- 1Department of Anesthesiology and Intensive Care Medicine, Pulmonary Engineering Group, University Hospital Carl Gustav Carus, Technische Universität Dresden, Dresden, Germany
- 2Departamento de Engenharia Eletrônica, Federal University of Minas Gerais, Belo Horizonte, Brazil
- 3Department of Orthodontics, Technische Universität Dresden, Dresden, Germany
- 4Institute of Anatomy, Technische Universität Dresden, Dresden, Germany
- 5Department of Surgical Sciences and Integrated Diagnostics, IRCCS San Martino IST, University of Genoa, Genoa, Italy
In experimental acute respiratory distress syndrome (ARDS), random variation of tidal volumes (VT) during volume controlled ventilation improves gas exchange and respiratory system mechanics (so-called stochastic resonance hypothesis). It is unknown whether those positive effects may be further enhanced by periodic VT fluctuation at distinct frequencies, also known as deterministic frequency resonance. We hypothesized that the positive effects of variable ventilation on lung function may be further amplified by periodic VT fluctuation at specific frequencies. In anesthetized and mechanically ventilated pigs, severe ARDS was induced by saline lung lavage and injurious VT (double-hit model). Animals were then randomly assigned to 6 h of protective ventilation with one of four VT patterns: (1) random variation of VT (WN); (2) P04, main VT frequency of 0.13 Hz; (3) P10, main VT frequency of 0.05 Hz; (4) VCV, conventional non-variable volume controlled ventilation. In groups with variable VT, the coefficient of variation was identical (30%). We assessed lung mechanics and gas exchange, and determined lung histology and inflammation. Compared to VCV, WN, P04, and P10 resulted in lower respiratory system elastance (63 ± 13 cm H2O/L vs. 50 ± 14 cm H2O/L, 48.4 ± 21 cm H2O/L, and 45.1 ± 5.9 cm H2O/L respectively, P < 0.05 all), but only P10 improved PaO2/FIO2 after 6 h of ventilation (318 ± 96 vs. 445 ± 110 mm Hg, P < 0.05). Cycle-by-cycle analysis of lung mechanics suggested intertidal recruitment/de-recruitment in P10. Lung histologic damage and inflammation did not differ among groups. In this experimental model of severe ARDS, periodic VT fluctuation at a frequency of 0.05 Hz improved oxygenation during variable ventilation, suggesting that deterministic resonance adds further benefit to variable ventilation.
Introduction
Mechanical ventilation with variable tidal volumes (VT) has been shown to improve gas exchange as well as lung mechanics (Lefevre et al., 1996; Suki et al., 1998; Mutch et al., 2000, 2005; Funk et al., 2004; Bellardine et al., 2006; Graham et al., 2011a,b) and attenuate ventilator induced lung injury (VILI) (Boker et al., 2002; Spieth et al., 2009b; Thammanomai et al., 2013; de Magalhães et al., 2016; Samary et al., 2016) in different models of the acute respiratory distress syndrome (ARDS). In these studies, artificial as well as biologically variable VT patterns have been investigated.
During variable ventilation, VT patterns are often described using the coefficient of variation (CV), even when probability density distributions are not strictly normal. Early investigations focused on the optimization of CV to improve lung function (Arold et al., 2002; Spieth et al., 2009a) and attenuate lung injury (Kiss et al., 2016). In oleic acid induced lung injury in porcine, a CV ≥ 40% was associated with an optimal combination of gas exchange and lung mechanics, while a CV of 30% attenuated VILI (Kiss et al., 2016).
More recently, different VT probability density distributions were investigated in mice. In lung injury induced by hydrochloric acid, a VT probability density distribution tailored to maximize recruitment allowed better gas exchange and respiratory mechanics with reduced lung inflammation compared to random variable ventilation (Thammanomai et al., 2008, 2013).
Aside from this, advanced VT pattern properties (e.g., power distribution, auto-correlation, complexity) have been only addressed once in a model of oleic acid induced lung injury in pigs comparing biologically and random white noise variable VT patterns (Froehlich et al., 2008). Both patterns yielded similar improvements in gas exchange and lung mechanics arguably because both patterns did not differ relevantly in terms of auto-correlation, given the possible range of values. Except for a study in a numerical model of respiratory mechanics (Ma et al., 2011) the impact of determinism during randomly variable ventilation have not been addressed in experimental models of ARDS yet.
In the present study, we investigated the effects of distinct VT pattern frequencies, so-called deterministic frequency resonance, on gas exchange, lung mechanics, hemodynamics, histology, and inflammation during variable controlled mechanical ventilation in experimental ARDS. We hypothesized that, during volume controlled mechanical ventilation, deterministic frequency resonance through VT fluctuation improves lung function and reduces lung injury during variable ventilation.
Methods
The local animal care committee approved the experimental protocol (TVA 24-9168.11-1/2011-22) (Landesdirektion Dresden, Dresden, Saxony, Germany).
Anesthesia and Initial Ventilator Settings
In total, 40 female pigs with mean body weight of 40.4 kg (29.9–50.5 kg, German landrace) were investigated. Animals were pre-medicated intramuscularly with 10 mg/kg ketamine (Ketamin-ratiopharm; Ratiopharm, Ulm, Germany) and 1 mg/kg midazolam (Midazolam, Ratiopharm, Ulm, Germany), and had the trachea intubated with a cuffed 8.0-mm ID endotracheal tube. Initially, mechanical ventilation (Evita XL, Dräger Medical, Lübeck, Germany) was performed in volume-controlled mode with the following settings: fraction of inspired oxygen (FIO2) = 1.0, VT = 10 mL/kg, positive end-expiratory pressure (PEEP) = 5 cm H2O, inspiratory to expiratory time ratio (I:E) = 1:1 and respiratory rate (RR) to keep PaCO2 in the range of 35–45 mm Hg.
Anesthesia was maintained by means of continuous intravenous infusion of midazolam (1–2 mg/kg/h) and ketamine (10–20 mg/kg/h). Muscle paralysis was achieved by continuous administration of atracurium (1–2 mg/kg/h), whereas the volume status was maintained with a continuous infusion of Ringer's acetate (RA- Ringer-Acetat-Lösung Bernburg, Serumwerk Bernburg AG, Bernburg, Germany) at 10 mL/kg/h. The external jugular vein and internal carotid artery were cannulated with 8.5 Fr. Sheaths. The arterial line was used for continuous blood pressure measurements and blood sampling. A pulmonary artery catheter (Opticath, Abbott, Abbott Park, Chicago, IL, USA) was advanced through the venous sheath into the pulmonary artery for continuous measurement of pulmonary arterial blood pressure, mixed venous blood sampling and cardiac output measurements. During the whole experiments, animals were kept supine.
