- 1Université Paris-Saclay, CEA, CNRS, Inserm, BioMaps, Orsay, France
- 2Division MR Physics, Center for Medical Physics and Biomedical Engineering, Medical University of Vienna, Vienna, Austria
This paper addresses the scientific and technological challenges related to the development of wireless radio frequency (RF) coils for magnetic resonance imaging (MRI) based on published literature together with the authors' interpretation and further considerations. Key requirements and possible strategies for the wireless implementation of three important subsystems, namely the MR receive signal chain, control signaling, and on-coil power supply, are presented and discussed. For RF signals of modern MRI setups (e.g., 3 T, 64 RF receive channels), with on-coil digitization and advanced methods for dynamic range (DR ≥ 16-bit) and data rate compression, still data rates > 500 Mbps will be required. For wireless high-speed MR data transmission, 60 GHz WiGig and optical wireless communication appear to be suitable strategies; however, on-coil functionality during MRI scans remains to be verified. Besides RF signals, control signals for on-coil components, e.g., active detuning, synchronization to the MR system, and B0 shimming, have to be managed. Wireless power supply becomes an important issue, especially with a large amount of additional on-coil components. Wireless power transfer systems (>10 W) seem to be an attractive solution compared to bulky MR-compatible batteries and energy harvesting with low power output. In our opinion, completely wireless RF coils will ultimately become feasible in the future by combining efficient available strategies from recent scientific advances and novel research. Besides ongoing improvement of all three subsystems, innovations are specifically required regarding wireless technologies, MR compatibility, and wireless power supply.
Introduction
Magnetic resonance imaging (MRI) has become one of the major tools in non-invasive medical diagnostics, providing a multitude of quantitative and functional information with ever-increasing performance. The constant search for improved sensitivity and specificity in MR examinations has coined the trend toward MR scanners with higher static magnetic field strength (B0) [1, 2] and radio frequency (RF) coil arrays with larger numbers of individual receive elements [3]. Today's high-end clinical MR scanners have a static magnetic field strength of 3 T (together with first clinical 7 T systems being installed currently) and feature up to 64 receive channels (128 or more in some research units), allowing for shorter examination times using parallel imaging [4, 5]. Typically, the excitation of the nuclear spins is done with a large high-power RF transmit coil—the system body coil—included in the scanner bore, while signal detection is performed with a local receive-only coil array, followed by on-coil preamplification and digitization in either the MR room or the technical cabinet, or rarely, on-coil. Coaxial cables are commonly used to transfer the received RF signal to the image reconstruction unit outside the MR scanner room and to power active electronic devices, such as preamplifiers, typically using DC current running on the coaxial cable's shield, which requires a bias-tee arrangement usually already integrated in commercial scanner hardware and thus avoids supplementary power cables. In addition, single wires carrying DC control signals are routed together with the coaxial cables, e.g., to bias PIN diodes as part of an on-coil switching circuitry. With increasing cabling complexity of modern high field scanners equipped with high-density and/or mechanically flexible receive arrays, the use of a large number of coaxial and wire cables gives rise to several challenges.
One main concern with cabling is the increased patient risk due to local heating phenomena associated to currents induced on the cable shields during RF transmission and fast switching of magnetic field gradients [6–8]. Secondly, as each receive element requires its own set of coaxial cable and wires, adjacent routing of cables may lead to cross talk and increase coupling between receive elements, causing a significant reduction of RF detection sensitivity. Since the coaxial cables are routed within the system body coil, a partial loss of transmit power may also occur, as some of the RF power is dissipated in the coil's cabling rather than in the target patient tissue. Baluns and RF traps [9, 10], conventionally used to reduce the abovementioned electromagnetic issues, make the receive coil heavy, bulky, and potentially intimidating and ill-fitting for patients. Moreover, handling of the coil becomes cumbersome and delicate in a way that the coil installation can occupy a significant fraction of the total exam time. This is of particular concern for applications requiring very long coaxial cables, such as abdominal MRI.
