- 1Optics and Photonics Research Group, Faculty of Engineering, University Park, University of Nottingham, Nottingham, United Kingdom
- 2School of Life Sciences, University of Nottingham, Nottingham, United Kingdom
Quantum diamond sensors containing Nitrogen Vacancy (NV) centers were integrated into the culture of spontaneously electrically active cultures of live mouse primary cortical neurons. Two diamond formats were used enabling extracellular studies of cells cultured directly on a planar diamond plate and intracellular studies via endocytosis mediated integration of nanoscale diamond particles within cells. Over a 14 days culture period functional neuronal networks formed as confirmed by endogenous calcium transients detected via fluorescent imaging of a calcium probe (Fluo-5 AM). Spatially correlated images of photoluminescence from NV centers were also recorded demonstrating successful acquisition of optically detected magnetic resonance from regions of endogenously firing neuronal cultures. Further, a fast and simple measurement protocol based on acquisition of NV photoluminescence without and with the application of near resonant microwaves is presented. This protocol was applied to live signaling cells and dead cells. The relative photoluminescence from NVs with and without microwaves varied for the case of live as compared to dead cells warranting future investigation. Overall this work presents a protocol for integration of NV diamond sensors in a spontaneously active network of neuronal cells of relevance to the field of neuroscience. Photoluminescence from NV centers was detected with high spatiotemporal resolution using measurement protocols potentially capable of single shot detection of neuronal activity. It is anticipated that the methodologies introduced in this work will underpin the establishment of NV diamond sensors as a radically new measurement platform capable of rapid, non-destructive functional studies of cells.
Introduction
Spontaneous neuronal activity is a fundamental feature of all known neuronal networks and has been well-documented in such various systems as the cerebral cortex, hippocampus, retina, and spinal cord, both in vitro and in vivo [1–3]. In dissociated primary cortical cultures, as with other neuronal networks, the development of spontaneous electrical activity undergoes a global increase in complexity over time, with neurons exhibiting network-wide activity in characteristic spatiotemporal patterns [4, 5]. Indeed, a prominent feature of network maturation in vitro is the development of spontaneous synchronized bursts of activity across the network, which strongly correlate with synaptic density/strength [6, 7]. There is great interest in understanding the formation and maturation of neuronal activity due to the essential role this activity has in developmental processes including neurogenesis, migration, programmed cell death, cellular differentiation, and synaptic plasticity [3]. Moreover, spontaneous synchronized burst activity has been presented as a functional hallmark of developing neocortical networks [3]. In vitro cultures of isolated primary cortical neurons represent a valuable biological system for neurobiologists to study the development of neuronal networks, their activity, and the role this activity has in functional maturation of cortical circuits [8]. Indeed, cortical neurons within culture self-organize to form spontaneously active networks generating action potentials and intracellular calcium transients that resemble many basic properties of spontaneous activity observed in the immature neocortex in vivo [3].
Allied to the need for relevant biological systems to study neuronal network development is the demand for suitable electrophysiological techniques to study neuronal activity. The gold standard for in vitro electrophysiological studies is patch clamping [9]. Multiple patch clamping recording configurations (whole-cell, perforated-patch, cell-attached, outside-out) are available enabling high temporal resolution recordings from single neurons down to single ion channels. The high sensitivity of patch clamping allows the assessment of passive electrophysiological properties such as membrane potential, input resistance, and capacitance, as well as the study of spontaneous action potentials or current-induced depolarizations [10]. Unfortunately patch clamping is experimentally challenging and does not allow population level studies to characterize important hallmarks of developing neuronal networks, namely the establishment of network wide synchronous firing [11]. Electrophysiological techniques founded on the use of microelectrode arrays (MEAs) that enable parallel and multi-site extracellular recordings of cell activity are now widely used to study the coordinated activity of neuronal networks on a global level [12]. Typically, MEAs consist of regularly spaced transducers in a planar array enabling sampling of the electrical signals from many cells simultaneously, thus lacking single cell resolution. This approach allows long-term recordings of extracellular field potentials with spatial resolution determined by the density and spacing of transducers [13] and the density of the neuronal culture [14].
Alternative approaches are founded on the use of fluorescent reporters that enable subcellular spatial resolution of neuronal activity simultaneously across whole networks at a population level [15]. Moreover, fluorescent microscopy techniques coupled with fluorescent probes, are capable of recording changes in voltage and specific ion flux across cell membranes. To this end, fluorescent reporters of changes in intracellular calcium concentrations associated with action potentials are widely used [16, 17]. Indeed, calcium is an important second messenger, and following a burst of action potentials, depolarization of the neuron opens different voltage-gated ion channels, including voltage-gated calcium channels, resulting in a rapid and transient calcium influx that can also trigger Ca2+ release from intracellular stores resulting in a transient increase of cytoplasmic calcium [1, 6, 17, 18]. Advantageously, fluorescent studies of calcium transients enable the investigation of global neuronal network dynamics (e.g., analysis of network ensembles, network synchronicity) as well as single cell kinetics (e.g., frequency and amplitude of calcium events). A shortcoming of such fluorescent methods however is the rapid photobleaching that can occur in the case of organic fluorophores, cytotoxicity of probes, and limited sensitivity of probes to changes in cell electrophysiology.
