- 1Key Laboratory of Animal Physiology and Biochemistry, College of Veterinary Medicine, Nanjing Agricultural University, Nanjing, China
- 2Department of Veterinary Physiology and Biochemistry, Faculty of Animal Husbandry and Veterinary Sciences, Sindh Agriculture University, Tandojam, Pakistan
In our previous study, we demonstrated that butyrate induced ruminal epithelial growth through cyclin D1 upregulation. Here, we investigated the influence of butyrate on the expression of genes associated with cell cycle and apoptosis in rumen epithelium. Goats (n = 24) were given an intra ruminal infusion of sodium butyrate at 0.3 (group B, n = 12) or 0 (group A, n = 12) g/kg of body weight (BW) per day before morning feeding for 28 days and were slaughtered (4 goat/group) at 5,7 and 9 h after butyrate infusion. Rumen fluid was analyzed for short chain fatty acids (SCFAs) concentration. Ruminal tissues were analyzed for morpho-histrometry and the expressions of genes associated with cell cycle and apoptosis. The results revealed that the ruminal butyrate concentration increased (P < 0.05) in B compared to group A. Morphometric analysis showed increased (P < 0.05) papillae size associated with higher number of cell layers in epithelial strata in B compared to A. Butyrate-induced papillae enlargement was coupled with enhanced mRNA expression levels (P < 0.05) of cyclin D1, CDK2, CDK4, and CDK6 (G0/G1 phase regulators) at 5 h, cyclin E1 (G1/S phase regulator) at 7 h and cyclin A and CDK1 (S phase regulators) at 9 h post-infusion compared to A group. In addition, the mRNA expression levels of apoptotic genes, i.e., caspase 3, caspase 9 and Bax at 5 h post-infusion were upregulated (P < 0.05) in group B compared to group A. The present study demonstrated that butyrate improved ruminal epithelial growth through concurrent and time-dependent changes in the expressions of genes involved in cell proliferation and apoptosis. It seems that the rate of proliferation was higher than the apoptosis which was reflected in epithelial growth.
Introduction
Short chain fatty acids (SCFA) are generated in the rumen by microbial fermentation of dietary carbohydrates. Absorption of SCFA across the epithelium contributes 70–80% of the total energy requirement of the ruminants. Acetate, propionate and butyrate are the main SCFA in rumen fluid (Bergman, 1990; Nozière et al., 2000) which induces morpho-functional alteration in ruminal papillae (Gäbel et al., 1991a,b; Krehbiel et al., 1992; Nozière et al., 2000). Conversely, an increase of SCFA absorption has been linked to the surface area enlargement of ruminal papillae in ruminants fed high concentrate diets (Dirksen et al., 1984; Gäbel et al., 1991a,b). The latter could be ascribed to an increased abundance of gene transcripts related to epithelial proliferation (Penner et al., 2011). Butyrate is a four-carbon SCFA normally produced in the smallest ratio of 5–15% in rumen (Bergman, 1990; Nozière et al., 2000). It is preferred metabolic fuels for rumen epithelium and concerned with mucosal health maintenance (Hamer et al., 2008; Leonel and Alvarez-Leite, 2012). The inner wall of the rumen is covered with rumen papillae which increases the surface area for absorption of nutrients (Malhi et al., 2013). The morphological and functional changes in ruminal papillae can be largely ascribed to butyrate (Krehbiel et al., 1992; Shen et al., 2005). Previous studies indicated that sodium butyrate modulates epithelial growth through cell proliferation, differentiation and apoptosis in the small intestine of piglets and stomach of calves and goats (Kotunia et al., 2004; Guilloteau et al., 2009; Malhi et al., 2013). Butyrate improved papillae size and thereby increased surface area in the dorsal sac of the rumen in bulls (Kowalski et al., 2015). Increasing luminal butyrate concentration either by enhancing its endogenous production through dietary manipulation (Guilloteau et al., 2010) or by exogenous administration (Sakata and Tamate, 1978; Shen et al., 2005) has been shown to enhance ruminal epithelial proliferation. The proliferative effects of butyrate differ not only with the age and physiological status of the animals but also with the dose, route and rate of butyrate administration. In young calves and starved adult sheep butyrate induces epithelial proliferation at high doses > 2.5 g/kg of BW/day (Sakata and Tamate, 1978; Mentschel et al., 2001) and in fed cattle at low doses < 0.5 g/kg of BW/day (Shen et al., 2005). Furthermore, the rapid infusion of butyrate induced proliferation whereas the slow infusion method did not (Sakata and Tamate, 1978; Mentschel et al., 2001). In addition to cell proliferation, apoptosis is a vital process involved in the maintenance of the cell homeostasis and modulates the organ growth. Besides rapid proliferation, butyrate also stimulates the apoptosis in order to maintain the normal cell homeostasis (Mentschel et al., 2001). Oral administration of butyrate reduced apoptosis in ruminal epithelium of calves. In colonic epithelial cell culture, the butyrate deprived medium reduced cell number in each phase of cell cycle, with the maximum reduction in G1 phase caused by apoptosis (Luciano et al., 1996). In vivo butyrate administration exerts selective effects on cells depending on their phenotypes. Butyrate increased proliferation of normal intestinal cells in piglets (Kien et al., 2008) whereas inhibited proliferation and increased apoptosis in artificially induced-tumor cells (aberrant crypt foci, ACF) in rat intestine (Clarke et al., 2012). It seems that butyrate stimulates apoptosis only in unwanted or defective cells produced during rapid proliferation.
Though the considerable studies have been carried out to evaluate the influence of butyrate on ruminal epithelial growth but the underlined molecular mechanism particularly in reference with cell cycle and apoptosis has not been fully discovered yet. In continuation with our previous study, in which we showed that butyrate induced ruminal epithelial growth is associated with cyclin D1 upregulation, the present study was intended to explore further the influence of butyrate on expression of genes involved in cell cycle and apoptosis.
Materials and Methods
The experimental plan and procedures were approved by Animal Care and Use Committee for Livestock at Nanjing Agricultural University following the requirements of the regulations for the administration of affairs concerning experimental animals under the “The State Science and Technology Commission of China, 1988” act.
Experimental Design, Goat Management, and Infusion Method
Twenty four rumen-fistulated goats (Boer × Yangtze River Delta White) of approximately 4 months of age; 18.29 ± 0.64 kg of body weight (BW) at the start of the experiment were used in this study. The goats were housed in individual cemented floor pens (1.2 m × 1.0 m) and were randomly divided into two groups, i.e., A and B (n = 12 each). Animals in group B were given intraruminal infusion of sodium butyrate (Merck, Hohenbrunn, Germany) at the dose rate of 0.3 g/kg of BW added in 50 ml of 0.1 mol/l potassium phosphate buffer. Whereas animals in group A received the same amount of buffer without butyrate. The butyrate infusion was performed 1 h before morning feeding within 10–15 s. Animals were fed concentrate (200 g) two times daily in 2 equal meals at 8:00 am and 5:00 pm and provided with free access to hay and water. Table 1 depicts all of the ingredients in the feed and their chemical compositions. Body weight of goats was recorded weekly. The duration of the experiment was 28 days.
Sampling and chemical analysis of the feed was performed on days 7 and 28. On day 14 of infusion trial the ruminal fluid samples were collected in equal portion from atrium ruminis, ventral rumen and caudal dorsal and caudal ventral regions by suction through inserting a pipe and combined to form one sample at 0, 1, 2.5, 3.5, 5, and 7 h post-infusion. The pH was determined by digital pH meter and then the samples were immediately strained through 4 layers of gauze. Afterwards, 1 ml of 5% HgCl2 solution was added to 20 ml of rumen fluid and preserved at -20°C for SCFA analysis. Goats were slaughtered on day 28 (4 goats from each group) at three different time points, i.e., 5, 7, and 9 h after last infusion. After slaughter, the abdomens were immediately opened, and the stomachs were exteriorized. After emptying and washing with PBS (pH 7.4), ruminal tissues (approximately 2 cm2) from the atrium ruminis, ventral rumen sac and ventral blind sac were taken for morphological analyses of the papillae. These tissues were fixed in 4% paraformaldehyde solution. Epithelium for RNA extraction was taken from ventral blind sac (∼10 g). After cleansing with ice-cold PBS, the epithelium was detached from muscle layer by hand, shifted into liquid nitrogen and then stored at -80°C until analyzed for PCR.
Determination of Ruminal SCFA Concentration
The SCFA concentrations in the ruminal fluids were detected using a HP6890N chromatograph (Agilent Technologies, Wilmington, DE, United States) according to a standard method (Yang et al., 2012). The carrier gas was nitrogen (99.99% purity) and was applied at a steady flow rate of 2.8 ml/min with a split ratio of 1:30. The capillary column temperature was fixed at 140°C for 4 min and then increased at 25°C/min to 240°C. Regarding the injection port and the flame-ionization detector (FID), the temperatures were set to 180 and 250°C, respectively. Crotonic acid (CH3CH) was used as the internal standard.
