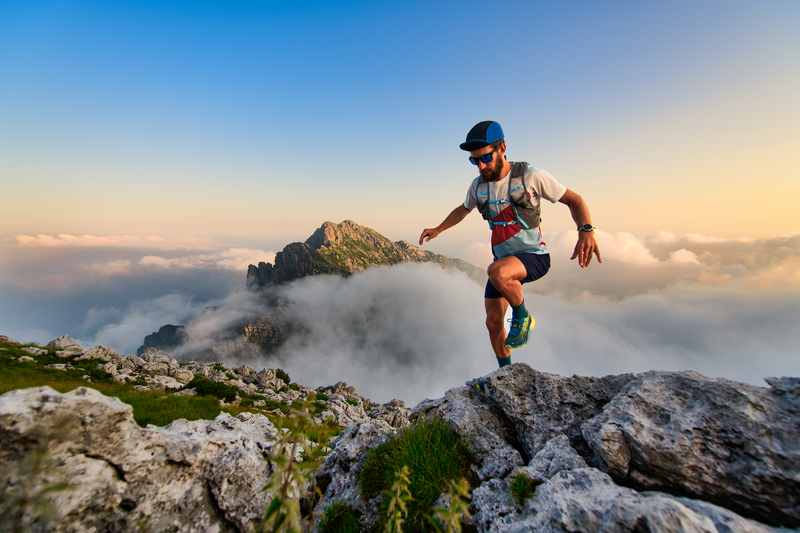
94% of researchers rate our articles as excellent or good
Learn more about the work of our research integrity team to safeguard the quality of each article we publish.
Find out more
ORIGINAL RESEARCH article
Front. Physiol. , 17 August 2018
Sec. Integrative Physiology
Volume 9 - 2018 | https://doi.org/10.3389/fphys.2018.01122
The aim of the present work was to study the consequences of chronic exercise training on factors involved in the regulation of mitochondrial remodeling and biogenesis, as well as the ability to produce energy and improve insulin sensitivity and glucose uptake in rat brown adipose tissue (BAT). Male Wistar rats were divided into two groups: (1) control group (C; n = 10) and (2) exercise-trained rats (ET; n = 10) for 8 weeks on a motor treadmill (five times per week for 50 min). Exercise training reduced body weight, plasma insulin, and oxidized LDL concentrations. Protein expression of ATP-independent metalloprotease (OMA1), short optic atrophy 1 (S-OPA1), and dynamin-related protein 1 (DRP1) in BAT increased in trained rats, and long optic atrophy 1 (L-OPA1) and mitofusin 1 (MFN1) expression decreased. BAT expression of nuclear respiratory factor type 1 (NRF1) and mitochondrial transcription factor A (TFAM), the main factors involved in mitochondrial biogenesis, was higher in trained rats compared to controls. Exercise training increased protein expression of sirtuin 1 (SIRT1), peroxisome proliferator-activated receptor γ coactivator 1α (PGC1α) and AMP-activated protein kinase (pAMPK/AMPK ratio) in BAT. In addition, training increased carnitine palmitoyltransferase II (CPT II), mitochondrial F1 ATP synthase α-chain, mitochondrial malate dehydrogenase 2 (mMDH) and uncoupling protein (UCP) 1,2,3 expression in BAT. Moreover, exercise increased insulin receptor (IR) ratio (IRA/IRB ratio), IRA-insulin-like growth factor 1 receptor (IGF-1R) hybrids and p42/44 activation, and decreased IGF-1R expression and IR substrate 1 (p-IRS-1) (S307) indicating higher insulin sensitivity and favoring glucose uptake in BAT in response to chronic exercise training. In summary, the present study indicates that chronic exercise is able to improve the energetic profile of BAT in terms of increased mitochondrial function and insulin sensitivity.
Brown adipose tissue (BAT) plays a major role in body energy expenditure and contains numerous mitochondria that function to mediate adaptive thermogenesis and protect against hypothermia and obesity (Cannon and Nedergaard, 2004). The activation of BAT offers a new way to battle obesity and other metabolic disorders, closely associated with both mitochondrial function alterations and reduced mitochondrial density (Patti et al., 2003; Morino et al., 2005).
Mitochondria are dynamic organelles that continuously are subjected to dynamic processes: (a) the fusion of two mitochondria in a single; (b) the fission of mitochondria into smaller ones; and (c) the biogenesis, required for cell growth and adaptation to increased oxidative stress and nutritional deprivation. Mitochondrial dynamics is a highly regulated process that controls mitochondrial density in the cells and may be changed depending on the physiological cell state. In mammals, mitochondrial fusion is mediated by mitofusin 1 and 2 (Mfn1 and Mfn2) and the dynamic-related GTPase, optic atrophy-1 (OPA1), which are located in the outer and inner membranes, respectively (Malka et al., 2005; Quiros et al., 2012). Mitochondrial fission is mediated by dynamin-related protein 1 (Drp1), which is mainly located in the cytosol (Smirnova et al., 2001). Mitochondria go through continuous cycles of selective fusion and fission, referred to as the “mitochondrial life cycle,” to maintain the quality of its function. Deregulation of fusion/fission events appears to be involved in several diseases. The functions of OPA1 are controlled by alternative splicing and proteolysis of different isoforms, long and short isoforms (L-OPA1 and S-OPA1) (Head et al., 2009). The ATP-independent metalloprotease OMA1, a proteolytic enzyme located in the inner mitochondrial membrane, is responsible for OPA1 proteolysis (Quiros et al., 2013). Stress conditions, such as the induction of apoptosis and the specific dissipation of mitochondrial membrane potential, induce the cleavage of OPA1 to short isoforms by OMA1, resulting in the inhibition of mitochondrial fusion (Head et al., 2009; Jiang et al., 2014). Peroxisome proliferator-activated receptor-γ coactivator 1α (PGC1α) is a major regulator of mitochondrial biogenesis by activating different transcription factors, including the nuclear respiratory factor type 1 (Nrf1). Nrf1 interacts with mitochondrial transcription factor A (Tfam), which drives transcription and replication of mitochondrial DNA and, therefore, mitochondrial biogenesis (Vina et al., 2009). Both AMP-activated protein kinase (AMPK) and the silent information regulator protein (Sir2) homolog SIRT1 are activators of PGC1α (Fulco and Sartorelli, 2008).