An emerging technology with promise to enable the activity of individual and networks of neuronal cells to be studied with high sensitivity and unprecedented spatiotemporal resolution is centered on exploitation of the spin and charge dependent optical properties of an atomic defect within diamond, namely the Nitrogen Vacancy (NV) center [19–21]. The NV center has attracted attention as a potential fluorescent probe for use in biological imaging due to its high quantum yield, robust luminescence, which does not bleach or blink under normal conditions, and the inherent low cytotoxicity of diamond [22, 23]. There is growing interest in exploiting the quantum sensing capabilities of the NV center to probe biological systems with unprecedented sensitivity [24–28]. To this end significant effort is being directed at measurement schemes for spatiotemporal mapping of live cell activity at a network level with nanoscale resolution. In particular, several theoretical studies have numerically simulated the time dependent transmembrane potential of a morphologically reconstructed hippocampal CA1 pyramidal neuron [29, 30]. Predictions confirmed the feasibility of imaging planar neuron activity non-invasively at millisecond temporal resolution and micron spatial resolution over wide-fields using NV based magnetic field sensing protocols [29]. On a smaller scale the quantum dynamics of a NV probe in proximity to a model system consisting of an ion channel in a lipid bilayer were investigated theoretically. This study focused on the quantum decoherence of the NV probe caused by ion channel operation and concluded the NV center is sufficiently sensitive to the flow of ions through the channel to allow real-time detection [31]. Landmark experimental studies demonstrated single-neuron axon potential magnetic sensing exterior to live invertebrate giant axons [26]. Here exogenous signals were injected down the giant axons, which acted effectively as a current carrying wire, and the resulting magnetic field detected using NV magnetometry. These experimental findings were subsequently used in a theoretical study based on a forward modeling scheme to determine the feasibility of NV based sensing protocols to track extracellular electric potentials from transmembrane currents and the extracellular magnetic field from intracellular axial currents in cells [30]. This methodology demonstrated the feasibility of precise measurement of action potential waveforms from individual giant axons, as well as correlations between the magnetic field generated and the action potential conduction velocity and propagation direction. In addition to magnetic field sensing protocols studies have also demonstrated voltage induced switching of the charge state of the NV center between the negative and neutral states, which owing to the different emission spectra of these charge states led to modulation of fluorescence [32]. Significantly this study detected clear fluorescence modulation with voltage changes of the order of those known to exist in functional neuronal cultures opening up an alternative NV sensing method to monitor neuronal network activity. Combined, the theoretical and experimental studies reported to date demonstrate the feasibility of NV based quantum sensing protocols to study neuronal network activity that exploit the known dependence of NV center emission on quantum decoherence, local electromagnetic noise, and charge state.
Fundamental to the realization of all NV sensing schemes as approaches for studying network activity this is the establishment of viable strategies for the integration of NV diamond sensors within cell culture protocols that support the formation of active neuronal networks. Moreover, it is critical that future studies of well-characterized biological systems are performed, in particular of endogenous signaling within widely used experimental systems in neuronal studies [29]. Herein, such strategies are demonstrated within cultures of highly interconnected neuronal networks of murine primary cortical neurons [17]. Significantly, this work demonstrates for the first time to the authors' knowledge NV sensing protocols within cultures of highly interconnected neuronal networks, which generate spontaneous and synchronized waves of endogenous neuronal activity. The work presents two culture formats to support either extracellular studies of cells cultured directly on a planar diamond plate or intracellular studies via endocytosis mediated integration of nanoscale diamond particles within cells. NV sensing protocols based on the acquisition of optically detected magnetic resonance (ODMR) spectra and microwave modulation of photoluminescence were deployed in a widefield format. Time lapse recordings of NV photoluminescence was performed across cultures of live and dead neuronal cells alongside spatially correlated fluorescent detection of calcium activity. It is envisaged that the protocols reported here, in combination with knowledge from the existing body of literature will facilitate the implementation of NV diamond sensors for widefield, high resolution spatiotemporal mapping of endogenous neuronal activity within biologically relevant conditions.
Materials and Methods
Mouse Primary Cortical Cultures
All animal studies were conducted in compliance with the ethics and animal welfare in place in the University of Nottingham, in accordance to the 1986 Animals (Scientific Procedures) act.
C57/BL6 mouse embryos at E16–E17 developmental stage were culled and their brains removed. The brain cortices were dissected and the meninges separated under a dissection microscope. The tissue was further incubated in Hanks Balanced Salt Solution (HBSS, Ca2+ and Mg2+-free; Gibco) with 1 mg/ml trypsin and 5 mg/ml DNAseI (both Sigma) at 37°C/5% CO2 for 30 min. Following treatment with 0.05% (v/v) trypsin inhibitor (Life Technologies), the tissue was washed in Neurobasal media (Gibco) and 5 mg/ml DNAseI was added before mechanical dissociation of the tissue. The cells were finally washed in Neurobasal media and spun down at 250 × g for 5 min. Dissociated cells were further resuspended in Neurobasal media supplemented with 1x GlutaMax and 2% (v/v) B-27 (both Gibco) and seeded in 35 mm μ-Dishes high (Ibidi) with or without diamond plate at 1.25 × 105/cm2. Neuronal cortical networks were allowed to develop for 14 days before measurements were performed. Following measurement the live cultures of cells were fixed in a solution of 4% paraformaldehyde for 30 min followed by washing in phosphate buffered saline (PBS). The samples were then stored in PBS overnight at 40°C, after which experiments previously performed on live cultures, were repeated for the dead cortical network.
Loading of Fluorescent Calcium Indicator and Measurement of Calcium Transients
Fluo-5 AM (Molecular Probes) was used to monitor intracellular calcium transients in cortical neuronal networks at DIV14–15 in culture. Fluo-5 AM was mixed to equal volume of 20% Pluronic F-127 (Molecular Probes) to help disperse the non-polar ester in imaging buffer (135 mM NaCl, 3 mM KCl, 10 mM HEPES, 15 mM glucose, 1 mM MgSO4, 2 mM CaCl2, pH 7.4). Primary cultures were incubated with the 1 μM Fluo-5 AM in imaging buffer for 30 min at room temperature for dye loading and next incubated with imaging buffer for 30 min at room temperature for de-esterification of intracellular AM esters. Spontaneous neuronal activity was recorded for up to 250 s by acquiring images of the fluorescent emission from the Fluo-5AM probe using an inverted fluorescence microscope (Olympus IX83) and a sCMOS camera (Photometrics 95 Prime B).
