- 1Global Diving Research, Ottawa, ON, Canada
- 2Fundación Oceanografic de la Comunidad Valenciana, Valencia, Spain
- 3Aarhus Institute of Advanced Studies, Aarhus University, Aarhus, Denmark
- 4Sea Mammal Research Unit, Scottish Oceans Institute, University of St Andrews, St Andrews, United Kingdom
- 5Chicago Zoological Society's Sarasota Dolphin Research Program, Mote Marine Laboratory, Sarasota, FL, United States
Bottlenose dolphins (Tursiops truncatus) are highly versatile breath-holding predators that have adapted to a wide range of foraging niches from rivers and coastal ecosystems to deep-water oceanic habitats. Considerable research has been done to understand how bottlenose dolphins manage O2 during diving, but little information exists on other gases or how pressure affects gas exchange. Here we used a dynamic multi-compartment gas exchange model to estimate blood and tissue O2, CO2, and N2 from high-resolution dive records of two different common bottlenose dolphin ecotypes inhabiting shallow (Sarasota Bay) and deep (Bermuda) habitats. The objective was to compare potential physiological strategies used by the two populations to manage shallow and deep diving life styles. We informed the model using species-specific parameters for blood hematocrit, resting metabolic rate, and lung compliance. The model suggested that the known O2 stores were sufficient for Sarasota Bay dolphins to remain within the calculated aerobic dive limit (cADL), but insufficient for Bermuda dolphins that regularly exceeded their cADL. By adjusting the model to reflect the body composition of deep diving Bermuda dolphins, with elevated muscle mass, muscle myoglobin concentration and blood volume, the cADL increased beyond the longest dive duration, thus reflecting the necessary physiological and morphological changes to maintain their deep-diving life-style. The results indicate that cardiac output had to remain elevated during surface intervals for both ecotypes, and suggests that cardiac output has to remain elevated during shallow dives in-between deep dives to allow sufficient restoration of O2 stores for Bermuda dolphins. Our integrated modeling approach contradicts predictions from simple models, emphasizing the complex nature of physiological interactions between circulation, lung compression, and gas exchange.
Introduction
The physiological adaptations that optimize foraging in marine mammals have long interested researchers. Optimal foraging theory implies that marine mammals should change dive behavior and metabolic pathways, and the fraction of aerobic and anaerobic metabolism based on dive depth and prey availability (Carbone and Houston, 1996; Cornick and Horning, 2003). Considerable work has been dedicated to understanding the aerobic limitations of diving air-breathing vertebrates, as these help with understanding foraging limits and efficiency. Kooyman et al. (1983) described the maximum dive duration until increasing blood lactate levels as the aerobic dive limit (ADL). The calculated ADL (cADL) was later defined as the total O2 stores divided by the rate of O2 consumption (Butler and Jones, 1997), and has been estimated in a number of species (Kooyman and Ponganis, 1998; Butler, 2006). However, metabolic rate may change over the course of a dive or foraging bout, and a few studies have subsequently estimated the cADL from measured (respirometry: Castellini et al., 1992; Reed et al., 1994, 2000; Hurley and Costa, 2001; Sparling and Fedak, 2004; Fahlman et al., 2008a, 2013) or estimated diving metabolic rate (doubly labeled water, or a proxy of metabolic rate: Boyd et al., 1995; Froget et al., 2001; Butler et al., 2004; Fahlman et al., 2008b).
Most studies agree that the majority of dive durations are well within the ADL/cADL, as this increases foraging efficiency and reduces lengthy surface intervals required to remove accumulated anaerobic by-products such as lactate (Kooyman et al., 1983). However, some species of otariids that feed on the benthos appear to exceed their cADL regularly, while those that feed in shallower water have shorter dive durations and seldom exceed the cADL (Costa et al., 2001). These differences may indicate true variation in foraging behavior, but may also be suggestive of morphological or physiological differences within or between closely related species that alter cADL (Hückstädt et al., 2016). For example, the muscle mass in previous studies was assumed to be similar to that of the Weddell seal (Costa et al., 2001), and such assumptions are often necessary as available data do not exist for all variables and species. However, variation in underwater swimming behavior (Williams, 2001; Fahlman et al., 2013), or morphological variation in muscle mass and fiber type (Pabst et al., 2016) may significantly alter the metabolic cost of foraging.
Large variations in dive behavior also exist within species. In the common bottlenose dolphin (Tursiops truncatus), different ecotypes have evolved to occupy different ecological niches (Mead and Potter, 1995; Hoelzel et al., 1998). Coastal bottlenose dolphins inhabit coastal areas and generally perform short (<60 s), shallow (<10 m) dives (Mate et al., 1995), while offshore bottlenose dolphins inhabit offshore, deep-water habitats and regularly dive below 200 m (Klatsky et al., 2007). Interestingly, neither resting metabolic rate nor lung compliance differed between the two ecotypes (Fahlman et al., 2018a,b), but blood hematocrit was significantly higher in the offshore ecotype (Klatsky et al., 2007; Schwacke et al., 2009; Fahlman et al., 2018a). In addition, the offshore ecotype is generally larger (Klatsky et al., 2007), possibly due to larger muscle mass to help increase the available O2 stores and diving capacity. In an attempt to better understand the physiological limitations of these two ecotypes, and to explore how variation in morphology and anatomy may alter gas dynamics during diving, we used a previously published gas exchange model (Fahlman et al., 2009; Hooker et al., 2009; Hodanbosi et al., 2016) to estimate blood and tissue gas tensions for O2 (PO2), CO2 (PCO2), and N2 (PN2) from high resolution (1 Hz) dive records from coastal bottlenose dolphins from Sarasota Bay, Florida, and offshore bottlenose dolphins sampled near the island of Bermuda.
Materials and Methods
Model
The model described in this paper uses the breath-hold diving gas dynamics model developed by Fahlman et al. (2009) and Fahlman et al. (2006), which has been used to estimate lung, blood, and tissue gas tensions in a number of species (Hooker et al., 2009; Kvadsheim et al., 2012; Hodanbosi et al., 2016). A brief summary of the model is included below, with the specific changes made for the current modeling effort. The model was parameterized for bottlenose dolphins and was based on published values available for this species when possible, and otherwise on published values for beaked whales or phocids (as detailed below).
As in previous work, the body was partitioned into 4 compartments; brain (B), fat (F), muscle (M), and central circulation (CC), and one blood compartment (BL, arterial and mixed venous). In the current study, bone was included in the fat compartment as the bones of deep diving whales are high in fat content (Higgs et al., 2010). The central circulatory compartment included heart, kidney, and liver. The muscle compartment included muscle, skin, connective tissue, and all other tissues (Fahlman et al., 2009). In previous studies, the alimentary tract was placed in the central circulation. However, due to its lower metabolic rate, it was placed in the muscle compartment in the current study. The size of each compartment as well as the myoglobin and hemoglobin concentrations were initially taken from data on coastal dolphins (Mallette et al., 2016). As there is little or no information about the body composition of offshore dolphins, changes were made to the body composition of the offshore ecotype to be more like a beaked whale (Pabst et al., 2016).
Lung Gas Stores and Gas Exchange
Gas exchange occurred between the lungs and blood compartment and between the blood compartment and each other compartment (Fahlman et al., 2006). The O2, CO2, and N2 stores in the lung consisted only of a gas phase and were assumed to be homogenous. We assumed that there was no diffusion resistance at the lung-surface interface when an animal was breathing at the water surface (Farhi, 1967). Thus, arterial blood tension of N2 (PaN2), O2 (PaO2), and CO2 (PaCO2) were assumed to be equal to the alveolar partial pressures. For an animal breathing at the surface, we assumed that alveolar partial pressures of N2 (PAN2), O2 (PAO2), and CO2 (PACO2) were, respectively, 0.74 ATA, 0.133 ATA, and 0.065 ATA (Fahlman et al., 2015), with 0.062 ATA being water vapor.
During diving, hydrostatic pressure compresses the respiratory system, which causes a pressure-dependent pulmonary shunt to develop (Fahlman et al., 2009). The parameters that describe the structural properties for the alveolar space and conducting airways (Equations 4 and 5 in Bostrom et al., 2008) were updated based on previously published compliance values for the bottlenose dolphin (Table 1, Fahlman et al., 2011, 2015; Moore et al., 2014).
