and in SVF cells is different (Kheterpal et al., 2011), thereby, suggesting that the niche establishment is associated with specific changes in energy production in the surrounding cells of ASCs. As it was shown in other clonogenic niches located in bone marrow, brain, or in tumor loci, elevated production of lactate is a prerequisite for maintaining stem cells pool, whereas changes in lactate concentrations serve as a signal for cell proliferation and differentiation being responsible for the so-called Warburg’s effect and reverse Warburg’s effect (increased glucose uptake followed by extensive glycolysis in stem/progenitor cells or in surrounding accessory cells, respectively) (Álvarez et al., 2014; Malinovskaya et al., 2016). Along the process of differentiation, preadipocytes acquire a glycolysis-independent mode of energy production and come to utilize more fatty acids (Roberts et al., 2009). As expected, expression of lactate transporters – MCT1 and MCT4 – is progressively elevated in adipocytes during white and brown adipogenesis, which is important for lactate influx and support of mitochondrial energy production (Petersen et al., 2017). Lactate serves as a “browning” signal for white adipose cells: in an MCT-dependent manner, it increases the expression of UCP1 and mitochondrial activity (Vergnes and Reue, 2014). Moreover, brown adipose cells most abundantly express GPR81 as lactate receptor (Liu et al., 2009), and in mature adipocytes, activation of GPR81 receptors leads to the inhibition of lipolysis (i.e., in insulin action) (Rooney and Trayhurn, 2011). It is especially interesting to note that expression of GPR81 is required for stem cell maintenance (Choi et al., 2015), but whether BADSCs express and use GPR81 activity for their own needs within the SVF niche remain to be evaluated.
In white adipose tissues, expression of glycolytic enzymes is elevated during growth of adipocytes and glycolysis is activated in mitochondrial uncoupling (Sabater et al., 2014). It should be noted that brown adipocytes differ from white adipocytes in that brown adipocytes convert a greater proportion of metabolized glucose into (lactate + pyruvate) and a smaller proportion into fatty acids (de novo lipogenesis) than do white adipocytes (Saggerson et al., 1988). Since reduction of de novo lipogenesis may correspond to the insulin-resistant state in adipose tissue or may reflect secondary changes in processes requiring fatty acids-derived products as regulatory factors (protein acetylation, PPARγ signaling) (Guilherme et al., 2017), one can assume that suppression of glycolytic production of lactate in differentiating SVF adipocytes should relate to differentiation-coupled changes in epigenetic mechanisms. Adult cow BADSCs cultured in vitro demonstrate reduction of histone H3K9 acetylation (marker of transcriptionally active chromatin), presumably, due to reduced pluripotency potential of the stem cells and their commitment to a particular lineage or to cellular senescence (Abouhamzeh et al., 2015), which is a general phenomenon in the differentiation of stem cells. Thus, dynamic changes in the glycolytic activity of BADSCs as well as surrounding cells within the adipose niches would affect differentiation of stem cells due to secondary alterations in lactate bioavailability and fatty acids metabolism.
Hydrogen sulfide (H2S) serves as a regulator of glycolysis in several cell types (Lee et al., 2014). The PVAT is an important source of endogenously produced H2S (Schleifenbaum et al., 2010; Gollasch, 2017). On the contrary, H2S supports the proliferation and viability of ASCs (Dongó et al., 2014; Aykan et al., 2015); therefore, one can assume that it might relate to H2S-mediated effects on glycolytic production of lactate within the SVF niche. This possible link between H2S-producing ability and glycolysis efficacy in SVF cells remains to be evaluated.
Another important property of glycolysis and lactate production is intracellular NAD+ regeneration coupled to pyruvate-lactate conversion. Therefore, greater glycolysis and higher lactate production in the early stages of the development of the BADSCs could be important for maintaining intracellular NAD+ levels adequate for the actual metabolic needs of actively proliferating and differentiating cells. However, this is true only for conditions when mitochondrial respiration is suppressed because action of lactate as mitochondrial fuel would require reverse conversion and rise in NADH concentrations. Thus, differentiation of cells within the SVF niche should be associated with depletion of the intracellular pool of NAD+.
Adipose cells are well equipped with NAD+-generating and -consuming machinery. As an example, expression of visfatin (nicotinamide phosphoribosyltransferase involved in the salvage pathway of NAD+ biosynthesis) is prevalent in visceral adipose tissue (Coelho et al., 2013). The NAD+-consuming enzymes are NAD+-glycohydrolases (CD38, CD157), poly (ADP-ribose) polymerase, and histone deacetylases (HDAC or sirtuins). The NAD+-glycohydrolase/CD38 is a receptor and an enzyme-generating second messenger with Ca2+-mobilizing activity (i.e., cyclic ADP-ribose) and is expressed widely in brain, liver, adipose tissue, and immune cells. Activity of CD38 regulates many biological functions, i.e., immune response, insulin secretion, contraction of cardiomyocytes, glial activation, and secretion of neuropeptides (Malavasi et al., 2008; Salmina et al., 2009; Lopatina et al., 2012). A progressive increase in the expression of CD38 in adult adipose tissue in aging relates to depletion of intracellular NAD+ levels and can be responsible for mitochondrial dysfunction in a sirtuin-dependent manner (Camacho-Pereira et al., 2016). Recently, a complex experimental approach with flow cytometry, cell-sorting protocols, and immunohistochemistry revealed that CD38 should be considered as a marker of preadipocytes that are committed to the adipogenic differentiation program (CD45- CD31- CD34-low CD38+ cells); therefore, the number of CD38-immunopositive cells with reduced proliferative potential is increased in extensive adipogenesis (Carriere et al., 2017). In support of this observation, CD38 deficiency was found to inhibit adipogenesis-activating fatty acid synthase via the Sirt1/PPARγ-signaling pathway (Wang et al., 2018). It is tempting to speculate that an increase in CD38 expression might occur within the SVF niche at the stage of differentiation of preadipocytes and correspond to relative suppression of glycolysis. As a result of the two mechanisms (overexpression of CD38 and reduction of glycolytic flux), intracellular NAD+ levels in ASCs may decrease dramatically.
It is apparent that such changes in the production and utilization of lactate may correspond also to the activity of HDACs; sirtuins. As it was suggested, energy shortage under the conditions of caloric restriction, starvation, and exercise activate sirtuins, whereas high-fat feeding suppresses the activity of sirtuins (Jokinen et al., 2017). Expression of sirtuins in adipose stem cells varies in a region-specific manner (Mariani et al., 2017). In the brown adipose tissue, the activity of various sirtuins is required for the regulation of mitochondrial number, mitochondrial respiration, glucose uptake, and thermogenesis, whereas, in the white adipose tissue, other effects of sirtuins activity have been detected (control of adipokines production, adipogenesis) (Jokinen et al., 2017). Particularly, sirtuins 1 and 2 suppress adipogenesis under fasting conditions (Rodriguez et al., 2013), whereas mitochondrial sirtuin 3 in brown adipocytes affects positively the expression of UCP1 and thermogenesis (Ansari et al., 2017). Thus, NAD+ bioavailability may dictate the expression levels and activity of sirtuins in ASCs and neighboring cells [i.e. (pre)adipocytes], thereby, providing a mechanistic link between the intensity of glycolysis, metabolism, and clonogenic capacity of ASCs. Figure 1 illustrates the role of lactate and NAD+ metabolism in supporting the functional competence of ASCs.
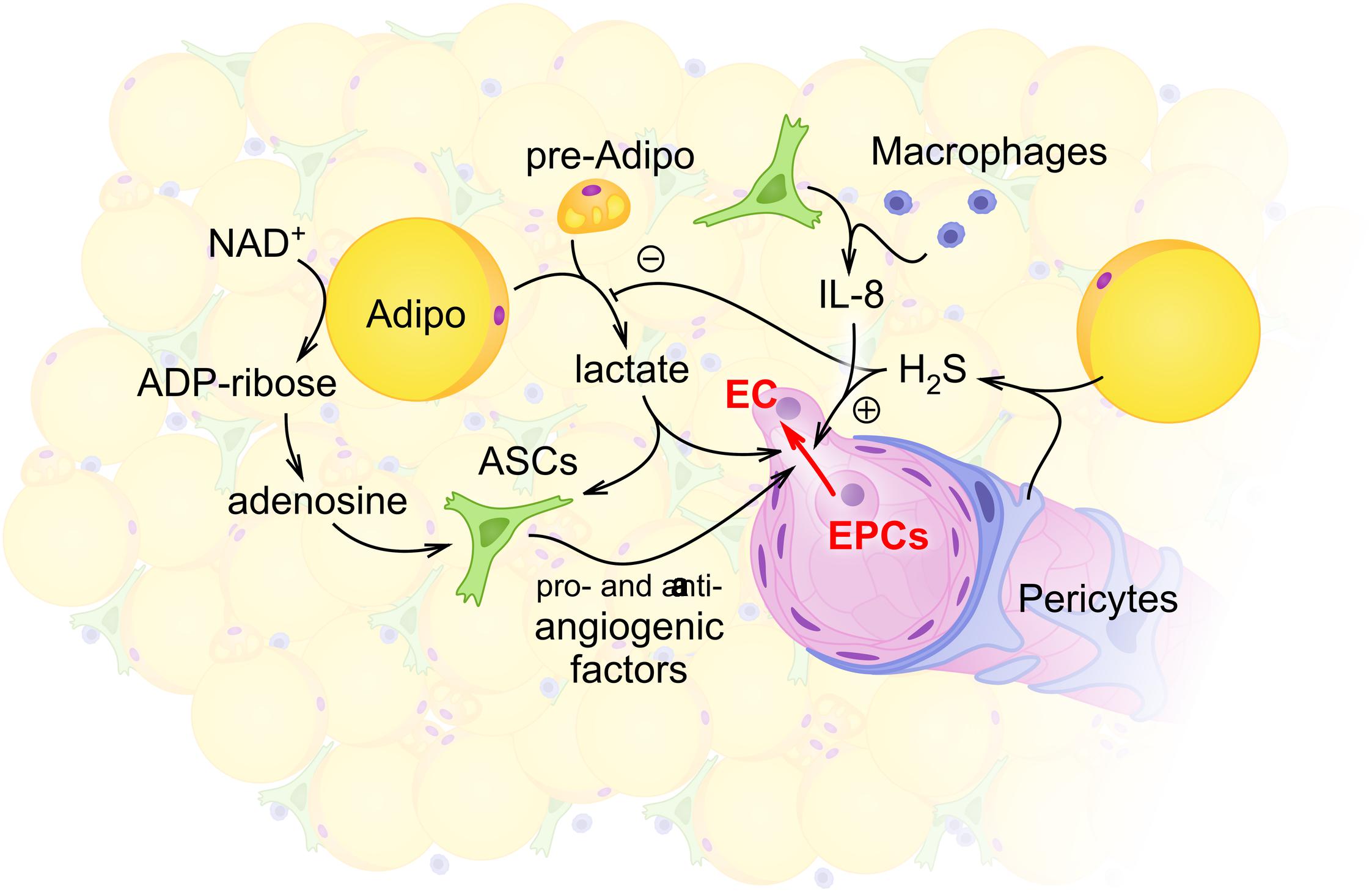
FIGURE 1. Schematic illustration of intercellular communications within the stromal vascular fraction (SVF) with the focus on adipocyte- and ASCs-mediated regulation of angiogenesis. Within the SVF, adipocytes (Adipo) and preadipocytes (pre-Adipo) serve as a major source of glycolytically produced lactate. Elevated levels of lactate in the extracellular space support proangiogenic activity of ASCs whose maintenance also depends on glycolytic flux and mitochondrial respiration. Some other locally produced molecules [hydrogen sulfide H2S in pericytes (P) or adipocytes; adenosine and interleukin-8 (IL-8) in ASCs or macrophages] contribute to angiogenesis control within the SVF. As a result, activity of endothelial cells (ECs) and EPCs provides angiogenesis and vascular remodeling adjusted to the current metabolic and functional needs of adipose tissue.