Induction of Lung Injury
Lung injury was induced by saline lung lavage and injurious mechanical ventilation (double-hit model), as described elsewhere (Silva et al., 2013). Briefly, multiple lavages with warmed normal saline solution was performed until PaO2/FIO2 fell below 200 mm Hg and remained stable at this level for ≥ 30 min (1st hit). Following that, ventilator induced lung injury was performed with the following settings: driving pressure of 60 cm H2O, PEEP = 0 cm H2O, RR = 10 min−1, for 5 min (2nd hit). After the 2nd hit previous ventilator settings at baseline 1 were resumed, resulting in PaO2/FIO2 < 100 mmHg. Lung injury was considered stable, when PaO2 did not increase within 15 min.
Patterns of Tidal Volume Variability
It was shown in the literature that the positive effects of variable ventilation with random variation of VT depend on the probability distribution in general and more specifically on the coefficient of variation (stochastic resonance). Therefore, in order to delineate potential effects of deterministic frequency resonance and stochastic resonance, VT patterns were constructed with the constraint of identical probability distribution (Suki et al., 1998; Brewster et al., 2005). Deterministic resonance requires a (sub-) system to be stimulated by frequency specific excitation. Potential sub-systems related directly to the respiratory system have dynamic characteristics (Table 1 and Supplementary Material).
Three sequences of VT cycles with distinct variability patterns were constructed. Initially, a random white noise VT sequence with Gaussian distribution was generated using Matlab version R14 (Natick, MA, USA) (WN). The probability density histogram of the original sequence was subdivided in two, respectively in five sections of equal areas under the Gaussian curve. In this way two VT sequences were generated taking one value of each consecutive area under the curve, as shown in Figure 1. Each VT pattern consisted of the exactly same 600 values and thus had identical probability density distribution (cmp. Supplementary Table 1). At a mean respiratory rate of 30 bpm each pattern had a length of ~20 min. Each pattern was repeated 18 times during the 6 h of therapy. Mean VT of all patterns was 6 ml/kg and single cycle VT values ranged from 0.6 to 11.4 ml/kg (99.7% of all values). 95% of all VT values were in the range of 2.4 … 9.6 ml/kg.
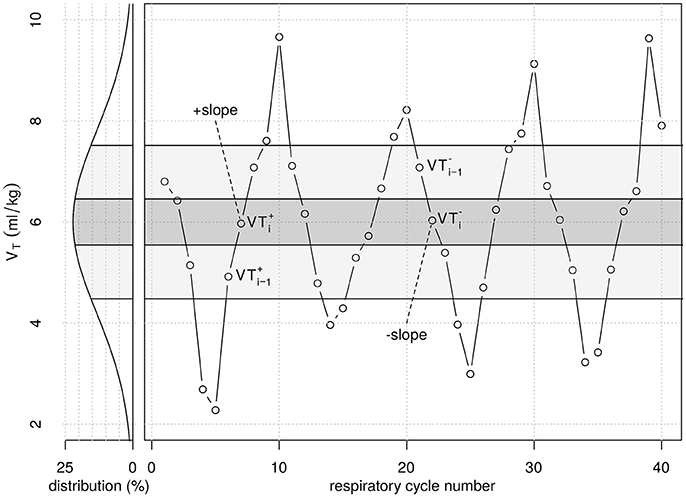
Figure 1. Graphical illustration of part of the tidal volume (VT) series from period pattern P10. Original VT values were randomly generated following a Gaussian distribution (white noise), with respective probability density (left). VT values were then taken from each of the five probability density regions shown (quintiles of the original random VT series) and re-ordered from lowest to highest (+slope) and highest to lowest (−slope) VT values, repetitively. VTi−1 represents VT in the second quintile during +slope, while VTi−1 represents VT in the fourth quintile during −slope. VTi+ and VTi− represent VT in the third quintile during−slope and +slope, respectively, which value is close to the mean of 6 mL/kg. The period pattern P04 was generated similarly, dividing the probability distribution curve into two density regions only.
Accordingly, the pattern of the second sequence showed periodic recurrence every four cycles (P04). Considering a respiratory frequency of 30 min−1 (the average value used in the present study), this periodicity corresponds to a main frequency of 0.13 Hz, coinciding with the dynamics of lung recruitment and stretch induced calcium mobilization (Wirtz and Dobbs, 1990), whereas the third pattern using periodic recurrence every 10 cycles (P10) resulted in a main frequency of 0.05 Hz (considering a respiratory rate 30 min−1) coinciding with dynamics of lung derecruitment and surfactant production and release (Bates and Irvin, 2002; Massa et al., 2008). All three variable VT patterns were applied by remote control of the mechanical ventilator (Evita XL, Dräger Medical, Lübeck, Germany), as described by our group (Spieth et al., 2009b).
Protocol for Measurements
Measurements of gas exchange, hemodynamics, respiratory variables and distribution of ventilation were performed after instrumentation (Baseline 1) and induction of lung injury (Injury). Protective mechanical ventilation was then initiated in volume controlled ventilation mode with the following settings: VT = 6 mL/kg, PEEP = 12 cm H2O, I:E = 1:1, RR ≤ 35 min−1 titrated to maintain pHa > 7.30 and FIO2 = 0.7. After a stabilization period of 30 min, measurements were repeated (Baseline 2). Following that, animals were randomly assigned to one of the following mechanical ventilation: (1) non-variable VT (VCV); (2) variable VT with white noise pattern (WN); (3) variable periodic VT pattern with a periodic recurrence of 4 respiratory cycles (P04); and (4) variable periodic VT pattern with a periodic recurrence of 10 respiratory cycles (P10). Other ventilator settings were kept constant in all groups, and animals ventilated during a period of 6 h, with measurements performed every hour (Times 1–6).
Respiratory System Mechanics
Airflow () was measured by the internal sensor of the mechanical ventilator. Airway pressure (Paw) was monitored by a pressure transducer (163PC01D48-PCB, Sensortechnics GmbH, Germany) placed at proximal side of the tracheal tube. Signals were acquired at sample frequency of 500 Hz using a data acquisition card (NI USB-6210, National Instruments, Austin, TX, USA) connected to a PC, and synchronized off-line according to the maximal co-variance criterion.
Respiratory system resistance (R) and elastance (E = E1+E2·VT) were determined by least-means-squares (LMS) fitting of the respiratory signals to the equation of motion:
where E1 is the volume-independent and E2 the volume-dependent E, and P0 the end-expiratory airway pressure. The contribution of volume-dependent E to total E (%E2) was calculated as described elsewhere (Kano et al., 1994).
The amount of inter-tidal recruitment-/derecruitment (R/D) was estimated from cycle-to-cycle differences in E. A given respiratory cycle was classified as -slope or +slope if its VT was within a range of 6 mL/kg ± 0.045 mL/kg ( and ) and its preceding cycle had a VT value within 7–9 mL/kg () or 3-5 mL/kg (), respectively (Figure 1). The difference in E measured between −slope and +slope cycles was calculated as ΔE = E−slope – E+slope. Negative ΔE-values were classified as inter-tidal recruitment, whereas positive ΔE-values were indicative of inter-tidal derecruitment.