The insulin receptor (IR) is a member of the tyrosine kinase receptor superfamily with an essential role in glucose metabolism (Benyoucef et al., 2007; Ward and Lawrence, 2009). The IR is closely related to other receptors such as the IGF type I receptor (IGF-IR) that is involved in normal growth and development (Ward and Lawrence, 2009). In mammals, alternative splicing gives rise to two isoforms of IR: IRA and IRB. IRB has 12 additional amino acids encoded by exon 11 (Whittaker et al., 2002). This sequence is located immediately downstream of the ligand binding domain but does not affect insulin binding affinity (Whittaker et al., 2002; Menting et al., 2013). Moreover, IRA is predominantly expressed during fetal development enhancing the effects of IGF-II (Frasca et al., 1999). Conversely, IRB is the predominant IR in adult tissues, including the liver, where it triggers the metabolic effects of insulin (Malaguarnera et al., 2011).
Exercise elicits changes and adaptations in the energy metabolism in skeletal muscle, liver, as well as in white and BAT (Laye et al., 2009; Slocum et al., 2013; Drake et al., 2016). One of the most profound effects of exercise training is an increase in mitochondrial content in skeletal muscle, since higher mitochondrial content will increase fat utilization and reduce the formation of lactic acid and other fatigue related substances at a given submaximal workload (Stisen et al., 2006). In white adipose tissue from healthy non-obese rats, exercise training increased the expression of several proteins involved in mitochondrial biogenesis regulation, such as PGC1α and TFAM (Sutherland et al., 2009). However, information on the effects of chronic exercise on mitochondrial dynamics in BAT is scarce. Moreover, uncoupling proteins (UCPs) play a pivotal role in the metabolic adaptation of BAT to exercise training, increasing the oxidation of metabolic substrates necessary for sustaining enhanced thermogenesis in order to produce heat (Boss et al., 1998; Ricquier, 2006). On the other hand, it has been described that chronic exercise improves insulin sensitivity (Wagner et al., 2016; Jimenez-Maldonado et al., 2017), favoring glucose uptake in the muscle (Kim, 2016), controlling glucose tolerance (Molsted et al., 2013), as well as preventing vascular dysfunction (Lee et al., 2011). However, there is nothing described whether exercise might improve insulin sensitivity in BAT and what mechanisms might be involved in such improvement.
Therefore, the aim of the present work was to study the consequences of chronic exercise training on factors involved in the regulation of mitochondrial dynamic and the ability to produce energy and improve insulin sensitivity and glucose uptake in rat BAT. For this purpose, protein expression levels of factors involved in mitochondrial remodeling (OMA1, OPA1, Mfn1, and Drp1), mitochondrial biogenesis (NRF1 and TFAM), and main factors from PGC1α signaling cascades as well as proteins implicated in insulin signaling pathway (IR, IRA, IRB, IGF-1R, p-ERK, p-AKT, p-IRS-1) were investigated in interscapular BAT from rats subjected to chronic exercise training. In addition, to evaluate mitochondrial proteins involved in energy/heat production, the expression levels of carnitine palmitoyltransferase II (CPTII), mitochondrial malate dehydrogenase 2 (mMDH), mitochondrial F1 ATP synthase α-chain and UCP1,2,3 were also determined in rat interscapular BAT.
Three-month-old male Wistar rats (initial weight: 334.6 ± 3.9 g) were obtained from the Animal Quarters of the Autonoma University of Madrid (Registration number EX-021U; ES-280790000097). This study was carried out in accordance with the recommendations of the European Union guidelines on the ethical care of experimental animals (DG XI of the European Commission, Directive 2010/63/EU, of September 22, 2010, Annex IV). The protocol was approved by the Ethics Committee of the Autonoma University of Madrid (CEI-38-839; RD 1201/2005). Rats were housed at a constant room temperature, humidity, and light cycle (12:12-h light-dark) and had free access to tap water and standard rat chow (#2014, Harlan Teklad, MN, United States) ad libitum. Animals were weighed every 2 weeks. Rats were divided into two experimental groups: (1) control group (C; n = 10) and (2) rats trained for 8 weeks (ET; n = 10). Rats were fasting overnight for 12 h before euthanasia. After being sacrificed the muscle soleus and the interscapular BAT pads were removed, weighed, and washed in saline solution, dried and immediately frozen and stored at -80°C.
Exercise training was performed on a motor treadmill (Motor-driven Treadmill LI8706, Letica Scientific Instruments, Barcelona, Spain) for 8 weeks, five times per week for 50 min each session, gradually progressing toward 55–65% of maximal running speed (15–20 m/min), as described elsewhere (Graham and Rush, 2004; Blanco-Rivero et al., 2013). To determine the maximal exercise capacity, rats were subjected to a progressive exercise test on a treadmill using an incremental speed protocol of 5 m/min every 3 min and no grade until exhaustion. The treadmill exercise test was repeated after 5 weeks of exercise training in order to adjust training intensity. Rats were considered to be exhausted when they could no longer run at the treadmill speed (Blanco-Rivero et al., 2013). The sedentary or control rats were handled at least twice a week for habituation to the experimental protocols.
Citrate synthase activity was used as a marker of muscle oxidative activity (Alp et al., 1976). The enzyme activity was measured in whole muscle homogenates, and the complex resulting from acetyl-CoA and oxaloacetate was determined at 412 nm (ASYS UVM 340, Biochrom, Cambridge, United Kingdom) and 25°C, at an interval of 10 min. Citrate synthase activity was expressed as nmol/min per mg of protein.
Plasma concentrations of glucose, total cholesterol, and triglycerides were determined using spectrophotometric techniques in an autoanalyzer (Vitros Fusion 5,1, Diagnostics Ortho Clinical, Johnson & Johnson, New Brunswick, NJ, United States).
Plasma concentration of insulin and oxidized LDL were measured with specific quantitative sandwich enzyme immunoassay (EZRMI-13K, Millipore-Bedford, Burlington, MA, United States and Biomedica Medizinprodukte GmbH & Co., KG, Vienna, Austria, respectively). Absorbance results were read on a spectrophotometer at a wavelength of 450 nm (Reader UVM 340, Asys Hitech GmbH, Austria).