Pro-Angiogenic Activity Within Adipose Clonogenic Niches
Reciprocal interactions connect two mechanisms – adipogenesis and angiogenesis. Expansion of adipose tissue requires capillary growth; therefore, either in embryonic or in adult stages of ontogenesis, the development of adipose tissue corresponds to the intensity of local angiogenesis/vasculogenesis.
The SVF niches serve as a platform for angiogenesis and neovascularization, where VEGF and PDGF appear as major regulatory signals (Klar et al., 2016; Bora and Majumdar, 2017). Current data suggest that SVF serves as an important source of endothelial progenitors, endothelial cells, and pericytes, thereby, contributing to vessel remodeling and growth (Han et al., 2015). Adipose tissue itself is a big source of angiogenesis activators and inhibitors (Cao, 2007). Pro-angiogenic activity of PVAT is provided by the secretion of soluble factors with strong angiogenic potential, i.e., vascular endothelial growth factor (VEGF), acidic fibroblast growth factor (aFGF), monocyte chemoattractant protein-1 (MCP-1), insulin like growth factor-binding protein-3 (IGFBP-3), glia-derived neurotrophic factor (GDNF), and hepatocyte growth factor (HGF) (Voros et al., 2005; Rittig et al., 2012; Horimatsu et al., 2017). The CD248-immunopositive SVF ASCs show higher pro-angiogenic potential than do CD248-cells (Zielins et al., 2015; Brett et al., 2017). The CD248 (endosialin) is a positive regulator of the development of pericytes (Tomkowicz et al., 2010). In mice, endothelial cells in vessels within the adipose tissue may give rise to new adipocytes coupled via tight junction proteins at the very early stages of their differentiation in vivo (Tran et al., 2012). Pro-angiogenic activity of adipose niche cells has been confirmed numerous times in vitro: 14 days coculture of mice endothelial cells and ASCs drives Wnt-regulated angiogenesis and functional vessel formation in 3D collagen matrices (Cai et al., 2017), ASCs support greater sprouting of endothelial cells being cocultured on bioengineered polycaprolactone/gelatin nanofibrous scaffolds (Kook et al., 2018), and coculture of ASCs with adipose-derived microvascular endothelial cells results in enhanced vascular network formation during 14 days of incubation period in vitro (Freiman et al., 2016).
Antiangiogenic activity of adipose tissue relies on the stimulated secretion of adiponectin, endostatin, thrombospondin-1, soluble VEGF receptor 2 type, and transforming growth factor-beta (TGFβ) (Cao, 2007). The functional activity of adipocytes controls secretion of pro- and antiangiogenic factors, i.e., cold stress stimulates production of VEGFA in brown adipose tissue and it directly activates proliferation of adipocytes precursor and endothelial cells (Wang et al., 2015). On the contrary, fatty acids mobilized from adipocytes could serve as ligands for PPAR receptors, thereby, affecting endothelial cells, i.e., as it was shown for PPARα receptors expressed in brain microvascular endothelial cells (BMECs) within the blood-brain barrier (BBB), thus leading to hyperpermeability of BBB (Caroline et al., 2009). Exosomes released from ASCs and containing microRNA-181b-5p are able to stimulate angiogenesis through activation of BMECs, particularly, under hypoxic conditions (Yang et al., 2018).
As it was mentioned earlier, endothelial cells and pericytes may serve as precursors of ASCs (Tran et al., 2012). Indeed, SVF contains a CD31-, S100+ cell type that can differentiate into adipocytes and endothelial cells and CD31+ SVF cells can be converted to adipocytes in vitro (Wosnitza et al., 2007). Human SVF-derived cells retrieved from the adipose tissue can differentiate into endothelial cells, and being transplanted into mice are able to support angiogenesis in vivo, whereas dedifferentiated mature human adipocytes display the potential to acquire the endothelial phenotype in vitro and promote vessel-like tube formation (Planat-Benard et al., 2004). Recent data suggest that bone marrow-derived cells (probably mesenchymal cells and endothelial progenitors) may also contribute to the pool of adipocytes (Arner and Rydén, 2017); even some controversial observations exist (Koh et al., 2007) that might be explained by the presence of distinct subpopulations of progenitors (Jiang et al., 2014).
Contacts between endothelial cells and ASCs are necessary for inducing pro- or antiangiogenic secretory activity in stem cells. As an example, endothelial cells contacting ASCs in vitro induce expression of activin A, which is an important factor in controlling vasculogenesis (Merfeld-Clauss et al., 2015). Assessment of vasculogenesis in vitro in 3D fibrin matrices demonstrated that direct interactions of human umbilical vein endothelial cells (HUVECs) and ASCs are required for promotion of angiogenesis: culture of HUVEC alone due to extensive secretion of VEGF, angiogenin, angiopoietin-1, MCP-1, matrix metaloproteinases, and many other proangiogenic factors as well as angiogenesis inhibitors (i.e., thrombospondin-1, platelet factor 4, serpin F1, etc.) (Rohringer et al., 2014). The most interesting finding of this study was a mechanism of acquiring pericyte phenotype by ASCs cocultured with HUVEC, especially in the regions of newly formed tubes bifurcations within the vascular network. It might be pertinent to note that human pericytes obtained from adipose tissue display better differentiating phenotypes compared to pericytes developed from MSCs (Pierantozzi et al., 2015).
Endothelial progenitor cells (EPCs) with high angiogenic potential can be isolated easily from SVF (Van Pham et al., 2016) and used further for bioengineering purposes. It is important to mention that adipose tissue-derived EPCs demonstrate higher proliferative potential than EPCs obtained from ASCs themselves (Zhou et al., 2015). The EPCs isolated from adipose tissue have been shown also to be present in the microvessel fraction, particularly, in sub- or periendothelial space close to pericytes (Ergun et al., 2011). Taking into consideration the close relations of adipocytes and endothelial cells as well as the high degree of their interlineage plasticity, mesenchymal-to-endothelial transformation of ASCs (Zhou et al., 2015), one may assume that this phenomenon contributes to various (patho)physiological processes as was shown earlier for several types of vessel remodeling and neovascularization (Ubil et al., 2014). Since these events are driven usually by local chronic hypoxia, initiation of mesenchymal-to-endothelial transition of ASCs should be associated with excessive adipogenesis and enlargement of adipose tissue clusters. It is well known that hypoxia is associated always with lactate accumulation; therefore, local production of lactate within SVF could regulate differentiation of ASCs into endothelial cells. Indeed, lactate has been shown to serve as a positive regulator of angiogenesis, including model systems with specially designed matrices (lactate-releasing or demonstrating gradients in lactate concentrations) (Hunt et al., 2007; Porporato et al., 2012; Malinovskaya et al., 2016; Salmin et al., 2017), and lactate-enriched microenvironment within clonogenic niches might be able to promote vasculogenesis in the loci of extensive adipogenesis. The proangiogenic activity of lactate often requires IL-8-driven proliferation of endothelial cells (Polet and Feron, 2013), and adipose cells appear to be good producers of IL-8 into the systemic circulation (Bruun et al., 2004). Recent experimental data on the stimulated microgravity-induced angiogenesis-supporting expression pattern in ASCs (elevated levels of Serpin E1, Serpin F1, insulin growth factor binding protein (IGFBP), VEGF, and IL-8 in ASCs) (Ratushnyy et al., 2018) confirm partially the mechanism of lactate-mediated proangiogenic effects within the SVF niche.
In sum, the population of ASCs in SVF consists of pluripotent stem cells that could be considered as vasculogenic precursors able to differentiate into endothelial cells and pericytes to support angiogenesis/vasculogenesis and vessel remodeling. Thus, it is very reasonable to expect that ASCs-derived cells are very promising materials for establishment of bioengineered constructs for regenerative and vessel-replacement needs.
Conclusion and Perspectives: Novel Vascular Engineering Strategies in Cardiovascular and Cerebrovascular Diseases
Initial attempts in utilizing the regenerative potential of ASCs have been connected with infusions of ASCs. Intracoronary infusion of ASCs in experimental animals with a model of transmural myocardial infarction resulted in increased left ventricle ejection fraction, elevated thickness of ventricular wall in the infarction area, and improved vascular density at the border zone at 4 weeks after the infusion (Valina et al., 2007). Systemic delivery of ASCs was applied in animal models of myocardial infarction (Hong et al., 2014). In parallel, application of ASCs has been progressing as target differentiation of ASCs in vitro to produce desirable cell lines, i.e., into beating cardiomyocytes due to epigenetic modifications of human ASCs or their coculture with rodent cardiac cells (Choi et al., 2010) and cardiac pacemakerlike cells due to transfection of rodent ASCs with TBX18 gene and their coculture with neonatal rodent cardiomyocytes (Yang et al., 2016).