Immunofluorescence
Cortical neurons cultured on diamond plates or containing nanodiamonds were fixed in 4% (w/v) paraformaldehyde (3.6% sucrose (w/v), 1x PBS, 5 mM MgCl2, pH 7.4; ThermoFisher) for 30 min at room temperature, washed with 10 mM Glycine in PBS and further permeabilised in PBS/Glycine-Triton (1x PBS, 10 mM glycine, 0.2% (v/v) Triton X-100; Sigma) for 20 min. The cultures were then blocked for 1 h at room temperature with 3% (w/v) BSA in PBS (Sigma), followed by incubation with anti-Gfap (1:100; Abcam) and anti-acetylated tubulin (1:300; clone 611B-1, Sigma-Aldrich) in blocking buffer at 4°C/overnight. After PBS-Triton 0.1% (v/v) washes, secondary antibodies Alexa Fluor 488 and 568 (1:300; Molecular Probes) were incubated where appropriate for 1 h.
Diamond Samples
Two formats of diamond were used in this work, namely diamond plates and nanodiamonds. The diamond plates used were composed of electronic grade single-crystal grown by chemical vapor deposition. The dimension of the plates was 7.0 × 7.0 × 0.08 mm. The crystallographic orientation of the widest surface of the diamond plate was (100)-oriented and polished to a smoothness of < 0.5 nm Ra (Delaware Diamond knives). The diamond plates were implanted with 14N+ ions at an energy of 4 keV with a dose of 1013 ions/cm2 (Innovian corp). Concentration profiles of the implanted 14N+ ions were determined with the Monte Carlo simulation code Stopping and Range of Ions in Matter (SRIM) [http://www.srim.org] with a projected depth of 14.7 nm and straggle of 5.6 nm. Following implantation the samples were annealed under Nitrogen gas according to methods reported by Chu et al. [33]. Prior to seeding with neuronal cells, the plates were cleaned by keeping them under acid reflux for 2 h using a 1:1 mixture of concentrated sulfuric and nitric acid. This was followed by surface oxidation under air for 10 h at 465°C and another step of refluxing in acid mixture for 2 h. The diamond plates were then rinsed with sterile deionized water and adhered to 35 mm μ-Dishes (Ibidi) using Norland optical adhesive. For experiments involving NDs commercially available red fluorescent NDs of nominal diameter 100 nm and containing over 1,000 NV centers each (brFND-100; FND Biotech, Taiwan) were added to cultures at a concentration of 30 μg/ml 24 h prior to NV sensing to allow cell-mediated endocytosis of the NDs.
NV Based Sensing
The instrumentation used for acquisition of ODMR spectra and microwave modulated photoluminescence from NV centers for both diamond formats is depicted in Figure 1. In both cases photoluminescence from NV centers within close proximity to live cultures of cortical neuronal cells was imaged in a wide-field format on an inverted fluorescence microscope (Olympus IX83). Microwaves (MWs) were delivered to the sample via a 2 cm diameter copper loop antenna coated in flexible silicone mounted on a micrometer translational stage and placed in contact with the cell media in the culture dish. The antenna was fed with MWs generated using a Keysight N2581B vector signal generator and amplified using an AR 20S1G4 MW amplifier. The signal generator power and the MW amplifier gain were 5 dBm and 30%, respectively. The samples were illuminated with an attenuated 500 mW, 528 nm fiber coupled multimode laser (LDi, 89 North), which effectively eliminated diffraction artifacts commonly encountered with single mode excitation sources. The excitation light was focused on to the back focal plane of a high Numerical Aperture (NA) objective lens (1.49 NA), and the emitted photoluminescence filtered through a 650 nm long pass filter and imaged onto a sCMOS camera (Photometrics 95 Prime B). Image acquisition was synchronized with MW protocols using an Olympus Real-Time Controller via the Olympus CellSens software (Tokyo, Japan).
The sensing protocols used are depicted in Figure 2. In the case of cell cultures containing nanodiamonds, Figure 2A, ODMR spectra were acquired in the absence of an externally applied magnetic field owing to the unknown orientation of NVs within the nanodiamonds. MW modulated photoluminescence was also recorded under continuous wave (CW) optical illumination. The protocol, depicted in Figure 2Aiii, involved time course recordings of photoluminescence with MWs applied followed by recordings without MWs. In the case of the diamond plates, Figure 2B, the crystallographic orientation of the NV centers within the plate was known and here NV sensing was performed in the presence of a cylindrical neodyninium magnetic (e-magnetsuk) mounted on a three axis micrometer translational stage. Application of such a bias external magnetic field results in line splitting within the ODMR spectra as depicted in Figure 2Bii. Using this experimental arrangement time course recordings of NV center photoluminescence was recorded with and without microwaves as illustrated in Figure 2Biii. The resulting images for both diamond formats were analyzed alongside the corresponding calcium recordings for the same fields of view. Regions of interest within the calcium reporter images identified to contain functioning neuronal cells were spatially correlated with images of NV photoluminescence. The mean pixel intensity associated with NV photoluminescence within each ROI was calculated as a function of MW frequency (2.77–2.97 GHz), to generate ODMR spectra, and over time with MWs on (2.93 GHz with bias magnet, 2.87 GHz without bias magnet) and MWs off.