Total lung capacity (TLC) included the volume of the dead space (trachea and bronchi, VD), and the maximum alveolar volume (VA), i.e., TLC = VD + VA. It was assumed that gas exchange only occurred in the alveoli and when the diving alveolar volume (DVA) was equal to 0, gas exchange stopped. We used the equation initially developed by Kooyman (1973), to estimate TLC (TLCest = 0.135 , where Mb is body mass in kg), and later validated for the dolphin (Fahlman et al., 2011, 2015). Dead space volume was assumed to be 7% of TLC (Kooyman, 1973; Fahlman et al., 2011). The relationship between pulmonary shunt and DVA was determined using a power function (Bostrom et al., 2008) for data from the harbor seals (Equation 6A in Fahlman et al., 2009).
All pressures were corrected for water vapor pressure, assuming that the respiratory system was fully saturated at 37°C.
Blood and Tissue Gas Stores
As in previous studies, the blood and tissue stores of N2, CO2, and O2 were determined by the solubility coefficients for each gas, as previously detailed (Fahlman et al., 2006). For O2 and CO2, the average concentration of hemoglobin for each population was used to estimate the blood O2 stores and CO2 storage capacity (Table 2). Tissue gas content was determined as previously detailed (Fahlman et al., 2006, 2009).
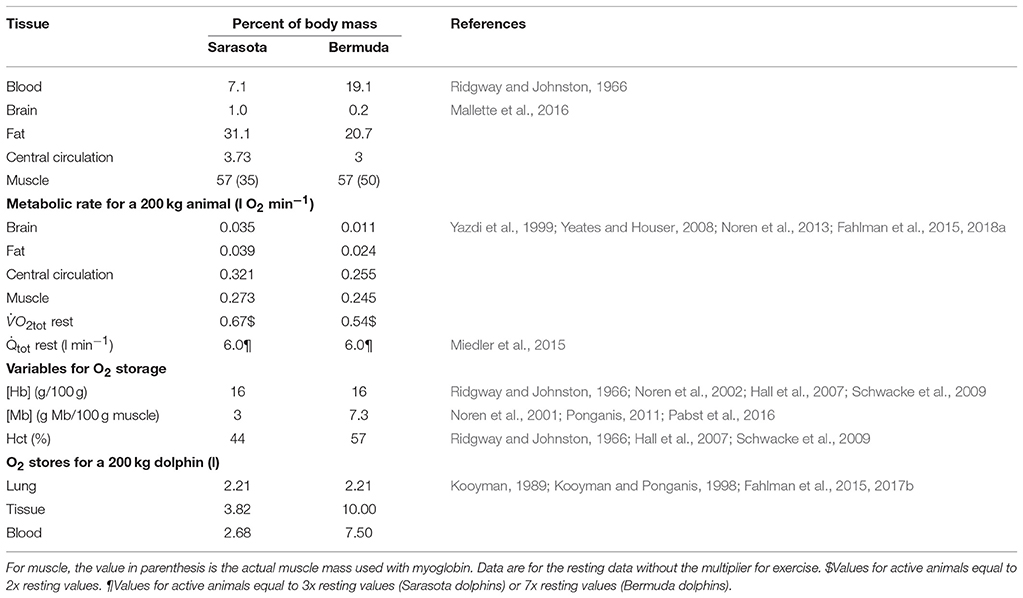
Table 2. Body compartment composition (% of body mass), estimated compartment metabolic rate (rest), and the value used in the model, cardiac output at rest/diving and at the surface, male adult dolphins.
Compartment Size, Cardiac Output, and Blood Flow Distribution
For the coastal ecotype sampled in Sarasota Bay, the relative size of each compartment was taken from previous studies in coastal bottlenose dolphins (Table 2; Ridgway and Johnston, 1966; Mallette et al., 2016). For blood and muscle we used species-specific values for blood hematocrit (Ridgway and Johnston, 1966; Hall et al., 2007; Schwacke et al., 2009; Fahlman et al., 2018b), and myoglobin concentration (Noren et al., 2001; Ponganis, 2011). For the deep-diving offshore dolphins, we initially assumed that the animals were similar to the coastal ecotype from Sarasota Bay. However, these assumptions resulted in a cADL that was too short for all Bermuda dolphins. We therefore adjusted the body composition of the Bermuda dolphins, assuming that their body composition was similar to that of deep diving beaked whales (Pabst et al., 2016), and used the body composition for Mesoplodon from our previous studies (Table 2; Hooker et al., 2009).
For the current study, we assumed that the cardiac output () at the surface was 3 times higher than the measured in resting bottlenose dolphins (31.5 ml min−1 kg−1, Miedler et al., 2015). To account for differences in Mb, mass specific (s) was adjusted using Equation (5) in Fahlman et al. (2009). During diving, the was reduced to 1/3 of the value at the surface to account for the dive response, i.e., the diving was the same as that measured in resting animals. Blood flow distributions to each tissue at the surface and during diving were iteratively tested to maximize utilization of O2 to increase the aerobic dive duration (Table 2).
Dive Data Used
We used dive data collected from high resolution digital audio and movement recording tags (DTAG, Johnson and Tyack, 2003) attached to the dorsal side of each dolphin by means of four small suction cups, and programmed to release after <24 h. DTAGs were deployed during 2013–2016 in Sarasota Bay and in August of 2016 off the coast of Bermuda, during capture-release operations (e.g., Wells et al., 2004; Klatsky et al., 2007). The dolphins were tagged and data collected under permits issued by NMFS (Scientific Research Permit Number 15543) and the Bermuda Government, Department of Environment and Natural Resources (Research permit number SP160401r).
Data processing was done using the DTAG toolbox (soundtags.st-andrews.ac.uk). Tag depth was sampled at 200 Hz and subsequently down-sampled to 25 Hz during post-processing using a linear phase 10 Hz low-pass FIR filter. Surfacings were used to estimate the zero-pressure offset and to characterize and remove the effect of temperature on estimated depth.
A dive was defined as a submergence deeper than 1.5 m (1.15 ATA) and longer than 10 s. The start and end of each dive was calculated as the first and last point of the dive that exceeded 0.1 m depth, and the surface interval was defined as the time from current dive to previous dive.
Results
Respiratory Compliance
The updated parameters that defined the structural properties (compliance) of the respiratory system resulted in a stiffer upper airway and more compliant alveolar space as compared with the values used in previous studies (Figure 1A), which affected the pulmonary shunt (Figure 1B). The parameters for respiratory compliance were updated and the model output compared with the results from the previous model (Figures 1C,D). The end-dive gas tension increased with both dive duration and maximum dive depth for N2 and O2, while for CO2 there was a slight decrease (Figures 1C,D).
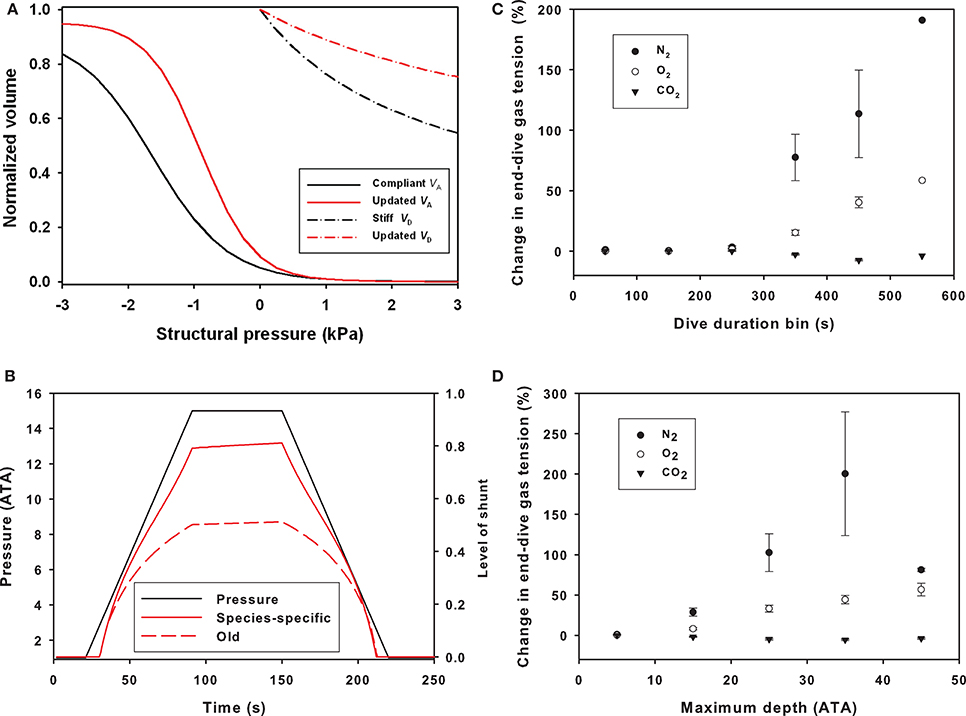
Figure 1. (A) Normalized volume (VA, alveolar volume; VD, dead space/tracheal volume) vs. structural pressure for alveolar and dead space compliances based on the estimate from Bostrom et al. (2008), or updated estimates from bottlenose dolphins (Fahlman et al., 2011, 2015). (B) Differences in pulmonary shunt with old and updated compliance values for the respiratory system in dolphins during a representative dive to 150 m for an animal with a body composition like the Bermuda dolphin (Fahlman et al., 2009). The average compliant alveoli and medium compliant trachea were used for the base model, and this model was used as a basis of comparison with all other simulations. Changes in end-dive mixed venous N2, O2, and CO2 levels against (C) dive duration (sec) or (D) maximum dive depth (ATA) when comparing old and revised lung compliance values. The y-axis is the change in percent for [(old-new)/old * 100]. These changes reflect how the structural properties alter the shunt and ventilation-perfusion mismatch (Garcia Párraga et al., 2018).