Morphometric and Histomorphometric Analyses
One centimeter square piece of paraformaldehyde fixed epithelium, taken from each of the anterior, ventral and ventral blind sac of the rumen was used for morphological analyses of the papillae. Papilla was held gently with forceps and the caliper was slowly opened wide, from base to the tip of papilla to measure the length, and from one side to the other at the mid of papillae to measure the width. The papillae were cut from these epithelial sections and counted (density, number/cm2). For every goat in groups B and A, 15 papillae were measured from each of the samples of the atrium ruminis, ventral rumen sac and ventral blind sac. The surface areas of the rumen mucosa (mm2/cm2) were determined as the length × width × density × 2.
For the histomorphological evaluations, the formalin fixed tissue samples from the atrium ruminis were taken, dehydrated, cleared and embedded in paraffin. Sections of 4-μm thickness were cut and stained by the standard hematoxylin and eosin (H&E) procedure. For each tissue, 25 to 30 papillae were embedded for paraffin-sectioning and microscopic observation. From these, the 4 paraffin sections with the best orientation of papillae in the median sagittal plane were used to evaluate the morphological characteristics of the ruminal papillae by using Image-Pro Plus 6.0 (Media Cybernetics Inc., Bethesda, MD, United States).
For each papillae three visual fields were used for counting cell density from three cell layers of the epithelium, i.e., SS+ SG = the stratum germinativum (SGv) and stratum basale (SB), to reveal the following parameters:
(i) The epithelial cell density of the SGvs in numbers of cells per mm2 (n/mm2).
(ii) The epithelial cells in SB are in single row and there counting unit is numbers of cells per mm (n/mm).
Total RNA Extraction, cDNA Synthesis and Quantitative Real-Time PCR
The guanidinium thiocyanate-phenol-chloroform extraction method reported by Chomczynski and Sacchi (2006) was used for the total RNA extraction from the homogenized ruminal tissue. The RNA concentration and integrity were evaluated based on extinction measurements at 260 and 280 nm made with a Biophotometer (Eppendorf, Hamburg, Germany). The absorption ratio (260:280) was between 1.8 and 2.0 for all of the RNA samples, which indicated high RNA purity in every sample. Aliquots of RNA samples were subjected to electrophoresis through a 1.4% agarose-formaldehyde gel to verify integrity. The RNA concentrations in the samples were then adjusted to1 μg/μl. Random hexamer primers (Invitrogen, Shanghai, China) and Moloney murine leukemia virus (M-MLV) reverse transcriptase (Fermentas, Burlington, ON, Canada) were used for the cDNA synthesis. The target genes of interest and their respective sources and primer sequences are listed in Table 2.
The relative gene expression was determined via real-time polymerase chain reaction (PCR) by using the MyiQ2 two-color real-time PCR detection system (Bio-Rad Laboratories, Inc., Hercules, CA, United States). Real-time PCR was performed in a total volume of 20 μl containing 1× iQSYBR Green Supermix (Bio-Rad Laboratories Inc., Hercules, CA, United States), a mixture of the forward and reverses primers (500 nM each), cDNA template (1 ng), and a known amount of sterile water for volume adjustment. An initial cycle of 30 s at 95°C was used to denature the cDNA. Forty PCR cycles involving denaturation at 95°C for 10 s and primer annealing and extension at 55°C for 30 s were then performed. A standard dilution series was used to calculate the amplification efficiencies of all of the primers before performing the PCRs for the experimental samples. The efficiencies of all the primers used were between 97 and 101%. Gene expression was normalized to GAPDH. All samples were run in triplicate. These experiments were repeated twice with 80–90% similarity. Melt curve analyses were performed after all of the PCR analyses. The data were analyzed via PCR array data analyses based on the ΔCt method with normalization of the raw data to GAPDH; i.e., ΔCt = Cttarget -CtGAPDH, where Ct = the cycle threshold. The relative gene expression values were calculated using the formula 2-ΔΔCt, as explained by (Livak and Schmittgen, 2001). All samples were analyzed in triplicate.
Statistical Analysis
Data are expressed as means ± SE. Differences with a P-value of <0.05 were considered significant. An independent sample t-test (two-tailed test) was used to compare the data between two groups. All statistical analyses were performed by using SPSS software (version 13.0.1 for Windows; SPSS, Chicago, IL, United States).
Results
Ruminal Short Chain Fatty Acid Concentration
Figure 1 shows the effect of butyrate infusion on ruminal fermentation pattern of goats. At 0 h the individual and total SCFA concentrations did not differ between the groups, however, at 1 h after butyrate infusion, the molar concentration (mmol/l) of butyrate (17.96) in B increased by about 99.78% (P < 0.01) compared to A (8.99). Whereas the molar concentrations (mmol/l) of acetate (48.93 in B vs. 54.50 in A) and propionate (13.54 in B vs. 17.41 in A) were decreased (P < 0.05) in B compared to A. The concentrations of both acetate and propionate in group B returned to the base-line levels by 2.5 h whereas the butyrate concentration remained significantly elevated up to 2.5 h and then returned to its base-line value by 3.5 h.
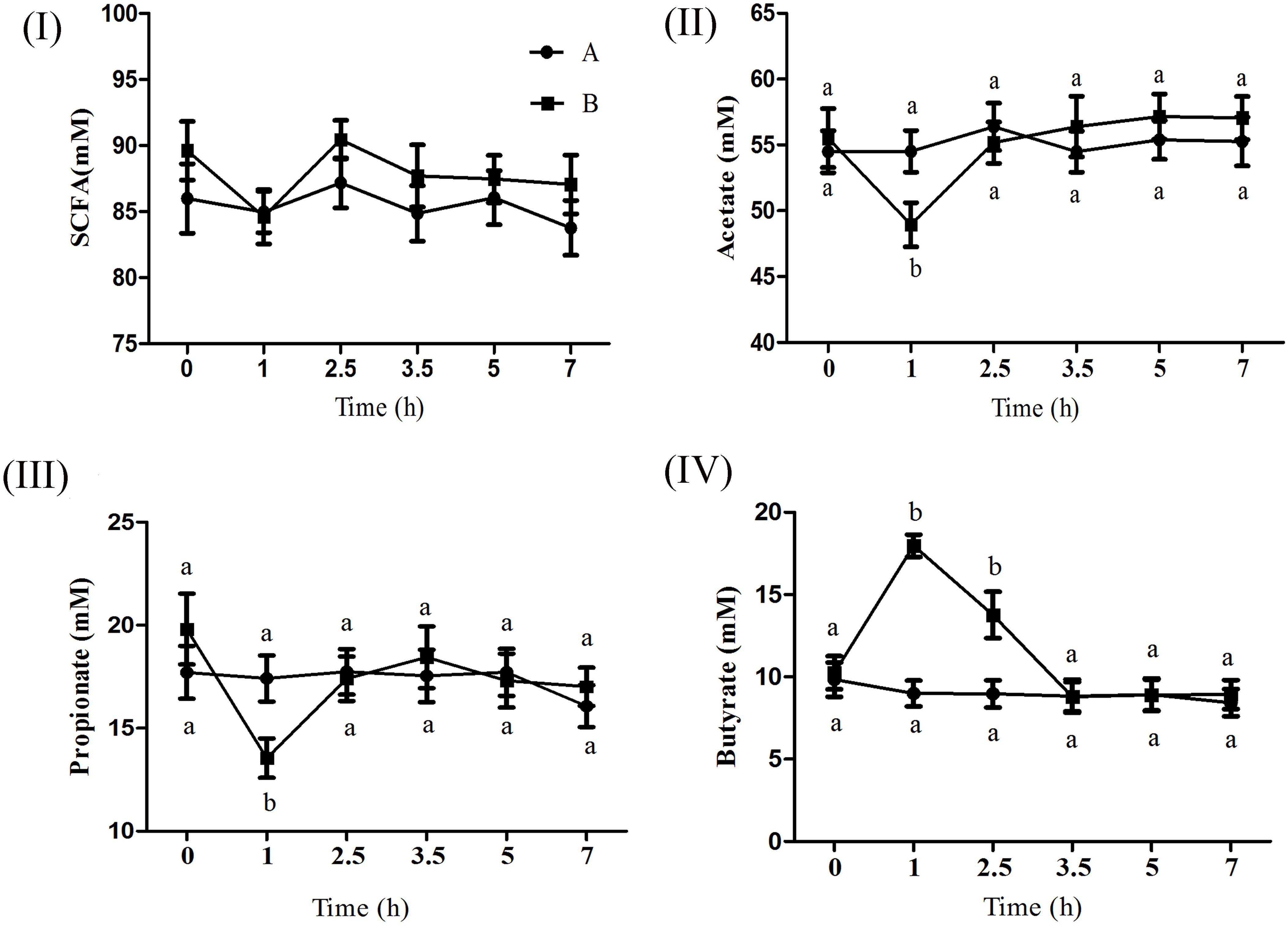
FIGURE 1. Effect of ruminal butyrate infusion on the molar concentration of total short chain fatty acids (I), acetate (II), propionate (III), and butyrate (IV) at 6 time points on day 14th of butyrate infusion. The goats were infused by 0.1 M potassium phosphate buffer (50 ml) with sodium butyrate at 0.3 (B = butyrate group, n = 12) or 0 (A = control group, n = 12) g/kg of BW per day for 28 days in the experimental duration. Values (means ± SE) differ if they do not share a common letter: a,bP < 0.05.