Distribution of Regional Ventilation
Regional ventilation distribution was assessed by electrical impedance tomography (EIT). Detailed methods and analysis of EIT are described in detail in Supplementary Material. Briefly, a 16 electrode belt was placed at mid chest circumference and connected to the EIT Evolution Kit 2 (Draeger Medical AG, Germany). The distribution of relative ventilation in ventral, central and dorsal regions was quantified, and the homogeneity, contrast and energy of tidal images were determined.
Hemodynamics and Gas Exchange
Mean arterial and pulmonary arterial pressures were measured continuously (MAP and MPAP, respectively). Cardiac output (CO) was measured with a pulmonary artery catheter by thermodilution, and oxygen derived variables, including venous admixture, were obtained using standard formulae.
Arterial and mixed venous blood samples were analyzed for respiratory gases and pH using an ABL 505 blood gas analyzer (Radiometer, Copenhagen, Denmark), and for oxygen saturation and hemoglobin concentration with an OSM3 Hemoximeter (Radiometer) calibrated for swine blood. Gas tension measurements were performed at 37°C and corrected for body temperature measured by the pulmonary arterial catheter. To compensate for the influence of varying VT on blood gases, blood samples were drawn along four to five respiratory cycles, 8–10 s respectively. During P10, two subsequent blood samples were taken and values averaged.
Post Mortem Processing
At the end of the observation period, heparin was administered (1,000 IU/kg i.v.) (Ratiopharm, Ulm, Germany) and animals were killed by i.v. injection of 2 g thiopental (Inresa, Arzneimittel GmbH, Freiburg, Germany) followed by 50 mL KCl 1 M (Serumwerk Bernburg, Germany). Lungs were removed maintaining continuous positive airway pressure (CPAP) equal to the PEEP level during the observation period. Samples from gravitationally dependent (dorsal) and non-dependent (ventral) areas of the right lower lung lobe were snap-frozen in liquid nitrogen and stored at −80°C until further analysis. For tissue histologic evaluation, the left lower lung lobe was perfused with 4% buffered formaldehyde solution, while CPAP equivalent to the PEEP level during the observation period was maintained at the airway. Lung tissue samples of ~8 cm3 were taken from ventral and dorsal zones of the left lower lung lobe.
Markers of Inflammation and Mechanical Cell Stress
Total RNA from lung tissue was isolated with TRI reagent (Sigma-Aldrich GmbH, Deisenhof, Germany) according to the manufacturer's protocol, followed by purification with NucleoSpin RNA II columns (Macherey&Nagel, Düren, Germany). The complementary deoxyribonucleic acid was synthesized with the Revert AidTM H Minus First Strand Synthesis Kit (MBI Fermentas, St. Leon Roth, Germany) from 1 μg total RNA according to instructions of the fabricant. Using cyclophilin A and ß2-microglobulin as housekeeping genes, the mRNA expression of the inflammatory mediators tumor necrosis factor α (TNF-α), interleukin 6 and 8 (IL-6, IL-8), amphiregulin and tenascin-c were quantified with quantitative real-time polymerase chain reaction (Maxima SYBR Green qPCR MasterMix, Fermentas, St. Leon Roth, Germany) in the iCycler MyiQ2 real time polymerase chain reaction system (BioRad, Munich, Germany). The total protein content in lung tissue was measured using the BioRad Protein Assay (BioRad, Munich, Germany). Protein levels of TNF-α, IL-6, and IL-8 were measured in lung tissue using commercial ELISA kits (R&D Systems, Wiesbaden, Germany) according to the manufacturer's instructions.
Histological Damage
Following perfusion fixation and immersion in 4% buffered formaldehyde solution for 7 days, tissue samples were embedded in paraffin, cut in slices of 5 μm thickness and stained with hematoxylin-eosin for further analysis. Photomicrographs at magnifications of x25, x100 and x400 were taken from four non-overlapping fields of view per section using a light microscope. Diffuse alveolar damage (DAD) was quantified by one of the authors (MK), who is an expert anatomist and was blinded to the therapy groups, using a weighted scoring system, as described elsewhere (Spieth et al., 2007). Briefly, values from 0 to 5 were used to represent the severity of seven features of DAD, i.e. alveolar edema, interstitial edema, hemorrhage, inflammatory infiltration, epithelial destruction, micro atelectasis and over distension, with 0 standing for no effect and 5 for maximum severity. Additionally, the extent of each feature characteristic per field of view was determined with values of 0 to 5, with 0 standing for no appearance and 5 for complete involvement. The cumulated DAD Score was calculated as the sum of a product of severity and extent of all features, resulting in values within the range from 0 to 175.
Statistical Analysis
The sample size calculations for testing the primary hypothesis (periodic VT variation during variable ventilation improves PaO2/FIO2) was based on effects obtained from pilot studies. Accordingly, a sample size of 10 animals would provide appropriate power (1-β = 0.80) to identify a significant (α = 0.05) mean difference of at least 40 ± 60 mmHg, taking two-tailed tests and multiple comparisons (n = 6) into account (α* = 0.0083, α* Bonferroni adjusted).
Data are presented as mean ± standard deviation, unless stated otherwise. For lung function variables, group differences at Baseline 1, Injury and Baseline 2 were assessed using one-way ANOVA followed by pairwise T-test with p-value adjustment according to Bonferroni. Differences among groups were tested using two factorial repeated measures ANCOVA with between factor VT-pattern, within factor time and covariate of the respective parameter at Baseline 2. Post-hoc analysis and adjustment for multiple comparisons were performed according to Sidak's procedure. Lung histology, gene expression of pro-inflammatory markers as well as their protein levels in lung tissue were assessed using Kruskal–Wallis test followed by pairwise Mann–Whitney U test with adjustment for multiple comparisons according to Bonferroni–Holm procedure. Correlation analysis was performed using linear least mean squares modeling. A p-value of P ≤ 0.05 was considered statistically significant. Statistical analyses was performed using R Statistical programming language (R Core Team, 2016).
Results
Bodyweight (38.2 ± 5.0, 42.1 ± 3.5, 42.4 ± 5.7, and 38.7 ± 6.9 kg in VCV, WN, P04 and P10, respectively) and number of lavages (11, 5–17; 10, 8–13; 10, 4–16, and 12, 5–19; median, min–max for VCV, WN, P04, and P10, respectively) did not differ among groups. Sample signal tracings at the start of therapy for each VT pattern group are depicted in Figure 2.