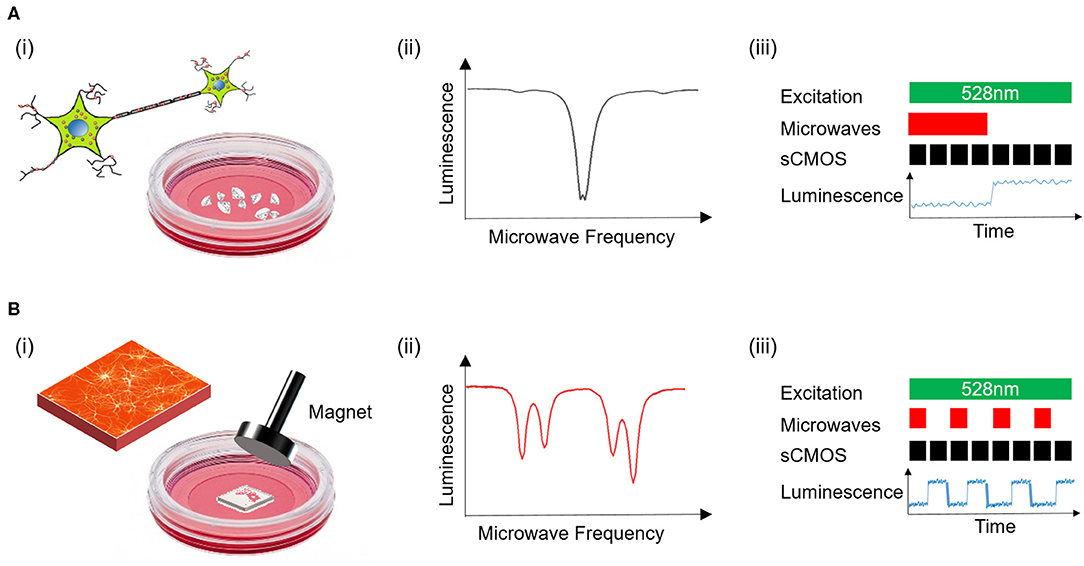
Figure 2. Depiction of experimental measurement protocols for nanodiamonds (A) and the diamond plate (B). Experimental studies of nanodiamonds were performed in the absence of an externally applied bias magnetic field (Ai), producing an ODMR spectrum with one minimum (Aii). The measurement protocol for detection of magnetically modulated photoluminescence from nanodiamonds is also shown (Aiii). An externally applied magnetic field was used in diamond plate sensing protocols (Bi), producing ODMR spectra with multiple minima (Bii). The protocol for MW modulation of photoluminescence in the case of the diamond plate is also depicted (Biii).
Results and Discussion
Figure 3 depicts the methodology used for in vitro culture of isolated cortical neurons with nanodiamonds (Figure 3Ai) and on a diamond plate (Figure 3Bi). Figures 3Aii,Bii display fixed cell immunofluorescence images of cells after 14 days of culture for the case of nanodiamonds and a diamond plate, respectively. Both images highlight the nucleus within cells, depicted as blue, and the tubulin, depicted as green. The co-localization of nanodiamond with cells is also evident in Figure 3Aii, as displayed in the red channel. Live images of cells obtained using differential interference contrast (DIC) microscopy are also shown for the nanodiamond (Figure 3Aiii) and diamond plate protocols (Figure 3Biii) which display the morphology of cells and network structure consistent with that expected of cortical neuronal networks after 14 days of culture.
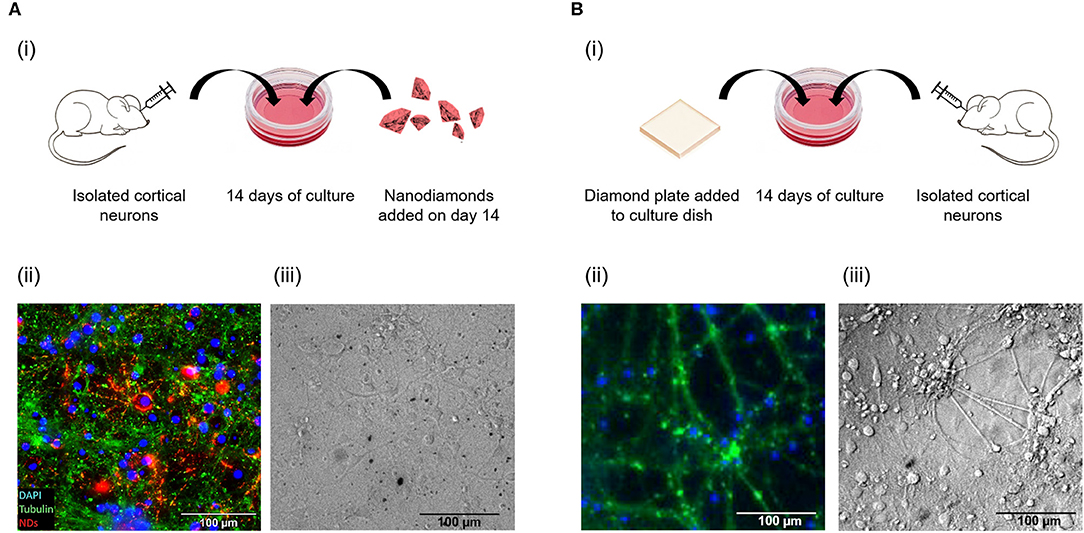
Figure 3. Schematic of culture protocol with nanodiamonds (Ai), and the diamond plate (Bi). Immunofluorescence images are also shown of cells containing nanodiamonds added at day 14 (Aii) and cells after 14 days of culture on a diamond plate (Bii). In addition differential interference contrast (DIC) of live cells containing nanodiamonds (Aiii), and live cells on the diamond plate (Biii), are shown.
To further demonstrate the functional viability of neuronal cultures, Fluo5-AM loading was used to evaluate calcium transients in cells cultured using the nanodiamond (Figure 4A) and diamond plate (Figure 4B) protocols. Video recordings of the time course expression of Fluo5-AM were made (Supplementary Videos 1, 2) with exemplar image frames shown in Figures 4Ai,Bi. The spatial expression of the fluorescence signal within these cultures is depicted in the green channel within these images. Time traces of Fluo-5AM expression extracted from multiple ROIs within the field of view with temporally varying expression of calcium activity are also shown, recorded over 200 s and 5 s time periods. The corresponding frequency domain results of data obtained for 5 s is also shown Figures 4Aiv,Biv. For both diamond formats there is a dominant frequency of 0.5 Hz suggesting cultures integrated with diamond plates and nanodiamonds both support similar rates of activity consummate with slow rhythms seen in dissociated neuronal cultures [16]. Collectively these results indicate spontaneous and co-ordinated activity is occurring across the neuronal networks. These results support the conjecture that the culture protocols presented herein allowed the establishment of a functional network with spontaneously signaling neurons within proximity of NV based diamond sensors.