Consequently, the use of coaxial cables is one of the bottlenecks that have to be overcome to develop the next generation of coil arrays with improved sensitivity and less patient risk in high field MRI. Several approaches were proposed for the replacement of coaxial receive cables in MR experiments by optical fibers for analog [11–17] or digital [18–24] MR signal transmission. While the use of optical fibers avoids safety issues and reduces signal interferences, the positioning and handling of the receive coils are still limited by the length, placement, and maximum curvature of the optical fibers.
Fully wireless RF coils could lead to a safer, more cost- and time-efficient receive system for MRI and ultimately enable lightweight, flexible, or even “wearable” coil arrays (e.g., [23–26]), improving patient comfort and supporting the evolution of on-coil sensor integration.
Challenges in the development of wireless RF coils can especially be related to the harsh MR environment as all envisioned devices must be designed to be MR compatible, i.e., not ferro- or strongly para-magnetic. Additionally, all parts must function robustly in the strong static B0 field and handle coil vibrations, patient movement, bore reflections, and most importantly, gradient and RF fields present during MRI. To this end, some sensitive parts can be covered by Faraday cages. Possible current induction on the devices should be avoided with regard to patient safety, and added on-coil devices, e.g., digitization units or wireless transceivers, must appear transparent during imaging. Also, it is desirable to preserve high linearity and a low system noise figure (<1 dB [27]) even with the inclusion of wireless technologies. Especially for flexible arrays, a reduction of the total amount, size, and weight of on-coil components is crucial.