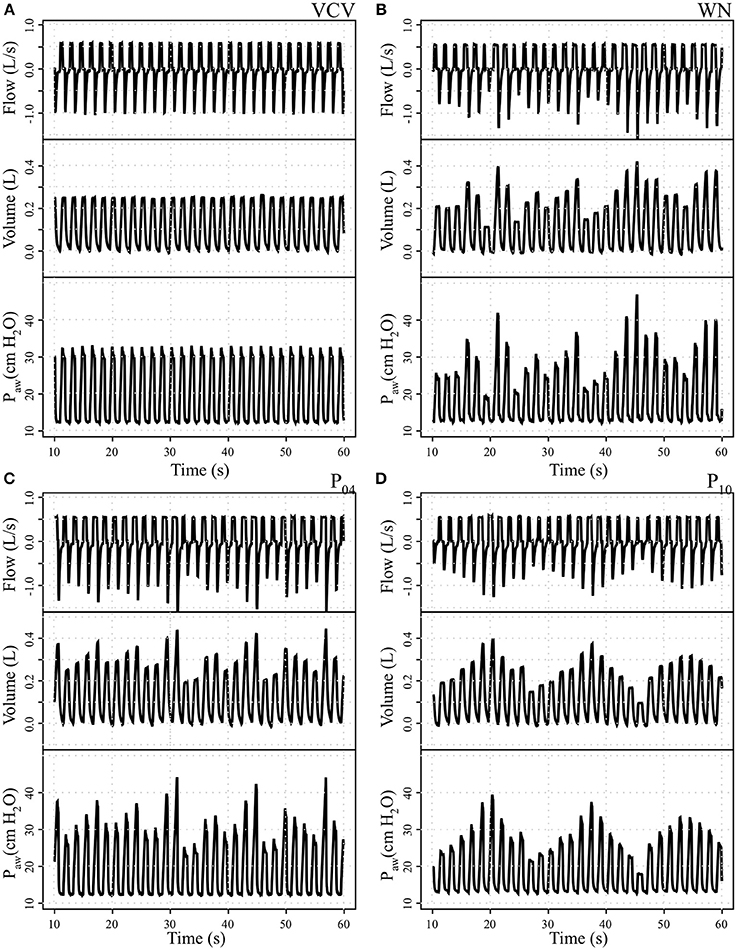
Figure 2. Tracing records of airway flow, volume, and airway pressure (Paw) in one representative animal per group. (A) Conventional volume controlled ventilation (VCV); (B) variable ventilation with Gaussian white noise pattern (WN); (C) variable ventilation with periodicity of 4 cycles (P04) and (D) variable ventilation with periodicity of 10 cycles (P10).
Hemodynamics and gas exchange variables also did not differ among groups, except to PaO2/FIO2, which was higher in P10 compared to VCV (Figure 3 and Table 2).
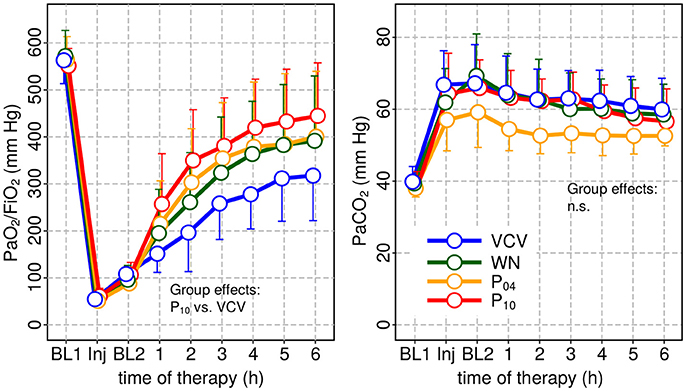
Figure 3. Arterial oxygenation (PaO2/FIO2) and arterial carbon-dioxide partial pressure PaCO2 at baseline 1 (BL1), injury and baseline 2 (BL2) and during subsequent therapy. PaO2 was significantly increased only for P10 compared to VCV.
Peak airway pressure Paw, peak, plateau airway pressure Paw, plat and mean airway pressure Paw, mean were significantly reduced in WN, P04 and P10 compared to VCV. E, E2, and %E2 were reduced in WN, P04 and P10 compared to VCV, whereas R did not differ among groups (Table 3 and Figure 4). In VCV, WN and P04, but not P10, correlation analyses revealed that PaO2/FIO2 decreased proportionally to E (Figure 5).
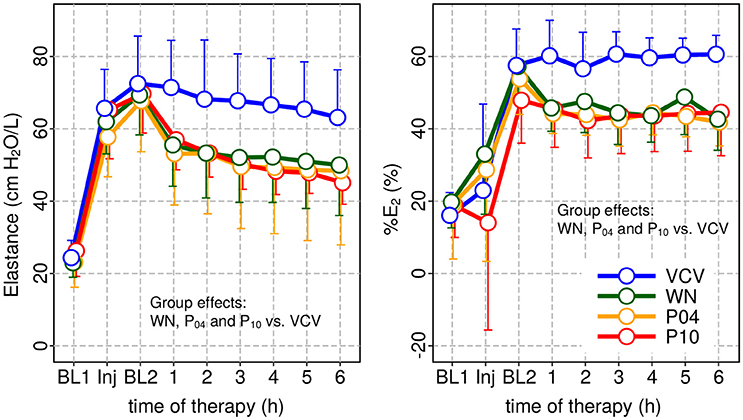
Figure 4. Dynamic respiratory system elastance (E) and relative volume dependence of E (%E2) at baseline 1 (BL1), injury and baseline 2 and during subsequent therapy period. In all patterns of variable ventilation E and %E2 were significantly reduced compared to VCV.
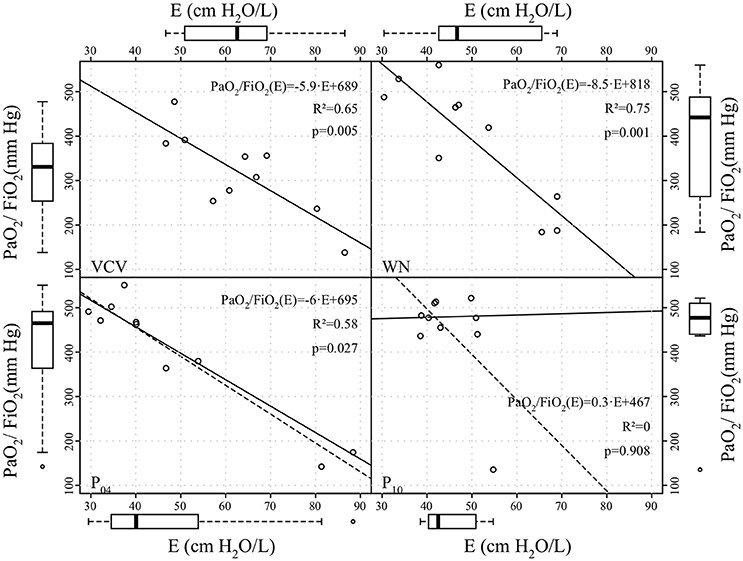
Figure 5. Correlation between arterial partial oxygen pressure to inspiratory oxygen fraction ratio (PaO2/FiO2) and dynamic respiratory system elastance (E) at end of the therapy period. Straight continuous and dashed lines represent linear regression lines without and with outliers, respectively; the coefficients of determination (R2) were calculated excluding outliers.