Interscapular BAT samples (100 mg) were homogenized in 300 μL of lysis buffer (ReadyPreTM 2-D Starter Kit Rehydration/Sample Buffer #1632106; Bio-Rad Laboratories, Inc., Hercules, CA, United States) mixed with protease inhibitor cocktail (Roche Applied Science, Mannheim, Germany) and phenylmethylsulfonyl fluoride (Sigma-Aldrich, Co., Spain) with the automatic homogenizer Bullet Blender, following the manufacturer’s instructions (Cultek, SSB14B, Next Advance, Inc., United States). After homogenization, samples were kept on ice for 30 min, shaken briefly and centrifuged at 10,000 g for 10 min at 4°C. Finally, proteins were collected from supernatant. Samples were separated by sodium dodecyl sulfate-polyacrylamide gel electrophoresis under reducing conditions. After electrophoresis, samples were transferred to polyvinylidene difluoride membranes (Millipore-Bedford, Burlington, MA, United States). The membranes were blocked in PBS containing 0.1% Tween-20 and 5% dry skimmed milk for 1 h at room temperature, and were then incubated in the same buffer with specific antibodies for 18 h at 4°C. OPA1 [1:2000; 612607] (BD Biosciences, Madrid, Spain); OMA1 [1:500; ab104316], Mfn1 [1:1000; ab57602], Drp1 [1:500; ab56788], AMPK [1:1000; ab80039], phospho AMPK (pAMPK) [1:1000; ab72845], SIRT1 [1:500; ab110304], PGC1α [1:250; ab106814], uncoupling protein 1 (UCP-1) [1:1000; ab23841], UCP-2 [1:500; ab67241], UCP-3 [1:1000; ab3477], NRF1 [1:1000; ab175932], and TFAM [1:1000; ab131607] (Abcam, Cambridge, United Kingdom); mitochondrial F1 ATP synthase α-chain [1:1000; sc-58613], carnitine palmitoyltransferase II (CPTII) [1:1000; sc-20526], mitochondrial malate dehydrogenase 2 (mMDH) [1:1000; sc133777], IR [1:1000; sc-711] and insulin-like growth factor 1 receptor (IGF-1R) [1:1000; sc-713] (Santa Cruz Biotechnology, Dallas, TX, United States); p-IRS-1 (S307) [1:1000; 13110], and p-p42/44 (Thr202/Tyr204) [1:1000; 9102] (Cell Signaling, St. Louis, MO, United States). The anti-IRB antibody (plus exon 11) was kindly provided by Dr. Sesti and Dr. Hribal. After washing, detection was made through incubation with peroxidase-conjugated secondary antibody, and developed using an ECL chemiluminescence kit (Millipore-Bedford, Burlington, MA, United States). As loading control we used β-actin [1:10000] (Sigma Aldrich, Co., Spain). In addition, Red Ponceau staining was used to show the quality of proteins and efficacy of protein transfer to the membrane (not shown). The detection for blots was made using the GeneGnome5 (Syngene Bio Imaging; Synoptics Ltd., Cambridge, United Kingdom) obtaining a chemiluminescence imaging. Results are expressed as an n-fold increase over the values of the control group in densitometric arbitrary units.
A total of 150 μg protein extracts from BAT was immunoprecipitated with IRB isoform antibody. The supernatants from the first immunoprecipitation were immunoprecipitated with IRβ antibody (recognizes two IR isoforms). Thus, immune complexes (only IRA) were collected on protein A-agarose beads and submitted to SDS–PAGE. Finally, the immunoblots were incubated with anti-IGF-1R antibodies to study the association between IRA or IRB isoform and IGF-1R.
All analyses and graphs were performed using GraphPad Prism 5 (GraphPad Software). Data were compared by the Lilliefors test followed by Student’s t-test. Values are presented as means ± standard error of mean (SEM). The level of significance was set at p < 0.05.
As expected, exercise training reduced (p < 0.05) body weight and body weight gain in rats. Exercise training decreased (p < 0.05) basal plasma insulin levels without modifying glucose levels. Plasma total cholesterol and triglyceride levels were similar in both control and trained rats. Chronic exercise training decreased (p < 0.05) plasma oxidize LDL concentration. Citrate synthase activity in soleus was higher (p < 0.05) in trained rats compared to control animals (Table 1).
To evaluate the effects of exercise training on mitochondrial remodeling, OMA1, OPA1, Mfn1, and Drp1 were evaluated. Exercise training increased (p < 0.05) protein expression of OMA1 and S-OPA1 in BAT, and expression of L-OPA1 decreased (p < 0.05) in rats under chronic exercise compared to control (Figures 1A–C). Mfn1 protein expression decreased (p < 0.05) in BAT of trained rats compared to control rats (Figure 1D). Furthermore, chronic exercise training increased (p < 0.05) protein expression of Drp1 expression in BAT (Figure 1E).
FIGURE 1. Quantitative analyses of protein levels by western blot for (A) ATP-independent metalloprotease OMA1, (B) long optic atrophy 1 (L-OPA1), (C) short optic atrophy 1 (S-OPA1), (D) mitofusin 1 (Mfn1), and (E) dynamin related protein 1 (Drp1) in brown adipose tissue of control rats (C) and trained rats (ET) for 8 weeks. Data are expressed as mean ± SEM. (n = 10 animals per group). ∗p < 0.05 vs. C.
To evaluate the effects of exercise training on mitochondrial biogenesis, NRF1 and TFAM were evaluated. Chronic exercise training increased (p < 0.05) protein expression of NRF1 and TFAM protein expression in BAT, suggesting an enhancement of mitochondrial biogenesis (Figures 2A,B).
FIGURE 2. Quantitative analyses of protein levels by western blot for (A) nuclear respiratory factor type 1 (NRF1) and (B) mitochondrial transcription factor A (TFAM) in brown adipose tissue of control rats (C) and trained rats (ET) for 8 weeks. Data are expressed as mean ± SEM. (n = 10 animals per group). ∗p < 0.05 vs. C.