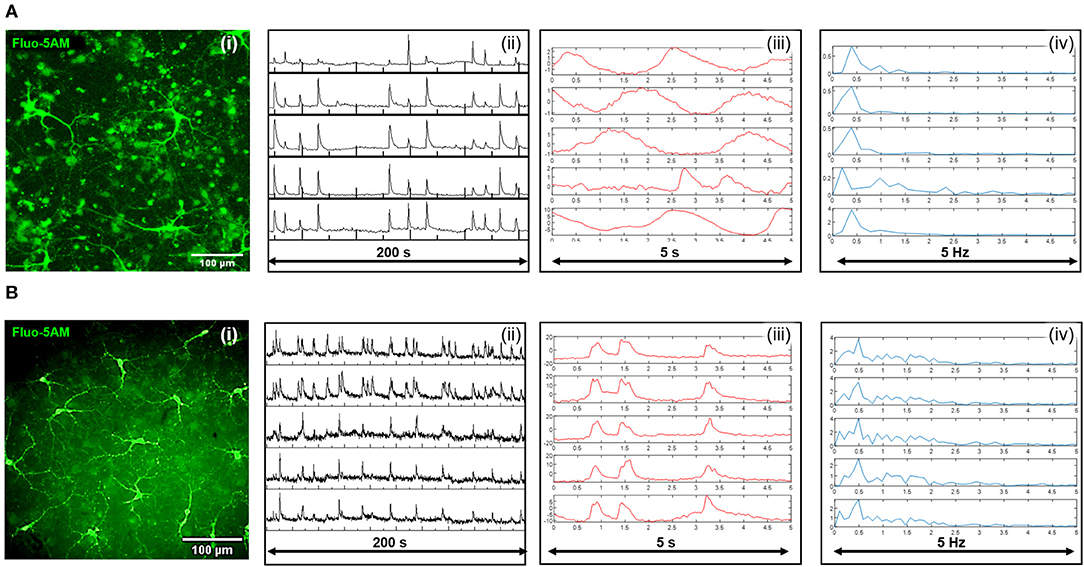
Figure 4. Widefield fluorescent images of the calcium reporter Fluo-5AM are shown for the case of nanodiamonds (Ai), and the diamond plate (Bi). Time course traces of mean pixel intensity extracted from temporal recording of Fluo-5AM from five separate regions of interest are shown over a 200 s period for nanodiamonds (Aii) and the diamond plate (Bii). Time recordings sampled at a higher temporal frequency for a 5 s time period are also shown for the nanodiamond (Aiii), and diamond plate (Biii), formats along with their corresponding frequency domain signals, respectively (Aiv) and (Biv).
Figure 5 presents results from applying NV sensing protocols, as depicted in Figure 2A, to live cultures of neuronal networks containing nanodiamonds. ODMR spectra from ROIs within the culture that are spatially correlated with ROIs identified via calcium imaging to contain active cells are displayed in Figure 5A. The results are typical of ODMR spectra acquired in the absence of externally applied bias magnetic fields with the minor splitting in the spectrum attributed to diamond lattice strain. Time course recordings of photoluminescence from the same ROIs were also taken with (red, solid line) and without (blue, dashed line) MWs. Figure 5B displays the time varying change in photoluminescence relative to the minimum intensity within each time series. A moving average of window size three was applied to the data to reduce signal noise. In all ROIs selected the photoluminescence in the absence of microwaves is higher than during the application of microwaves close to the resonant frequency as expected based on the electronic structure of the NV− defect. The time traces appear to contain periodic signals that maybe related to transient changes in the electrical and magnetic environment in proximity to the nanodiamonds associated with network activity. Potential mechanisms producing the observed modulation in photoluminescence include charge state switching of the NV centers and spin noise associated with net changes in ion flux from clusters of cells.
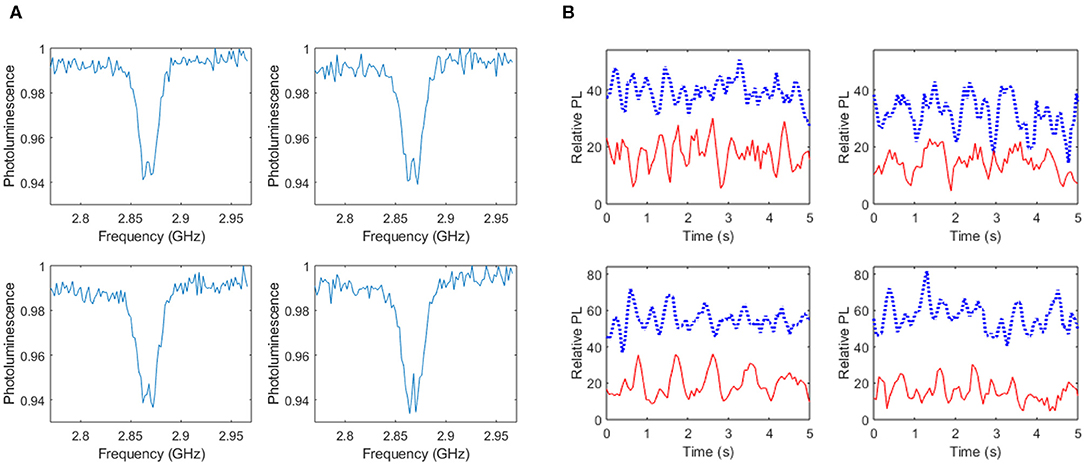
Figure 5. Results of nanodiamond based NV sensing within live cultures of cells. ROIs within the calcium dye image field of view indicating active cells were spatially correlated with ROIs within an identical field of view of images based on NV photoluminescence. ODMR spectra from four ROIs is shown in (A) in addition to temporal recordings of photoluminescence relative to the minimum intensity within each time series (B) for the case of MWs off (blue, dashed line) and MWs on (red, solid line).