Dive Data
There were no significant differences in body mass (Mb, P > 0.1, Welch t-value: 1.8, df = 2), body length (P > 0.9, t-value: 0.02, df = 5), average number of dives per hour (P < 0.1, Welch t-value: 0.3, df = 5), and average surface interval (P > 0.1, Welch t-value: 1.3, df = 2, Table 3) between ecotypes. There were significant differences in the dive behavior between the two populations, with dive duration (P < 0.01, Welch t-value: 4.37, df = 2), maximum dive depth (P < 0.05, Welch t-value: 3.95, df = 2), and mean depth per dive (P < 0.05, Welch t-value: 3.57, df = 2) being significantly higher in the Bermuda dolphins (Table 3, Figures 2, 3). For dives < 60 s, the Sarasota dolphins never exceeded a dive depth of 5 m (Figure 3A). As the dive duration increased >60 s, so did the maximum depth and also the variation (Figure 3A). For the Bermuda dolphins, there was a significant increase in the dive duration as the dive depth increased (Figure 3B). Dives < 60 s showed little variation and few exceeded 10 m (Figure 3B). As the dive duration increased beyond 100 s, the variation in maximum dive depth increased and dives exceeding 100 m became more common (Figure 3B). We found no indication that the surface interval increased with dive duration of the previous (X2 = 0.27, df = 1, P > 0.6) or next dive X2 = 0.05, df = 1, P > 0.8).
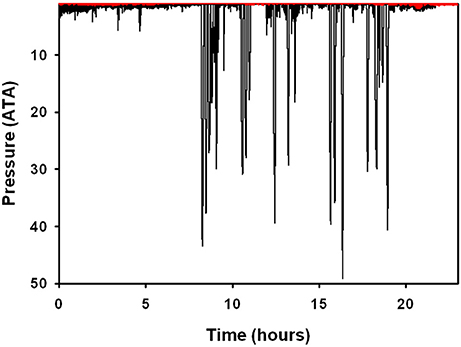
Figure 2. Representative dive data from two bottlenose dolphins in Sarasota Bay, Florida (red line), and Bermuda (black line). Data are plotted on the same axes range to show the differences in diving capacity/behavior. Pressure in ATA where 1 ATA is at the surface and 2 ATA is at 10 m depth.
Cardiac Output and Blood Flow Distribution
For both populations, we assumed that the diving was equal to resting values measured in the bottlenose dolphins (Table 4; Miedler et al., 2015). The blood flow distribution for the Sarasota dolphins was able to vary greatly, due to their shorter and shallower dive pattern. For the Bermuda dolphins, deviation from a certain blood flow distribution caused tissues to run out of O2. The specific variation varied slightly between individual animals, but one distribution pattern that focused perfusion to the central circulation, and minimized flow to the muscle, allowed all dolphins to complete their dives aerobically (Table 4).
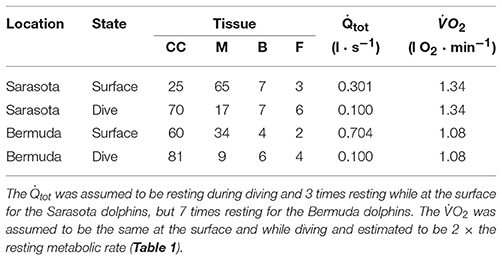
Table 4. Blood flow distribution (% of total cardiac output), cardiac output (), rate of O2 consumption, for central circulation (CC), muscle (M), brain (B), and fat (F) body compartments for a 200 kg dolphin for animals in Sarasota or Bermuda.
The blood flow required at the surface to assure that tissues received enough blood to restore O2 stores at the surface differed between the two ecotypes. For the Sarasota dolphins, the minimum at the surface was 3 times higher than during diving. A surface at least 7 times higher than during diving was required for the Bermuda population to ensure that tissues were saturated with O2; any lower surface would result in tissue PO2 continuously decreasing with each dive. In addition, for the Bermuda dolphins, changes in perfusion associated with diving, i.e., the dive response, was set to occur only for dives deeper than 20 m. Consequently, the elevated was required at the surface to help restore blood and tissue O2 stores.
Blood and Tissue Gas Tensions
In the Sarasota dolphins, there was considerable variation in end-dive blood and tissue PO2 with dive duration. In Figure 4, the muscle is used as a representative tissue to show these variations. In Figure 4A, the large variation in muscle PO2 is shown when the muscle tension is plotted against dive duration. There was a consistent exponential decrease with dive depth (Figure 4B). A decrease in the blood and tissue PO2 was obvious in the Bermuda dolphins for both dive duration (Figure 4C) and depth (Figure 4D). For the Bermuda dolphins, the decrease in tissue PO2 varied depending on the level of pulmonary shunt, and the shunt decreased the end dive PO2 for dives of the same duration or maximum depth (Figures 4C,D). The effect of the shunt on end-dive PO2 was most obvious for the muscle compartment, but was also seen in the other tissues (data not shown).
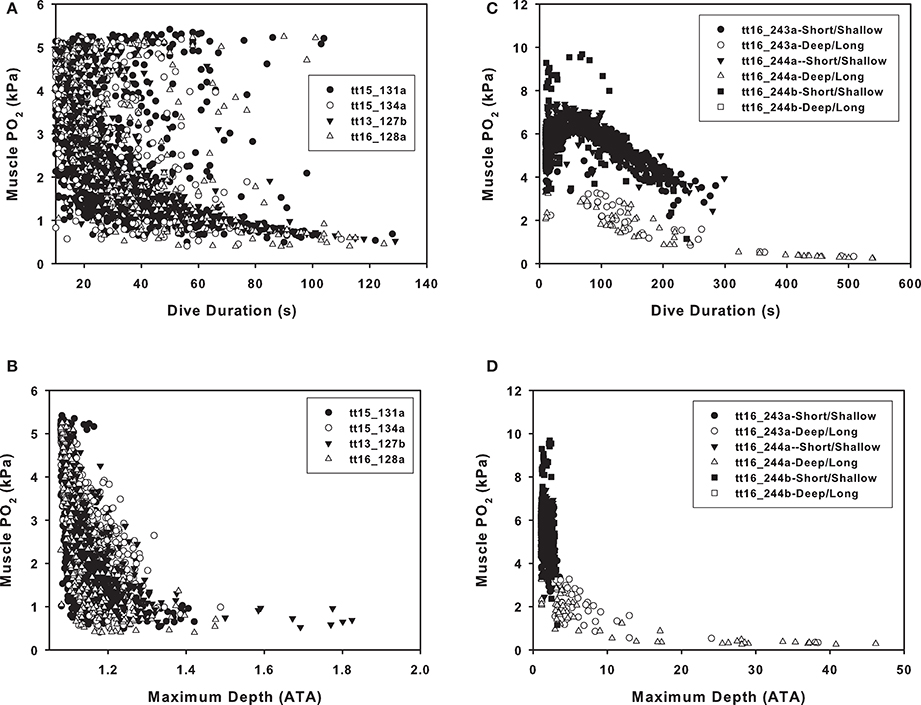
Figure 4. Estimated end-dive muscle PO2 (kPa) vs. (A,C) dive duration or (B,D) maximum dive depth (ATA, 1 ATA = 98.07 kPa) in (A,B) Sarasota or (B,D) Bermuda dolphins.
The estimated blood and tissue PO2 for a representative dive for dolphins from Sarasota (Figure 5A) or Bermuda (Figure 5B) showed similar patterns of a decrease in PO2 during a dive. However, the longer and deeper dive duration resulted in greater changes in blood and tissue PO2 values in the offshore ecotype. Figures 5C,D show the effect of depth on lung volume and pulmonary shunt.