Compared to WN, ΔE was significantly higher within the P10 pattern, although the tidal volume of the respective preceding cycle ΔVT, i−1 was lowest in the P10 group (Figure 6).
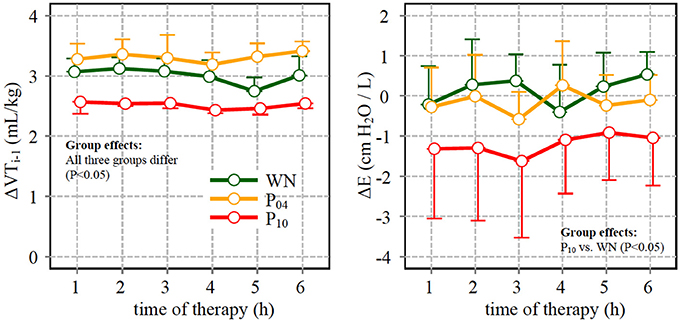
Figure 6. Left: difference between tidal volume (VT) in the descending limb of a given cycle and in the ascending limb of the preceding cycle (ΔVT,i−1) (see also text and Figure 1); Right: difference of dynamic respiratory system elastance (ΔE) calculated for cycles with VT value close to the mean (6 mL/kg) in the descending and in the ascending slopes (VTi- and VTi+, respectively) of the respective pattern.
The distribution of regional ventilation did not differ significantly among groups. Analysis of tidal EIT images showed that homogeneity and energy increased, whereas contrast decreased during variable ventilation compared to VCV, irrespective of the VT pattern (see Supplementary Material).
DAD Score (Table 4 and Figure 7), gene expression of markers of inflammation and cell mechanical stress (Table 5), and protein content of pro-inflammatory markers in lung tissue (Table 6), did not differ significantly among groups.
Discussion
The main finding of this study was that, in an experimental model of severe ARDS in pigs, periodic VT fluctuation at a frequency of 0.05 Hz enhanced oxygenation and increased inter-tidal R/D during variable ventilation.
To our knowledge, this is the first study comparing different types of variable ventilation with periodic VT fluctuation in a large animal model of severe ARDS. Three tidal volume patterns with distinct amplitude-frequency spectra were compared to conventional controlled mechanical ventilation. While WN has, by definition, a constant, frequency independent spectrum amplitude, there were distinct peaks in the spectra of the pattern P04 and P10 at one quarter (fP04 = 0.13 Hz) and one tenth (fP10 = 0.05 Hz) of the average respiratory frequency (0.5 Hz), respectively. In mice with hydrochloric acid injured lungs, a decaying probability density distribution tailored toward high VT values outperformed the original variable ventilation pattern based on a Gaussian distribution with respect to lung mechanics and gas exchange (Thammanomai et al., 2008, 2013), likely due to lung recruitment (Graham et al., 2011b). Different from these previous studies, we selected VT patterns with identical probability density distributions.
Our data showed that oxygenation was significantly improved only in P10, when VT fluctuated at a frequency of 0.05 Hz. In contrast, E was significantly reduced in all variable ventilation groups compared to VCV, a finding that is in line with previous studies (Arold et al., 2002; Funk et al., 2004; Spieth et al., 2009b). The correlation analysis revealed that, with increasing VT fluctuation period, variations in PaO2/FiO2 were less well explained by variations in E. There are two possible explanations for this observation: (1) with increasing period lung recruitment was more stable over multiple respiratory cycles, for example due to enhanced surfactant production/release; (2) periodic VT fluctuation redistributed perfusion more effectively, improving ventilation/perfusion matching.
To investigate whether inter-tidal R/D cycling occurs along with periodic VT variation we performed the cycle-type analysis selecting respiratory cycles with mean VT whose preceding cycle had a higher or lower VT, −slope or +slope. Due to the selection criteria that were chosen in order to gain a comparable number of cycles independent of the VT pattern and cycle class, the difference of VT of the preceding cycles Δ VT,i+1 differed between VT patterns being significantly lower in P10 compared to P04 and WN. Therefore, ΔE should be of lesser magnitude for P10 compared to the other patterns, if inter-tidal R/D would be absent. However while ΔE was not significantly different from zero in patterns WN and P04, it was significantly negative in P10 (Figure 6), indicating the presence of inter-tidal R/D of two or more cycles in P10. It is worth noting that this type of analysis would yield differences between −slope and +slope cycles in every viscoelastic tissue or material with relevant hysteresis independent of R/D. However the increased difference between cycle types is more likely due to a type of prolonged R/D dynamics in agreement with (Bellardine et al., 2006), as P10 proved to be the only type of variable ventilation able to improve oxygenation. This may suggest that R/D on a shorter time scale, which may occur during ventilation with reduced or no periodicity in tidal volume patterns, is less effective in improving lung function in this type of injury.
The reduction of the frequency VT pattern amplified arterial oxygenation PaO2. The possible mechanism may be stabilization of lung recruitment by increased inter-tidal but decreased intra-tidal R/D. But other factors such as surfactant production/release and redistribution of perfusion may be triggered by periodic VT variation and may contribute additionally to improvements in lung function. However arterial oxygenation may not increase during an indefinite reduction of VT pattern frequency, since a VT frequency of zero corresponds to conventional volume controlled ventilation. If the VT frequency of maximal arterial oxygenation coincides with the frequency response of one participating subsystem of respiration, this frequency dependent response would be characteristic for deterministic resonance. The identification of such frequency and related subsystem were beyond the scope of this investigation. However further research on this issue would not only foster our knowledge of the respiratory system and its interactions but would facilitate further development of protective ventilation strategies.
Several mechanisms may explain the improvement of gas exchange and lung mechanics through periodic VT patterns. Lung recruitment can be facilitated by surfactant release due to stretch of lung epithelial type II cells. Its magnitude is related to an instantaneous Ca2+ mobilization decaying with a time constant of 9.13 s (Wirtz and Dobbs, 1990) and simultaneous surfactant secretion with a time constant of 19.7 s (Haller et al., 1998) to 94.6 s (Majumdar et al., 2012). Therefore, in lung areas subjected to inter-tidal R/D high VT cycles may activate surfactant secretion more effectively, while this surfactant is released after the strain impulse has decayed during subsequent low VT cycles (Edwards, 2001).
In contrast to intra-tidal, inter-tidal R/D may have beneficial effects on ventilation/perfusion matching due to redistribution of perfusion toward lung regions with improved ventilation (Sylvester et al., 2012). The transient response of local acute hypoxic pulmonary (HPV) vasoconstriction is characterized by a time constant of 151 ± 24.8 s in humans (Morrell et al., 1995) and 120 s in dogs (Grant and Schneider, 1983). Thus, only 1.6% of the final HPV response is reached during intra-tidal R/D (approximately T = 2 s), whereas 8% is reached in regions that remain open/closed for ~10 s, which was likely achieved in P10.