Protein expression of SIRT1, ratio pAMPK/AMPK and PGC1α were measured to evaluate factors regulating mitochondrial biogenesis. Protein expression of SIRT1 and ratio pAMPK/AMPK in BAT was higher (p < 0.05) in trained rats compared to control rats (Figures 3A,B). It was accompanied of increased expression (p < 0.05) of PGC1α in trained rats (Figure 3C).
FIGURE 3. Quantitative analyses of protein levels by western blot for (A) sirtuin 1 (SIRT1), (B) pAMPK/AMPK ratio and (C) peroxisome proliferator-activated receptor γ coactivator 1α (PGC1α) in brown adipose tissue of control rats (C) and trained rats (ET) for 8 weeks. Data are expressed as mean ± SEM. (n = 10 animals per group). ∗p < 0.05 vs. C.
Exercise training increased (p < 0.05) CPTII, mitochondrial F1 ATP synthase α-chain expression and mMDH (Figures 4A–C) in BAT. UCP1, UCP2, and UCP3 expression increased (p < 0.05) in BAT from rats under chronic exercise compared to controls (Figures 5A–C).
FIGURE 4. Quantitative analyses of protein levels by western blot for (A) carnitine palmitoyltransferase II (CPTII), (B) mitochondrial F1 ATP synthase α-chain ATP synthase and (C) mitochondrial malate dehydrogenase 2 (mMDH) in brown adipose tissue of control rats (C) and trained rats (ET) for 8 weeks. Data are expressed as mean ± SEM. (n = 10 animals per group). ∗p < 0.05 vs. C.
FIGURE 5. Quantitative analyses of protein levels by western blot for (A) uncoupling protein 1 (UCP1), (B) UCP2, and (C) UCP3 in brown adipose tissue of control rats (C) and trained rats (ET) for 8 weeks. Data are expressed as mean ± SEM. (n = 10 animals per group). ∗p < 0.05 vs. C.
Exercise training increased (p < 0.05) the expression of total IR as compared to controls (Figure 6A). Regarding IR isoforms, exercise increased (p < 0.05) the expression of IRA while it did not change IRB levels (Figures 6B,C). However, the expression of IGF-1R was dramatically decreased (p < 0.05) in rats under chronic exercise (Figure 6D). Next, we measured the association between IGF-1R and IR isoforms (Figure 6E), and the results revealed that chronic exercise induced an increased formation of IRA/IGF-1R hybrid receptors as compared to IRB/IGF-1R hybrids. Finally, we evaluated the insulin sensitivity in control and exercise training groups by means of ERK and S307-IRS-1 phosphorylation. Our results showed that chronic exercise improves insulin sensitivity increasing ERK phosphorylation and decreasing S307-IRS-1 phosphorylation (Figure 6F). The loading control, α-tubulin, of Figures 6A,F is the same because both Western blots against IRβ and p-ERK antibodies were performed in the same membrane (Supplementary Presentation 1).
FIGURE 6. Insulin signaling in BAT from control rats (C) and trained rats (ET). Effect of chronic exercise in protein levels of (A) IR, (B) IRA, (C) IRB, (D) IGF-1R, (E) Hybrid receptors: IRA/IGF-1R or IRB/IGF-1R and (F) p-IRS-1 (S307) and p-p42/44 by Western blot. To analyze the specific isoforms IR, we first performed immunoprecipitations against IRB antibody and after immunoprecipitations against IRβ. Data are expressed as mean ± SEM. (n = 10 animals per group). ∗p < 0.05 vs. C. The loading control, α-tubulin, of panels (A,F) is the same because both Western blots against IRβ and p-ERK antibodies were performed in the same membrane.
The present study describes for the first time, that exercise training in rats modifies mitochondrial dynamics in BAT. Enhanced expression of factors regulating mitochondrial biogenesis and mitochondrial fission, together with decreased expression of factors regulating mitochondrial fusion were produced. These effects occurred together with enhanced expression of UCP1, UCP2, and UCP3 which suggests increased heat production, as a possible metabolic adaptation of BAT to chronic exercise training. Moreover, an increase of IRA/IRB ratio, IRA-IGF-1R hybrids, and p42/44 activation as well as a significant decrease of IGF-1R expression and p-IRS-1 (S307) indicate higher insulin sensitivity and favored glucose uptake in BAT in response to chronic exercise training.
Exercise training has long been known to promote mitochondrial biogenesis in skeletal muscle. However, the effects of chronic exercise training on mitochondrial dynamics in BAT are fairly unknown. The present study shows that chronic exercise training increased pAMPK/AMPK ratio and the expression of SIRT1 in BAT, which could be responsible for the observed enhanced expression of PGC1α. Indeed, SIRT1 deacetylates PGC1α while AMPK phosphorylates PGC1α, resulting in promotion of mitochondrial biogenesis (Dominy et al., 2010). Mitochondrial biogenesis depends upon the activity of nuclear transcription factors including NRF1 and TFAM (Virbasius and Scarpulla, 1994). Thus it could be proposed that under the present conditions, enhancement of PGC1α would activate NRF1 promoting the expression of TFAM, and consequently mitochondrial differentiation and biogenesis.
Fusion/fission equilibrium is a key element for mitochondrial growth and redistribution, and for maintenance of a quality mitochondrial network (Song et al., 2007; Head et al., 2009; Anand et al., 2014). Several human (Cartoni et al., 2005; Fealy et al., 2014) and experimental (Ding et al., 2010; Caffin et al., 2013) studies on mitochondrial fusion/fission equilibrium have been conducted in skeletal muscle during exercise, although mitochondrial remodeling in BAT in response to chronic exercise training is fairly unknown. The present study shows that exercise training increased OMA1 and S-OPA1 expression together with a decrease in L-OPA1. These results together with the observed reduction of Mfn1 expression indicates reduced mitochondrial fusion under the present experimental circumstances. Previous results also showed that chronic exercise training could be considered a stressful condition, increasing both OMA1 expression and cleavage of L-OPA1, which leads to an increased expression of S-OPA1 (Quiros et al., 2012). The proteolysis of OPA1 by OMA1 leads to the formation of several subunits of OPA1 by alternative splicing, generating fusion-incompetent OPA1 and cessation of inner membrane fusion (Malka et al., 2005). This situation is further supported by the observed increased expression of Drp1, a protein involved in mitochondrial fission. Therefore, it could be proposed that changes in mitochondrial biogenesis and fusion/fission equilibrium, could be considered as part of the metabolic adaptation of BAT to chronic exercise, in order to maintain overall mitochondrial quality.