Figure 6 presents results from NV based measurements for live and dead cells in the case of culture on the planar diamond plate using measurement protocols depicted in Figure 2B. In the case of live cell studies ROIs spatially correlated with active cells as indicated by calcium imaging were analyzed and ROIs from images of dead cells were chosen that contained cells morphologically indicative of neuronal cells. Figures 6A,B show ODMR spectra from separate ROIs within cultures of live and dead cells, respectively. The spectra are indicative of those obtained in the presence of an external bias magnetic field and it should be noted that asymmetries within spectra from live cells are a result of suboptimal bias field alignment with respect to the NV axis. Time course recordings of photoluminescence obtained with MWs consecutively on and off were analyzed for each ROI with a moving average of window size five applied. Figure 6C presents time traces of the difference in photoluminescence with MWs off to with MWs on, relative to the photoluminescence with MWs on, for four ROIs recorded from live cells (upper traces, red) and dead cells (lower traces, blue). Of note is the larger relative change in photoluminescence and the apparent time varying change in this value recorded from the live cells, as compared to the dead cells. The origin of these differences may arise from the time varying changes in electrical and magnetic activity within the live cell cultures as compared to the static conditions within the dead cell samples. However, regardless of the origin of these observations they warrant future investigation.
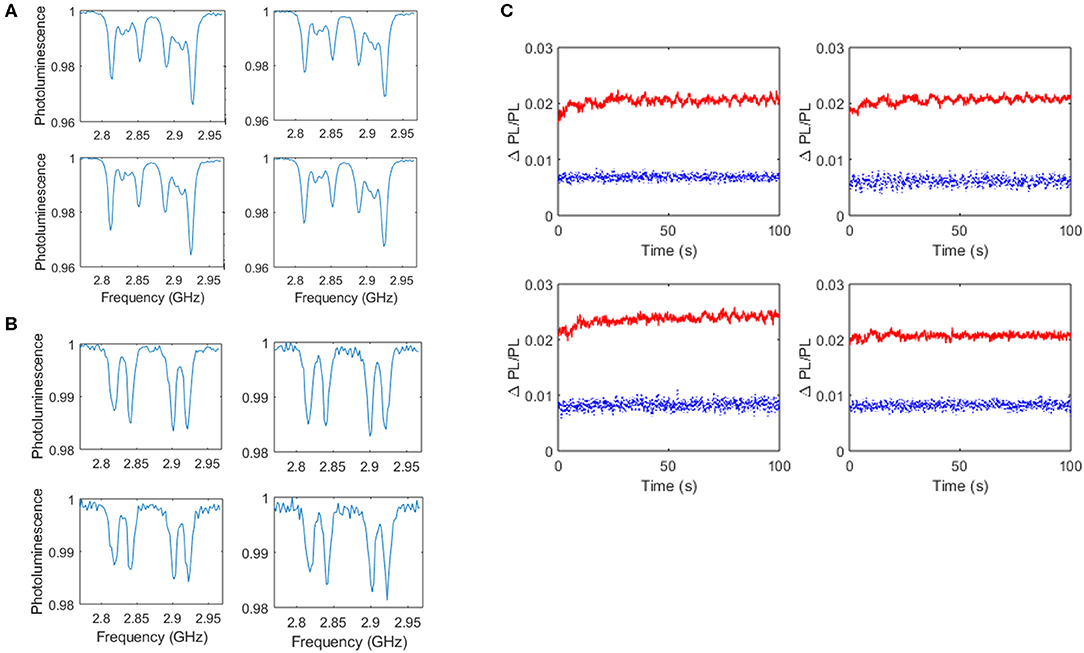
Figure 6. Results of diamond plate based NV sensing from live and dead cultures of neuronal networks. ODMR spectra of live cells from four ROIs spatially correlated with active cells as reported by Fluo-5AM are shown (A) in addition to ODMR spectra obtained from dead cells (B). Time traces of the difference in photoluminescence with MWs off to MWs on, relative to the photoluminescence with MWs on, for four ROIs recorded from live cells (upper traces, red, solid line) and dead cells (lower traces, blue, dashed line) are also shown (C).
Conclusion
In this paper, a methodology for integration of NV diamond sensors within a spontaneously active live network of cortical neurons was for the first time to the authors' knowledge presented. The strategies outlined will be fundamental to the realization of NV sensing schemes for studying cell function, in particular the spontaneous and synchronized endogenous electrical activity from networks of live neuronal cells. Here, NV based diamond sensors in two formats were deployed, namely nanodiamonds and diamond plates. In both cases calcium activity, as indicated by the fluorescent dye Fluo-5AM, was used as a reporter of network signaling in a widefield format. ROIs within cultures of live cells identified by calcium activity were spatially correlated with ROIs within images formed by recording photoluminescence from NV centers. Photoluminescence recorded within these ROIs as a function of MW frequency was analyzed to produce ODMR spectra in addition to acquisition of time course recordings of photoluminescence with and without near resonant MWs. Differences in photoluminescence modulation amplitude and frequency were observed with and without MWs and between live and dead cell cultures. Contributing factors to these observations may include changes in the NV center charge state and quenching of photoluminescence caused by changes in local spin noise associated with quantum decoherence of the NV probe caused by net variations in ion flux across the culture. In spite of this mechanistic uncertainty underpinning the experimental observations, this work presents a simple and fast approach to monitoring temporal changes in photoluminescence within live cultures of neuronal cells and is distinct from previously reported approaches based on magnetometry. Moreover, with instrumentation based improvements in photon collection and corresponding enhancement in signal to noise ratios and measurement speed it is envisaged that single shot detection of neuronal network activity is feasible. Overall, the work presented moves the NV field closer to the realization of NV centers within diamond as a next-generation tool suitable to address fundamental questions in neuroscience.