The dynamics of formation and resolution of atelectasis differ according to the type of lung injury, as well as PEEP levels. In a lung lavage model in pigs, duration of atelectasis formation varied from 20.0 ± 12.3 to 26.5 ± 14.0 s, while duration of atelectasis resolution ranged from 0.8 ± 0.6 to 10.7 ± 4.2 s (Neumann et al., 1998; Markstaller et al., 2001). This likely reduced cyclic R/D, explaining in part the benefits of the lower main frequency during variable ventilation. Possibly, a prolongation of the VT period up to a TP = 40 s (fP = 0.025 Hz) might yield a further increase on oxygenation, but this claim remains speculative.
The effects of periodicity of VT patterns on gas exchange and lung mechanics have been investigated in one experimental and one simulation study. In pigs with oleic acid-induced lung injury, biologically and white noise, computer-generated variable ventilation led to comparable lung mechanics and gas exchange (Froehlich et al., 2008). However, the so-called Hurst exponent H, i.e. a measure of long range correlation, might not have differed relevantly between both patterns (H = 0.62 for biological noise and H = 0.5 for computer generated white noise) considering its spectrum of possible values for H = 0 anti-correlation (so-called blue noise), H = 0.5 (no correlation, white noise, respiratory rate during deep sleep) and H = 1 auto-correlation (so-called pink noise, respiratory rate during awake state; Schumann et al., 2010).
In the presented study the novelty was that we introduced a deterministic auto-correlation with a fixed frequency similar to the numerical simulation study by Ma et al. In their model, taking R/D dynamics into account, increased periodicity did not improve lung mechanics. However, different from in-vivo models, other possible factors affected by periodicity, for example surfactant release and redistribution of perfusion and ventilation were not considered in their model (Ma et al., 2011).
Although changes in the distribution of tidal ventilation did not reach statistical significance, increased homogeneity in tidal impedance images in all variability groups suggests increased lung homogeneity during variable ventilation. However, in order to avoid artifacts of the moving diaphragm, a rather cranial, cross section of the lung was addressed, and we cannot exclude the possibility that R/D occurred in the most dependent (dorsal and caudal) lung regions, contributing to the observed effects of variable ventilation on lung function.
No relevant differences among groups were found regarding lung histology, gene expression as well as protein levels of markers of inflammation in lung tissue, which is somewhat in disagreement with previous work from our own group (Spieth et al., 2009b). A possible explanation is that in the present study we used a model of severe ARDS, which might be less responsive to variable ventilation.
The improvement of oxygenation through periodic variable ventilation ΔPaO2/FIO2 = 150 mmHg, seen in this study, compares well to other techniques as for example random variable ventilation ΔPaO2/FIO2 = 80 … 150 mm Hg (Huhle et al., 2016) and step-wise recruitment maneuvers' ΔPaO2/FIO2 = 150 mm Hg(Vivona et al., 2018) in the same model. In the double-hit model facilitated in this study the effects of recruitment on oxygenation might still be over-estimated compared to clinical ARDS where recruitment maneuvers' only improve oxygenation by ΔPaO2/FIO2 = 40 … 50 mm Hg (Hodgson et al., 2011). Furthermore the full benefits of periodic variable ventilation and the relevant mechanism remain to be elucidated.
Periodic variable ventilation is straightforward implementable in experimental and conventional ventilators that are remotely controllable as e.g., the Inspira ASVp (Harvard Apparatus, Massachusetts, USA), the FlexiVent (SciReq, Montreal, Canada) and Evita XL (Draeger Medical, Luebeck, Germany).
Limitations
Our study has several limitations. First, the applied experimental model of ARDS consisted of repeated surfactant lavage (first hit) followed by consecutive ventilator induced lung injury (second hit). This model of ARDS reproduces important features of human ARDS (Matute-Bello et al., 2008), but it does not reflect the complex clinical picture of ARDS precluding direct extrapolation of our results to the clinical scenario. Second, the observational period was limited to 6 h, and we cannot exclude different effects in the long term. Third, to enhance comparability between groups, we kept settings for FIO2 and PEEP constant during the observational period avoiding adjustments based on changes in gas exchange. A clinical approach including such adaptations may have produced different results. Fourth, a further prolongation of the VT period may have yielded different effects of periodicity on lung function in this animal model, but were not investigated. Last but not least the Gaussian distribution of the VT patterns used might not be tailored to the species and model of ARDS.
Conclusions
In this experimental model of severe ARDS, periodic VT fluctuation at a frequency of 0.05 Hz improved oxygenation during variable ventilation, suggesting that deterministic resonance adds further benefit to variable ventilation.
Data Availability Statement
The datasets used and/or analyzed during the current study are available from the corresponding author on reasonable request.
Author Contributions
AG and RH contributed to design the study, conducted experiments, analyzed, and interpreted all experimental data, and were major contributors in writing the manuscript. AB contributed to design the study, analyzed and interpreted data on respiratory mechanics, and contributed in writing the manuscript. TK and TB conducted experiments, analyzed and interpreted all experimental data, and were major contributors in writing the manuscript. IR contributed to design the study, analyzed, and interpreted data on molecular biology, and contributed in writing the manuscript. SK and NC contributed to design the study, analyze all data and write the manuscript. MK contributed to design the study, to analyze data on histologic damage, and contributed in writing the manuscript. PP contributed to design the study, analyzed and interpreted all experimental data, and was a major contributor in writing the manuscript. MA designed the study, obtained financial support, analyzed and interpreted all experimental data, and was a major contributor in writing the manuscript. All authors read and approved the final manuscript.
Funding
This study was funded by grant nr. GA 1256/7-1 from the Deutsche Forschungsgemeinschaft, Bonn, Germany.
Conflict of Interest Statement
MA has been granted patents on variable pressure support ventilation, which were licensed to Dräger Medical AG, Lübeck, Germany.
The remaining authors declare that the research was conducted in the absence of any commercial or financial relationships that could be construed as a potential conflict of interest.
Acknowledgments
The authors are thankful to Susanne Henninger Abreu, research nurse, and Sabine Müller, laboratory technician, of the Pulmonary Engineering Group at the Department of Anesthesiology and Intensive Care, University Hospital Carl Gustav Carus, Technische Universität Dresden, Dresden, Germany, for their support during experiments and laboratory analyses.