Mitochondrial content and respiratory capacity can be modified according to specific metabolic conditions such as exercise (Liesa and Shirihai, 2013). Under standard conditions, mitochondrial respiration is coupled to ATP production and constitutes the main source of ATP. However, as the coupling of respiration to ADP phosphorylation is less than 100% efficient energetically, respiration also releases heat (Ricquier, 2006). UCPs such as UCP1 are mitochondrial proteins able to dissipate the proton gradient generated by NADH-powered pumping of protons from the mitochondrial matrix to the mitochondrial intermembrane space. The energy lost in dissipating the proton gradient by UCP action is not used to produce ATP but to generate heat. Thus, UCP1 plays a key role in BAT physiology since it confers to brown adipocytes their specific capacity to dissipate oxidation energy as heat (Ricquier et al., 1986; Ricquier, 2006). As previously published, our study shows an increased expression of UCP1 indicating that exercise training increases UCP1 in order to produce heat (Boss et al., 1998). In addition, the results also show increased expression of UCP2 and UCP3 in BAT, probably as a co-operative response to chronic exercise training to enhance heat production. To our knowledge, few studies revealed increased expression of UCP2 and three during chronic exercise in BAT. PGC-1α, as a master regulator of mitochondrial biogenesis and oxidative metabolism, is highly expressed in tissues with high oxidative metabolism such as BAT and skeletal muscle (Knutti and Kralli, 2001; Puigserver and Spiegelman, 2003), and in our study would participate actively in the activation of UCP1. Similarly, it is known that is strongly induced in BAT by cold exposure, and in turn, stimulates the adaptive thermogenic program by inducing UCP1 gene (Ricquier et al., 1986; Puigserver et al., 1998). This is achieved by the numerous mitochondria in brown adipocytes and UCPs, associated with increased biogenesis and mitochondrial fission, which activates respiration and diverts oxidation-free energy to thermogenesis (Ricquier, 2006). In addition, several studies show that the increase in mitochondrial fission potentiates free fatty acid-induced uncoupling and enables the tissue to be more thermogenic (Wikstrom et al., 2014).
One of the consequences could be an increased mitochondrial capacity to generate ATP, would match energy demands during chronic exercise. This notion is supported by the observed increase of CPTII, mMDH, and ATP synthase, which promote fatty acid transport and beta-oxidation into the mitochondria, Krebs’s cycle and oxidative phosphorylation, respectively. On the other hand, an increase in uncoupling is common features of various conditions that lead to mitochondrial fragmentation (Parone et al., 2008; Wikstrom et al., 2014). This is further supported by the observed increased expression of Drp1 and S-OPA in BAT of rats subjected to chronic physical exercise. These results provide evidences that mitochondrial fission is not deleterious per se but it regulates uncoupled respiration and thus increases energy expenditure by promoting shifting nutrient oxidation toward heat production, rather than toward mitochondrial ATP synthesis (Twig and Shirihai, 2011).
Finally, it is widely known that physical exercise is able to ameliorate insulin sensitivity in myocytes by increasing glucose uptake (Birnbaum, 1989; James et al., 1989; Neufer and Dohm, 1993; Gurley et al., 2016). In this sense, we demonstrate that chronic exercise increases IR expression in BAT, moreover, we observe a decrease in IRS-1 (S307) phosphorylation as well as an increased p42/p44 phosphorylation suggesting that chronic exercise is also able to increase insulin sensitivity in BAT. In addition, it has been shown that the IRA/IRB ratio can be modified in several pathophysiological conditions (Nevado et al., 2006; Escribano et al., 2009), and our results show that chronic exercise training induces an increased IRA/IRB ratio in BAT. IRA has been described as a glucose uptake modulator in hepatocytes, vascular smooth muscle cells and beta cells (Nevado et al., 2006; Gomez-Hernandez et al., 2013; Escribano et al., 2015; Diaz-Castroverde et al., 2016a,b). Thus, the increased IRA/IRB ratio observed in chronic exercise trained rats could be also favoring glucose uptake in the BAT favoring glucose disposal and oxidation.
In summary, the present study indicates that chronic exercise is able to improve the energetic profile of BAT in terms of increased mitochondrial function and insulin sensitivity. Our results demonstrate a higher oxidative capacity of BAT that could help to improve the metabolic rate of the whole body in order to fight against lipid accumulation and obesity. A better understanding in the mechanisms involved could help to find new targets for obesity treatment.
NdlH and AG-H conceived and designed the experiments, performed the experiments, analyzed the data, and wrote the manuscript. MK-M and SB performed the experiments and analyzed the data. BM-F, JB-R, GB, and MB conceived and designed the experiments and analyzed the data. MH provided anti-IRB antibody (plus exon 11). VL conceived and designed the experiments, analyzed the data, and wrote the manuscript. All authors read and approved the final version of the manuscript.
This work was supported by grants from Comisión Interministerial de Ciencia y Tecnología de España (SAF-38530) and Ministerio de Ciencia e Innovación (IPT-0213-06000).
The authors declare that the research was conducted in the absence of any commercial or financial relationships that could be construed as a potential conflict of interest.