In this work, we focus on the realizability of completely wireless MR receive arrays by addressing and interrelating different aspects of the MR receive system. The aim is to outline feasible and efficient approaches toward wireless communication in MRI and prospect digital wireless RF devices, highlighting the most promising strategies as well as associated benefits and challenges.
Wireless Approaches for Different Parts of the MR Receive System
Three subsystems that have to undergo significant changes for wireless MRI were identified: the MR receive signal chain, control signaling, and on-coil power supply. Their functional blocks and respective possible physical location are depicted in Figure 1A. Different wireless transceiver positioning variants, estimated transmission distances, and angles are sketched in Figure 1B.
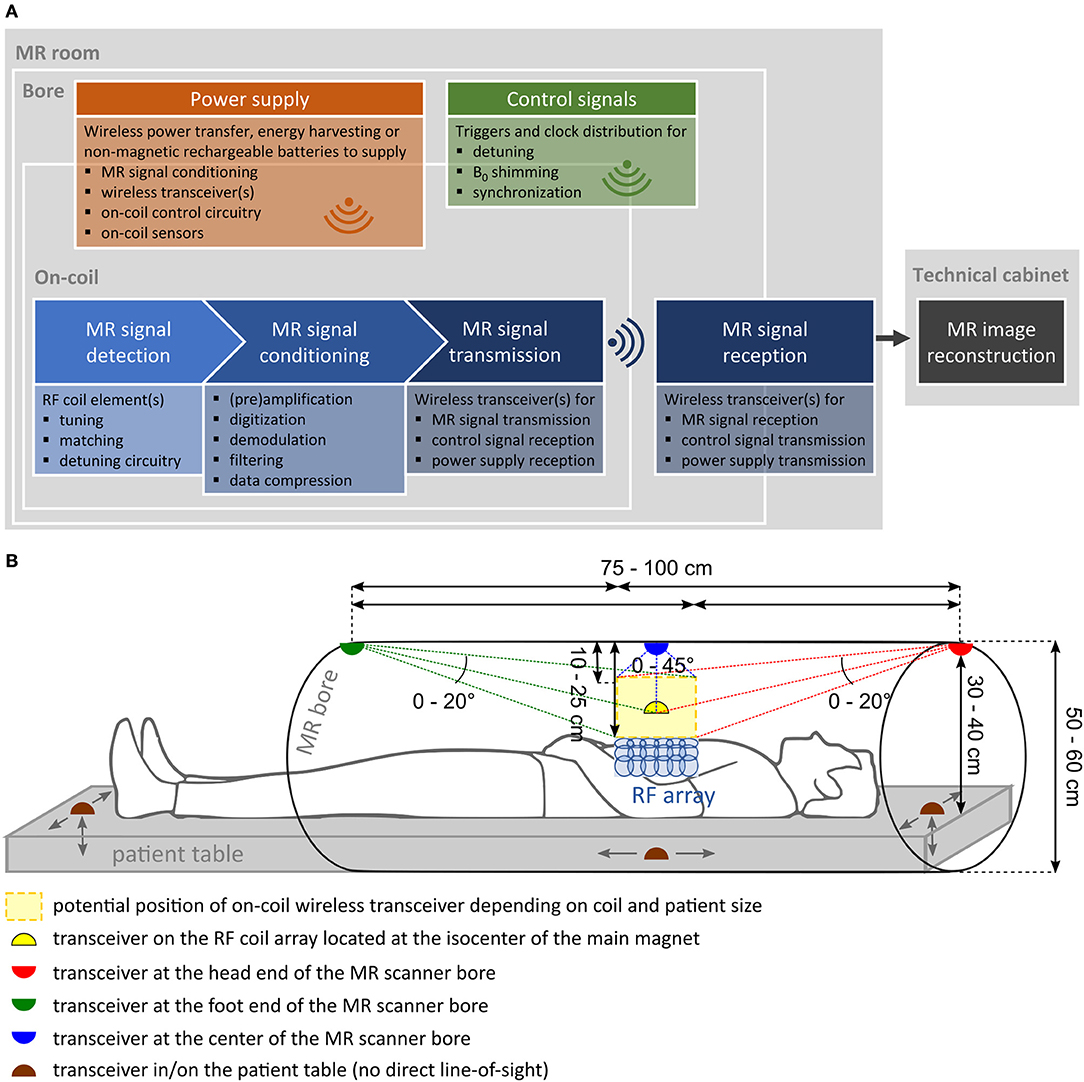
Figure 1. (A) Functional block diagram of a wireless MR receive chain consisting of three main subsystems: wireless MR signals (blue), control signals (green), and power supply (orange). (B) Side view of wireless transceiver positioning variants including transmission distance and angle estimations.
In Figure 2, the state of the art in wireless RF coil development, listing existing technologies or strategies for each respective subsystem, corresponding to sections “MR Receive Signal Chain”, “Control Signaling”, and “On-Coil Power Supply” in the manuscript, is summarized. Specific requirements that need to be met for each of the functional blocks are included, and benefits of current technology as well as current limitations or challenges encountered in their development