Data Availability Statement
The datasets generated for this study are available on request to the corresponding author.
Ethics Statement
Mice (C57/BL6) were housed, bred, and sacrificed (Schedule 1) in compliance with the Ethics and Animal Welfare in Accordance to the Animal (Scientific Procedures) Act 1986. Ethical review and approval was not required for the study in accordance with the local legislation and institutional requirements.
Author Contributions
RM-R carried out all experimental work required for culture of the cortical neural networks, calcium imaging, and immunostaining. In addition she worked alongside JP in the acquisition of optically detected magnetic resonance signals. JP initiated the work, designed the experimental protocols, carried out all optical measurements, and analyzed results. MM oversaw the work, analyzed experimental results, and led the writing of the manuscript. FD-B contributed to the experimental design and oversaw the neural network studies. All authors contributed to preparation of the manuscript.
Funding
The authors wish to acknowledge support from the European Research Council (ERC) via the ERC Consolidator Award, TransPhorm, grant number 683108 and the University of Nottingham's Technology Touching Life pump priming fund. FD-B and RM-R wish to acknowledge funding from the Wellcome Trust, grant number UNS56079.
Conflict of Interest
The authors declare that the research was conducted in the absence of any commercial or financial relationships that could be construed as a potential conflict of interest.
Acknowledgments
MM and JP wish to dedicate this publication to Dr. Simon Levett, our beloved colleague and friend, who sadly recently passed away. Simon was a fundamental member of our research team who was a constant support and source of scientific insight.
Supplementary Material
The Supplementary Material for this article can be found online at: https://www.frontiersin.org/articles/10.3389/fphy.2020.00255/full#supplementary-material
Supplementary Video 1. Time course expression of the calcium probe, Fluo-5AM, obtained from cells containing nanodiamonds.
Supplementary Video 2. Time course expression of the calcium probe, Fluo-5AM, obtained from cells cultured on the diamond plate.
References
1. Blankenship AG, Feller MB. Mechanisms underlying spontaneous patterned activity in developing neural circuits. Nat Rev Neurosci. (2010) 11:18–29. doi: 10.1038/nrn2759
2. Kirkby LA, Sack GS, Firl A, Feller MB. A role for correlated spontaneous activity in the assembly of neural circuits. Neuron. (2013) 80:1129–44. doi: 10.1016/j.neuron.2013.10.030
3. Luhmann HJ, Sinning A, Yang JW, Reyes-Puerta V, Stüttgen MC, Kirischuk S, et al. Spontaneous neuronal activity in developing neocortical networks: from single cells to large-scale interactions. Front Neural Circuits. (2016) 10:40. doi: 10.3389/fncir.2016.00040
4. Kamioka H, Maeda E, Jimbo Y, Robinson HPC, Kawana A. Spontaneous periodic synchronized bursting during formation of mature patterns of connections in cortical cultures. Neurosci Lett. (1996) 206:109–12. doi: 10.1016/S0304-3940(96)12448-4
5. Maeda E, Robinson HPC, Kawana A. The mechanisms of generation and propagation of synchronized bursting in developing networks of cortical neurons. J Neurosci. (1995) 15:6834–45. doi: 10.1523/JNEUROSCI.15-10-06834.1995
6. Opitz T, De Lima AD, Voigt T. Spontaneous development of synchronous oscillatory activity during maturation of cortical networks in vitro. J Neurophysiol. (2002) 88:2196–206. doi: 10.1152/jn.00316.2002
7. Muramoto K, Ichikawa M, Kawahara M, Kobayashi K, Kuroda Y. Frequency of synchronous oscillations of neuronal activity increases during development and is correlated to the number of synapses in cultured cortical neuron networks. Neurosci Lett. (1993) 163:163–5. doi: 10.1016/0304-3940(93)90372-R
8. Jimbo Y, Tateno T, Robinson HPC. Simultaneous induction of pathway-specific potentiation and depression in networks of cortical neurons. Biophys J. (1999) 76:670–8. doi: 10.1016/S0006-3495(99)77234-6
10. Hansen MG, Tornero D, Canals I, Ahlenius H, Kokaia Z. In vitro functional characterization of human neurons and astrocytes using calcium imaging and electrophysiology. Methods Mol Biol. (2019) 1919:73–88. doi: 10.1007/978-1-4939-9007-8_6
11. Triplett MA, Avitan L, Goodhill GJ. Emergence of spontaneous assembly activity in developing neural networks without afferent input. PLoS Comput Biol. (2018) 14:e1006421. doi: 10.1371/journal.pcbi.1006421
12. Ledo A, Lourenço CF, Laranjinha J, Gerhardt GA, Barbosa RM. Concurrent measurements of neurochemical and electrophysiological activity with microelectrode arrays: new perspectives for constant potential amperometry. Curr Opin Electrochem. (2018) 12:129–40. doi: 10.1016/j.coelec.2018.05.018
13. Obien MEJ, Deligkaris K, Bullmann T, Bakkum DJ, Frey U. Revealing neuronal function through microelectrode array recordings. Front Neurosci. (2015) 9:423. doi: 10.3389/fnins.2014.00423