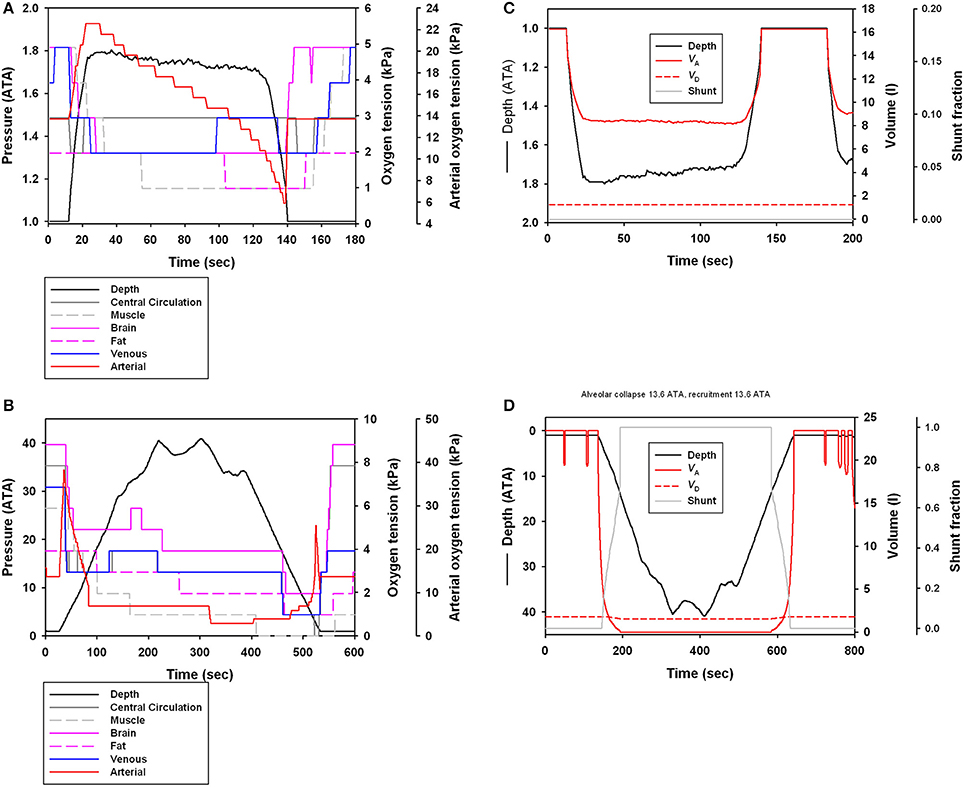
Figure 5. (A,B) Estimated central circulation, muscle, brain, fat, arterial, and venous PO2 (kPa) or (C,D) estimated shunt fraction, alveolar and tracheal volume for a long duration dive (depth in ATA, 1 ATA = 98.07 kPa) in (A,C) Sarasota and (B,D) Bermuda dolphin.
Discussion
In the current study, we modeled tissue and blood PO2, PCO2, and PN2 from fine-scale empirical dive data from bottlenose dolphins of both the coastal and offshore ecotypes to assess potential morphological or physiological adaptations that could help explain the large variation in dive behavior in these divergent populations. The results shows that the structural properties of the respiratory system have a significant effect on pulmonary gas exchange, and these changes are different for gases with different gas solubilities, agreeing with past work suggesting that variation in ventilation and perfusion may be important for managing gases during diving (West, 1962; Farhi and Yokoyama, 1967; Hodanbosi et al., 2016; Garcia Párraga et al., 2018). Furthermore, the results suggest that the deeper and longer dives of the offshore dolphins most likely reflect a greater O2 storage capacity, potentially combined with foraging of lower energetic cost. Future tagging studies to assess the energetic requirements of the different foraging strategies will be crucial to assess how close to their physiological limits each of these populations are living.
Theoretical work has suggested that the level of gas exchange, and blood flow distribution are important to alter blood and tissue gas levels (Fahlman et al., 2006, 2009). Previously, we suggested that the structural properties of the respiratory system could have a significant effect on the level of gas exchange during breath-hold diving (Bostrom et al., 2008; Fahlman et al., 2009), and how man-made disturbances may alter the risk of gas emboli through changes in the dive profile or physiology (Hooker et al., 2009; Kvadsheim et al., 2012). However, species-specific estimates for the structural properties of the respiratory system were not available and published values from a range of species were used (Bostrom et al., 2008; Fahlman et al., 2009, 2014; Hooker et al., 2009; Kvadsheim et al., 2012), but recent work has suggested that species-specific estimates may significantly alter the model estimates (Hodanbosi et al., 2016). We therefore used recently published data for respiratory compliance (Fahlman et al., 2011, 2015, 2018b), (Miedler et al., 2015), and metabolic rate (Fahlman et al., 2015, 2018a) for coastal and offshore bottlenose dolphin to update the model parameters. These changes altered the compression of the alveolar space, which affects the pressure dependence of the pulmonary shunt (Figure 1).
The updated parameters (Table 1), with stiffer airways and more compliant alveolar space, increased the level of shunt and reduced gas exchange throughout dives (Figures 1A,B). This significantly reduced N2 exchange during deeper dives as the alveolar space began compressing (Figures 1C,D). The updated parameters also affected O2 exchange, but the effect was less apparent. For CO2 there was little effect, and for longer and deeper dives, CO2 exchange was improved. This implies that variation in gas exchange from changes in the alveolar ventilation () and relationship (/) differs for gases with varying gas solubility (West, 1962; Farhi and Yokoyama, 1967). These results agree with theoretical modeling in California sea lions that showed that changes in the structural properties of the respiratory system have a significant effect on the exchange of O2, CO2, and N2 that differs between gas species (Hodanbosi et al., 2016). Together these studies provide additional support for a recent hypothesis suggesting that the lung architecture and varying / would enable marine mammals to manipulate which gases are exchanged during diving (Garcia Párraga et al., 2018). While the current model does not include the proposed mechanisms that would alter the / relationship, e.g., the effect of heterogeneous pulmonary blood flow and alveolar compression (collateral ventilation), it shows that varying the structural properties, which effectively reduces the /, the less soluble (N2) is significantly more affected as compared with the more soluble gases (O2 and CO2) (West, 1962; Farhi and Yokoyama, 1967). Thus, increasing upper airway stiffness and making the alveolar space more compliant causes the compression to occur at shallower depth, as originally hypothesized by Scholander (1940). This increases the level of shunt and reduces diffusion/ventilation, which has the greatest effect on gas with low solubility (West, 1962; Farhi and Yokoyama, 1967). In summary, we propose that the data in the current study agree with the suggestion that varying the / ratio may be an efficient way for deep divers to minimize N2 while still accessing pulmonary O2 and CO2 (Garcia Párraga et al., 2018).
The dive behaviors for the two dolphin forms were strikingly different, and demonstrate the large physiological plasticity in cetacean ecotypes. While these differences in dive behavior are undoubtedly related to differences in morphology, physiology and environment, the data presented here provide an interesting comparison of a species' capacity to vary physiological traits. Initially we used the available information for coastal dolphins to model tissue and blood gas dynamics in both populations (Table 2). For a 200 kg dolphin, the estimated O2 stores were 8.7 l (43.6 ml O2 kg−1), resulting in a calculated aerobic dive limit (cADL) of 6.5 min when assuming a field metabolic rate that is twice that of resting. This cADL is considerably greater than the observed maximum dive durations in the Sarasota dolphins ranging from 2.2 min in the current study (Table 3), or up to 4.5 min in previous work (R. Wells, unpublished observation, Wells et al., 2013). The diet of bottlenose dolphins in Sarasota is composed exclusively of fish (>15 different species found in stomach content of 16 stranded animals; Barros and Wells, 1998). Thus, their activity may require a variety of high energy activities from rapid body turns (pinwheel feeding) to tail slaps (fish whacking, kerpluncking) associated with prey capture (Nowacek, 2002), which would increase the daily metabolic rate and reduce the cADL. For a cADL of 2.2–4.5 min, the Sarasota ecotype would have a metabolic scope around 3-6 times their resting metabolic rate (3 times the estimated field metabolic rate in Table 3). The metabolic scope is the maximal aerobic metabolic rate divided by the basal metabolic rate and is typically in the range of 3–10; a scope >7 cannot be sustained over long periods (Peterson et al., 1990). Thus, a field metabolic rate that is 6 times higher than the resting value implies that this ecotype would be working at or close to the maximal aerobic capacity for a cADL of 2.2 min. However, another likely explanation is that the coastal dolphins here operate in an environment where they are seldom limited by their dive physiology. The bottlenose dolphins from which dive data were obtained primarily occupy shallow waters in and around Sarasota and Palma Sola bays, with water depths generally <10 m, and often less than a few meters. Thus, our results suggest that they can significantly increase their dive duration if necessary but that they may not need this to exploit prey in their shallow-water habitat.