Morphometric and Histometric Analysis of Rumen Papillae
Butyrate infusion significantly increased (P < 0.05) the papillae length, width and density in the atrium ruminis (AR), ventral rumen (VR), and ventral blind sac (VBS) compared to group A (Table 3 and Figure 2). The increases in papillae length, width and density led to an increase (P < 0.05) in the surface area of all the tested regions in group B compared to group A. Histometric analysis of rumen papillae revealed that the cell density in the stratum germinativum (SGv) and stratum basale (SB) increased (P < 0.05) in group B compared with group A (Table 4 and Figure 3).
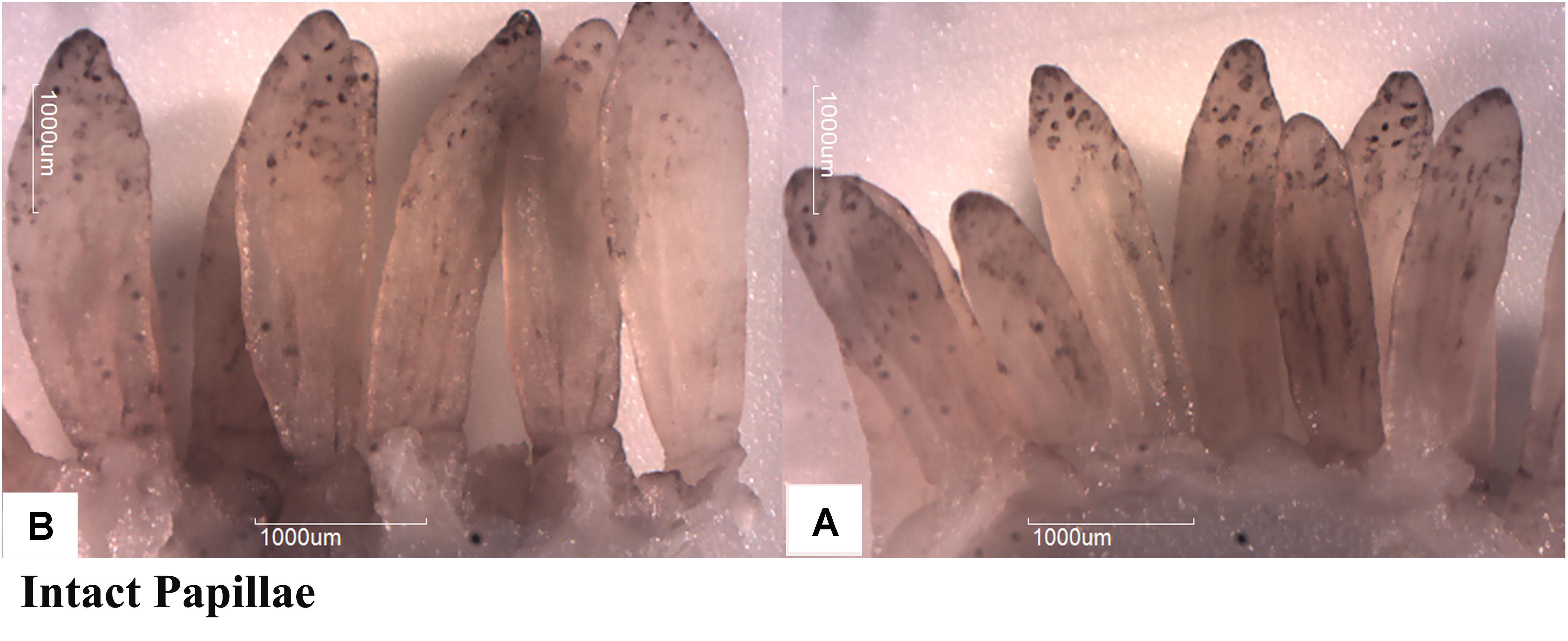
FIGURE 2. Effect of ruminal butyrate infusion on papillae growth. Goats were infused by 0.1 M potassium phosphate buffer (50 ml) with sodium butyrate at 0.3 (B = butyrate group, n = 12) or 0 (A = control group, n = 12) g/kg of BW per day for 28 days.
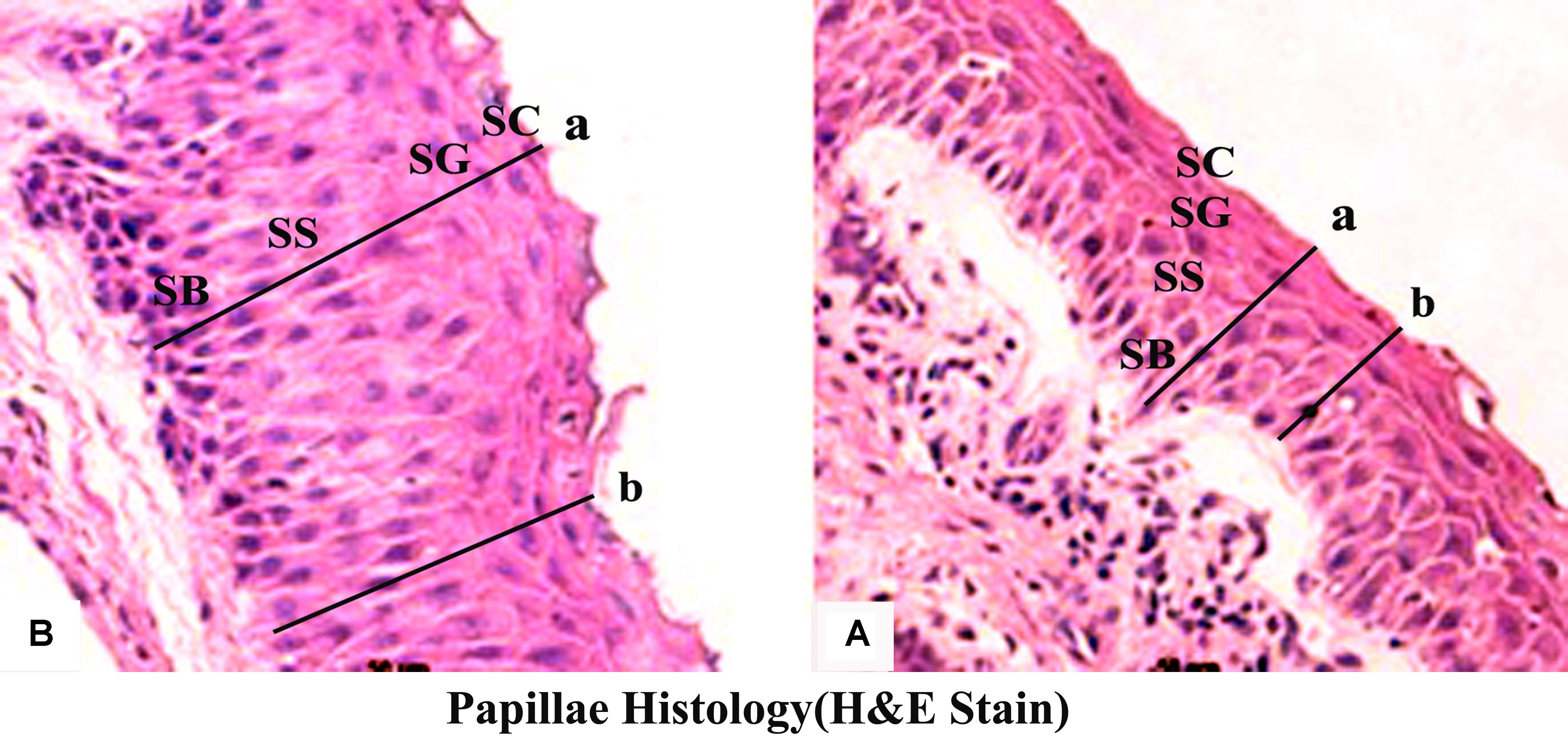
FIGURE 3. Effect of ruminal butyrate infusion on rumen papillae histology. Goats were infused by 0.1 M potassium phosphate buffer (50 ml) with sodium butyrate at 0.3 (B = butyrate group, n = 12) or 0 (A = control group, n = 12) g/kg of BW per day for 28 days. Histomicrographs showing epithelial strata at maximum (a) and minimum (b) depths in epithelium of goats. Morphological characteristics of the ruminal papillae observed by using Image-Pro Plus 6.0 (Media Cybernetics Inc., Bethesda, MD, United States).