14. Wagenaar DA, Pine J, Potter SM. An extremely rich repertoire of bursting patterns during the development of cortical cultures. BMC Neurosci. (2006) 7:11. doi: 10.1186/1471-2202-7-11
15. Berdondini L, Imfeld K, MacCione A, Tedesco M, Neukom S, Koudelka-Hep M, et al. Active pixel sensor array for high spatio-temporal resolution electrophysiological recordings from single cell to large scale neuronal networks. Lab Chip. (2009) 9:2644–51. doi: 10.1039/b907394a
16. Cohen E, Ivenshitz M, Amor-Baroukh V, Greenberger V, Segal M. Determinants of spontaneous activity in networks of cultured hippocampus. Brain Res. (2008) 1235:21–30. doi: 10.1016/j.brainres.2008.06.022
17. Takayama Y, Moriguchi H, Kotani K, Jimbo Y. Spontaneous calcium transients in cultured cortical networks during development. IEEE Trans Biomed Eng. (2009) 56:2949–56. doi: 10.1109/TBME.2009.2028419
18. Grienberger C, Konnerth A. Imaging calcium in neurons. Neuron. (2012) 73:862–85. doi: 10.1016/j.neuron.2012.02.011
19. Wrachtrup J, Von Borczyskowski C, Bernard J, Orritt M, Brown R. Optical detection of magnetic resonance in a single molecule. Nature. (1993) 363:244–5. doi: 10.1038/363244a0
20. Jelezko F, Wrachtrup J. Single defect centres in diamond: a review. Phys Status Solidi Appl Mater Sci. (2006) 203:3207–25. doi: 10.1002/pssa.200671403
21. Rondin L, Tetienne JP, Hingant T, Roch JF, Maletinsky P, Jacques V. Magnetometry with nitrogen-vacancy defects in diamond. Reports Prog Phys. (2014) 77:5. doi: 10.1002/chin.201442289
22. Schirhagl R, Chang K, Loretz M, Degen CL. Nitrogen-vacancy centers in diamond: nanoscale sensors for physics and biology. Annu Rev Phys Chem. (2014) 65:83–105. doi: 10.1146/annurev-physchem-040513-103659
23. Zhu Y, Li J, Li W, Zhang Y, Yang X, Chen N, et al. The biocompatibility of nanodiamonds and their application in drug delivery systems. Theranostics. (2012) 2:302–12. doi: 10.7150/thno.3627
24. Le Sage D, Arai K, Glenn DR, Devience SJ, Pham LM, Rahn-Lee L, et al. Optical magnetic imaging of living cells. Nature. (2013) 496:486–9. doi: 10.1038/nature12072
25. Steinert S, Ziem F, Hall LT, Zappe A, Schweikert M, Götz N, et al. Magnetic spin imaging under ambient conditions with sub-cellular resolution. Nat Commun. (2013) 4:1607. doi: 10.1038/ncomms2588
26. Barry JF, Turner MJ, Schloss JM, Glenn DR, Song Y, Lukin MD, et al. Optical magnetic detection of single-neuron action potentials using quantum defects in diamond. Proc Natl Acad Sci USA. (2016) 113:14133–8. doi: 10.1073/pnas.1601513113
27. Kucsko G, Maurer PC, Yao NY, Kubo M, Noh HJ, Lo PK, et al. Nanometre-scale thermometry in a living cell. Nature. (2013) 500:54–8. doi: 10.1038/nature12373
28. Simpson DA, Morrisroe E, McCoey JM, Lombard AH, Mendis DC, Treussart F, et al. Non-neurotoxic nanodiamond probes for intraneuronal temperature mapping. ACS Nano. (2017) 11:12077–86. doi: 10.1021/acsnano.7b04850
29. Hall LT, Beart GCG, Thomas EA, Simpson DA, McGuinness LP, Cole JH, et al. High spatial and temporal resolution wide-field imaging of neuron activity using quantum NV-diamond. Sci Rep. (2012) 2:401. doi: 10.1038/srep00401
30. Karadas M, Wojciechowski AM, Huck A, Dalby NO, Andersen UL, Thielscher A. Feasibility and resolution limits of opto-magnetic imaging of neural network activity in brain slices using color centers in diamond. Sci Rep. (2018) 8:4503. doi: 10.1038/s41598-018-22793-w
31. Hall LT, Hill CD, Cole JH, Städler B, Carusoc F, Mulvaneyd P, et al. Monitoring ion-channel function in real time through quantum decoherence. Proc Natl Acad Sci USA. (2010) 107:18777–82. doi: 10.1073/pnas.1002562107
32. Karaveli S, Gaathon O, Wolcott A, Sakakibara R, Shemesh OA, Peterka DS, et al. Modulation of nitrogen vacancy charge state and fluorescence in nanodiamonds using electrochemical potential. Proc Natl Acad Sci USA. (2016) 113:3938–43. doi: 10.1073/pnas.1504451113
Keywords: nitrogen vacancy, diamond, quantum sensing, neurons, neuronal activity
Citation: Price JC, Mesquita-Ribeiro R, Dajas-Bailador F and Mather ML (2020) Widefield, Spatiotemporal Mapping of Spontaneous Activity of Mouse Cultured Neuronal Networks Using Quantum Diamond Sensors. Front. Phys. 8:255. doi: 10.3389/fphy.2020.00255
Received: 06 January 2020; Accepted: 09 June 2020;
Published: 11 August 2020.
Edited by:
Huan-Cheng Chang, Academia Sinica, TaiwanReviewed by:
Georg Hildenbrand, Heidelberg University, GermanyChia-Yu (Cathy) Huang, National Central University, Taiwan
Copyright © 2020 Price, Mesquita-Ribeiro, Dajas-Bailador and Mather. This is an open-access article distributed under the terms of the Creative Commons Attribution License (CC BY). The use, distribution or reproduction in other forums is permitted, provided the original author(s) and the copyright owner(s) are credited and that the original publication in this journal is cited, in accordance with accepted academic practice. No use, distribution or reproduction is permitted which does not comply with these terms.
*Correspondence: Melissa Louise Mather, bWVsaXNzYS5tYXRoZXJAbm90dGluZ2hhbS5hYy51aw==