The Bermuda dolphins, on the other hand, had significantly longer dives than the Sarasota animals. A considerable number of dives exceeded the cADL, even when calculated from twice the resting metabolic rate, and the dolphin ran out of O2 during long dives. For this reason, we hypothesized that the offshore dolphins must have increased O2 stores to help increase their dive duration. We are not aware of any published body composition data for offshore delphinids, and to increase the O2 stores we therefore modeled the body compartments according to the proposed body composition for deep-diving beaked whales, with increased blood and muscle O2 storage (Peterson et al., 1990) that significantly increased the total O2 stores (98.6 ml O2 kg−1), and the cADL (18.2 min, Table 2). With a maximal dive duration of 9 min, this provided a considerable scope for the Bermuda dolphins. The O2 storage capacity of the sperm whale (81 ml O2 kg−1), hooded seal (90 ml O2 kg−1), elephant seal (94 ml O2 kg−1), and Weddell seal (89 ml O2 kg-1, Ponganis, 2015) are lower than our estimated value. The calculations made in the current study are based on assumptions about the blood volume, and muscle mass of these animals, and it is likely that the O2 storage capacity is lower and the cADL shorter. If we assume that the longest dive represent the upper limit of the cADL, we would need an O2 storage capacity of approximately 49 ml O2 kg−1). Thus, we can predict that the O2 storage capacity is somewhere between these two values for the offshore ecotype.
In addition to a greater O2 storage capacity, Bermuda dolphins may also have a greater capacity to alter diving metabolic rate. In a previous study, we estimated the field metabolic rate of coastal ecotype bottlenose dolphins to be around 11.7–23.4 ml O2 min−1 kg−1. As there were no differences in the resting metabolic rate of the coastal (Fahlman et al., 2018a) or offshore populations (Fahlman et al., 2018b), this field metabolic rate for offshore dolphins resulted in a cADL of 4.2–8.4 min, which is closer to the maximum dive duration seen for these animals. Studies have indicated that the metabolic cost during longer and deeper dives is similar to or lower than the resting metabolic rate at the surface (Hurley and Costa, 2001; Fahlman et al., 2013), and deeper dives may provide cost savings as the animals may be able to glide during long portions of the dive (Hurley and Costa, 2001; Williams, 2001; Fahlman et al., 2013). Thus, offshore dolphins may also have reduced cost of foraging that increases their cADL. Assessing field metabolic rate from these two populations using validated metabolic proxies, such as activity (acceleration, Fahlman et al., 2008b, 2013), or heart rate (McPhee et al., 2003), could be used to determine how energy is partitioned in this population and resolve some of these questions. In fact, the DTAG data provide such an opportunity and is an objective for future studies.
The dive data from the Bermuda dolphins are suggestive of 2 different types of dives; one shallow (10–70 m, Figure 3B) that changes little in depth with duration and another dive type starting at around 100 m depth with a steep increase in dive duration with depth (Figure 3B). It is not surprising that deeper dives are generally longer, as the transit to depth is a significant portion of the dive duration. During the deeper dives, the pulmonary shunt alters the blood and tissue gas content, as shown for the end-dive muscle PO2 in Figure 4C. Without access to pulmonary PO2, the blood O2 was reduced and more O2 used from the muscle, pushing down end-dive PO2. This was not seen in the shallow diving Sarasota dolphins (Figure 4A), which never dove to depths where the pulmonary shunt began to alter gas tensions.
The differences in dive behavior had a significant effect on the gas tension in the blood and tissues (Figure 5). For both populations there was a continuous decrease in blood gases during the dive but the decrease was more extreme for the Bermuda dolphins (Figure 5B), and matching the blood flow to various tissues was more restrictive. This agrees with the suggestion made in previous modeling work that an important aspect of the dive response is to distribute the available perfusion to central tissues like the heart and brain, while blood flow to the muscle should be minimal (Davis and Kanatous, 1999). This allows muscle metabolism to be fueled by endogenous O2 and assures that the matching of utilization of endogenous and vascular O2 increases the duration of the dive that is fueled by aerobic metabolism (Davis and Kanatous, 1999).
For both ecotypes, the while diving was assumed equal to the resting value measured in bottlenose dolphins (Miedler et al., 2015). The surface in the Sarasota ecotype was set to 3 times higher than resting, which sufficiently restored O2 and removed CO2 from the blood and tissues to avoid continuous changes with repeated dives. In the Bermuda animals, on the other hand, a surface of 3 times resting was not sufficient to restore the O2 or remove the CO2 during the longer dives. Initially, was increased to 7 times higher than during diving, but while this improved restoration of blood and tissue O2 and CO2 levels, there were still continuous changes with repeated dives. By keeping the elevated for submersions <20 m, however, we were able to sufficiently restore O2 and remove enough CO2 to prevent accumulating changes across repeated dives. These results suggest that needs to be maintained during intervening short and shallow dives to allow restoration of normal blood and tissue gas tensions. Staying submerged during this time may help increase the PO2 and thereby the uptake of O2. The suggestion that there is little or no cardiovascular modification during shallow dives may be controversial, as most studies have reported changes in heart rate during diving. For example, in the bottlenose dolphin resting at the surface or while submerged the average heart rate were 105 and 40 beats min−1, respectively (Noren et al., 2012). However, the past study provides an estimated surface resting heart rate that is influenced by the respiratory sinus arrhythmia (RSA), which will significantly elevate the resting values. In a more recent paper the surface resting heart rate when accounting for the RSA ranged from 27 to 54 beats min−1 (Miedler et al., 2015), thus not very different from the resting diving heart rate reported by Noren et al. (2012). In addition, in the past study it was also reported that the diving heart rate increased with underwater activity by between 40 and 79% (Noren et al., 2012). While our estimated at depths shallower than 20 m may be overestimated, there is experimental evidence that the perfusion is modulated while submerged and future studies could look at how this changes during short and shallow dives.
In the Sarasota dolphins, the dive depth caused a reduction in alveolar volume, but the low pressure did not cause tracheal compression or induce a pulmonary shunt (Figure 5C). In the Bermuda dolphins, the arterial PO2 increased during the descent to a maximum value, and then rapidly declined until the alveoli were reinflated during the ascent at which a second peak was observed (Figure 5B). We previously suggested that this pattern of arterial gas tension would support Scholander's hypothesis of pressure-induced hyperoxia as the lungs are compressed, followed by an increasing shunt as the alveoli are compressed until atelectasis (alveolar collapse), in this case at 126 m (Figure 5D), when the arterial side reflects the mixed venous (Fahlman et al., 2009; McDonald and Ponganis, 2012). This is supported by empirically measured arterial and venous gas tensions in both seals (Falke et al., 1985) and sea lions (McDonald and Ponganis, 2012). As the dolphin ascends, the alveoli are recruited at a depth of 126 m and gas exchange commences again. At this point, there is a second peak as pulmonary O2 again saturates the blood (Figures 5C,D). The second peak for arterial PO2 is lower as compared with the peak right before atelectasis, despite equivalent pulmonary PO2. During the dive, the O2 in the blood is continuously consumed, causing both the arterial and venous PO2 to decrease. As the alveoli are recruited at depth, the pulmonary PO2 increases. The high pulmonary and low venous PO2 result in an elevated partial pressure gradient that favors diffusion and gas exchange. Thus, pulmonary O2 diffuses into the pulmonary capillary and helps saturate the arterial blood. In addition, CO2 is removed from the blood into the lung. Both these processes help prepare the dolphin to minimize the duration of the surface interval as CO2 is being removed and O2 being taken up before reaching the surface. Elevated gas exchange at depth also increases N2 exchange, increasing the tissue and blood tension and the risk for gas emboli. However, the elevated pulmonary pressure as the alveoli open results in an elevated / ratio, favoring O2 and CO2 exchange, while limiting N2 exchange (Garcia Párraga et al., 2018). In addition, it has been hypothesized that marine mammals are able to separate the lung into two regions; one region that is ventilated and another atelactic area, with the latter being perfused (Garcia Párraga et al., 2018). This matching of pulmonary blood flow to hypoxic/atelactic regions result in selective gas exchange (Olson et al., 2010; Garcia Párraga et al., 2018), which during normal dives would help reduce the risk for gas emboli, and help prepare the dolphin to restore the O2 used and removing the CO2 produced (West, 1962; Farhi and Yokoyama, 1967; Olson et al., 2010). There is also evidence for an arterio-venous shunt that alters the blood gas tensions and could help minimize inert gas uptake during the ascent and help arterialize venous blood (Garcia Párraga et al., 2018).