Supplementary Material
The Supplementary Material for this article can be found online at: https://www.frontiersin.org/articles/10.3389/fphys.2018.00905/full#supplementary-material
Abbreviations
ARDS, Acute respiratory distress syndrome; CPAP, Continuous positive airway pressure ventilation mode; CV, Coefficient of variation in %, defined as CV =*100 with VT,I; CVP, Central venous pressure in mm Hg; DAD, Diffuse alveolar damage score; E, Total respiratory system elastance: E = E1 + E2 · VT in cm H2Oml−1; E1, Volume independend respiratory system elastance in cm H2O·ml−1; E2, Volume dependend respiratory system elastance in cm H2O·ml−2; %E2, Volume dependence index of respiratory system elastance in %; HR, Heart rate in min−1; MAP, Mean arterial pressure in mm Hg; MPAP, Mean pulmonary arterial pressure in mm Hg; P0, Minimal airway pressure per respiratory cycle in cm H2O; WN, P04, P10, Pattern of variable ventilation without, every 4 and every 10 resp. cycles recurrence (periodicity); Paw, mean|plat|max, Minimal, mean, plateau and maximal airway pressure in cm H2O; PCWP, Pulmonary capillary wedge pressure in mm Hg; PEEP, Positive end-expiratory pressure in cm H2O; R, Respiratory system resistance in cm H2O·s·ml−1; R/D, Recruitment / derecruitment: repeated opening and closing of lung regions; RR, Respiratory rate set at the ventilator in min−1; VCV, Conventional volume controlled ventilation; VT, Mean inspiratory tidal volume in ml·kg−1 actual body weight; VT, I, Inspiratory tidal volume of the i-th respiratory cycle.
References
Arold, S. P., Mora, R., Lutchen, K. R., Ingenito, E. P., and Suki, B. (2002). Variable tidal volume ventilation improves lung mechanics and gas exchange in a rodent model of acute lung injury. Am. J. Respir. Crit. Care Med. 165, 366–371. doi: 10.1164/ajrccm.165.3.2010155
Bates, J. H., and Irvin, C. G. (2002). Time dependence of recruitment and derecruitment in the lung: a theoretical model. J. Appl. Physiol. 93, 705–713. doi: 10.1152/japplphysiol.01274.2001
Bellardine, C. L., Hoffman, A. M., Tsai, L., Ingenito, E. P., Arold, S. P., Lutchen, K. R., et al. (2006). Comparison of variable and conventional ventilation in a sheep saline lavage lung injury model. Crit. Care Med. 34, 439–445. doi: 10.1097/01.CCM.0000196208.01682.87
Boker, A., Graham, M. R., Walley, K. R., McManus, B. M., Girling, L. G., Walker, E., et al. (2002). Improved arterial oxygenation with biologically variable or fractal ventilation using low tidal volumes in a porcine model of acute respiratory distress syndrome. Am. J. Respir. Crit. Care Med. 165, 456–462. doi: 10.1164/ajrccm.165.4.2108006
Brewster, J. F., Graham, M. R., and Mutch, W. A. C. (2005). Convexity, Jensen's inequality and benefits of noisy mechanical ventilation. J. R. Soc. Interface R. Soc. 2, 393–396. doi: 10.1098/rsif.2005.0043
de Magalhães, R. F., Samary, C. S., Santos, R. S., de Oliveira, M. V., Rocha, N. N., Santos, C. L., et al. (2016). Variable ventilation improves pulmonary function and reduces lung damage without increasing bacterial translocation in a rat model of experimental pneumonia. Respir. Res. 17:158. doi: 10.1186/s12931-016-0476-7
Edwards, Y. S. (2001). Stretch stimulation: its effects on alveolar type II cell function in the lung. Comp. Biochem. Physiol. A. Mol. Integr. Physiol. 129, 245–260. doi: 10.1016/S1095-6433(01)00321-X
Froehlich, K. F., Graham, M. R., Buchman, T. G., Girling, L. G., Scafetta, N., West, B. J., et al. (2008). Physiological noise versus white noise to drive a variable ventilator in a porcine model of lung injury. Can. J. Anaesth. 55, 577–586. doi: 10.1007/BF03021431
Funk, D., Graham, M. R., Girling, L., Thliveris, J., McManus, B., Walker, E., et al. (2004). A comparison of biologically variable ventilation to recruitment manoeuvres in a porcine model of acute lung injury. Respir. Res. 5:22. doi: 10.1186/1465-9921-5-22
Graham, M. R., Goertzen, A. L., Girling, L. G., Friedman, T., Pauls, R. J., Dickson, T., et al. (2011a). Quantitative computed tomography in porcine lung injury with variable versus conventional ventilation: recruitment and surfactant replacement. Crit. Care Med. 39, 1721–1730. doi: 10.1097/CCM.0b013e3182186d09
Graham, M. R., Gulati, H., Kha, L., Girling, L. G., Goertzen, A., and Mutch, W. A. (2011b). Resolution of pulmonary edema with variable mechanical ventilation in a porcine model of acute lung injury. Can. J. Anaesth. 58, 740–750. doi: 10.1007/s12630-011-9517-3
Grant, B. J., and Schneider, A. M. (1983). Dynamic response of local pulmonary blood flow to alveolar gas tensions: analysis. J. Appl. Physiol. 54, 445–452.
Haller, T., Ortmayr, J., Friedrich, F., Völkl, H., and Dietl, P. (1998). Dynamics of surfactant release in alveolar type II cells. Proc. Natl. Acad. Sci. U.S.A. 95, 1579–1584. doi: 10.1073/pnas.95.4.1579
Hodgson, C. L., Tuxen, D. V., Davies, A. R., Bailey, M. J., Higgins, A. M., Holland, A. E., et al. (2011). A randomised controlled trial of an open lung strategy with staircase recruitment, titrated PEEP and targeted low airway pressures in patients with acute respiratory distress syndrome. Crit. Care 15:R133. doi: 10.1186/cc10249
Huhle, R., Pelosi, P., and de Abreu, M. G. (2016). Variable ventilation from bench to bedside. Crit. Care 20:62. doi: 10.1186/s13054-016-1216-6
Kano, S., Lanteri, C. J., Duncan, A. W., and Sly, P. D. (1994). Influence of nonlinearities on estimates of respiratory mechanics using multilinear regression analysis. J. Appl. Physiol. 77, 1185–1197.
Kiss, T., Silva, P. L., Huhle, R., Moraes, L., Santos, R. S., Felix, N. S., et al. (2016). Comparison of different degrees of variability in tidal volume to prevent deterioration of respiratory system elastance in experimental acute lung inflammation. Br. J. Anaesth. 116, 708–715. doi: 10.1093/bja/aew093
Lefevre, G. R., Kowalski, S. E., Girling, L. G., Thiessen, D. B., and Mutch, W. A. (1996). Improved arterial oxygenation after oleic acid lung injury in the pig using a computer-controlled mechanical ventilator. Am. J. Respir. Crit. Care Med. 154, 1567–1572. doi: 10.1164/ajrccm.154.5.8912782
Ma, B., Suki, B., and Bates, J. H. (2011). Effects of recruitment/derecruitment dynamics on the efficacy of variable ventilation. J. Appl. Physiol. 110, 1319–1326. doi: 10.1152/japplphysiol.01364.2010
Majumdar, A., Arold, S. P., Bartolák-Suki, E., Parameswaran, H., and Suki, B. (2012). Jamming dynamics of stretch-induced surfactant release by alveolar type II cells. J. Appl. Physiol. 112, 824–831. doi: 10.1152/japplphysiol.00975.2010
Markstaller, K., Eberle, B., Kauczor, H.-U., Scholz, A., Bink, A., Thelen, M., et al. (2001). Temporal dynamics of lung aeration determined by dynamic CT in a porcine model of ARDS. Br. J. Anaesth. 87, 459–468. doi: 10.1093/bja/87.3.459
Massa, C. B., Allen, G. B., and Bates, J. H. (2008). Modeling the dynamics of recruitment and derecruitment in mice with acute lung injury. J. Appl. Physiol. 105, 1813–1821. doi: 10.1152/japplphysiol.90806.2008
Matute-Bello, G., Frevert, C. W., and Martin, T. R. (2008). Animal models of acute lung injury. Am. J. Physiol. Lung Cell. Mol. Physiol. 295, L379–L399. doi: 10.1152/ajplung.00010.2008
Morrell, N. W., Nijran, K. S., Biggs, T., and Seed, W. A. (1995). Magnitude and time course of acute hypoxic pulmonary vasoconstriction in man. Respir. Physiol. 100, 271–281.