The Supplementary Material for this article can be found online at: https://www.frontiersin.org/articles/10.3389/fphys.2018.01122/full#supplementary-material
Alp, P. R., Newsholme, E. A., and Zammit, V. A. (1976). Activities of citrate synthase and NAD+-linked and NADP+-linked isocitrate dehydrogenase in muscle from vertebrates and invertebrates. Biochem. J. 154, 689–700. doi: 10.1042/bj1540689
Anand, R., Wai, T., Baker, M. J., Kladt, N., Schauss, A. C., Rugarli, E., et al. (2014). The i-AAA protease YME1L and OMA1 cleave OPA1 to balance mitochondrial fusion and fission. J. Cell Biol. 204, 919–929. doi: 10.1083/jcb.201308006
Benyoucef, S., Surinya, K. H., Hadaschik, D., and Siddle, K. (2007). Characterization of insulin/IGF hybrid receptors: contributions of the insulin receptor L2 and Fn1 domains and the alternatively spliced exon 11 sequence to ligand binding and receptor activation. Biochem. J. 403, 603–613. doi: 10.1042/BJ20061709
Birnbaum, M. J. (1989). Identification of a novel gene encoding an insulin-responsive glucose transporter protein. Cell 57, 305–315. doi: 10.1016/0092-8674(89)90968-9
Blanco-Rivero, J., Roque, F. R., Sastre, E., Caracuel, L., Couto, G. K., Avendano, M. S., et al. (2013). Aerobic exercise training increases neuronal nitric oxide release and bioavailability and decreases noradrenaline release in mesenteric artery from spontaneously hypertensive rats. J. Hypertens. 31, 916–926. doi: 10.1097/HJH.0b013e32835f749c
Boss, O., Samec, S., Desplanches, D., Mayet, M. H., Seydoux, J., Muzzin, P., et al. (1998). Effect of endurance training on mRNA expression of uncoupling proteins 1, 2, and 3 in the rat. FASEB J. 12, 335–339. doi: 10.1096/fasebj.12.3.335
Caffin, F., Prola, A., Piquereau, J., Novotova, M., David, D. J., Garnier, A., et al. (2013). Altered skeletal muscle mitochondrial biogenesis but improved endurance capacity in trained OPA1-deficient mice. J. Physiol. 591, 6017–6037. doi: 10.1113/jphysiol.2013.263079
Cannon, B., and Nedergaard, J. (2004). Brown adipose tissue: function and physiological significance. Physiol. Rev. 84, 277–359. doi: 10.1152/physrev.00015.2003
Cartoni, R., Leger, B., Hock, M. B., Praz, M., Crettenand, A., Pich, S., et al. (2005). Mitofusins 1/2 and ERRalpha expression are increased in human skeletal muscle after physical exercise. J. Physiol. 567, 349–358. doi: 10.1113/jphysiol.2005.092031
Diaz-Castroverde, S., Baos, S., Luque, M., Di Scala, M., Gonzalez-Aseguinolaza, G., Gomez-Hernandez, A., et al. (2016a). Prevalent role of the insulin receptor isoform a in the regulation of hepatic glycogen metabolism in hepatocytes and in mice. Diabetologia 59, 2702–2710.
Diaz-Castroverde, S., Gomez-Hernandez, A., Fernandez, S., Garcia-Gomez, G., Di Scala, M., Gonzalez-Aseguinolaza, G., et al. (2016b). Insulin receptor isoform a ameliorates long-term glucose intolerance in diabetic mice. Dis. Model Mech. 9, 1271–1281.
Ding, H., Jiang, N., Liu, H., Liu, X., Liu, D., Zhao, F., et al. (2010). Response of mitochondrial fusion and fission protein gene expression to exercise in rat skeletal muscle. Biochim. Biophys. Acta 1800, 250–256. doi: 10.1016/j.bbagen.2009.08.007
Dominy, J. E. Jr., Lee, Y., Gerhart-Hines, Z., and Puigserver, P. (2010). Nutrient-dependent regulation of PGC-1alpha’s acetylation state and metabolic function through the enzymatic activities of Sirt1/GCN5. Biochim. Biophys. Acta 1804, 1676–1683. doi: 10.1016/j.bbapap.2009.11.023
Drake, J. C., Wilson, R. J., and Yan, Z. (2016). Molecular mechanisms for mitochondrial adaptation to exercise training in skeletal muscle. FASEB J. 30, 13–22. doi: 10.1096/fj.15-276337
Escribano, O., Gomez-Hernandez, A., Diaz-Castroverde, S., Nevado, C., Garcia, G., Otero, Y. F., et al. (2015). Insulin receptor isoform a confers a higher proliferative capability to pancreatic beta cells enabling glucose availability and IGF-I signaling. Mol. Cell. Endocrinol. 409, 82–91. doi: 10.1016/j.mce.2015.03.008
Escribano, O., Guillen, C., Nevado, C., Gomez-Hernandez, A., Kahn, C. R., and Benito, M. (2009). Beta-cell hyperplasia induced by hepatic insulin resistance: role of a liver-pancreas endocrine axis through insulin receptor A isoform. Diabetes Metab. Res. Rev. 58, 820–828.