In a number of marine mammals, there is an anticipatory pre-surfacing tachycardia, which likely increases (Fedak et al., 1988; Thompson and Fedak, 1993; Andrews et al., 1997; Noren et al., 2012; Williams et al., 2017). During natural undisturbed dives, it seems that the change in heart rate begins during the initial stages of the ascent, but a large increase occurs during the later stages of the ascent close to the surface. Close to the surface, the blood and tissue tension would be supersaturated with N2 and the gas would move from the tissues to the lungs (Fahlman et al., 2009). Elevated at this stage of the dive would result in a decrease in the / ratio, which would enhance exchange and removal of N2. It has been hypothesized that disturbance of normal diving homeostasis, such as exposure to man-made sound, capture in nets, or any stressful situation may alter the physiology and result in conditions that enhance N2 uptake and the risk for gas emboli (Fernandez et al., 2005; Hooker et al., 2009; Fahlman et al., 2014; García-Párraga et al., 2014). In turtles, it has been suggested that the stress associated with incidental capture in fishing gear result in a sympathetic response, which opens the arterial pulmonary sphincter, increases pulmonary blood flow, decreases the/ ratio and increase N2 uptake and risk of gas emboli (García-Párraga et al., 2014, 2017; Fahlman et al., 2017a). In cetaceans, active management of the pulmonary perfusion has been hypothesized as a mechanism that allow the cetaceans to vary the level of the / match in the lung, and develop a shunt even at shallow depths that does not depend on hydrostatic compression (Garcia Párraga et al., 2018). Similar to the turtles, stress in cetaceans may also alter perfusion and the/ relationship causing increased pulmonary blood flow, increased uptake of N2 and risk of gas emboli (Fahlman et al., 2006; Hooker et al., 2009). Theoretical modeling has indicated that stress related changes in may increase the risk for gas emboli, and empirical data in the narwhal indicate that the large changes in the diving heart rate occurs deeper under stressful situations (around 170 m) as compared with natural dives (Fahlman et al., 2009, 2014; Hooker et al., 2009; Williams et al., 2017). Thus, this reversal of the diving bradycardia may result in elevated pulmonary blood flow, changes in blood flow distribution within the lung and changes in /, resulting in elevated N2 uptake and risk for gas emboli (Garcia Párraga et al., 2018).
In summary, in the current study we show that the dive behavior in deep diving bottlenose dolphins likely requires morphological differences resulting in a greater O2 storage capacity as compared with the coastal ecotype. It is also possible that the foraging behavior of the offshore population, with longer periods of gliding, results in lower foraging metabolic costs. Our results also indicate the importance of species-specific data when predicting physiological responses of animals. By updating the compliance estimates for the respiratory system, we show additional evidence of how variation in the ventilation/perfusion relationship is able to alter exchange of gases. This result provides additional support for our hypothesis of how marine mammals manage gases during diving and how stress may alter physiology and cause increased N2 uptake and risk of gas emboli from forming.
Data Availability
The theoretical model and data used in this paper are made freely available at: osf.io/eyxpa.
Author Contributions
AF helped collect the dive data, performed the modeling work, data analysis, wrote the first draft of the paper. FHJ collected and extracted the dive data, and helped edit the paper. PT provided the tools to collect the dive data, and helped edit the paper. RW helped collect the dive data and edited the paper.
Funding
AF (N00014-17-1-2756), PT (N000141512553) and FHJ (N00014-14-1-0410) were supported by the Office of Naval Research, and FHJ by an AIASCOFUND fellowship from Aarhus Institute of Advanced Studies, Aarhus University, under EU's FP7 program (Agreement No. 609033). PT received funding from the MASTS pooling initiative (The Marine Alliance for Science and Technology for Scotland) and their support is gratefully acknowledged. MASTS is funded by the Scottish Funding Council (grant reference HR09011) and contributing institutions. Funding for the Sarasota Bay and Bermuda field-work was provided by Dolphin Quest, Inc., and Office of Naval Research (N00014-14-1-0563).
Conflict of Interest Statement
The authors declare that the research was conducted in the absence of any commercial or financial relationships that could be construed as a potential conflict of interest.
Acknowledgments
A special thanks to the many volunteers and staff at Dolphin Quest and the Sarasota Dolphin Research Project who made collection of the dive data possible. A special thanks to Nigel Pollard, the Bermuda Government, the Bermuda Aquarium, Museum and Zoo (BAMZ), and NOAA.
References
Andrews, R. D., Jones, D. R., Williams, J. D., Thorson, P. H., Oliver, G. W., Costa, D. P., et al. (1997). Heart rates of northern Elephant seals diving at sea and resting on the beach. J. Exp. Biol. 200, 2083–2095.
Barros, N. B., and Wells, R. S. (1998). Prey and feeding patterns of resident bottlenose dolphins (Tursiops truncatus) in Sarasota Bay, Florida. J. Mam. 79, 1045–1059. doi: 10.2307/1383114
Bostrom, B. L., Fahlman, A., and Jones, D. R. (2008). Tracheal compression delays alveolar collapse during deep diving in marine mammals. Resp. Physiol. Neurobiol. 161, 298–305. doi: 10.1016/j.resp.2008.03.003
Boyd, I. L., Woakes, A. J., Butler, P. J., Davis, R. W., and Williams, T. M. (1995). Validation of heart rate and doubly labelled water as measures of metabolic rate during swimming in California sea lions. Func. Ecol. 9, 151–160. doi: 10.2307/2390559
Butler, P. J. (2006). Aerobic dive limit. What is it and is it always used appropriately? Comp. Biochem. Physiol. A. 145, 1–6. doi: 10.1016/j.cbpa.2006.06.006
Butler, P. J., Green, J. A., Boyd, I. L., and Speakman, J. R. (2004). Measuring metabolic rate in the field: the pros and cons of the doubly labelled water and heart rate methods. Func. Ecol. 18, 168–183. doi: 10.1111/j.0269-8463.2004.00821.x
Butler, P. J., and Jones, D. R. (1997). Physiology of diving birds and mammals. Physiol. Rev. 77, 837–899. doi: 10.1152/physrev.1997.77.3.837
Carbone, C., and Houston, A. I. (1996). The optimal allocation of time over the dive cycle: an approach based on aerobic and anaerobic respiration. Anim. Behav. 51, 1247–1255. doi: 10.1006/anbe.1996.0129
Castellini, M. A., Kooyman, G. L., and Ponganis, P. J. (1992). Metabolic rates of freely diving Weddell seals: correlations with oxygen stores, swim velocity and diving duration. J. Exp. Biol. 165, 181–194.
Cornick, L. A., and Horning, M. (2003). A test of hypotheses based on optimal foraging considerations for a diving mammal using a novel experimental approach. Can. J. Zool. 81, 1799–1807. doi: 10.1139/z03-179
Costa, D. P., Gales, N. J., and Goebel, M. E. (2001). Aerobic dive limit: how often does it occur in nature? Comp. Biochem. Physiol. A. 129, 771–783. doi: 10.1016/S1095-6433(01)00346-4
Davis, R. W., and Kanatous, S. B. (1999). Convective oxygen transport and tissue oxygen consumption in Weddell seals during aerobic dives. J. Exp. Biol. 202, 1091–1113.