Gene Expression Analyses
The mRNA expression levels of cyclin D1, CDK4, CDK6, CDK2, and p21 were significantly increased (P < 0.05) in group B compared to A at 5 h after butyrate infusion (Figure 4I). Whereas the butyrate infusion increased (P < 0.05) the mRNA expression levels of cyclin E1 at 7 h and that of cyclin A and CDK1at 9 h post-infusion compared to A (Figures 5I, 6I). The assessment of genes showed that the butyrate infusion caused significant increase (P < 0.05) in mRNA expression levels of caspase 3, caspase 9 and Bax at 5 h post-infusion compared to control (Figure 4II). However, the expression levels of apoptotic genes at 7 and 9 h post-infusion were remained unchanged (P > 0.05) between the groups (Figures 5II, 6II). Furthermore, the increased mRNA expression levels of cyclin D1, cyclin E1, CDK2, 4, and 6, and P21, caspase 3, caspase 9 and Bax were positively correlated with the ruminal butyrate concentration in B and the differences were significant (P ≤ 0.05, Table 5).
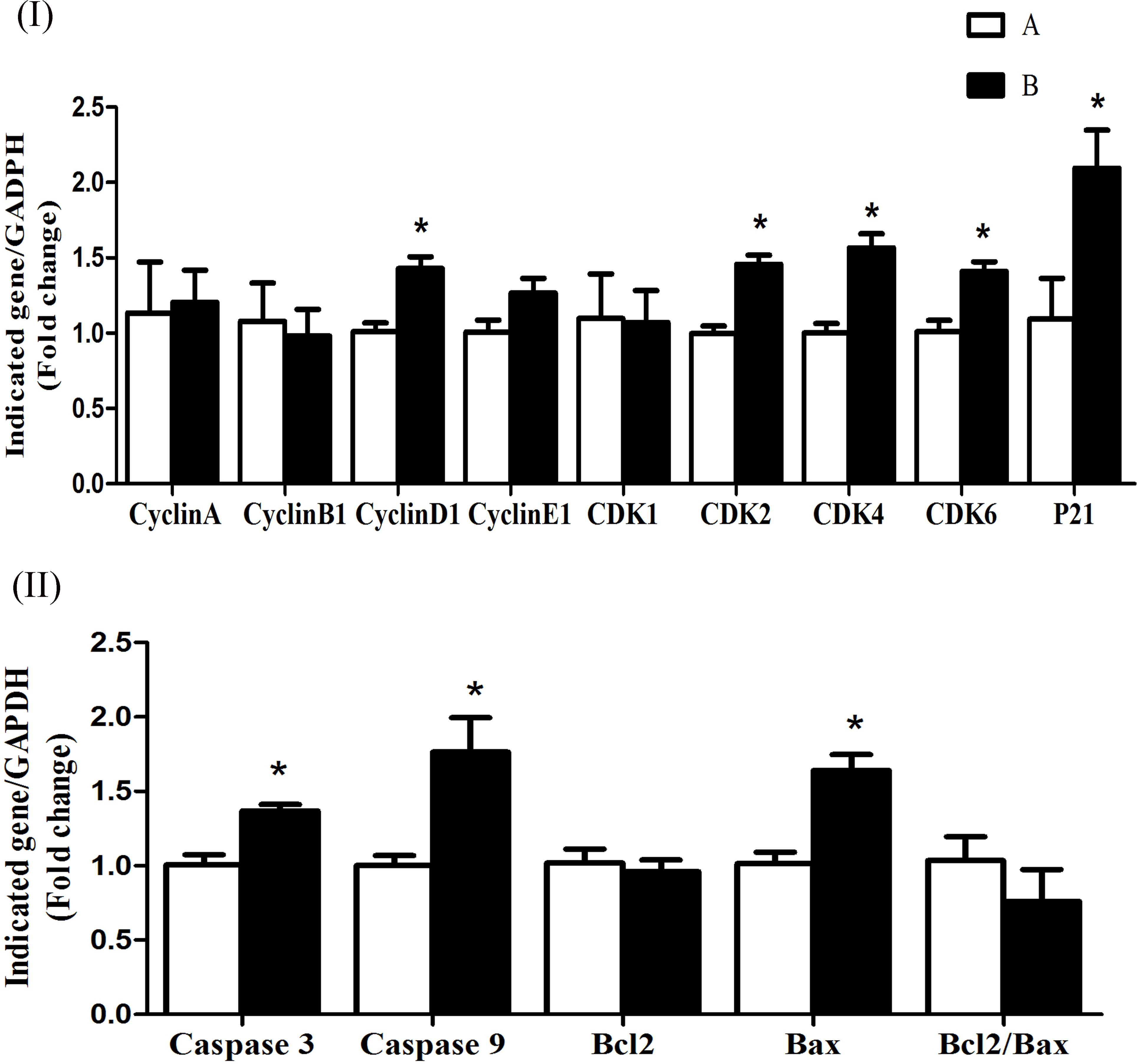
FIGURE 4. Effect of ruminal butyrate infusion on mRNA expression of (I) proliferation and cell cycle inhibition and (II) apoptosis related genes in the rumen epithelium of goats. Goats were infused by 0.1 M potassium phosphate buffer (50 ml) with sodium butyrate at 0.3 (B = butyrate group, n = 4) or 0 (A = control group, n = 4) g/kg of BW per day for 28 days and slaughtered on day 28 at 5 h after infusion. The levels of gene expression were calculated with real-time PCR in comparison with GAPDH mRNA. Values are mean ± SE. Asterisks exhibit the differences between groups with ∗P < 0.05.
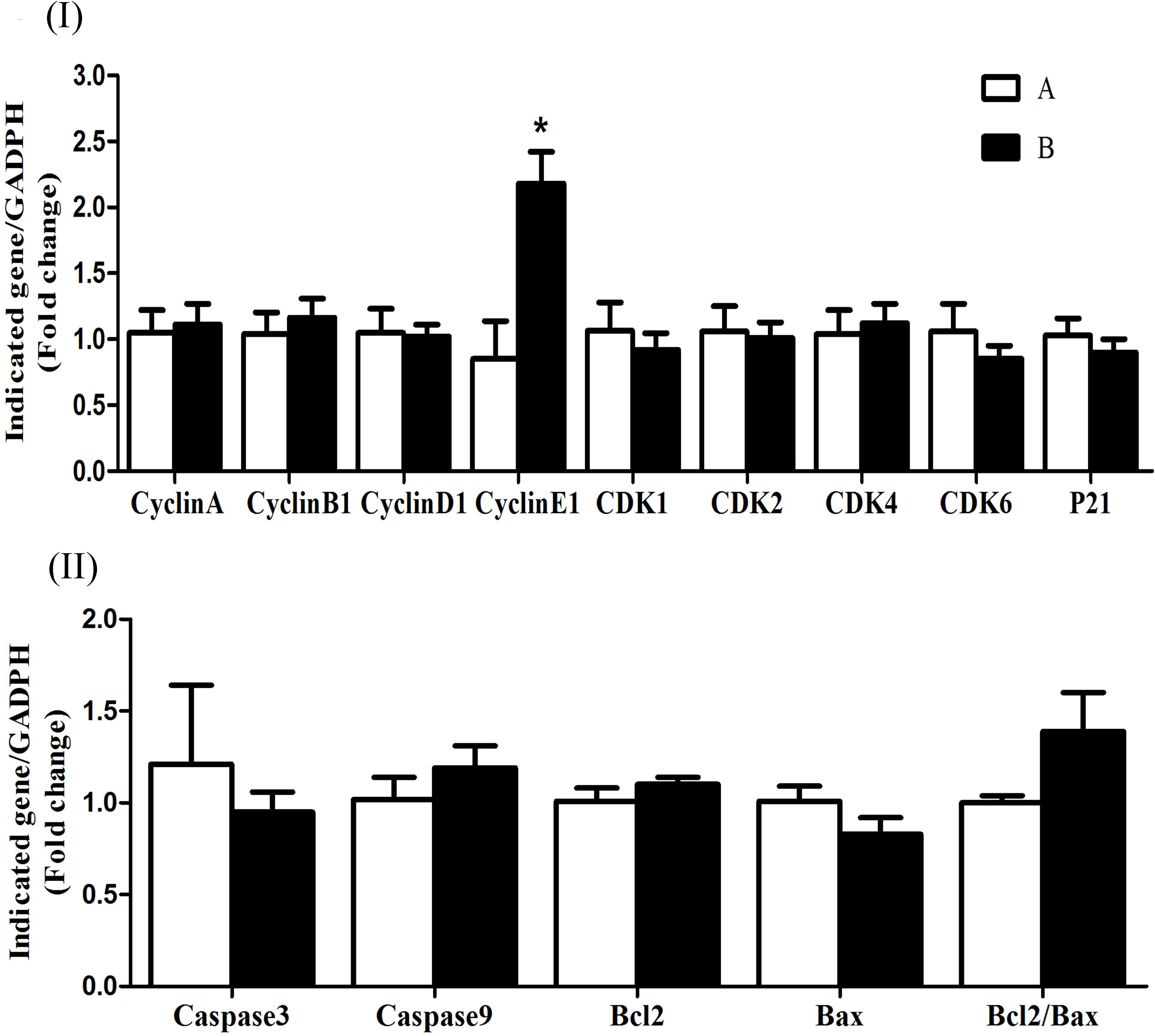
FIGURE 5. Effect of ruminal butyrate infusion on mRNA expression of (I) proliferation and cell cycle inhibition and (II) apoptosis related genes in the rumen epithelium of goats. Goats were infused by 0.1 M potassium phosphate buffer (50 ml) with sodium butyrate at 0.3 (B = butyrate group, n = 4) or 0 (A = control group, n = 4) g/kg of BW per day for 28 days and slaughtered on day 28 at 7 h after infusion. The levels of gene expression were calculated with real-time PCR in comparison with GAPDH mRNA. Values are mean ± SE. Asterisks exhibit the differences between groups with ∗P < 0.05.