Positive results have been obtained in rats with experimental ischemic stroke after intravenous administration of adipose tissue-derived MSCs (improvement of neurogenesis, oligodendrogenesis, synaptogenesis, and cerebral angiogenesis) (Gutiérrez-Fernández et al., 2013). In neonatal rats with perinatal hypoxic-ischemic brain injury, when ASCs were implanted with adipose stem cells-derived EPCs and neural progenitors, they demonstrated improved status (Hsueh et al., 2015). Alzheimer’s disease is known to have a significant vascular component in its pathogenesis (Kester et al., 2014); therefore, utilization of the proangiogenic capacity of ASCs might have therapeutic potential. However, various target processes seem to be involved in the positive action of ASCs and their derivates in experimental neurodegeneration. Neurogenic differentiation of ASCs could be achieved in vitro (Safford et al., 2002), while stimulation of endogenous neurogenesis was registered in mice with an Alzheimer’s disease model treated with ASCs (Yan et al., 2014). When human ASCs were injected intravenously or intracerebroventricularly into aging mice, improvements of their locomotor activity and cognitive function have been registered (Park et al., 2013). It is interesting that breakdown of the BBB seen in Alzheimer’s disease may contribute positively to the efficacy of ASCs-based therapy: intravenously injected human ASCs were able to reach brain tissue in transgenic Alzheimer’s disease model mice, but not in control mice (Ha et al., 2014). Another original approach was suggested to correct amyloid-beta proteolysis in the brain by means of neprilysin-carrying human ASCs-derived exosomes (Katsuda et al., 2015). This approach has been developed when neprilysin-enriched exosomes were found as products of activated ASCs (Katsuda et al., 2013). Thus, not only ASCs themselves of ASCs-originated neuronal cells, but also ASCs-derived exosomes could be considered as a therapeutic tool in Alzheimer’s type of neurodegeneration. Very recent data suggest that activated ASCs might be also efficient in the mouse model of Parkinson’s disease (Chi et al., 2018).
However, more preclinical studies and clinical trials are required since assessment of safety should be considered always in order to get rational conclusions on the clinical applications of ASCs in cardiovascular and cerebrovascular/neurodegenerative diseases (Toyserkani et al., 2017).
The next phase in the application of ASCs is linked to engineering the blood microvascular in vitro networks as a platform for drug testing or as a prototype for tissue implants based on ASCs cultured alone or with endothelial cells. Such attempts already have produced rather promising results (Murohara, 2009; Knezevic et al., 2017). Multilineage properties (assessed by differentiation of ASCs into a wide spectrum of cells) and high regenerative capacity (confirmed by analysis of angiogenic activity, senescence-resistant phenotype, and susceptibility to oxidative stress in vitro) of ASCs compared with bone marrow-derived stromal mesenchymal cells make them attractive candidates for bioengineering tasks. Particularly, their application on 3D scaffolds or in microfluidic systems, where regenerative potential of seeded cells or their response to the matrix architecture are of great importance, is very promising (Lee et al., 2016). Behavior of ASCs in microfluidic devices (strong proliferation and migration abilities) (Wadhawan et al., 2012) confirms good potential for the application of ASCs in dynamic cell models, multicellular ensembles, and microphysiological systems as well as for tissue-printing purposes (Zhang et al., 2017). As an example, the multi chamber dynamic system was developed successfully to study effects of various stimuli on ASCs differentiation toward myocardial phenotype (Pavesi et al., 2014).
Finally, ASCs could be considered as a source of cells for bioengineering constructions mimicking clonogenic niches in vitro. Establishment of a clonogenic/angiogenic microenvironment is a very complex problem whose solving would provide great progress in constructing implantable regeneration-supporting devices or supporting survival of grafted cells in vivo. Several attempts have been made to produce human SVF in vitro by placing ASCs and ASCs-supporting cells (pericytes, endothelial cells) on perfused scaffolds (Scherberich et al., 2010; Cerino et al., 2017; Costa et al., 2017). The results confirmed strong angiogenic potential of ASCs and release of proangiogenic factors and development of vascular network (Cerino et al., 2017; Costa et al., 2017). Recently, tissue-engineered vascular grafts with BADSCs have been proposed as promising novel alternatives to replace diseased vessels in cardiovascular and cerebrovascular diseases (Wang et al., 2016, 2017). Another task is very close on technological issues: development of ASCs-based adipose tissue microchip (“fat-on-chip”) with possible diagnostic, therapeutic, pharmacological, and toxicological applications (Loskill et al., 2016, 2017; Chen et al., 2017; Tanataweethum et al., 2017; Li and Easley, 2018).
All the earlier-mentioned approaches for the application of ASCs for diagnostic and therapeutic purposes are summarized in Figure 2.
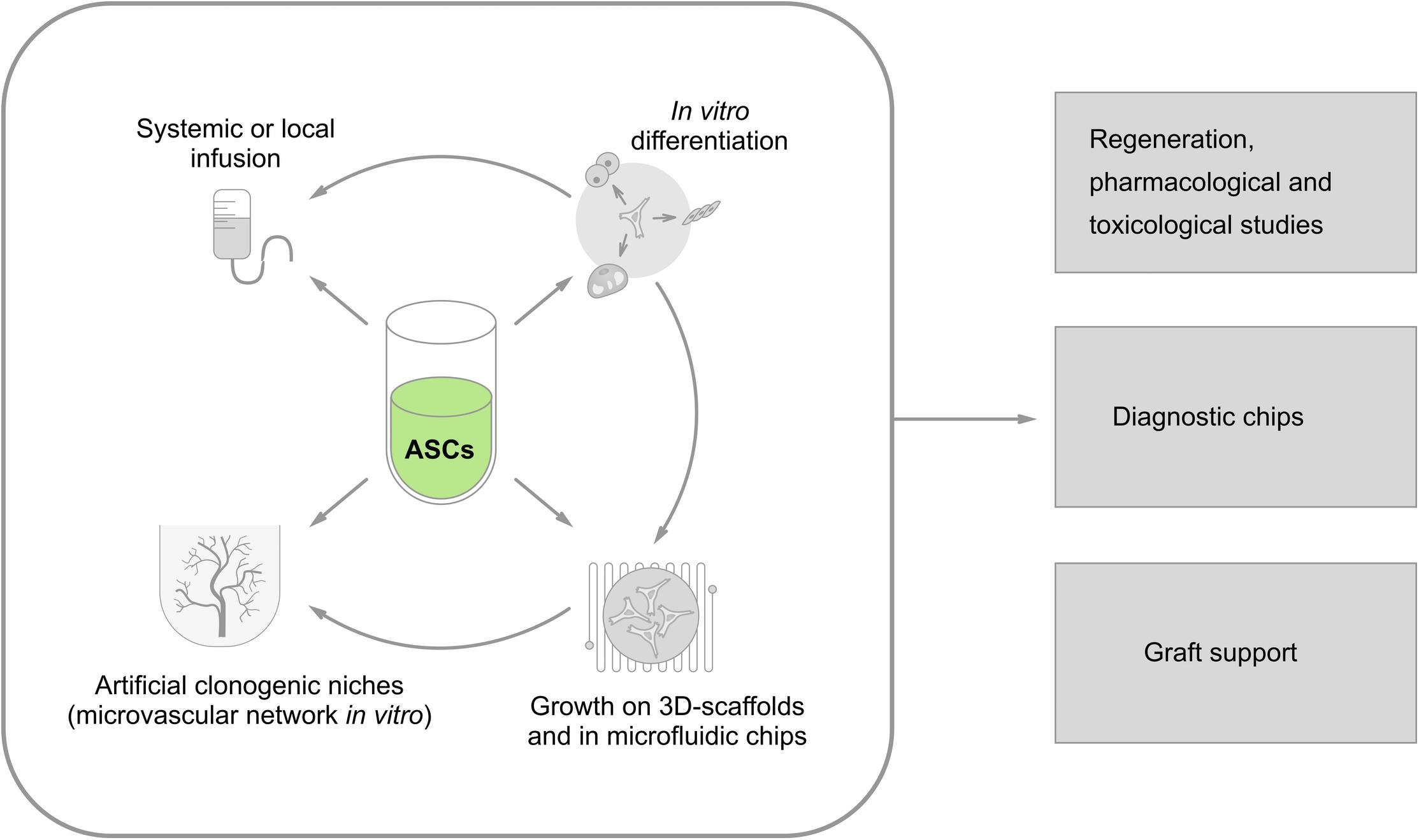
FIGURE 2. Current approaches to vascular engineering strategies utilizing ASCs as a source of vascular and perivascular cells. Isolated ASCs might be used for: (i) systemic (intravenous) or local (i.e., intracoronary) administration; (ii) in vitro differentiation toward the desired phenotype (myocardial cells, pacemakerlike cells, endothelial cells, smooth muscle cells, neuronal cells, etc.); (iii) establishment of 3D models and microfluidic systems in vitro; and (iv) development of artificial clonogenic niches for controlled in vitro production of stem cells supported by microvascular network). Then, all these approaches could be used in: (i) regeneration therapeutic protocols aimed to re-establish tissue components, including (micro)vessels; (ii) drug/xenobiotic testing in vitro; (iii) diagnostic devices in “lab-on-chip format”; (iv) supporting devices for grafted cells (as vascular scaffolds) or bioreactors for efficient generation of stem and progenitor cells in vitro.
In sum, remarkable progress has been made in the last decades in deciphering the molecular mechanisms of the functional activity of ASCs, particularly, in relation to their control of angiogenesis. Vascular scaffolds existing in real niches (i.e., in adipose tissue SVF, bone marrow niche, brain neurogenic niches, oligovascular niches) can be reproduced in vitro. Thus, adequate support for maintenance of stem cells and control of their differentiation suggests new approaches for the optimization of tissue-regeneration protocols, particularly, in the complex therapy of cardiovascular and cerebrovascular diseases.