Fahlman, A., Brodsky, M., Wells, R., McHugh, K., Allen, J., Barleycorn, A., et al. (2018a). Field energetics and lung function in wild bottlenose dolphins, Tursiops truncatus, in Sarasota Bay Florida. Roy. Soc. Open. Sci. 5:171280. doi: 10.1098/rsos.171280
Fahlman, A., Crespo Picazo, J. L., Sterba-Boatwright, B., Stacy, B. A., and Garcia-Parraga, D. (2017a). Defining risk variables causing gas embolism in loggerhead sea turtles (Caretta caretta) caught in trawls and gillnets. Sci. Rep. 7:2739. doi: 10.1038/s41598-017-02819-5
Fahlman, A., Hooker, S. K., Olszowka, A., Bostrom, B. L., and Jones, D. R. (2009). Estimating the effect of lung collapse and pulmonary shunt on gas exchange during breath-hold diving: the Scholander and Kooyman legacy Resp. Physiol. Neurobiol. 165, 28–39. doi: 10.1016/j.resp.2008.09.013
Fahlman, A., Loring, S. H., Ferrigno, M., Moore, C., Early, G., Niemeyer, M., et al. (2011). Static inflation and deflation pressure-volume curves from excised lungs of marine mammals. J. Exp. Biol. 214, 3822–3828. doi: 10.1242/jeb.056366
Fahlman, A., Loring, S. H., Levine, G., Rocho-Levine, J., Austin, T., and Brodsky, M. (2015). Lung mechanics and pulmonary function testing in cetaceans. J. Exp. Biol. 218, 2030–2038. doi: 10.1242/jeb.119149
Fahlman, A., Moore, M. J., and Garcia-Parraga, D. (2017b). Respiratory function and mechanics in pinnipeds and cetaceans. J. Exp. Biol. 220, 1761–1763. doi: 10.1242/jeb.126870
Fahlman, A., Olszowka, A., Bostrom, B., and Jones, D. R. (2006). Deep diving mammals: dive behavior and circulatory adjustments contribute to bends avoidance. Respir. Physiol. Neurobiol. 153, 66–77. doi: 10.1016/j.resp.2005.09.014
Fahlman, A., Svärd, C. D., Rosen, A. S., Jones, D. R., and Trites, A. W. (2008a). Metabolic costs of foraging and the management of O2 and CO2 stores in Steller sea lions. J. Exp. Biol. 211, 3573–3580. doi: 10.1242/jeb.023655
Fahlman, A., Svärd, C. D., Rosen, A. S., Wilson, R. S., and Trites, A. W. (2013). Activity as a proxy to estimate metabolic rate and to partition the metabolic cost of diving vs. breathing in pre- and post-fasted Steller sea lions. Aquat. Biol. 18, 175–184. doi: 10.3354/ab00500
Fahlman, A., Tyack, P. L., Miller, P. J., and Kvadsheim, P. H. (2014). How man-made interference might cause gas bubble emboli in deep diving whales. Front. Physiol. 5:13. doi: 10.3389/fphys.2014.00013
Fahlman, A., McHugh, K., Allen, J., Barleycorn, A., Allen, A., Sweeney, J., et al. (2018b). Resting metabolic rate and lung function in wild offshore common bottlenose dolphins, tursiops truncatus, near bermuda. Front. Physiol. 9:886. doi: 10.3389/fphys.2018.00886
Fahlman, A., Wilson, R., Svärd, C. D, Rosen, A. S., and Trites, A. W. (2008b). Activity and diving metabolism correlate in Steller sea lion Eumetopias jubatus. Aquat. Biol. 2, 75–84. doi: 10.3354/ab00039
Falke, K. J., Hill, R. D., Qvist, J., Schneider, R. C., Guppy, M., Liggins, G. C., et al. (1985). Seal lung collapse during free diving: evidence from arterial nitrogen tensions. Science 229, 556–557. doi: 10.1126/science.4023700
Farhi, L. E. (1967). Elimination of inert gas by the lung. Respir. Physiol. 3, 1–11. doi: 10.1016/0034-5687(67)90018-7
Farhi, L. E., and Yokoyama, T. (1967). Effects of ventilation-perfusion inequality on elimination of inert gases. Resp. Physiol. 3, 12–20. doi: 10.1016/0034-5687(67)90019-9
Fedak, M. A., Pullen, M. R., and Kanwisher, J. (1988). Circulatory responses of seals to periodic breathing: heart rate and breathing during exercise and diving in the laboratory and open sea. Can. J. Zool. 66, 53–60. doi: 10.1139/z88-007
Fernandez, A., Edwards, J. F., Rodruiquez, F., Espinosa de los Monteros, A., Herraez, M. P., Castro, P., et al. (2005). “Gas and fat embolic syndrome” involving a mass stranding of beaked whales (Family Ziphiidae) exposed to anthropogenic sonar signals. Vet. Pathol. 42, 446–457. doi: 10.1354/vp.42-4-446
Froget, G., Butler, P. J., Handrich, Y., and Woakes, A. J. (2001). Heart rate as an indicator of oxygen consumption: influence of body condition in the king penguin. J. Exp. Biol. 204, 2133–2144.
García-Párraga, D., Crespo-Picazo, J. L., Bernaldo de Quirós, Y., Cervera, V., Martí-Bonmati, L., Díaz-Delgado, J., et al. (2014). Decompression Sickness (“the bends”) in Sea Turtles. Dis. Aquat. Org. 111, 191–205. doi: 10.3354/dao02790
Garcia Párraga, D., Moore, M., and Fahlman, A. (2018). Pulmonary ventilation– perfusion mismatch: a novel hypothesis for how diving vertebrates may avoid the bends. Proc. Roy. Soc. B. 285:20180482. doi: 10.1098/rspb.2018.0482
García-Párraga, D., Valente, A. L. S., Stacy, B. A., and Wyneken, J. (2017). “Cardiovascular system,” in Sea Turtle Health and Rehabilitation, eds C. A. Manire, T. M. Norton, B. A. Stacy, C. A. Harms, and C. J. Innis (J. Ross Publishing), 295–320.
Hall, A. J., Wells, R. S., Sweeney, J. C., Townsend, F. I., Balmer, B. C., Hohn, A. A., et al. (2007). Annual, seasonal and individual variation in hematology and clinical blood chemistry profiles in bottlenose dolphins (Tursiops truncatus) from Sarasota Bay, Florida. Comp. Biochem. Physiol. A. 148, 266–277. doi: 10.1016/j.cbpa.2007.04.017
Higgs, N. D., Little, C. T. S., and Glover, A. G. (2010). Bones as biofuel: a review of whale bone composition with implications for deep-sea biology and palaeoanthropology. Proc. Roy. Soc. B. 278, 9–17. doi: 10.1098/rspb.2010.1267
Hodanbosi, M., Sterba-Boatwright, B., and Fahlman, A. (2016). Updating a gas dynamics model using estimates for California sea lions (Zalophus californianus). Resp. Physiol. Neurobiol. 234, 1–8. doi: 10.1016/j.resp.2016.08.006
Hoelzel, A. R., Potter, C. W., and Best, P. B. (1998). Genetic differentiation between parapatric “nearshore” and “offshore” populations of the bottlenose dolphin. Proc. Roy. Soc. B. 265, 1177–1183. doi: 10.1098/rspb.1998.0416
Hooker, S. K., Baird, R. W., and Fahlman, A. (2009). Could beaked whales get the bends? Effect of diving behaviour and physiology on modelled gas exchange for three species: Ziphius cavirostris, Mesoplodon densirostris and Hyperoodon ampullatus. Resp. Physiol. Neurobiol. 167, 235–246. doi: 10.1016/j.resp.2009.04.023
Hückstädt, L. A., Tift, M. S., Riet-Sapriza, F., Franco-Trecu, V., Baylis, A. M. M., Orben, R. A., et al. (2016). Regional variability in diving physiology and behavior in a widely distributed air-breathing marine predator, the South American sea lion Otaria byronia. J. Exp. Biol. 219, 2320–2330. doi: 10.1242/jeb.138677
Hurley, J. A., and Costa, D. P. (2001). Standard metabolic rate at the surface and during trained submersions in adult California sea lions (Zalophus californianus). J. Exp. Biol. 204, 3273–3281.
Johnson, M., and Tyack, P. L. (2003). A digital acoustic recording tag for measuring the response of wild marine mammals to sound. IEEE J. Ocean. Eng. 28, 3–12. doi: 10.1109/JOE.2002.808212
Klatsky, L. J., Wells, R. S., and Sweeney, J. C. (2007). Offshore bottlenose dolphins (Tursiops truncatus): movement and dive behavior near the Bermuda pedestal. J. Mam. 88, 59–66. doi: 10.1644/05-MAMM-A-365R1.1
Kooyman, G. L. (1973). Respiratory adaptations in marine mammals. Am. Zool. 13, 457–468. doi: 10.1093/icb/13.2.457
Kooyman, G. L., Castellini, M. A., Davis, R. W., and Maue, R. A. (1983). Aerobic diving limits of immature Weddell seals. J. Comp. Physiol. 151, 171–174. doi: 10.1007/BF00689915
Kooyman, G. L., and Ponganis, P. J. (1998). The physiological basis of diving to depth: birds and mammals. Ann. Rev. Physiol. 60, 19–32. doi: 10.1146/annurev.physiol.60.1.19
Kvadsheim, P. H., Miller, P. J. O., Tyack, P. L., Sivle, L. L. D., Lam, F.-P. A., and Fahlman, A. (2012). Estimated tissue and blood N2 levels and risk of in vivo bubble formation in deep-, intermediate- and shallow diving toothed whales during exposure to naval sonar. Front. Physiol. 3:125. doi: 10.3389/fphys.2012.00125
Mallette, S. D., McLellan, W. A., Scharf, F. S., Koopman, H. N., Barco, S. G., Wells, R. S., et al. (2016). Ontogenetic allometry and body composition of the common bottlenose dolphin (Tursiops truncatus) from the U.S. mid-Atlantic. Mar. Mam. Sci. 32, 86–121. doi: 10.1111/mms.12253
Mate, B. R., Rossbach, K. A., Nieukirk, S. L., Wells, R. S., Blair Irvine, A., Scott, M. D., et al. (1995). Satellite-monitored movements and dive behavior of a bottlenose dolphins (Tursiops truncatus) in Tampa Bay, Florida. Mar. Mam. Sci. 11, 452–463. doi: 10.1111/j.1748-7692.1995.tb00669.x
McDonald, B. I., and Ponganis, P. J. (2012). Lung collapse in the diving sea lion: hold the nitrogen and save the oxygen. Biol. Lett. 8, 1047–1049. doi: 10.1098/rsbl.2012.0743
McPhee, J. M., Rosen, D. A. S., Andrews, R. D., and Trites, A. W. (2003). Predicting metabolic rate from heart rate in juvenile Steller sea lions Eumetopias jubatus. J. Exp. Biol. 206, 1941–1951. doi: 10.1242/jeb.00369
Mead, J. G., and Potter, C. W. (1995). Recognizing Two Populations off the Bottlenose Dolphin (Tursiops Truncatus) of the Atlantic Coast of North America- Morphologic and Ecologic Considerations. International Biological Research Institute Reports, 32–44.