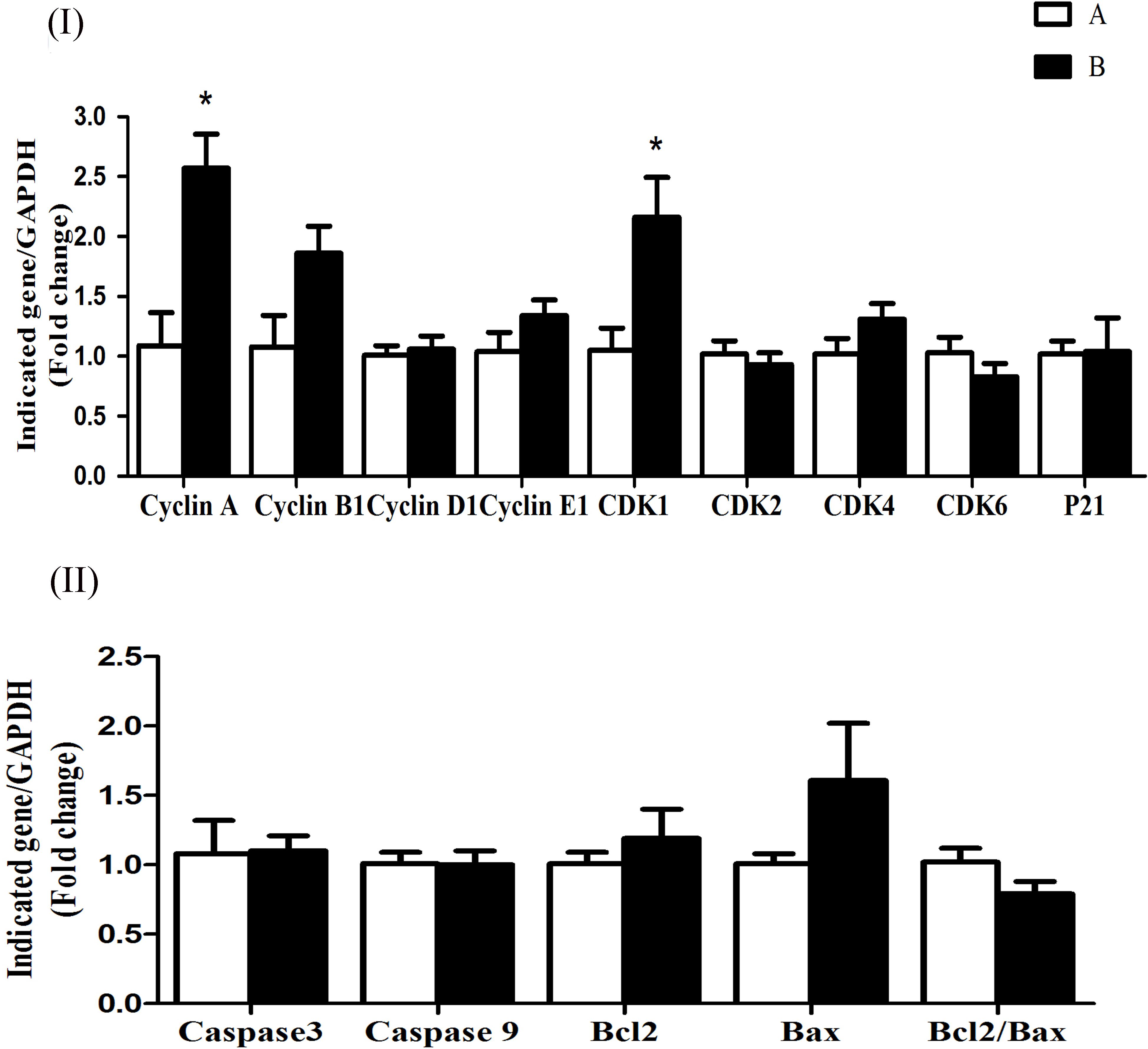
FIGURE 6. Effect of ruminal butyrate infusion on mRNA expression of (I) proliferation and cell cycle inhibition and (II) apoptosis related genes in the rumen epithelium of goats. Goats were infused by 0.1 M potassium phosphate buffer (50 ml) with sodium butyrate at 0.3 (B = butyrate group, n = 4) or 0 (A = control group, n = 4) g/kg of BW per day for 28 days and slaughtered on day 28 at 9 h after infusion. The levels of gene expression were calculated with real-time PCR in comparison with GAPDH mRNA. Values are mean ± SE. Asterisks exhibit the differences between groups with ∗P < 0.05.
Discussion
Fermentation Pattern and Morphology of Rumen
Butyrate concentration in rumen fluid of B group increased from 1 to 2.5 h post-infusion compared to A group and declined subsequently. Conversely the molar concentration of acetate and propionate significantly decreased at 1 h post-infusion. The changes in the concentration of three major ruminal SCFAs in response to intraruminal infusion of butyrate are consistent with previous study (Malhi et al., 2013). The reduction of acetate and propionate might be due to inhibitory impact of butyrate on their production by ruminal microbes (Li et al., 2012) or butyrate could promote the absorption of acetate and propionate by the ruminal epithelium (Malhi et al., 2013). In the present study the ruminal infusion of butyrate increased dimensions and density of papillae in atrium ruminis, ventral sac and ventral blind sac of rumen. Furthermore, the histometric analysis showed that the number of cell layers in SGv and density of cells in SB increased in butyrate infused goats compared to control. This could be largely attributed to increased intraruminal butyrate concentration as previously reported by (Mentschel et al., 2001; Shen et al., 2005; Malhi et al., 2013).
Genes Expression Related to Proliferation and Apoptosis
In the present study, the butyrate infusion showed time-dependent effects on genes related to cell cycle and apoptosis in ruminal epithelium. The mRNA expressions level of cell cycle genes involved in early to mid G1 phase, i.e., cyclin D1, CDK2, CDK4, and CDK6 increased at 5 h and those involved in late G1 phase, i.e., cyclin E1 at 7 h after butyrate infusion. Based on histo-morphometric studies, many researchers have demonstrated that butyrate improves rumen epithelial growth through increased cell proliferation (Sakata and Tamate, 1978; Mentschel et al., 2001; Shen et al., 2004). The cell cycle of a mammalian cell including ruminal epithelium is a complex process consisting of four phases including G1, G2, S, and M (Goodlad, 1981; Mathew et al., 2010). In general, the rumen epithelial cells complete the cell cycle in a period of 24 h, however, the duration may increase or decrease depending upon shortening or elongation of various cell cycle phases under the influence of diet or dietary-produced SCFA (Goodlad, 1981). The cell cycle phases are strictly regulated by cyclins and cyclin-dependent kinases (CDK) proteins (Matsushime et al., 1992; Jacks and Weinberg, 1996). Cyclin D1 forms a complex with either CDK4/6 that promotes the cells from early to mid G1 phase (Mathew et al., 2010). The upregulation of cyclin D1 and CDK 4/6 at 5 h after butyrate infusion suggest that butyrate enhanced the transition of cells through G0/mid-G1 phase. The cyclin E1 mRNA synthesis is initiated during the G1 phase and its expression reaches at its peak in late G1 (Petersen et al., 1999; Coverley et al., 2000). In the present study, the increased cyclin E1 mRNA expression level in ruminal epithelial tissue collected at 7 h post-infusion suggest that the cell population was in G1/S transition phase. If we presume that at the time of butyrate infusion (0 h) the ruminal epithelial cells were in G0-phase, the cells took 4–5 h to enter mid-G1 phase and another 2–3 h to enter the mid/late G1 phase and the total duration of G1-phase would be 6–7 h. In sheep fed roughage-based diet, the maximum duration of G1 phase in rumen epithelial cells was 8.2 h which reduced to 6.5 h when the sheep were transitioned from roughage to concentrate based-diet (Goodlad, 1981). Previous studies have shown that butyrate induces ruminal epithelial growth in goats by shortening G1 phase through cyclin D1 upregulation (Malhi et al., 2013). Moreover, the cyclin D1 expression was influenced by sampling time. According to our previous report, ruminal tissue samples collected at 3 h post-infusion of butyrate already showed significant increase in cyclin D1 mRNA expression level compared to control whereas at 7 h post-infusion did not (Malhi et al., 2013). In the present study, we also observed that cyclin D1 expression was remained elevated at the ruminal tissue samples collected at 5 h post-infusion but not at 7 h post-infusion (Figure 4). On the other hand, we found significantly increased CDK4 expression (Figure 4) but they not observe any change in CDK4 (Malhi et al., 2013). The reason of this contradiction is not clear. To our best knowledge, the influence of in vivo butyrate on cyclins A, B and E1, and CDK 1 and 2 and their possible role in ruminal epithelial growth has not been previously reported. However, high concentrate diet-induced ruminal epithelial proliferation was associated with modulation of cell cycle genes including cyclins D1, E1, A and B, and CDK 2/4 and 6 (Gui and Shen, 2016). The activity of cyclin E requires CDK2 with which it forms cyclin E1/CDK2 complex that derives the cell cycle through late G1/S phase (Sherr and Roberts, 1999, 2004). However, in the present study, we did not observe any change in CDK2 mRNA expression. This suggests that there could be an alternate mechanism for binding with cyclin E1 to drive the late G1 events of cell cycle. The loss of CDK2 in mice did not affect G1/S transition and cell cycle progression and it was suggested that either cyclin E1 performed independent of CDK2 (Berthet et al., 2003; Ortega et al., 2003) or possibly formed cyclin E/CDK1 complex (Aleem et al., 2005). Recently, it has been shown that cyclin E1 activates CDK3 and forms cyclin E/CDK3 complex to promote G1/S transition (Satyanarayana and Kaldis, 2009).