Author Contributions
YP, NM, TT, VS, and ABS conceived and wrote the manuscript. YK, OL, AM, AY, and ANS wrote the manuscript. AY designed the figures. All authors contributed to the final version of the manuscript. ABS supervised the project.
Funding
The authors are supported by the Ministry of Public Health, Russian Federation (State Assignment for Research, 2018–2020) (general analysis of ASCs and bioengineering constructions for modeling the clonogenic niches in vitro), and partially by the grant given by the President of Russian Federation for the leading research teams (HIII-6240.2018.7) (application of proangiogenic ASCs in neurodegeneration).
Conflict of Interest Statement
The authors declare that the research was conducted in the absence of any commercial or financial relationships that could be construed as a potential conflict of interest.
References
Abouhamzeh, B., Salehi, M., Hosseini, A., Masteri-Farahani, A. R., Fadai, F., Heidari, M. H., et al. (2015). DNA methylation and histone acetylation patterns in cultured bovine adipose tissue-derived stem cells (BADSCs). Cell J. 16, 466–475.
Algire, C., Medrikova, D., and Herzig, S. (2013). White and brown adipose stem cells: from signaling to clinical implications. Biochim. Biophys. Acta 1831, 896–904. doi: 10.1016/j.bbalip.2012.10.001
Álvarez, Z., Hyroššová, P., Perales, J. C., and Alcántara, S. (2014). Neuronal progenitor maintenance requires lactate metabolism and PEPCK-M-directed cataplerosis. Cereb. Cortex 26, 1046–1058. doi: 10.1093/cercor/bhu281
Ansari, A., Rahman, M. S., Saha, S. K., Saikot, F. K., Deep, A., and Kim, K. H. (2017). Function of the SIRT3 mitochondrial deacetylase in cellular physiology, cancer, and neurodegenerative disease. Aging Cell 16, 4–16. doi: 10.1111/acel.12538
Arner, P., and Rydén, M. (2017). The contribution of bone marrow-derived cells to the human adipocyte pool. Adipocyte 6, 187–192. doi: 10.1080/21623945.2017.1306158
Aykan, A., Ozturk, S., Sahin, I., Avcu, F., Sagkan, R. I., and Isik, S. (2015). The effects of hydrogen sulfide on adipocyte viability in human adipocyte and adipocyte-derived mesenchymal stem cell cultures under ischemic conditions. Ann. Plast. Surg. 75, 657–665. doi: 10.1097/sap.0000000000000595
Baer, P. C. (2014). Adipose-derived mesenchymal stromal/stem cells: an update on their phenotype in vivo and in vitro. World J. Stem Cells 6, 256–265. doi: 10.4252/wjsc.v6.i3.256
Basse, A. L., Isidor, M. S., Winther, S., Skjoldborg, N. B., Murholm, M., Andersen, E. S., et al. (2017). Regulation of glycolysis in brown adipocytes by HIF-1α. Sci. Rep. 7:4052. doi: 10.1038/s41598-017-04246-y
Berry, D. C., Stenesen, D., Zeve, D., and Graff, J. M. (2013). The developmental origins of adipose tissue. Development 140, 3939–3949. doi: 10.1242/dev.080549
Berry, R., Rodeheffer, M. S., Rosen, C. J., and Horowitz, M. C. (2015). Adipose tissue residing progenitors (Adipocyte Lineage Progenitors and Adipose Derived Stem Cells (ADSC). Curr. Mol. Biol. Rep. 1, 101–109. doi: 10.1007/s40610-015-0018-y
Bobi, J., Solanes, N., Fernandez-Jimenez, R., Galan-Arriola, C., Dantas, A. P., Fernandez-Friera, L., et al. (2017). Intracoronary administration of allogeneic adipose tissue-derived mesenchymal stem cells improves myocardial perfusion but not left ventricle function, in a translational model of acute myocardial infarction. J. Am. Heart Assoc. 6:e005771. doi: 10.1161/jaha.117.005771
Bora, P., and Majumdar, A. S. (2017). Adipose tissue-derived stromal vascular fraction in regenerative medicine: a brief review on biology and translation. Stem Cell Res. Ther. 8:145. doi: 10.1186/s13287-017-0598-y
Brett, E., Zielins, E. R., Chin, M., Januszyk, M., Blackshear, C. P., Findlay, M., et al. (2017). Isolation of CD248-expressing stromal vascular fraction for targeted improvement of wound healing. Wound Repair Regen. 25, 414–422. doi: 10.1111/wrr.12542
Bruun, J. M., Lihn, A. S., Madan, A. K., Pedersen, S. B., Schiott, K. M., Fain, J. N., et al. (2004). Higher production of IL-8 in visceral vs. subcutaneous adipose tissue. Implication of nonadipose cells in adipose tissue. Am. J. Physiol. Endocrinol. Metab. 286, E8–E13. doi: 10.1152/ajpendo.00269.2003
Burgess, R. J., Agathocleous, M., and Morrison, S. J. (2014). Metabolic regulation of stem cell function. J. Intern. Med. 276, 12–24. doi: 10.1111/joim.12247
Cai, X., Xie, J., Yao, Y., Cun, X., Lin, S., Tian, T., et al. (2017). Angiogenesis in a 3D model containing adipose tissue stem cells and endothelial cells is mediated by canonical Wnt signaling. Bone Res. 5:17048. doi: 10.1038/boneres.2017.48
Camacho-Pereira, J., Tarrago, M. G., Chini, C. C. S., Nin, V., Escande, C., Warner, G. M., et al. (2016). CD38 dictates age-related NAD decline and mitochondrial dysfunction through an SIRT3-dependent mechanism. Cell Metab. 23, 1127–1139. doi: 10.1016/j.cmet.2016.05.006
Cao, Y. (2007). Angiogenesis modulates adipogenesis and obesity. J. Clin. Invest. 117, 2362–2368. doi: 10.1172/jci32239
Caroline, M., Maxime, C., Lucie, D., Bruno, D., Bart, S., Regis, B., et al. (2009). Peroxisome proliferator-activated receptor-alpha activation protects brain capillary endothelial cells from oxygen-glucose deprivation-induced hyperpermeability in the blood-brain barrier. Curr. Neurovasc. Res. 6, 181–193. doi: 10.2174/156720209788970081
Carriere, A., Jeanson, Y., Cote, J. A., Dromard, C., Galinier, A., Menzel, S., et al. (2017). Identification of the ectoenzyme CD38 as a marker of committed preadipocytes. Int. J. Obes. 41, 1539–1546. doi: 10.1038/ijo.2017.140
Carroll, S. H., Wigner, N. A., Kulkarni, N., Johnston-Cox, H., Gerstenfeld, L. C., and Ravid, K. (2012). A2B adenosine receptor promotes mesenchymal stem cell differentiation to osteoblasts and bone formation in vivo. J. Biol. Chem. 287, 15718–15727. doi: 10.1074/jbc.M112.344994
Cerino, G., Gaudiello, E., Muraro, M. G., Eckstein, F., Martin, I., Scherberich, A., et al. (2017). Engineering of an angiogenic niche by perfusion culture of adipose-derived stromal vascular fraction cells. Sci. Rep. 7:14252. doi: 10.1038/s41598-017-13882-3
Chazenbalk, G., Bertolotto, C., Heneidi, S., Jumabay, M., Trivax, B., Aronowitz, J., et al. (2011). Novel pathway of adipogenesis through cross-talk between adipose tissue macrophages, adipose stem cells and adipocytes: evidence of cell plasticity. PLoS One 6:e17834. doi: 10.1371/journal.pone.0017834
Chen, L., Wang, L., Li, Y., Wuang, L., Liu, Y., Pang, N., et al. (2018). Transplantation of normal adipose tissue improves blood flow and reduces inflammation in high fat fed mice with Hindlimb Ischemia. Front. Physiol. 9:197. doi: 10.3389/fphys.2018.00197
Chen, Y., Ramalingam, L., Wu, J., Moustaid-Moussa, N., and Li, W. (2017). An integrated biomimetic adipose tissue microchip. FASEB J. 31(1 Suppl.):590.4. doi: 10.1096/fasebj.31.1_supplement.590.4
Chi, K., Fu, R. H., Huang, Y. C., Chen, S. Y., Hsu, C. J., Lin, S. Z., et al. (2018). Adipose-derived stem cells stimulated with n-butylidenephthalide exhibit therapeutic effects in a mouse model of Parkinson’s disease. Cell Transpl. 27, 456–470. doi: 10.1177/0963689718757408
Choi, H. Y., Saha, S. K., Kim, K., Kim, S., Yang, G. -M., Kim, B., et al. (2015). G protein-coupled receptors in stem cell maintenance and somatic reprogramming to pluripotent or cancer stem cells. BMB Rep. 48, 68–80. doi: 10.5483/BMBRep.2015.48.2.250
Choi, Y. S., Dusting, G. J., Stubbs, S., Arunothayaraj, S., Han, X. L., Collas, P., et al. (2010). Differentiation of human adipose-derived stem cells into beating cardiomyocytes. J. Cell Mol. Med. 14, 878–889. doi: 10.1111/j.1582-4934.2010.01009.x
Coelho, M., Oliveira, T., and Fernandes, R. (2013). Biochemistry of adipose tissue: an endocrine organ. Arch. Med. Sci. 9, 191–200. doi: 10.5114/aoms.2013.33181
Costa, M., Cerqueira, M. T., Santos, T. C., Sampaio-Marques, B., Ludovico, P., Marques, A. P., et al. (2017). Cell sheet engineering using the stromal vascular fraction of adipose tissue as a vascularization strategy. Acta Biomater. 55, 131–143. doi: 10.1016/j.actbio.2017.03.034
de Oliveira Bravo, M., Carvalho, J. L., and Saldanha-Araujo, F. (2016). Adenosine production: a common path for mesenchymal stem-cell and regulatory T-cell-mediated immunosuppression. Purinerg. Signal. 12, 595–609. doi: 10.1007/s11302-016-9529-0