Mutch, W. A. C., Graham, M. R., Girling, L. G., and Brewster, J. F. (2005). Fractal ventilation enhances respiratory sinus arrhythmia. Respir. Res. 6:41. doi: 10.1186/1465-9921-6-41
Mutch, W. A., Harms, S., Lefevre, G. R., Graham, M. R., Girling, L. G., and Kowalski, S. E. (2000). Biologically variable ventilation increases arterial oxygenation over that seen with positive end-expiratory pressure alone in a porcine model of acute respiratory distress syndrome. Crit. Care Med. 28, 2457–2464. doi: 10.1097/00003246-200007000-00045
Neumann, P., Berglund, J. E., Fernández Mondéjar, E., Magnusson, A., and Hedenstierna, G. (1998). Dynamics of lung collapse and recruitment during prolonged breathing in porcine lung injury. J. Appl. Physiol. 85, 1533–1543. doi: 10.1152/jappl.1998.85.4.1533
R Core Team (2016). R: A Language and Environment for Statistical Computing. Vienna: R Foundation for Statistical Computing. Available online at: http://www.R-project.org/
Samary, C. S., Moraes, L., Santos, C. L., Huhle, R., Santos, R. S., Ornellas, D. S., et al. (2016). Lung functional and biologic responses to variable ventilation in experimental pulmonary and extrapulmonary acute respiratory distress syndrome. Crit. Care Med. 44:e553-62. doi: 10.1097/CCM.0000000000001611
Schumann, A. Y., Bartsch, R. P., Penzel, T., Ivanov, P. C., and Kantelhardt, J. W. (2010). Aging effects on cardiac and respiratory dynamics in healthy subjects across sleep stages. Sleep 33, 943–955. doi: 10.1093/sleep/33.7.943
Silva, P. L., Güldner, A., Uhlig, C., Carvalho, N., Beda, A., Rentzsch, I., et al. (2013). Effects of intravascular volume replacement on lung and kidney function and damage in nonseptic experimental lung injury. Anesthesiology 118, 395–408. doi: 10.1097/ALN.0b013e31827e554c
Spieth, P. M., Carvalho, A. R., Güldner, A., Pelosi, P., Kirichuk, O., Koch, T., et al. (2009a). Effects of different levels of pressure support variability in experimental lung injury. Anesthesiology 110, 342–350. doi: 10.1097/ALN.0b013e318194d06e
Spieth, P. M., Carvalho, A. R., Pelosi, P., Hoehn, C., Meissner, C., Kasper, M., et al. (2009b). Variable tidal volumes improve lung protective ventilation strategies in experimental lung injury. Am. J. Respir. Crit. Care Med. 179, 684–693. doi: 10.1164/rccm.200806-975OC
Spieth, P. M., Knels, L., Kasper, M., Domingues Quelhas, A., Wiedemann, B., Lupp, A., et al. (2007). Effects of vaporized perfluorohexane and partial liquid ventilation on regional distribution of alveolar damage in experimental lung injury. Intensive Care Med. 33, 308–314. doi: 10.1007/s00134-006-0428-7
Suki, B., Alencar, A. M., Sujeer, M. K., Lutchen, K. R., Collins, J. J., Andrade, J. S. Jr., et al. (1998). Life-support system benefits from noise. Nature 393, 127–128.
Sylvester, J. T., Shimoda, L. A., Aaronson, P. I., and Ward, J. P. T. (2012). Hypoxic pulmonary vasoconstriction. Physiol. Rev. 92, 367–520. doi: 10.1152/physrev.00041.2010
Thammanomai, A., Hamakawa, H., Bartolák-Suki, E., and Suki, B. (2013). Combined effects of ventilation mode and positive end-expiratory pressure on mechanics, gas exchange and the epithelium in mice with acute lung injury. PloS ONE 8:e53934. doi: 10.1371/journal.pone.0053934
Thammanomai, A., Hueser, L. E., Majumdar, A., Bartolák-Suki, E., and Suki, B. (2008). Design of a new variable-ventilation method optimized for lung recruitment in mice. J. Appl. Physiol. 104, 1329–1340. doi: 10.1152/japplphysiol.01002.2007
Vivona, L., Braune, A., Bluth, T., Kiss, T., Kircher, M., Boszak, C., et al. (2018). Variable ventilation is superior to conventional recruitment for reversal of atelectasis in anesthetized pigs. Am. J. Resp. Crit. Care Med. 197:A5122.
Keywords: experimental model, acute respiratory distress syndrome, mechanical ventilation, variable ventilation, gas exchange, respiratory mechanics, lung inflammation, lung damage
Citation: Güldner A, Huhle R, Beda A, Kiss T, Bluth T, Rentzsch I, Kerber S, Carvalho NC, Kasper M, Pelosi P and de Abreu MG (2018) Periodic Fluctuation of Tidal Volumes Further Improves Variable Ventilation in Experimental Acute Respiratory Distress Syndrome. Front. Physiol. 9:905. doi: 10.3389/fphys.2018.00905
Received: 23 April 2018; Accepted: 21 June 2018;
Published: 12 July 2018.
Edited by:
Walter Araujo Zin, Universidade Federal do Rio de Janeiro, BrazilReviewed by:
Ramon Farre, University of Barcelona, SpainBela Suki, Boston University, United States
Sabah Hussain, McGill University, Canada
Copyright © 2018 Güldner, Huhle, Beda, Kiss, Bluth, Rentzsch, Kerber, Carvalho, Kasper, Pelosi and de Abreu. This is an open-access article distributed under the terms of the Creative Commons Attribution License (CC BY). The use, distribution or reproduction in other forums is permitted, provided the original author(s) and the copyright owner(s) are credited and that the original publication in this journal is cited, in accordance with accepted academic practice. No use, distribution or reproduction is permitted which does not comply with these terms.
*Correspondence: Marcelo G. de Abreu, bWdhYnJldUB1a2RkLmRl
†These authors have contributed equally to this work.