Once the cells enter the S-phase the cyclin E1 expression is subsided and the synthesis of cyclin A mRNA is initiated and its expression becomes maximal with the progression of S-phase (Petersen et al., 1999; Coverley et al., 2000). In the present study, the cyclin A mRNA expression increased in sample collected at 9 h post-infusion which suggests that the cell population entered the S-phase. The activity of cyclin A requires either CDK1 or 2 to form the complex cyclin A/CDK1/2 which regulates the transition of cells through S-phase. In the present study, the CDK1 mRNA expression concurrently increased with cyclin A mRNA expression. Finally cyclin B is required prior to mitosis (G2/M-phase) (Petersen et al., 1999; Coverley et al., 2000) which binds with CDK1 to accomplish cell division (Riabowol et al., 1989). In the present study, no significant changes in cyclin B1 mRNA expression were observed in ruminal epithelial tissue. This can be explained by the fact that cell populations in the tissues collected at specific time points in the present study did not arrive at G2/M-phase of cell cycle. However, the tissue samples collected at 9 h showed that butyrate infusion caused slight increase in cyclin B1 expression which indicates the transition of cells through late-S/G2-M phase since the genes required in next phase of cycle initiate to express in late stage of previous phase (Petersen et al., 1999; Coverley et al., 2000). The rise in cyclin B1 expression could be expected if the fourth ruminal tissue sample were collected at certain time-point after 9 h post-infusion. The present data demonstrate that the proliferative effects of butyrate depend upon the specific stage of dividing cells. Thus, while performing in vivo experiments the location and tissue sampling time is very important because the tissue sample collected from a particular site at one specific time would represent the cell population in a particular phase of cell cycle with expression of associated genes (Li et al., 2009; Yang et al., 2012).
In the present study, the butyrate infusion increased p21 mRNA expression level in ruminal tissue collected at 5 h post-infusion. The p21 has anti-proliferative effects which binds with CDKs and prevent cell cycle progression and arrest cell cycle at any phase through G0/G2 phases (Ogryzko et al., 1997; Niculescu et al., 1998). It has been shown that the increased proliferation rate in rumen epithelium leads to rise in apoptosis in order to maintain the normal cell homeostasis (Mentschel et al., 2001). Consistent with our findings, (Crim et al., 2008) have shown that in vivo butyrate administration upregulated p21 expression in colonocytes of rats. In addition, butyrate-induced p21 upregulation increased apoptosis in artificially induced-tumor cells (aberrant crypt foci, ACF) in rats fed fish oil but no apoptotic effects were observed in ACF in rats fed corn oil. This suggests that apoptotic effects of butyrate depend upon the associated factors and physiological status of the cell even after p21 upregulation. Many studies have shown such selective effects of in vivo butyrate which depend upon physiological status and cellular phenotypes. Cecal infusion of butyrate increased proliferation of normal intestinal cells in piglets (Kien et al., 2008) whereas butyrate inhibited proliferation and increased apoptosis in artificially induced-ACF in rat intestine (Clarke et al., 2012). The results of present study demonstrate that butyrate induced apoptosis in ruminal epithelial cells during rapid proliferation, since the overall ratio of proliferation was higher than the apoptosis which was reflected in epithelial growth (Figure 7).
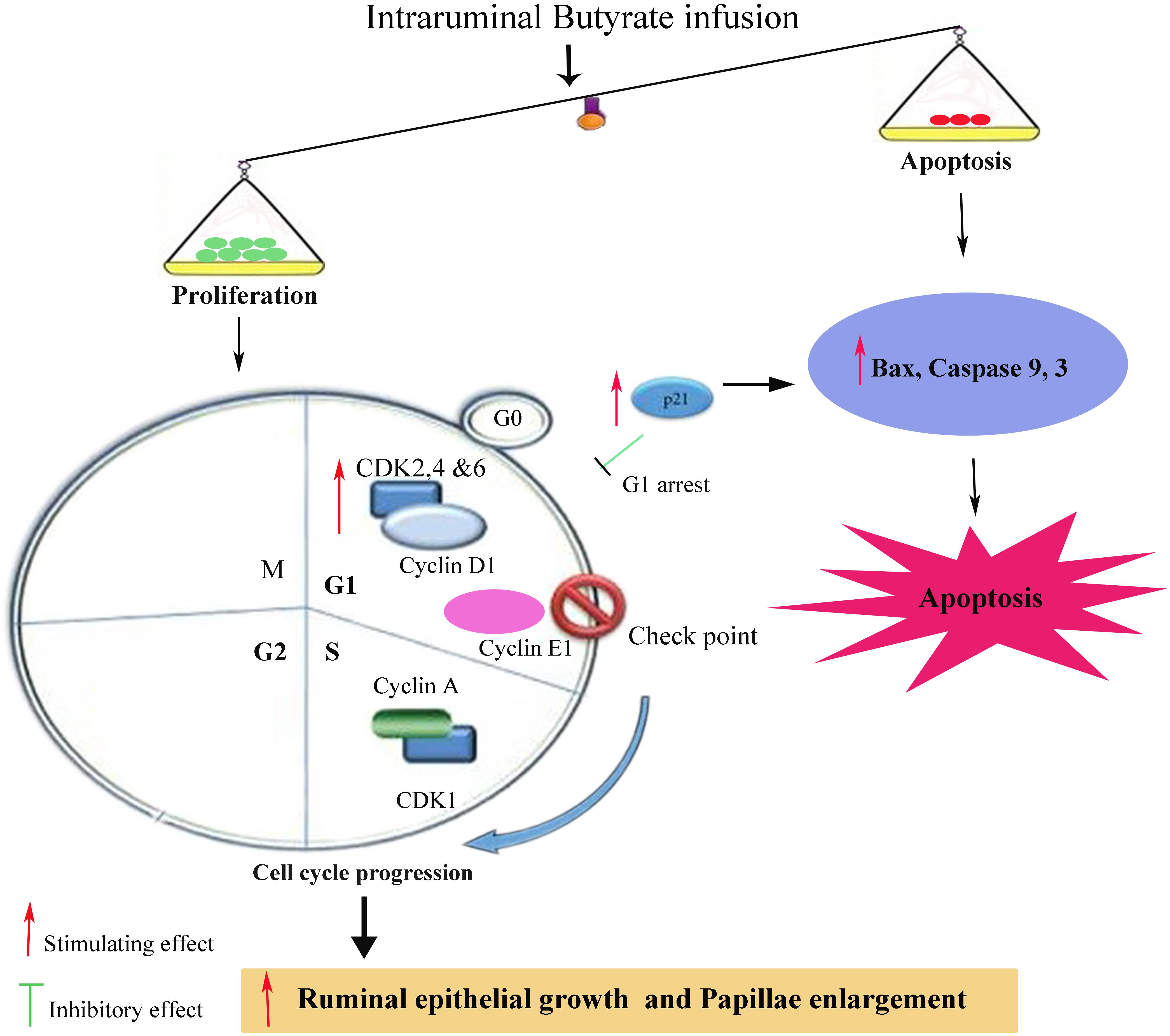
FIGURE 7. Schematic diagram showing influence of ruminal Sodium Butyrate (SB) infusion on expression of genes related to proliferation and apoptosis in rumen epithelium of goat. SB increased proliferation through upregulation of cell cycle related genes involved in early G1 (cyclin D1, CDK2, CDK4, and CDK6), mid G1 (cyclin E1) and S phase (cyclin A and CDK1). Simultaneously, SB also increased the mRNA expression level of p21, Bax, Caspases 3 and 9, leading to cell cycle arrest and apoptosis. Since, the rate of proliferation was higher than apoptosis which was reflected in ruminal epithelial growth and papillae enlargement.