Diehl-Wiesenecker, E., von Armin, C. A., Dupuis, L., Muller, H. P., Ludolph, A. C., and Kassubek, J. (2015). Adipose tissue distribution in patients with Alzheimer’s disease: a whole body MRI case-control study. J. Alzheimers Dis. 48, 825–832. doi: 10.3233/jad-150426
Dongó, E., Benkő, Z., Csizmazia,Á., Marosi, G., Grottke, A., Jücker, M., et al. (2014). H2S preconditioning of human adipose tissue-derived stem cells increases their efficacy in an in vitro model of cell therapy for simulated ischemia. Life Sci. 113, 14–21. doi: 10.1016/j.lfs.2014.07.023
Donninelli, G., Del Cornò, M., Pierdominici, M., Scazzocchio, B., Varì, R., Varano, B., et al. (2017). Distinct blood and visceral adipose tissue regulatory T Cell and innate lymphocyte profiles characterize obesity and colorectal cancer. Front. Immunol. 8:643. doi: 10.3389/fimmu.2017.00643
Drehmer, D. L., de Aguiar, A. M., Brandt, A. P., Petiz, L., Cadena, S. M. S. C., Rebelatto, C. K., et al. (2016). Metabolic switches during the first steps of adipogenic stem cells differentiation. Stem Cell Res. 17, 413–421. doi: 10.1016/j.scr.2016.09.001
Eisenstein, A., and Ravid, K. (2014). G protein-coupled receptors and adipogenesis: a focus on adenosine receptors. J. Cell. Physiol. 229, 414–421. doi: 10.1002/jcp.24473
Ergun, S., Tilki, D., and Klein, D. (2011). Vascular wall as a reservoir for different types of stem and progenitor cells. Antioxid. Redox Signal. 15, 981–995. doi: 10.1089/ars.2010.3507
Faris, R. J., Walker-Daniels, J., Jones, D. E., and Spurlock, M. E. (2012). Characterization of swine adipose tissue monocytic lineage cells. FASEB J. 26(1 Suppl.):819.42. doi: 10.1096/fasebj.26.1_supplement.819.42
Ferng, A. S., Marsh, K. M., Fleming, J. M., Conway, R. F., Schipper, D., Bajaj, N., et al. (2016). Adipose-derived human stem/stromal cells: comparative organ specific mitochondrial bioenergy profiles. Springerplus 5:2057. doi: 10.1186/s40064-016-3712-1
Freiman, A., Shandalov, Y., Rozenfeld, D., Shor, E., Segal, S., Ben-David, D., et al. (2016). Adipose-derived endothelial and mesenchymal stem cells enhance vascular network formation on three-dimensional constructs in vitro. Stem Cell Res. Ther. 7:5. doi: 10.1186/s13287-015-0251-6
Frese, L., Dijkman, P. E., and Hoerstrup, S. P. (2016). Adipose Tissue-Derived Stem Cells in Regenerative Medicine. Transfus Med Hemother 43, 268-274. doi: 10.1159/000448180
Fu, Y., Li, R., Zhong, J., Fu, N., Wei, X., Cun, X., et al. (2014). Adipogenic differentiation potential of adipose-derived mesenchymal stem cells from ovariectomized mice. Cell Prolif. 47, 604–614. doi: 10.1111/cpr.12131
Gharibi, B., Abraham, A. A., Ham, J., and Evans, B. A. (2011). Adenosine receptor subtype expression and activation influence the differentiation of mesenchymal stem cells to osteoblasts and adipocytes. J. Bone Miner. Res. 26, 2112–2124. doi: 10.1002/jbmr.424
Gnad, T., Scheibler, S., von Kugelgen, I., Scheele, C., Kilic, A., Glode, A., et al. (2014). Adenosine activates brown adipose tissue and recruits beige adipocytes via A2A receptors. Nature 516, 395–399. doi: 10.1038/nature13816
Gollasch, M. (2017). Adipose-vascular coupling and potential therapeutics. Annu. Rev. Pharmacol. Toxicol. 57, 417–436. doi: 10.1146/annurev-pharmtox-010716-104542
Gollasch, M., and Dubrovska, G. (2004). Paracrine role for periadventitial adipose tissue in the regulation of arterial tone. Trends Pharmacol. Sci. 25, 647–653. doi: 10.1016/j.tips.2004.10.005
Gollasch, M., Welsh, D. G., and Schubert, R. (2018). Perivascular adipose tissue and the dynamic regulation of Kv 7 and Kir channels: implications for resistant hypertension. Microcirculation 25:e12434 doi: 10.1111/micc.12434
Grudzenski, S., Baier, S., Ebert, A., Pullens, P., Lemke, A., Bieback, K., et al. (2017). The effect of adipose tissue-derived stem cells in a middle cerebral artery occlusion stroke model depends on their engraftment rate. Stem Cell Res. Ther. 8:96. doi: 10.1186/s13287-017-0545-y
Guilherme, A., Pedersen, D. J., Henchey, E., Henriques, F. S., Danai, L. V., Shen, Y., et al. (2017). Adipocyte lipid synthesis coupled to neuronal control of thermogenic programming. Mol. Metab. 6, 781–796. doi: 10.1016/j.molmet.2017.05.012
Gutiérrez-Fernández, M., Rodríguez-Frutos, B., Ramos-Cejudo, J., Teresa Vallejo-Cremades, M., Fuentes, B., Cerdán, S., et al. (2013). Effects of intravenous administration of allogenic bone marrow- and adipose tissue-derived mesenchymal stem cells on functional recovery and brain repair markers in experimental ischemic stroke. Stem Cell Res. Ther. 4:11. doi: 10.1186/scrt159
Ha, S., Ahn, S., Kim, S., Joo, Y., Chong, Y.H., Suh, Y.H., et al. (2014). In vivo imaging of human adipose-derived stem cells in Alzheimer’s disease animal model. J. Biomed. Opt. 19:051206. doi: 10.1117/1.jbo.19.5.051206
Han, S., Sun, H. M., Hwang, K. C., and Kim, S. W. (2015). Adipose-derived stromal vascular fraction cells: update on clinical utility and efficacy. Crit. Rev. Eukaryot. Gene Exp. 25, 145–152.
Hildebrand, S., Stumer, J., and Pfeifer, A. (2018). PVAT and its relation to brown, beige, and white adipose tissue in development and function. Front. Physiol. 9:70. doi: 10.3389/fphys.2018.00070
Hong, S. J., Hou, D., Brinton, T. J., Johnstone, B., Feng, D., Rogers, P., et al. (2014). Intracoronary and retrograde coronary venous myocardial delivery of adipose-derived stem cells in swine infarction lead to transient myocardial trapping with predominant pulmonary redistribution. Catheteriz. Cardiovasc. Intervent. 83, E17–E25. doi: 10.1002/ccd.24659
Horenstein, A. L., Chillemi, A., Zaccarello, G., Bruzzone, S., Quarona, V., Zito, A., et al. (2013). A CD38/CD203a/CD73 ectoenzymatic pathway independent of CD39 drives a novel adenosinergic loop in human T lymphocytes. Oncoimmunology 2:e26246. doi: 10.4161/onci.26246
Horimatsu, T., Kim, H. W., and Weintraub, N. L. (2017). The role of perivascular adipose tissue in non-atherosclerotic vascular disease. Front. Physiol. 8:969. doi: 10.3389/fphys.2017.00969
Hsueh, Y. Y., Chang, Y. J., Huang, C. W., Handayani, F., Chiang, Y. L., Fan, S. C., et al. (2015). Synergy of endothelial and neural progenitor cells from adipose-derived stem cells to preserve neurovascular structures in rat hypoxic-ischemic brain injury. Sci. Rep. 5:14985. doi: 10.1038/srep14985
Hunt, T. K., Aslam, R. S., Beckert, S., Wagner, S., Ghani, Q. P., Hussain, M. Z., et al. (2007). Aerobically derived lactate stimulates revascularization and tissue repair via redox mechanisms. Antioxid. Redox Signal. 9, 1115–1124. doi: 10.1089/ars.2007.1674
Ishii, M., and Iadecola, C. (2016). Adipocyte-derived factors in age-related dementia and their contribution to vascular and Alzheimer pathology. Biochim. Biophys. Acta 1862, 966–974. doi: 10.1016/j.bbadis.2015.10.029
Jeong, J. H., Chang, J. S., and Jo, Y. -H. (2018). Intracellular glycolysis in brown adipose tissue is essential for optogenetically induced nonshivering thermogenesis in mice. Sci. Rep. 8:6672. doi: 10.1038/s41598-018-25265-3
Jiang, Y., Berry, D. C., Jo, A., Tang, W., Arpke, R. W., Kyba, M., et al. (2017). A PPARγ transcriptional cascade directs adipose progenitor cell-niche interaction and niche expansion. Nat. Commun. 8:15926. doi: 10.1038/ncomms15926
Jiang, Y., Berry, D. C., Tang, W., and Graff, J. M. (2014). Independent stem cell lineages regulate adipose organogenesis and adipose homeostasis. Cell Rep. 9, 1007–1022. doi: 10.1016/j.celrep.2014.09.049
Jing, L., Tamplin, O. J., Chen, M. J., Deng, Q., Patterson, S., Kim, P. G., et al. (2015). Adenosine signaling promotes hematopoietic stem and progenitor cell emergence. J. Exp. Med. 212, 649–663. doi: 10.1084/jem.20141528
Jokinen, R., Pirnes-Karhu, S., Pietiläinen, K. H., and Pirinen, E. (2017). Adipose tissue NAD(+)-homeostasis, sirtuins and poly(ADP-ribose) polymerases -important players in mitochondrial metabolism and metabolic health. Redox Biol. 12, 246–263. doi: 10.1016/j.redox.2017.02.011
Joo, H. J., Kim, J. H., and Hong, S. J. (2017). Adipose tissue-derived stem cells for myocardial regeneration. Korean Circ. J. 47, 151–159. doi: 10.4070/kcj.2016.0207
Katsuda, T., Oki, K., and Ochiya, T. (2015). Potential application of extracellular vesicles of human adipose tissue-derived mesenchymal stem cells in Alzheimer’s disease therapeutics. Methods Mol. Biol. 1212, 171–181. doi: 10.1007/7651_2014_98