Fealy, C. E., Mulya, A., Lai, N., and Kirwan, J. P. (2014). Exercise training decreases activation of the mitochondrial fission protein dynamin-related protein-1 in insulin-resistant human skeletal muscle. J. Appl. Physiol. 117, 239–245. doi: 10.1152/japplphysiol.01064.2013
Frasca, F., Pandini, G., Scalia, P., Sciacca, L., Mineo, R., Costantino, A., et al. (1999). Insulin receptor isoform a, a newly recognized, high-affinity insulin-like growth factor II receptor in fetal and cancer cells. Mol. Cell. Biol. 19, 3278–3288. doi: 10.1128/MCB.19.5.3278
Fulco, M., and Sartorelli, V. (2008). Comparing and contrasting the roles of AMPK and SIRT1 in metabolic tissues. Cell Cycle 7, 3669–3679. doi: 10.4161/cc.7.23.7164
Gomez-Hernandez, A., Escribano, O., Perdomo, L., Otero, Y. F., Garcia-Gomez, G., Fernandez, S., et al. (2013). Implication of insulin receptor a isoform and IRA/IGF-IR hybrid receptors in the aortic vascular smooth muscle cell proliferation: role of TNF-alpha and IGF-II. Endocrinology 154, 2352–2364. doi: 10.1210/en.2012-2161
Graham, D. A., and Rush, J. W. (2004). Exercise training improves aortic endothelium-dependent vasorelaxation and determinants of nitric oxide bioavailability in spontaneously hypertensive rats. J. Appl. Physiol. 96, 2088–2096. doi: 10.1152/japplphysiol.01252.2003
Gurley, J. M., Griesel, B. A., and Olson, A. L. (2016). Increased skeletal muscle GLUT4 expression in obese mice after voluntary wheel running exercise is posttranscriptional. Diabetes Metab. Res. Rev. 65, 2911–2919. doi: 10.2337/db16-0305
Head, B., Griparic, L., Amiri, M., Gandre-Babbe, S., and Van Der Bliek, A. M. (2009). Inducible proteolytic inactivation of OPA1 mediated by the OMA1 protease in mammalian cells. J. Cell Biol. 187, 959–966. doi: 10.1083/jcb.200906083
James, D. E., Strube, M., and Mueckler, M. (1989). Molecular cloning and characterization of an insulin-regulatable glucose transporter. Nature 338, 83–87. doi: 10.1038/338083a0
Jiang, X., Jiang, H., Shen, Z., and Wang, X. (2014). Activation of mitochondrial protease OMA1 by Bax and Bak promotes cytochrome c release during apoptosis. Proc. Natl. Acad. Sci. U.S.A. 111, 14782–14787. doi: 10.1073/pnas.1417253111
Jimenez-Maldonado, A., Virgen-Ortiz, A., Melnikov, V., Rodriguez-Hernandez, A., Gamboa-Dominguez, A., Montero, S., et al. (2017). Effect of moderate and high intensity chronic exercise on the pancreatic islet morphometry in healthy rats: BDNF receptor participation. Islets 9, 1–10. doi: 10.1080/19382014.2016.1260796
Kim, J. C. (2016). The effect of exercise training combined with PPARgamma agonist on skeletal muscle glucose uptake and insulin sensitivity in induced diabetic obese Zucker rats. J. Exerc. Nutrition Biochem. 20, 42–50. doi: 10.20463/jenb.2016.06.20.2.6
Knutti, D., and Kralli, A. (2001). PGC-1, a versatile coactivator. Trends Endocrinol. Metab. 12, 360–365. doi: 10.1016/S1043-2760(01)00457-X
Laye, M. J., Rector, R. S., Warner, S. O., Naples, S. P., Perretta, A. L., Uptergrove, G. M., et al. (2009). Changes in visceral adipose tissue mitochondrial content with type 2 diabetes and daily voluntary wheel running in OLETF rats. J. Physiol. 587, 3729–3739. doi: 10.1113/jphysiol.2009.172601
Lee, S., Park, Y., and Zhang, C. (2011). Exercise training prevents coronary endothelial dysfunction in type 2 diabetic mice. Am. J. Biomed. Sci. 3, 241–252. doi: 10.5099/aj110400241
Liesa, M., and Shirihai, O. S. (2013). Mitochondrial dynamics in the regulation of nutrient utilization and energy expenditure. Cell Metab. 17, 491–506. doi: 10.1016/j.cmet.2013.03.002
Malaguarnera, R., Frasca, F., Garozzo, A., Giani, F., Pandini, G., Vella, V., et al. (2011). Insulin receptor isoforms and insulin-like growth factor receptor in human follicular cell precursors from papillary thyroid cancer and normal thyroid. J. Clin. Endocrinol. Metab. 96, 766–774. doi: 10.1210/jc.2010-1255
Malka, F., Guillery, O., Cifuentes-Diaz, C., Guillou, E., Belenguer, P., Lombes, A., et al. (2005). Separate fusion of outer and inner mitochondrial membranes. EMBO Rep. 6, 853–859. doi: 10.1038/sj.embor.7400488
Menting, J. G., Whittaker, J., Margetts, M. B., Whittaker, L. J., Kong, G. K., Smith, B. J., et al. (2013). How insulin engages its primary binding site on the insulin receptor. Nature 493, 241–245. doi: 10.1038/nature11781
Molsted, S., Harrison, A. P., Eidemak, I., Dela, F., and Andersen, J. L. (2013). Improved glucose tolerance after high-load strength training in patients undergoing dialysis. Nephron Clin. Pract. 123, 134–141. doi: 10.1159/000353231
Morino, K., Petersen, K. F., Dufour, S., Befroy, D., Frattini, J., Shatzkes, N., et al. (2005). Reduced mitochondrial density and increased IRS-1 serine phosphorylation in muscle of insulin-resistant offspring of type 2 diabetic parents. J. Clin. Invest. 115, 3587–3593. doi: 10.1172/JCI25151
Neufer, P. D., and Dohm, G. L. (1993). Exercise induces a transient increase in transcription of the GLUT-4 gene in skeletal muscle. Am. J. Physiol. 265, C1597–C1603. doi: 10.1152/ajpcell.1993.265.6.C1597
Nevado, C., Valverde, A. M., and Benito, M. (2006). Role of insulin receptor in the regulation of glucose uptake in neonatal hepatocytes. Endocrinology 147, 3709–3718. doi: 10.1210/en.2005-1663
Parone, P. A., Da Cruz, S., Tondera, D., Mattenberger, Y., James, D. I., Maechler, P., et al. (2008). Preventing mitochondrial fission impairs mitochondrial function and leads to loss of mitochondrial DNA. PLoS One 3:e3257. doi: 10.1371/journal.pone.0003257
Patti, M. E., Butte, A. J., Crunkhorn, S., Cusi, K., Berria, R., Kashyap, S., et al. (2003). Coordinated reduction of genes of oxidative metabolism in humans with insulin resistance and diabetes: potential role of PGC1 and NRF1. Proc. Natl. Acad. Sci. U.S.A. 100, 8466–8471. doi: 10.1073/pnas.1032913100
Puigserver, P., and Spiegelman, B. M. (2003). Peroxisome proliferator-activated receptor-gamma coactivator 1 alpha (PGC-1 alpha): transcriptional coactivator and metabolic regulator. Endocr. Rev. 24, 78–90. doi: 10.1210/er.2002-0012
Puigserver, P., Wu, Z., Park, C. W., Graves, R., Wright, M., and Spiegelman, B. M. (1998). A cold-inducible coactivator of nuclear receptors linked to adaptive thermogenesis. Cell 92, 829–839. doi: 10.1016/S0092-8674(00)81410-5
Quiros, P. M., Ramsay, A. J., and Lopez-Otin, C. (2013). New roles for OMA1 metalloprotease: from mitochondrial proteostasis to metabolic homeostasis. Adipocyte 2, 7–11. doi: 10.4161/adip.21999
Quiros, P. M., Ramsay, A. J., Sala, D., Fernandez-Vizarra, E., Rodriguez, F., Peinado, J. R., et al. (2012). Loss of mitochondrial protease OMA1 alters processing of the GTPase OPA1 and causes obesity and defective thermogenesis in mice. EMBO J. 31, 2117–2133. doi: 10.1038/emboj.2012.70
Ricquier, D. (2006). Fundamental mechanisms of thermogenesis. C. R. Biol. 329, 578–586. doi: 10.1016/j.crvi.2005.10.010
Ricquier, D., Bouillaud, F., Toumelin, P., Mory, G., Bazin, R., Arch, J., et al. (1986). Expression of uncoupling protein mRNA in thermogenic or weakly thermogenic brown adipose tissue. Evidence for a rapid beta-adrenoreceptor-mediated and transcriptionally regulated step during activation of thermogenesis. J. Biol. Chem. 261, 13905–13910.