Miedler, S., Fahlman, A., Valls Torres, M., Álvaro Álvarez, T., and Garcia-Parraga, D. (2015). Evaluating cardiac physiology through echocardiography in bottlenose dolphins: using stroke volume and cardiac output to estimate systolic left ventricular function during rest and following exercise. J. Exp. Biol. 218, 3604–3610. doi: 10.1242/jeb.131532
Moore, C., Moore, M. J., Trumble, S., Niemeyer, M., Lentell, B., McLellan, W., et al. (2014). A comparative analysis of marine mammal tracheas. J. Exp. Biol. 217, 1154–1166. doi: 10.1242/jeb.093146
Noren, D. P., Holt, M. M., Dunkin, R. C., and Williams, T. M. (2013). The metabolic cost of communicative sound production in bottlenose dolphins (Tursiops truncatus). J. Exp. Biol. 216, 1624–1629. doi: 10.1242/jeb.083212
Noren, S. R., Kendall, T., Cuccurullo, V., and Williams, T. M. (2012). The dive response redefined: underwater behavior influences cardiac variability in freely diving dolphins. J. Exp. Biol. 215, 2735–2741. doi: 10.1242/jeb.069583
Noren, S. R., Lacave, G., Wells, R. S., and Williams, T. M. (2002). The development of blood oxygen stores in bottlenose dolphins (Tursiops truncatus): implications for diving capacity. J. Zool. 258, 105–113. doi: 10.1017/S0952836902001243
Noren, S. R., Williams, T. M., Pabst, D. A., McLellan, W. A., and Dearolf, J. L. (2001). The development of diving in marine endotherms: preparing the skeletal muscles of dolphins, penguins, and seals for activity during submergence. J. Comp. Physiol. B. 171, 127–134. doi: 10.1007/s003600000161
Nowacek, D. P. (2002). Sequential foraging behaviour of bottlenose dolphins, Tursiops truncatus, in Sarasota Bay, FL. Behaviour 139, 1125–1145. doi: 10.1163/15685390260437290
Olson, K. R., Whitfield, N. L., Bearden, S. E., St Leger, J., Nilson, E., Gao, Y., et al. (2010). Hypoxic pulmonary vasodilation: a paradigm shift with a hydrogen sulfide mechanism. Am. J. Physiol. Regul. Integr. Comp. Physiol. 298, R51–R60. doi: 10.1152/ajpregu.00576.2009
Pabst, D. A., McLellan, W. A., and Rommel, S. A. (2016). How to build a deep diver: the extreme morphology of mesoplodonts. Int. Comp. Biol. 56, 1337–1348. doi: 10.1093/icb/icw126
Peterson, C. C., Nagy, K. A., and Diamond, J. (1990). Sustained metabolic scope. Proc. Natl. Acad. Sci. U.S.A. 87, 2324–2328. doi: 10.1073/pnas.87.6.2324
Ponganis, P. J. (2015). Diving Physiology of Marine Mammals and Seabirds. Cornwall: Cambridge University Press.
Reed, J. Z., Chambers, C., Fedak, M. A., and Butler, P. J. (1994). Gas exchange of captive freely diving grey seals (Halichoerus grypus). J. Exp. Biol. 191, 1–18.
Reed, J. Z., Chambers, C., Hunter, C. J., Lockyer, C., Kastelein, R., Fedak, M. A., et al. (2000). Gas exchange and heart rate in the harbour porpoise, Phocoena phocoena. J. Comp. Physiol. B. 170, 1–10. doi: 10.1007/s003600050001
Ridgway, S. H., and Johnston, D. G. (1966). Blood oxygen and ecology of porpoises of three genera. Science 151, 456–458. doi: 10.1126/science.151.3709.456
Scholander, P. F. (1940). Experimental investigations on the respiratory function in diving mammals and birds. Hvalrådets Skrifter 22, 1–131.
Schwacke, L. H., Hall, A. J., Townsend, F. I., Wells, R. S., Hansen, L. J., Hohn, A. A., et al. (2009). Hematologic and serum biochemical reference intervals for free-ranging common bottlenose dolphins (Tursiops truncatus) and variation in the distributions of clinicopathologic values related to geographic sampling site. Am. J. Vet. Res. 70, 973–985. doi: 10.2460/ajvr.70.8.973
Sparling, C. E., and Fedak, M. A. (2004). Metabolic rates of captive grey seals during voluntary diving. J. Exp. Biol. 207, 1615–1624. doi: 10.1242/jeb.00952
Thompson, D., and Fedak, M. A. (1993). Cardiac responses of grey seals during diving at sea. J. Exp. Biol. 174, 139–154.
Wells, R., McHugh, K., Douglas, D., Shippee, S., McCabe, E. B., Barros, N., et al. (2013). Evaluation of potential protective factors against metabolic syndrome in bottlenose dolphins: feeding and activity patterns of dolphins in Sarasota Bay, Florida. Front. Endo. 4:139. doi: 10.3389/fendo.2013.00139
Wells, R. S., Rhinehart, H. L., Hansen, L. J., Sweeney, J. C., Townsend, F. I., Stone, R., et al. (2004). Bottlenose dolphins as marine ecosystem sentinels: developing a health monitoring system. EcoHealth 1, 246–254. doi: 10.1007/s10393-004-0094-6
West, J. B. (1962). Regional differences in gas exchange in the lung of erect man. J. Appl. Physiol. 17, 893–898. doi: 10.1152/jappl.1962.17.6.893
Williams, T. M. (2001). Intermittent swimming by mammals: a strategy for increased energetic efficiency during diving. Am. Zool. 41, 166–176. doi: 10.1093/icb/41.2.166
Williams, T. M., Blackwell, S. B., Richter, B., Sinding, M.-H. S., and Heide-Jørgensen, M. P. (2017). Paradoxical escape responses by narwhals (Monodon monoceros). Science 358, 1328–1331. doi: 10.1126/science.aao2740
Yazdi, P., Kilian, A., and Culik, B. M. (1999). Energy expenditure of swimming bottlenose dolphins (Tursiops truncatus). Mar. Biol. 134, 601–607. doi: 10.1007/s002270050575
Keywords: diving physiology, modeling and simulations, gas exchange, marine mammals, decompression sickness, blood gases, hypoxia
Citation: Fahlman A, Jensen FH, Tyack PL and Wells RS (2018) Modeling Tissue and Blood Gas Kinetics in Coastal and Offshore Common Bottlenose Dolphins, Tursiops truncatus. Front. Physiol. 9:838. doi: 10.3389/fphys.2018.00838
Received: 16 April 2018; Accepted: 14 June 2018;
Published: 17 July 2018.
Edited by:
Jose Pablo Vazquez-Medina, University of California, Berkeley, United StatesReviewed by:
Luis Huckstadt, University of California, Santa Cruz, United StatesMichael Tift, University of California, San Diego, United States
Copyright © 2018 Fahlman, Jensen, Tyack and Wells. This is an open-access article distributed under the terms of the Creative Commons Attribution License (CC BY). The use, distribution or reproduction in other forums is permitted, provided the original author(s) and the copyright owner(s) are credited and that the original publication in this journal is cited, in accordance with accepted academic practice. No use, distribution or reproduction is permitted which does not comply with these terms.
*Correspondence: Andreas Fahlman, afahlman@whoi.edu
†Frants H. Jensen orcid.org/0000-0001-8776-3606