Katsuda, T., Tsuchiya, R., Kosaka, N., Yoshioka, Y., Takagaki, K., Oki, K., et al. (2013). Human adipose tissue-derived mesenchymal stem cells secrete functional neprilysin-bound exosomes. Sci. Rep. 3:1197. doi: 10.1038/srep01197
Kester, M. I., Goos, J. D., Teunissen, C. E., Benedictus, M. R., Bouwman, F. H., Wattjes, M. P., et al. (2014). Associations between cerebral small-vessel disease and Alzheimer disease pathology as measured by cerebrospinal fluid biomarkers. JAMA Neurol. 71, 855–862. doi: 10.1001/jamaneurol.2014.754
Kheterpal, I., Ku, G., Coleman, L., Yu, G., Ptitsyn, A. A., Floyd, Z. E., et al. (2011). Proteome of human subcutaneous adipose tissue stromal vascular fraction cells versus mature adipocytes based on DIGE. J. Proteome Res. 10, 1519–1527. doi: 10.1021/pr100887r
Kim, J. H., Choi, S. C., Park, C. Y., Park, J. H., Choi, J. H., Joo, H. J., et al. (2016). Transplantation of immortalized CD34+ and CD34- Adipose-derived stem cells improve cardiac function and mitigate systemic pro-inflammatory responses. PLoS One 11:e0147853. doi: 10.1371/journal.pone.0147853
Kim, W. S., Han, J., Hwang, S. J., and Sung, J. H. (2014). An update on niche composition, signaling and functional regulation of the adipose-derived stem cells. Expert Opin. Biol. Ther. 14, 1091–1102. doi: 10.1517/14712598.2014.907785
Klar, A. S., Guven, S., Zimoch, J., Zapiorkowska, N. A., Biedermann, T., Bottcher-Haberzeth, S., et al. (2016). Characterization of vasculogenic potential of human adipose-derived endothelial cells in a three-dimensional vascularized skin substitute. Pediatr. Surg. Int. 32, 17–27. doi: 10.1007/s00383-015-3808-7
Knezevic, L., Schaupper, M., Muhleder, S., Schimek, K., Hasenberg, T., Marx, U., et al. (2017). Engineering blood and lymphatic microvascular networks in fibrin matrices. Front. Bioeng. Biotechnol. 5:25. doi: 10.3389/fbioe.2017.00025
Koh, Y. J., Kang, S., Lee, H. J., Choi, T. S., Lee, H. S., Cho, C. H., et al. (2007). Bone marrow-derived circulating progenitor cells fail to transdifferentiate into adipocytes in adult adipose tissues in mice. J. Clin. Invest. 117, 3684–3695. doi: 10.1172/jci32504
Kong, L. R., Zhou, Y. P., Chen, D. R., Ruan, C. C., and Gao, P. J. (2018). Decrease of perivascular adipose tissue browning is associated with vascular dysfunction in spontaneous hypertensive rats during aging. Front. Physiol. 9:400. doi: 10.3389/fphys.2018.00400
Kook, Y. M., Kim, H., Kim, S., Heo, C. Y., Park, M. H., Lee, K., et al. (2018). Promotion of vascular morphogenesis of endothelial cells co-cultured with human adipose-derived mesenchymal stem cells using polycaprolactone/gelatin nanofibrous scaffolds. Nanomaterials 8:E117. doi: 10.3390/nano8020117
Lee, H. W., Lee, H. C., Park, J. H., Kim, B. W., Ahn, J., Kim, J. H., et al. (2015). Effects of intracoronary administration of autologous adipose tissue-derived stem cells on acute myocardial infarction in a porcine model. Yonsei Med. J. 56, 1522–1529. doi: 10.3349/ymj.2015.56.6.1522
Lee, J., Abdeen, A. A., Tang, X., Saif, T. A., and Kilian, K. A. (2016). Matrix directed adipogenesis and neurogenesis of mesenchymal stem cells derived from adipose tissue and bone marrow. Acta Biomater. 42, 46–55. doi: 10.1016/j.actbio.2016.06.037
Lee, M., Ban, J. J., Yang, S., Im, W., and Kim, M. (2018). The exosome of adipose-derived stem cells reduces beta-amyloid pathology and apoptosis of neuronal cells derived from the transgenic mouse model of Alzheimer’s disease. Brain Res. 1691, 87–93. doi: 10.1016/j.brainres.2018.03.034
Lee, Y. H., Petkova, A. P., and Granneman, J. G. (2013). Identification of an adipogenic niche for adipose tissue remodeling and restoration. Cell Metab. 18, 355–367. doi: 10.1016/j.cmet.2013.08.003
Lee, Y. H., Petkova, A. P., Konkar, A. A., and Granneman, J. G. (2015). Cellular origins of cold-induced brown adipocytes in adult mice. FASEB J. 29, 286–299. doi: 10.1096/fj.14-263038
Lee, Y. H., Petkova, A. P., Mottillo, E. P., and Granneman, J. G. (2012). In vivo identification of bipotential adipocyte progenitors recruited by beta3-adrenoceptor activation and high-fat feeding. Cell Metab. 15, 480–491. doi: 10.1016/j.cmet.2012.03.009
Lee, Z. W., Teo, X. Y., Tay, E. Y., Tan, C. H., Hagen, T., Moore, P. K., et al. (2014). Utilizing hydrogen sulfide as a novel anti-cancer agent by targeting cancer glycolysis and pH imbalance. Br. J. Pharmacol. 171, 4322–4336. doi: 10.1111/bph.12773
Li, X., and Easley, C. J. (2018). Microfluidic systems for studying dynamic function of adipocytes and adipose tissue. Anal. Bioanal. Chem. 410, 791–800. doi: 10.1007/s00216-017-0741-8
Liu, C., Wu, J., Zhu, J., Kuei, C., Yu, J., Shelton, J., et al. (2009). Lactate inhibits lipolysis in fat cells through activation of an orphan G-protein-coupled receptor, GPR81. J. Biol. Chem. 284, 2811–2822. doi: 10.1074/jbc.M806409200
Lopatina, O., Inzhutova, A., Salmina, A. B., and Higashida, H. (2012). The roles of oxytocin and CD38 in social or parental behaviors. Front. Neurosci. 6:182. doi: 10.3389/fnins.2012.00182
Loskill, P., Mathur, A., Conklin, B. R., Stahl, A., Lee, L., and Healy, K. E. (2016). Organs-on-a-chip – Microphysiological platforms as in vitro models of cardiac and adipose tissue. Toxicol. Lett. 258;S153. doi: 10.1016/j.toxlet.2016.06.1586
Loskill, P., Sezhian, T., Tharp, K. M., Lee-Montiel, F. T., Jeeawoody, S., Reese, W. M., et al. (2017). WAT-on-a-chip: a physiologically relevant microfluidic system incorporating white adipose tissue. Lab Chip 17, 1645–1654. doi: 10.1039/c6lc01590e
Luna, A. C. L., Madeira, M. E. P., Conceição, T. O., Moreira, J. A. L. C., Laiso, R. A. N., and Maria, D. A. (2014). Characterization of adipose-derived stem cells of anatomical region from mice. BMC Res. Notes 7:552. doi: 10.1186/1756-0500-7-552
Lynch, F. M., Withers, S. B., Yao, Z., Werner, M. E., Edwards, G., Weston, A. H., et al. (2013). Perivascular adipose tissue-derived adiponectin activates BK(Ca) channels to induce anticontractile responses. Am. J. Physiol. Heart Circ. Physiol. 304, H786–795. doi: 10.1152/ajpheart.00697.2012
Majesky, M. W., Dong, X. R., Hoglund, V., Daum, G., and Mahoney, W. M. (2011). The adventitia: a progenitor cell niche for the vessel wall. Cells Tissues Organs 195, 73–81. doi: 10.1159/000331413
Malavasi, F., Deaglio, S., Funaro, A., Ferrero, E., Horenstein, A. L., Ortolan, E., et al. (2008). Evolution and function of the ADP ribosyl cyclase/CD38 gene family in physiology and pathology. Physiol. Rev. 88, 841–886. doi: 10.1152/physrev.00035.2007
Malinovskaya, N. A., Komleva, Y. K., Salmin, V. V., Morgun, A. V., Shuvaev, A. N., Panina, Y. A., et al. (2016). Endothelial progenitor cells physiology and metabolic plasticity in brain angiogenesis and blood-brain barrier modeling. Front. Physiol. 7:599. doi: 10.3389/fphys.2016.00599
Mariani, S., Di Rocco, G., Toietta, G., Russo, M. A., Petrangeli, E., and Salvatori, L. (2017). Sirtuins 1-7 expression in human adipose-derived stem cells from subcutaneous and visceral fat depots: influence of obesity and hypoxia. Endocrine 57, 455–463. doi: 10.1007/s12020-016-1170-8
Merfeld-Clauss, S., Lupov, I. P., Lu, H., March, K. L., and Traktuev, D. O. (2015). Adipose stromal cell contact with endothelial cells results in loss of complementary vasculogenic activity mediated by induction of activin A. Stem Cells 33, 3039–3051. doi: 10.1002/stem.2074
Mildmay-White, A., and Khan, W. (2017). Cell surface markers on adipose-derived stem cells: a systematic review. Curr. Stem Cell Res. Ther. 12, 484–492. doi: 10.2174/1574888x11666160429122133
Murohara, T. (2009). Autologous adipose tissue as a new source of progenitor cells for therapeutic angiogenesis. J. Cardiol. 53, 155–163. doi: 10.1016/j.jjcc.2009.01.003
Nagata, H., Ii, M., Kohbayashi, E., Hoshiga, M., Hanafusa, T., and Asahi, M. (2016). Cardiac adipose-derived stem cells exhibit high differentiation potential to cardiovascular cells in C57BL/6 mice. Stem Cells Transl. Med. 5, 141–151. doi: 10.5966/sctm.2015-0083
Oliva-Olivera, W., Coin-Araguez, L., Lhamyani, S., Clemente-Postigo, M., Torres, J. A., Bernal-Lopez, M. R., et al. (2017). Adipogenic impairment of adipose tissue-derived mesenchymal stem cells in subjects with metabolic syndrome: possible protective role of FGF2. J. Clin. Endocrinol. Metab. 102, 478–487. doi: 10.1210/jc.2016-2256