For normal growth and tissue homeostasis a balance between cell proliferation and apoptosis is important. Depending on the cellular context these two antagonistic processes are linked via shared molecular machinery (Evan et al., 1995). In the present study, the butyrate infusion caused upregulation of proapoptotic genes, i.e., Bax, caspase 3 and caspase 9 in ruminal epithelial tissues at 5 h post-infusion, however, the expressions of apoptotic genes at 7 and 9 h were not influenced by the butyrate treatment. This suggests that butyrate induced apoptosis in ruminal epithelial cells by arresting cell cycle at G1. The inhibitory effects of butyrate on G1 phase of cell cycle and the induction of apoptosis have been well established (Li and Elsasser, 2005; Li and Li, 2006; Mathew et al., 2010). As mentioned earlier that p21 is also upregulated at the same time point which suggests the involvement of p21 pathway in butyrate-induced apoptosis. Consistent with our findings, previous studies have shown that in vivo butyrate administration increased apoptosis through p21-dependent pathway in prostate cancer cell lines implanted in mice (Kuefer et al., 2004). Over expression of caspase 3, caspase 9, and Bax in the present study indicate that butyrate involved p21-dependent intrinsic pathway mediated by Bax and caspase 3. In prostate cancer cells butyrate treatment up-regulated Bax (Mu et al., 2013).
Conclusion
The present study demonstrates that butyrate stimulates epithelial growth by modulating both proliferative and apoptotic genes. Butyrate enhanced ruminal epithelial proliferation by shortening various cell cycle phases through associated cyclins/CDKs upregulation. Besides proliferation, butyrate induced apoptosis in rumen epithelium through activation of Bax, caspase 3, and caspase 9. Since the ratio of proliferation was higher than the apoptosis which was reflected in epithelial growth. To our knowledge, we report for the first time the effects of in vivo butyrate infusion on cell cycle and apoptotic genes in rumen epithelium of goats. Moreover, the environment of large intestine in mammals generally resembles with the environment of rumen so the results of present study can be extrapolated on mammals including human beings. However, the results of present study may be carefully interpreted as rumen epithelium is a complex tissue consisting of four different types of cell layers and the effects of butyrate vary with cell phenotypes. Nevertheless, this study provides a basis for understanding the molecular mechanism underlying the butyrate action on ruminal epithelial growth.
Author Contributions
JS wrote the paper. ZS conceived and designed the study. HG and BZ acquired the data. JS, HG, ZL, and BZ analyzed and/or interpreted the data. ZS, HG, and ZL drafted and revised the manuscript critically for important intellectual content. All authors read and approved the final manuscript.
Conflict of Interest Statement
The authors declare that the research was conducted in the absence of any commercial or financial relationships that could be construed as a potential conflict of interest.
Acknowledgments
The authors thank Chinese National “973” Project (No. 2011CB100801), the Priority Academic Program Development of Jiangsu Higher Education Institutions (PAPD), and Sindh Agriculture University, Tandojam, Pakistan, under the project titled “Strengthening and Development of Sindh Agriculture University Tandojam” for the financial support. The authors also thank Dr. Moolchand Malhi in Sindh Agriculture University, Pakistan for his kind help in the revision of this manuscript.
References
Aleem, E., Kiyokawa, H., and Kaldis, P. (2005). Cdc2-cyclin E complexes regulate the G1/S phase transition. Nat. Cell Biol. 7, 831–836. doi: 10.1038/ncb1284
Bergman, E. (1990). Energy contributions of volatile fatty acids from the gastrointestinal tract in various species. Physiol. Rev. 70, 567–590. doi: 10.1152/physrev.1990.70.2.567
Berthet, C., Aleem, E., Coppola, V., Tessarollo, L., and Kaldis, P. (2003). Cdk2 knockout mice are viable. Curr. Biol. 13, 1775–1785. doi: 10.1016/j.cub.2003.09.024
Chomczynski, P., and Sacchi, N. (2006). The single-step method of RNA isolation by acid guanidinium thiocyanate–phenol–chloroform extraction: twenty-something years on. Nat. Protoc. 1, 581–585. doi: 10.1038/nprot.2006.83
Clarke, J. M., Young, G. P., Topping, D. L., Bird, A. R., Cobiac, L., Scherer, B. L., et al. (2012). Butyrate delivered by butyrylated starch increases distal colonic epithelial apoptosis in carcinogen-treated rats. Carcinogenesis 33, 197–202. doi: 10.1093/carcin/bgr254
Coverley, D., Pelizon, C., Trewick, S., and Laskey, R. A. (2000). Chromatin-bound Cdc6 persists in S and G2 phases in human cells, while soluble Cdc6 is destroyed in a cyclin A-cdk2 dependent process. J. Cell Sci. 113, 1929–1938.
Crim, K. C., Sanders, L. M., Hong, M. Y., Taddeo, S. S., Turner, N. D., Chapkin, R. S., et al. (2008). Upregulation of p21Waf1/Cip1 expression in vivo by butyrate administration can be chemoprotective or chemopromotive depending on the lipid component of the diet. Carcinogenesis 29, 1415–1420. doi: 10.1093/carcin/bgn144
Dirksen, G., Liebich, H., Brosi, G., Hagemeister, H., and Mayer, E. (1984). Morphology of the rumen mucosa and fatty acid absorption in cattle–important factors for health and production. Zentralbl. Veterinarmed. A 31, 414–430. doi: 10.1111/j.1439-0442.1984.tb01300.x
Evan, G. I., Brown, L., Whyte, M., and Harrington, E. (1995). Apoptosis and the cell cycle. Curr. Opin. Cell Biol. 7, 825–834. doi: 10.1016/0955-0674(95)80066-2
Gäbel, G., Bestmann, M., and Martens, H. (1991a). Influences of diet, short-chain fatty acids, lactate and chloride on bicarbonate movement across the reticulo-rumen wall of sheep. J. Vet. Med. Ser. A 38, 523–529.
Gäbel, G., Vogler, S., and Martens, H. (1991b). Short-chain fatty acids and CO2 as regulators of Na+ and Cl- absorption in isolated sheep rumen mucosa. J. Comp. Physiol. B 161, 419–426.
Goodlad, R. A. (1981). Some effects of diet on the mitotic index and the cell cycle of the ruminal epithelium of sheep. Q. J. Exp. Physiol. 66, 487–499. doi: 10.1113/expphysiol.1981.sp002590
Gui, H., and Shen, Z. (2016). Concentrate diet modulation of ruminal genes involved in cell proliferation and apoptosis is related to combined effects of short-chain fatty acid and pH in rumen of goats. J. Dairy Sci. 99, 6627–6638. doi: 10.3168/jds.2015-10446
Guilloteau, P., Martin, L., Eeckhaut, V., Ducatelle, R., Zabielski, R., and Van Immerseel, F. (2010). From the gut to the peripheral tissues: the multiple effects of butyrate. Nutr. Res. Rev. 23, 366–384. doi: 10.1017/S0954422410000247
Guilloteau, P., Zabielski, R., David, J., Blum, J., Morisset, J. A., Biernat, M., et al. (2009). Sodium-butyrate as a growth promoter in milk replacer formula for young calves. J. Dairy Sci. 92, 1038–1049. doi: 10.3168/jds.2008-1213
Hamer, H. M., Jonkers, D., Venema, K., Vanhoutvin, S., Troost, F. J., and Brummer, R. J. (2008). Review article: the role of butyrate on colonic function. Aliment. Pharmacol. Ther. 27, 104–119. doi: 10.1111/j.1365-2036.2007.03562.x
Jacks, T., and Weinberg, R. A. (1996). Cell-cycle control and its watchman. Nature 381, 643–644. doi: 10.1038/381643a0
Kien, C. L., Peltier, C. P., Mandal, S., Davie, J. R., and Blauwiekel, R. (2008). Effects of the in vivo supply of butyrate on histone acetylation of cecum in piglets. JPEN J. Parenter. Enteral. Nutr. 32, 51–56. doi: 10.1177/014860710803200151
Kotunia, A., Wolinski, J., Laubitz, D., Jurkowska, M., Rome, V., Guilloteau, P., et al. (2004). Effect of sodium butyrate on the small intestine. J. Physiol. Pharmacol. 55, 59–68.