are listed. The following general requirements apply to all of the mentioned subsystems and corresponding components: MR compatibility (no impact on MRI or component functioning), patient safety (no heating), linearity, low noise figure, low power consumption, low number of additional components, miniature component size, and minimum weight. For all wireless paths, a reliable, ideally lossless, spatial data transmission (≈10–100 cm, see Figure 1B) is required.
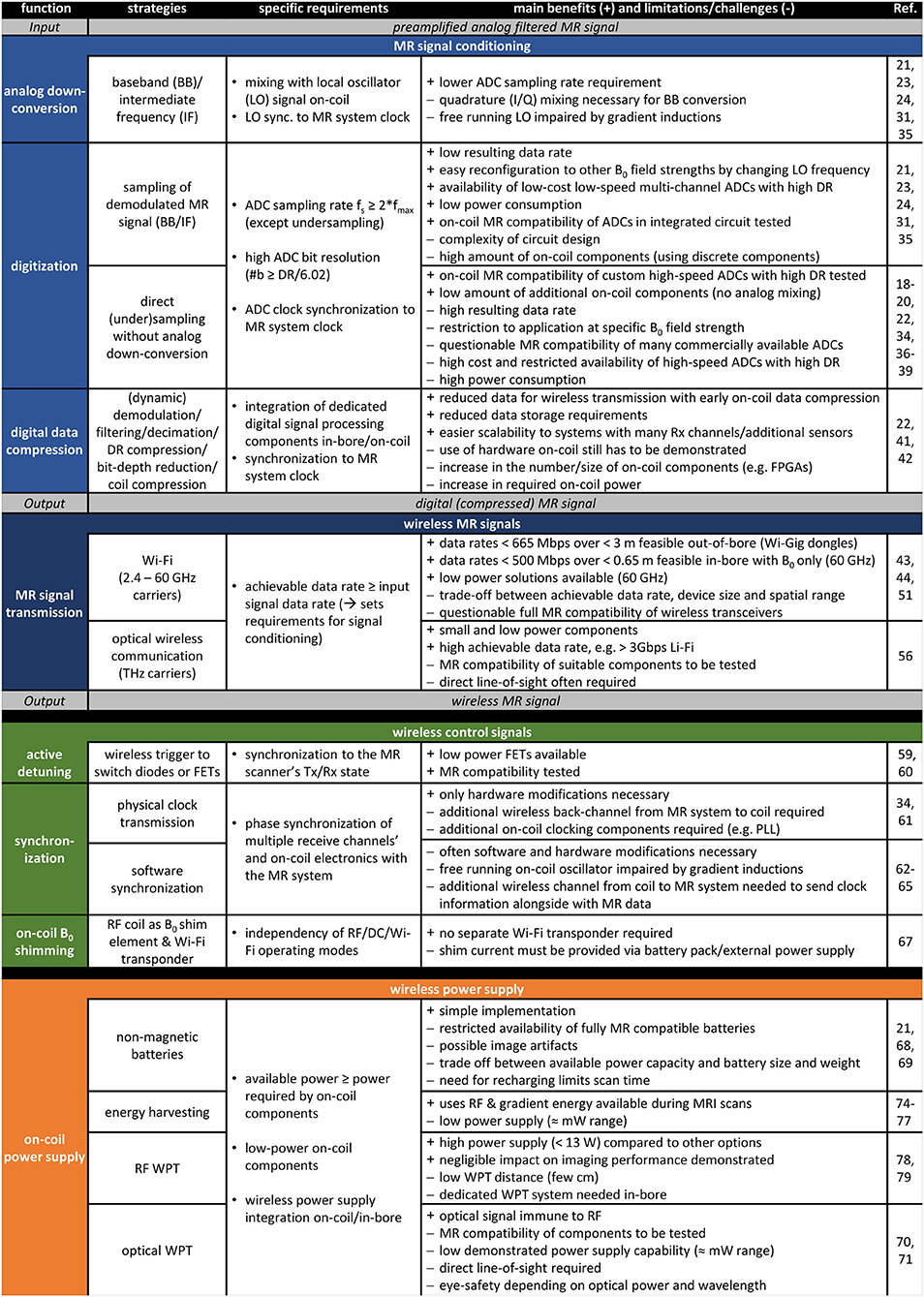
Figure 2. Summary of the state of the art in wireless radio frequency (RF) coil development. Existing technologies/strategies for each subsystem (i.e., MR receive signal chain, control signaling, and on-coil power supply) are analyzed listing specific requirements, benefits, as well as limitations or challenges encountered in their current development.
MR Receive Signal Chain
The features of the MR signal directly impact signal conditioning, which comprises (pre)amplification, digitization, analog and/or digital data compression, and filtering. The MR signal is characterized by high signal frequency (the Larmor frequency), depending on the investigated nucleus and B0 field strength, typically in the order of 50–300 MHz. Further, the DR easily reaches ~90 dB [28]. In extreme cases, especially for high-resolution 3D acquisitions at high B0 fields, the DR can attain up to ~120 dB [29, 30]. To enable proper signal conditioning for various imaging scenarios (frequency, DR, number of receive coil elements, etc.), necessary adaptations for a wireless receive chain imply the relocation of many components inside the MR bore or directly on-coil, e.g., adjustable gain amplifiers, analog-to-digital converters (ADCs), or mixers.