Ong, W. K., Tan, C. S., Chan, K. L., Goesantoso, G. G., Chan, X. H., Chan, E., et al. (2014). Identification of specific cell-surface markers of adipose-derived stem cells from subcutaneous and visceral fat depots. Stem Cell Rep. 2, 171–179. doi: 10.1016/j.stemcr.2014.01.002
Onogi, Y., Wada, T., Kamiya, C., Inata, K., Matsuzawa, T., Inaba, Y., et al. (2017). PDGFRbeta regulates adipose tissue expansion and glucose metabolism via vascular remodeling in diet-induced obesity. Diabetes Metab. Res. Rev. 66, 1008–1021. doi: 10.2337/db16-0881
Park, D., Yang, G., Bae, D. K., Lee, S. H., Yang, Y. H., Kyung, J., et al. (2013). Human adipose tissue-derived mesenchymal stem cells improve cognitive function and physical activity in ageing mice. J. Neurosci. Res. 91, 660–670. doi: 10.1002/jnr.23182
Park, H. J., Kim, J., Bak, S., and Lee, M. (2017). High salt intake induces adipogenesis by the modulation of MAPK/ERK1/2 pathway in both 3T3-L1 adipocytes and co-culture with macrophages. FASEB J. 31(1 Suppl.):947.2. doi: 10.1096/fasebj.31.1_supplement.947.2
Pavesi, A., Soncini, M., Zamperone, A., Pietronave, S., Medico, E., Redaelli, A., et al. (2014). Electrical conditioning of adipose-derived stem cells in a multi-chamber culture platform. Biotechnol. Bioeng. 111, 1452–1463. doi: 10.1002/bit.25201
Petersen, C., Nielsen, M. D., Andersen, E. S., Basse, A. L., Isidor, M. S., Markussen, L. K., et al. (2017). MCT1 and MCT4 expression and lactate flux activity increase during white and brown adipogenesis and impact adipocyte metabolism. Sci. Rep. 7:13101. doi: 10.1038/s41598-017-13298-z
Pierantozzi, E., Badin, M., Vezzani, B., Curina, C., Randazzo, D., Petraglia, F., et al. (2015). Human pericytes isolated from adipose tissue have better differentiation abilities than their mesenchymal stem cell counterparts. Cell Tissue Res. 361, 769–778. doi: 10.1007/s00441-015-2166-z
Planat-Benard, V., Silvestre, J. S., Cousin, B., Andre, M., Nibbelink, M., Tamarat, R., et al. (2004). Plasticity of human adipose lineage cells toward endothelial cells: physiological and therapeutic perspectives. Circulation 109, 656–663. doi: 10.1161/01.cir.0000114522.38265.61
Polet, F., and Feron, O. (2013). Endothelial cell metabolism and tumour angiogenesis: glucose and glutamine as essential fuels and lactate as the driving force. J. Intern. Med. 273, 156–165. doi: 10.1111/joim.12016
Porporato, P. E., Payen, V. L., De Saedeleer, C. J., Preat, V., Thissen, J. P., Feron, O., et al. (2012). Lactate stimulates angiogenesis and accelerates the healing of superficial and ischemic wounds in mice. Angiogenesis 15, 581–592. doi: 10.1007/s10456-012-9282-0
Ratushnyy, A., Ezdakova, M., Yakubets, D., and Buravkova, L. (2018). Angiogenic activity of human adipose-derived mesenchymal stem cells under simulated microgravity. Stem Cells Dev. 27, 831–837. doi: 10.1089/scd.2017.0262
Rezai Rad, M., Bohloli, M., Akhavan Rahnama, M., Anbarlou, A., Nazeman, P., and Khojasteh, A. (2017). Impact of tissue harvesting sites on the cellular behaviors of adipose-derived stem cells: implication for bone tissue engineering. Stem Cells Int. 2017:2156478. doi: 10.1155/2017/2156478
Rittig, K., Dolderer, J. H., Balletshofer, B., Machann, J., Schick, F., Meile, T., et al. (2012). The secretion pattern of perivascular fat cells is different from that of subcutaneous and visceral fat cells. Diabetologia 55, 1514–1525. doi: 10.1007/s00125-012-2481-9
Roberts, L. D., Virtue, S., Vidal-Puig, A., Nicholls, A. W., and Griffin, J. L. (2009). Metabolic phenotyping of a model of adipocyte differentiation. Physiol. Genomics 39, 109–119. doi: 10.1152/physiolgenomics.90365.2008
Rodriguez, R. M., Fernandez, A. F., and Fraga, M. F. (2013). Role of sirtuins in stem cell differentiation. Genes Cancer 4, 105–111. doi: 10.1177/1947601913479798
Rohringer, S., Hofbauer, P., Schneider, K. H., Husa, A. M., Feichtinger, G., Peterbauer-Scherb, A., et al. (2014). Mechanisms of vasculogenesis in 3D fibrin matrices mediated by the interaction of adipose-derived stem cells and endothelial cells. Angiogenesis 17, 921–933. doi: 10.1007/s10456-014-9439-0
Rooney, K., and Trayhurn, P. (2011). Lactate and the GPR81 receptor in metabolic regulation: implications for adipose tissue function and fatty acid utilisation by muscle during exercise. Br. J. Nutr. 106, 1310–1316. doi: 10.1017/s0007114511004673
Sabater, D., Arriarán, S., Romero, M. D. M., Agnelli, S., Remesar, X., Fernández-López, J. A., et al. (2014). Cultured 3T3L1 adipocytes dispose of excess medium glucose as lactate under abundant oxygen availability. Sci. Rep. 4:3663. doi: 10.1038/srep03663
Safford, K. M., Hicok, K. C., Safford, S. D., Halvorsen, Y. D., Wilkison, W. O., Gimble, J. M., et al. (2002). Neurogenic differentiation of murine and human adipose-derived stromal cells. Biochem. Biophys. Res. Commun. 294, 371–379. doi: 10.1016/s0006-291x(02)00469-2
Saggerson, E. D., McAllister, T. W., and Baht, H. S. (1988). Lipogenesis in rat brown adipocytes. Effects of insulin and noradrenaline, contributions from glucose and lactate as precursors and comparisons with white adipocytes. Biochem. J. 251, 701–709.
Salmin, V., Morgun, A., Khilazheva, E., Pisareva, N., Boitsova, E., Lavrentiev, P., et al. (2017). “Secret life of tiny blood vessels: lactate, scaffold and beyond,” in Proceedings of the Bioinformatics and Biomedical Engineering: IWBBIO 2017, Granada, 591–601.
Salmina, A. B., Okuneva, O. S., Malinovskaya, N. A., Zykova, L. D., Fursov, A. A., Morgun, A. V., et al. (2009). Changes in expression and activity of CD38 in astroglial cells after impairment of the neuron-glia relationship in the brain induced by perinatal hypoxia-ischemia. Neurochem. J. 3, 207–213. doi: 10.1134/s181971240903009x
Scherberich, A., Muller, A. M., Schafer, D. J., Banfi, A., and Martin, I. (2010). Adipose tissue-derived progenitors for engineering osteogenic and vasculogenic grafts. J. Cell. Physiol. 225, 348–353. doi: 10.1002/jcp.22313
Schleifenbaum, J., Kohn, C., Voblova, N., Dubrovska, G., Zavarirskaya, O., Gloe, T., et al. (2010). Systemic peripheral artery relaxation by KCNQ channel openers and hydrogen sulfide. J. Hypertens. 28, 1875–1882. doi: 10.1097/HJH.0b013e32833c20d5
Silva, F. J., Holt, D. J., Vargas, V., Yockman, J., Boudina, S., Atkinson, D., et al. (2014). Metabolically active human brown adipose tissue derived stem cells. Stem Cells 32, 572–581. doi: 10.1002/stem.1595
Silva, K. R., Côrtes, I., Liechocki, S., Carneiro, J. R. I., Souza, A. A. P., Borojevic, R., et al. (2017). Characterization of stromal vascular fraction and adipose stem cells from subcutaneous, preperitoneal and visceral morbidly obese human adipose tissue depots. PLoS One 12:e0174115. doi: 10.1371/journal.pone.0174115
Silva, K. R., Liechocki, S., Carneiro, J. R., Claudio-da-Silva, C., Maya-Monteiro, C. M., Borojevic, R., et al. (2015). Stromal-vascular fraction content and adipose stem cell behavior are altered in morbid obese and post bariatric surgery ex-obese women. Stem Cell Res. Ther. 6:72. doi: 10.1186/s13287-015-0029-x
Sullivan, M. O., Gordon-Evans, W. J., Fredericks, L. P., Kiefer, K., Conzemius, M. G., and Griffon, D. J. (2015). Comparison of mesenchymal stem cell surface markers from bone marrow aspirates and adipose stromal vascular fraction sites. Front. Vet. Sci. 2:82. doi: 10.3389/fvets.2015.00082
Sun, A. J., Qiao, L., Huang, C., Zhang, X., Li, Y. Q., and Yang, X. Q. (2018). Comparison of mouse brown and white adipose-derived stem cell differentiation into pacemaker-like cells induced by TBX18 transduction. Mol. Med. Rep. 17, 7055–7064. doi: 10.3892/mmr.2018.8792
Suzuki, E., Fujita, D., Takahashi, M., Oba, S., and Nishimatsu, H. (2015). Adipose tissue-derived stem cells as a therapeutic tool for cardiovascular disease. World J. Cardiol. 7, 454–465. doi: 10.4330/wjc.v7.i8.454
Tanataweethum, N., Yang, F., Zelaya, A., Karnik, S., Cohen, R. N., Brey, E., et al. (2017). Differentiation of primary pre-adipocytes into adipose tissue in a microfluidic chip. FASEB J. 31(1 Suppl.):886.8. doi: 10.1096/fasebj.31.1_supplement.886.8
Tomkowicz, B., Rybinski, K., Sebeck, D., Sass, P., Nicolaides, N. C., Grasso, L., et al. (2010). Endosialin/TEM-1/CD248 regulates pericyte proliferation through PDGF receptor signaling. Cancer Biol. Ther. 9, 908–915.