Kowalski, Z. M., Górka, P., Flaga, J., Barteczko, A., Burakowska, K., Oprządek, J., et al. (2015). Effect of microencapsulated sodium butyrate in the close-up diet on performance of dairy cows in the early lactation period. J. Dairy Sci. 98, 3284–3291. doi: 10.3168/jds.2014-8688
Krehbiel, C., Harmon, D., and Schnieder, J. (1992). Effect of increasing ruminal butyrate on portal and hepatic nutrient flux. J. Anim. Sci. 70, 904–914. doi: 10.2527/1992.703904x
Kuefer, R., Hofer, M. D., Altug, V., Zorn, C., Genze, F., Kunzi-Rapp, K., et al. (2004). Sodium butyrate and tributyrin induce in vivo growth inhibition and apoptosis in human prostate cancer. Br. J. Cancer 90, 535–541. doi: 10.1038/sj.bjc.6601510
Leonel, A. J., and Alvarez-Leite, J. I. (2012). Butyrate: implications for intestinal function. Curr. Opin. Clin. Nutr. Metab. Care 15, 474–479. doi: 10.1097/MCO.0b013e32835665fa
Li, C., and Elsasser, T. (2005). Butyrate-induced apoptosis and cell cycle arrest in bovine kidney epithelial cells: involvement of caspase and proteasome pathways. J. Anim. Sci. 83, 89–97. doi: 10.2527/2005.83189x
Li, M., Penner, G. B., Hernandezsanabria, E., Oba, M., and Guan, L. L. (2009). Effects of sampling location and time, and host animal on assessment of bacterial diversity and fermentation parameters in the bovine rumen. J. Appl. Microbiol. 107, 1924–1934. doi: 10.1111/j.1365-2672.2009.04376.x
Li, R. W., and Li, C. (2006). Butyrate induces profound changes in gene expression related to multiple signal pathways in bovine kidney epithelial cells. BMC Genomics 7:234. doi: 10.1186/1471-2164-7-234
Li, R. W., Wu, S., Baldwin, R. L. VI, Li, W., and Li, C. (2012). Perturbation dynamics of the rumen microbiota in response to exogenous butyrate. PLoS One 7:e29392. doi: 10.1371/journal.pone.0029392
Livak, K. J., and Schmittgen, T. D. (2001). Analysis of relative gene expression data using real-time quantitative PCR and the 2-ΔΔCT method. Methods 25, 402–408. doi: 10.1006/meth.2001.1262
Luciano, L., Hass, R., Busche, R., Von Engelhardt, W., and Reale, E. (1996). Withdrawal of butyrate from the colonic mucosa triggers “mass apoptosis” primarily in the G0/G1 phase of the cell cycle. Cell Tissue Res. 286, 81–92. doi: 10.1007/s004410050677
Malhi, M., Gui, H., Yao, L., Aschenbach, J. R., Gäbel, G., and Shen, Z. (2013). Increased papillae growth and enhanced short-chain fatty acid absorption in the rumen of goats are associated with transient increases in cyclin D1 expression after ruminal butyrate infusion. J. Dairy Sci. 96, 7603–7616. doi: 10.3168/jds.2013-6700
Mathew, O. P., Ranganna, K., and Yatsu, F. M. (2010). Butyrate, an HDAC inhibitor, stimulates interplay between different posttranslational modifications of histone H3 and differently alters G1-specific cell cycle proteins in vascular smooth muscle cells. Biomed. Pharmacother. 64, 733–740. doi: 10.1016/j.biopha.2010.09.017
Matsushime, H., Ewen, M. E., Strom, D. K., Kato, J.-Y., Hanks, S. K., Roussel, M. F., et al. (1992). Identification and properties of an atypical catalytic subunit (p34 PSK-J3/cdk4) for mammalian D type G1 cyclins. Cell 71, 323–334. doi: 10.1016/0092-8674(92)90360-O
Mentschel, J., Leiser, R., Mülling, C., Pfarrer, C., and Claus, R. (2001). Butyric acid stimulates rumen mucosa development in the calf mainly by a reduction of apoptosis. Arch. Anim. Nutr. 55, 85–102. doi: 10.1080/17450390109386185
Mu, D., Gao, Z., Guo, H., Zhou, G., and Sun, B. (2013). Sodium butyrate induces growth inhibition and apoptosis in human prostate cancer DU145 cells by up-regulation of the expression of annexin A1. PLoS One 8:74922. doi: 10.1371/journal.pone.0074922
Niculescu, A. B., Chen, X., Smeets, M., Hengst, L., Prives, C., and Reed, S. I. (1998). Effects of p21Cip1/Waf1 at both the G1/S and the G2/M cell cycle transitions: pRb is a critical determinant in blocking DNA replication and in preventing endoreduplication. Mol. Cell. Biol. 18, 629–643. doi: 10.1128/MCB.18.1.629
Nozière, P., Martin, C., Rémond, D., Kristensen, N. B., Bernard, R., and Doreau, M. (2000). Effect of composition of ruminally-infused short-chain fatty acids on net fluxes of nutrients across portal-drained viscera in underfed ewes. Br. J. Nutr. 83, 521–531.
Ogryzko, V. V., Wong, P., and Howard, B. H. (1997). WAF1 retards S-phase progression primarily by inhibition of cyclin-dependent kinases. Mol. Cell. Biol. 17, 4877–4882. doi: 10.1128/MCB.17.8.4877
Ortega, S., Prieto, I., Odajima, J., Martín, A., Dubus, P., Sotillo, R., et al. (2003). Cyclin-dependent kinase 2 is essential for meiosis but not for mitotic cell division in mice. Nat. Genet. 35, 25–31. doi: 10.1038/ng1232
Penner, G., Steele, M., Aschenbach, J., and McBride, B. (2011). Ruminant nutrition symposium: molecular adaptation of ruminal epithelia to highly fermentable diets. J. Anim. Sci. 89, 1108–1119. doi: 10.2527/jas.2010-3378
Petersen, B. O., Lukas, J., Sørensen, C. S., Bartek, J., and Helin, K. (1999). Phosphorylation of mammalian CDC6 by cyclin A/CDK2 regulates its subcellular localization. EMBO J. 18, 396–410. doi: 10.1093/emboj/18.2.396
Riabowol, K., Draetta, G., Brizuela, L., Vandre, D., and Beach, D. (1989). The cdc2 kinase is a nuclear protein that is essential for mitosis in mammalian cells. Cell 57, 393–401. doi: 10.1016/0092-8674(89)90914-8
Sakata, T., and Tamate, H. (1978). Rumen epithelial cell proliferation accelerated by rapid increase in intraruminal butyrate. J. Dairy Sci. 61, 1109–1113. doi: 10.3168/jds.S0022-0302(78)83694-7
Satyanarayana, A., and Kaldis, P. (2009). Mammalian cell-cycle regulation: several Cdks, numerous cyclins and diverse compensatory mechanisms. Oncogene 28, 2925–2939. doi: 10.1038/onc.2009.170
Shen, Z., Kuhla, S., Zitnan, R., Seyfert, H.-M., Schneider, F., Hagemeister, H., et al. (2005). Intraruminal infusion of n-butyric acid induces an increase of ruminal papillae size independent of IGF-1 system in castrated bulls. Arch. Anim. Nutr. 59, 213–225. doi: 10.1080/17450390500216894
Shen, Z., Seyfert, H. M., Löhrke, B., Schneider, F., Zitnan, R., Chudy, A., et al. (2004). An energy-rich diet causes rumen papillae proliferation associated with more IGF type 1 receptors and increased plasma IGF-1 concentrations in young goats. J. Nutr. 134, 11–17. doi: 10.1093/jn/134.1.11
Sherr, C. J., and Roberts, J. M. (1999). CDK inhibitors: positive and negative regulators of G1-phase progression. Genes Dev. 13, 1501–1512. doi: 10.1101/gad.13.12.1501
Sherr, C. J., and Roberts, J. M. (2004). Living with or without cyclins and cyclin-dependent kinases. Genes Dev. 18, 2699–2711. doi: 10.1101/gad.1256504
Keywords: butyrate, rumen epithelium, gene expression, cell cycle, cell apoptosis
Citation: Soomro J, Lu Z, Gui H, Zhang B and Shen Z (2018) Synchronous and Time-Dependent Expression of Cyclins, Cyclin-Dependant Kinases, and Apoptotic Genes in the Rumen Epithelia of Butyrate-Infused Goats. Front. Physiol. 9:496. doi: 10.3389/fphys.2018.00496
Received: 23 October 2017; Accepted: 18 April 2018;
Published: 23 May 2018.
Edited by:
Ghanshyam Upadhyay, City College of New York (CUNY), United StatesReviewed by:
Deepak Gurbani, University of Texas Southwestern Medical Center, United StatesSarbjeet Makkar, Washington University in St. Louis, United States
Khyati Shah, University of California, San Francisco, United States
Jingzhen Yuan, University of California, Los Angeles, United States
Copyright © 2018 Soomro, Lu, Gui, Zhang and Shen. This is an open-access article distributed under the terms of the Creative Commons Attribution License (CC BY). The use, distribution or reproduction in other forums is permitted, provided the original author(s) and the copyright owner are credited and that the original publication in this journal is cited, in accordance with accepted academic practice. No use, distribution or reproduction is permitted which does not comply with these terms.
*Correspondence: Zanming Shen, zmshen@njau.edu.cn; shenzanming@163.com