Slocum, N., Durrant, J. R., Bailey, D., Yoon, L., Jordan, H., Barton, J., et al. (2013). Responses of brown adipose tissue to diet-induced obesity, exercise, dietary restriction and ephedrine treatment. Exp. Toxicol. Pathol. 65, 549–557. doi: 10.1016/j.etp.2012.04.001
Smirnova, E., Griparic, L., Shurland, D. L., and Van Der Bliek, A. M. (2001). Dynamin-related protein Drp1 is required for mitochondrial division in mammalian cells. Mol. Biol. Cell 12, 2245–2256. doi: 10.1091/mbc.12.8.2245
Song, Z., Chen, H., Fiket, M., Alexander, C., and Chan, D. C. (2007). OPA1 processing controls mitochondrial fusion and is regulated by mRNA splicing, membrane potential, and Yme1L. J. Cell Biol. 178, 749–755. doi: 10.1083/jcb.200704110
Stisen, A. B., Stougaard, O., Langfort, J., Helge, J. W., Sahlin, K., and Madsen, K. (2006). Maximal fat oxidation rates in endurance trained and untrained women. Eur. J. Appl. Physiol. 98, 497–506. doi: 10.1007/s00421-006-0290-x
Sutherland, L. N., Bomhof, M. R., Capozzi, L. C., Basaraba, S. A., and Wright, D. C. (2009). Exercise and adrenaline increase PGC-1{alpha} mRNA expression in rat adipose tissue. J. Physiol. 587, 1607–1617. doi: 10.1113/jphysiol.2008.165464
Twig, G., and Shirihai, O. S. (2011). The interplay between mitochondrial dynamics and mitophagy. Antioxid. Redox Signal. 14, 1939–1951. doi: 10.1089/ars.2010.3779
Vina, J., Gomez-Cabrera, M. C., Borras, C., Froio, T., Sanchis-Gomar, F., Martinez-Bello, V. E., et al. (2009). Mitochondrial biogenesis in exercise and in ageing. Adv. Drug Deliv. Rev. 61, 1369–1374. doi: 10.1016/j.addr.2009.06.006
Virbasius, J. V., and Scarpulla, R. C. (1994). Activation of the human mitochondrial transcription factor a gene by nuclear respiratory factors: a potential regulatory link between nuclear and mitochondrial gene expression in organelle biogenesis. Proc. Natl. Acad. Sci. U.S.A. 91, 1309–1313. doi: 10.1073/pnas.91.4.1309
Wagner, H., Fischer, H., Degerblad, M., Alvarsson, M., and Gustafsson, T. (2016). Improvement of insulin sensitivity in response to exercise training in type 2 diabetes mellitus is associated with vascular endothelial growth factor a expression. Diab. Vasc. Dis. Res. 13, 361–366. doi: 10.1177/1479164116651391
Ward, C. W., and Lawrence, M. C. (2009). Ligand-induced activation of the insulin receptor: a multi-step process involving structural changes in both the ligand and the receptor. Bioessays 31, 422–434. doi: 10.1002/bies.200800210
Whittaker, J., Sorensen, H., Gadsboll, V. L., and Hinrichsen, J. (2002). Comparison of the functional insulin binding epitopes of the A and B isoforms of the insulin receptor. J. Biol. Chem. 277, 47380–47384. doi: 10.1074/jbc.M208371200
Keywords: mitochondrial dynamic, insulin sensitivity, exercise, brown adipose tissue, UCPs
Citation: de las Heras N, Klett-Mingo M, Ballesteros S, Martín-Fernández B, Escribano Ó, Blanco-Rivero J, Balfagón G, Hribal ML, Benito M, Lahera V and Gómez-Hernández A (2018) Chronic Exercise Improves Mitochondrial Function and Insulin Sensitivity in Brown Adipose Tissue. Front. Physiol. 9:1122. doi: 10.3389/fphys.2018.01122
Received: 11 May 2018; Accepted: 27 July 2018;
Published: 17 August 2018.
Edited by:
Joaquin Garcia-Estañ, Universidad de Murcia, SpainReviewed by:
Águeda González Rodríguez, Instituto de Investigación Sanitaria del Hospital Universitario de La Princesa, SpainCopyright © 2018 de las Heras, Klett-Mingo, Ballesteros, Martín-Fernández, Escribano, Blanco-Rivero, Balfagón, Hribal, Benito, Lahera and Gómez-Hernández. This is an open-access article distributed under the terms of the Creative Commons Attribution License (CC BY). The use, distribution or reproduction in other forums is permitted, provided the original author(s) and the copyright owner(s) are credited and that the original publication in this journal is cited, in accordance with accepted academic practice. No use, distribution or reproduction is permitted which does not comply with these terms.
*Correspondence: Natalia de las Heras, bmxhc2hlcmFAdWNtLmVz
Disclaimer: All claims expressed in this article are solely those of the authors and do not necessarily represent those of their affiliated organizations, or those of the publisher, the editors and the reviewers. Any product that may be evaluated in this article or claim that may be made by its manufacturer is not guaranteed or endorsed by the publisher.
Research integrity at Frontiers
Learn more about the work of our research integrity team to safeguard the quality of each article we publish.