Toyserkani, N. M., Jørgensen, M. G., Tabatabaeifar, S., Jensen, C. H., Sheikh, S. P., and Sørensen, J. A. (2017). Concise review: a safety assessment of adipose-derived cell therapy in clinical trials: a systematic review of reported adverse events. Stem Cells Transl. Med. 6, 1786–1794. doi: 10.1002/sctm.17-0031
Tozzi, M., and Novak, I. (2017). Purinergic receptors in adipose tissue as potential targets in metabolic disorders. Front. Pharmacol. 8:878. doi: 10.3389/fphar.2017.00878
Tran, K. V., Fitzgibbons, T., Min, S. Y., DeSouza, T., and Corvera, S. (2018). Distinct adipocyte progenitor cells are associated with regional phenotypes of perivascular aortic fat in mice. Mol. Metab. 9, 199–206. doi: 10.1016/j.molmet.2017.12.014
Tran, K. V., Gealekman, O., Frontini, A., Zingaretti, M. C., Morroni, M., Giordano, A., et al. (2012). The vascular endothelium of the adipose tissue give rise to both white and brown fat cells. Cell Metab. 15, 222–229. doi: 10.1016/j.cmet.2012.01.008
Ubil, E., Duan, J., Pillai, I. C. L., Rosa-Garrido, M., Wu, Y., Bargiacchi, F., et al. (2014). Mesenchymal–endothelial transition contributes to cardiac neovascularization. Nature 514, 585–590. doi: 10.1038/nature13839
Valina, C., Pinkernell, K., Song, Y. H., Bai, X., Sadat, S., Campeau, R. J., et al. (2007). Intracoronary administration of autologous adipose tissue-derived stem cells improves left ventricular function, perfusion, and remodelling after acute myocardial infarction. Eur. Heart J. 28, 2667–2677. doi: 10.1093/eurheartj/ehm426
Van Pham, P., Vu, N. B., Nguyen, H. T., and Phan, N. K. (2016). Isolation of endothelial progenitor cells from human adipose tissue. Biomed. Res. Ther. 3, 645–652. doi: 10.7603/s40730-016-0024-6
Vergnes, L., and Reue, K. (2014). Adaptive thermogenesis in white adipose tissue: is lactate the new brown(ing)? Diabetes 63, 3175–3176. doi: 10.2337/db14-0815
Voros, G., Maquoi, E., Demeulemeester, D., Clerx, N., Collen, D. S., and Lijnen, H. R. (2005). Modulation of angiogenesis during adipose tissue development in murine models of obesity. Endocrinology 146, 4545–4554. doi: 10.1210/en.2005-0532
Wadhawan, N., Kalkat, H., Natarajan, K., Ma, X., Gajjeraman, S., Andalur Nandagopal, S., et al. (2012). Growth and positioning of adipose-derived stem cells in microfluidic devices. Lab Chip 12, 4829–4834. doi: 10.1039/c2lc40891k
Wang, G. X., Zhao, X. Y., and Lin, J. D. (2015). The brown fat secretome: metabolic functions beyond thermogenesis. Trends Endrocrinol. Metab. 26, 231–237. doi: 10.1016/j.tem.2015.03.002
Wang, L., Hu, J., Sorek, C. E., Chen, E. Y., Ma, P. X., and Yang, B. (2016). Fabrication of tissue-engineered vascular grafts with stem cells and stem cell-derived vascular cells. Expert Opin. Biol. Ther. 16, 317–330. doi: 10.1517/14712598.2016.1118460
Wang, L. F., Miao, L. J., Wang, X. N., Huang, C. C., Qian, Y. S., Huang, X., et al. (2018). CD38 deficiency suppresses adipogenesis and lipogenesis in adipose tissues through activating Sirt1/PPARgamma signaling pathway. J. Cell Mol. Med. 22, 101–110. doi: 10.1111/jcmm.13297
Wang, Y., Yin, P., Bian, G. L., Huang, H. Y., Shen, H., Yang, J. J., et al. (2017). The combination of stem cells and tissue engineering: an advanced strategy for blood vessels regeneration and vascular disease treatment. Stem Cell Res. Ther. 8:194. doi: 10.1186/s13287-017-0642-y
Wosnitza, M., Hemmrich, K., Groger, A., Gräber, S., and Pallua, N. (2007). Plasticity of human adipose stem cells to perform adipogenic and endothelial differentiation. Differentiation 75, 12–23. doi: 10.1111/j.1432-0436.2006.00110.x
Yamaguchi, Y., Cavallero, S., Patterson, M., Shen, H., Xu, J., Kumar, S. R., et al. (2015). Adipogenesis and epicardial adipose tissue: a novel fate of the epicardium induced by mesenchymal transformation and PPARγ activation. Proc. Natl. Acad. Sci. U.S.A. 112, 2070–2075. doi: 10.1073/pnas.1417232112
Yan, Y., Ma, T., Gong, K., Ao, Q., Zhang, X., and Gong, Y. (2014). Adipose-derived mesenchymal stem cell transplantation promotes adult neurogenesis in the brains of Alzheimer’s disease mice. Neural Regen. Res. 9, 798–805. doi: 10.4103/1673-5374.131596
Yang, J. P., Anderson, A. E., McCartney, A., Ory, X., Ma, G., Pappalardo, E., et al. (2017). Metabolically active three-dimensional brown adipose tissue engineered from white adipose-derived stem cells. Tissue Eng. Part A 23, 253–262. doi: 10.1089/ten.TEA.2016.0399
Yang, M., Zhang, G. G., Wang, T., Wang, X., Tang, Y. H., Huang, H., et al. (2016). TBX18 gene induces adipose-derived stem cells to differentiate into pacemaker-like cells in the myocardial microenvironment. Int. J. Mol. Med. 38, 1403–1410. doi: 10.3892/ijmm.2016.2736
Yang, Y., Cai, Y., Zhang, Y., Liu, J., and Xu, Z. (2018). Exosomes secreted by adipose-derived stem cells contribute to angiogenesis of brain microvascular endothelial cells following oxygen–glucose deprivation in vitro through MicroRNA-181b/TRPM7 Axis. J. Mol. Neurosci. 65, 74–83. doi: 10.1007/s12031-018-1071-9
Zaborska, K. E., Wareing, M., Edwards, G., and Austin, C. (2016). Loss of anti-contractile effect of perivascular adipose tissue in offspring of obese rats. Int. J. Obes. 40, 1205–1214. doi: 10.1038/ijo.2016.62
Zannettino, A. C., Paton, S., Arthur, A., Khor, F., Itescu, S., Gimble, J. M., et al. (2008). Multipotential human adipose-derived stromal stem cells exhibit a perivascular phenotype in vitro and in vivo. J. Cell. Physiol. 214, 413–421. doi: 10.1002/jcp.21210
Zavan, B., Vindigni, V., Gardin, C., D’Avella, D., Della Puppa, A., Abatangelo, G., et al. (2010). Neural potential of adipose stem cells. Discov. Med. 10, 37–43.
Zemelko, V. I., Kozhucharova, I. V., Kovaleva, Z. V., Domnina, A. P., Pugovkina, N. A., Fridlyanskaya, I. I., et al. (2014). Brain-derived neurotrofic factor (BDNF) secretion of human mesenchymal stem cells isolated from bone marrow, endometrium and adipose tissue. Cell Tissue Biol. 8, 283–291. doi: 10.1134/s1990519x14040129
Zhang, J., Wei, X., Zeng, R., Xu, F., and Li, X. (2017). Stem cell culture and differentiation in microfluidic devices toward organ-on-a-chip. Future Sci. OA 3:FSO187. doi: 10.4155/fsoa-2016-0091
Zheng, C. X., Sui, B. D., Liu, N., Hu, C. H., He, T., Zhang, X. Y., et al. (2018). Adipose mesenchymal stem cells from osteoporotic donors preserve functionality and modulate systemic inflammatory microenvironment in osteoporotic cytotherapy. Sci. Rep. 8:5215. doi: 10.1038/s41598-018-23098-8
Zhou, J., and Qin, G. (2012). Adipocyte dysfunction and hypertension. Am. J. Cardiovasc. Dis. 2, 143–149.
Zhou, L., Xia, J., Qiu, X., Wang, P., Jia, R., Chen, Y., et al. (2015). In Vitro evaluation of endothelial progenitor cells from adipose tissue as potential angiogenic cell sources for bladder angiogenesis. PLoS One 10:e0117644. doi: 10.1371/journal.pone.0117644
Zielins, E. R., Januszyk, M., Luan, A., Brett, E. A., Paik, K., Walmsley, G. G., et al. (2015). Microfluidic single cell transcriptional analysis reveals subpopulations of adipose derived stromal cells with enhanced angiogenic potential. J. Am. Coll. Surg. 221:e26. doi: 10.1016/j.jamcollsurg.2015.08.366
Keywords: adipose tissue, adipocyte, stem cell, endothelial cells, angiogenesis, vasculature-on-chip
Citation: Panina YA, Yakimov AS, Komleva YK, Morgun AV, Lopatina OL, Malinovskaya NA, Shuvaev AN, Salmin VV, Taranushenko TE and Salmina AB (2018) Plasticity of Adipose Tissue-Derived Stem Cells and Regulation of Angiogenesis. Front. Physiol. 9:1656. doi: 10.3389/fphys.2018.01656
Received: 04 August 2018; Accepted: 02 November 2018;
Published: 26 November 2018.
Edited by:
Maik Gollasch, Charité – Universitätsmedizin Berlin, GermanyReviewed by:
Yasuhiko Yamamoto, Kanazawa University, JapanDmitry Tsvetkov, University of Tübingen, Germany
Copyright © 2018 Panina, Yakimov, Komleva, Morgun, Lopatina, Malinovskaya, Shuvaev, Salmin, Taranushenko and Salmina. This is an open-access article distributed under the terms of the Creative Commons Attribution License (CC BY). The use, distribution or reproduction in other forums is permitted, provided the original author(s) and the copyright owner(s) are credited and that the original publication in this journal is cited, in accordance with accepted academic practice. No use, distribution or reproduction is permitted which does not comply with these terms.
*Correspondence: Alla B. Salmina, YWxsYXNhbG1pbmFAbWFpbC5ydQ==