Signal Digitization
The choice of suitable digitization components is a critical task, as there is always a trade-off between achievable conversion rates, bit resolution, power dissipation, cost, and scalability to multi-channel systems. In general, on-coil digitization is advantageous, as it improves signal and phase stability, yielding better image quality, and offers easier scalability to multi-channel systems [18, 19, 31]. For component selection, the main challenges are related to the MR signal properties. Concerning the DR, ADCs should provide high bit resolutions (≥DR in decibels divided by 6.02 [28]) to correctly quantize analog MR signal amplitudes. To date, commercially available high-speed ADCs dedicated to MRI are limited to 16-bit [32, 33], insufficient for some imaging scenarios with very high DR. Concerning the sampling rate, one possibility is direct sampling at the Nyquist rate, employing ADCs capable of sampling at high rates greater than twice the Larmor frequency [34]. However, the essential imaging information of the MR signal lies only within a small signal bandwidth (maximum 1–2 MHz), determined by the maximum gradient strength and the field of view (FOV), modulated onto the carrier wave at the Larmor frequency. Therefore, demodulation of the amplified analog RF signal to baseband (around zero frequency) or to an intermediate frequency (IF) by mixing with a local oscillator (LO) signal on-coil before conversion to digital data is possible. This significantly lowers the ADC sampling rate requirement. Analog down-conversion is often used in traditional systems [31, 35] but can also be advantageous for easier system reconfiguration to other B0 fields and higher power efficiency (<240 mW/channel [21]). This was shown with broadband on-coil receivers for optical fiber transmission of digital signals from two [21] or four [24] wrist coil channels at 1.5–10.5 T. Direct undersampling corresponds to sampling at lower than twice the maximum frequency and digital demodulation at the same time. This technique was applied for single receive elements at 0.18 and 4.7 T [36, 37]. Multi-channel scalable solutions in combination with optical fibers were proposed for in-field receivers with one ADC per coil element at 1.5 and 3 T [18, 19], and four-channel ADCs for MRI up to 2.4 T with an eight-channel coil [38, 39]. Recent research also demonstrated a digital RF front end adaptable for 16 channels and useable from 1.5 to 11.7 T [20, 22]. Direct (under)sampling approaches are useful, as no analog conversion step is needed prior to digitization, and the amount of on-coil components is usually low. However, this technique can be demanding in terms of power consumption (>1 W/channel [20, 22]). Care has to be taken to remove signal ambiguities, e.g., by quadrature (I/Q) demodulation and digitization method-dependent signal filtering. Using I/Q demodulation, the number of components (e.g., amplifiers, ADCs, filters) after the quadrature mixer will be doubled, as there are two separate (I/Q) signal paths. Therefore, especially with discrete components, the form factor and power consumption of the receiver increase. Nevertheless, it can be advantageous to use baseband (I/Q) demodulation, e.g., in an integrated-circuit (IC) design [21, 23], to keep the resulting data rate at a minimum, which can be lower than with IF conversion or direct (under)sampling approaches.
Data Rate
Taken together, the required data rate for wireless transmission depends on the digitization approach and ADC bit resolution for any MR receive system with a specific B0 field strength, imaging bandwidth, and number of coil elements. Sequence parameters, such as the receive duty cycle (the ratio between acquisition and repetition time), also influence the effective data rate. Estimations of up to 2.6 Gbps, assuming two coil elements at 1.5 T with direct sampling (130 Msps, 20-bit, 50% receive duty cycle) or 64 coil elements at 7 T with baseband sampling of a high bandwidth signal (2 Msps, 20-bit I/Q, 50% receive duty cycle), reveal that these high resulting data rates are difficult to handle with current wireless technologies, as will be detailed in section “Wireless Transmission Technologies and Protocols”.
An evident remedy against high data rate and storage requirements is data compression, which can be realized in the analog domain by means of down-conversion before digitization as described above and/or in the digital domain, which requires dedicated signal processing units on-coil (e.g., a field-programmable gate array (FPGA) and digital frequency synthesizer [40]). Digital strategies for DR compression and coil-wise demodulation can be combined to efficiently reduce the data size to one-third of the original amount [41]. Nonetheless, with digital compression directly after digitization, the number of components and, therefore, also the power needed on-coil will increase [22, 42].
To give an estimate for the minimum data rate requirement, we take a modern clinical MRI setup at 3 T with 64 RF receive elements as a reference. In this case, a data rate of at least 512 Mbps would be desirable, assuming moderate signal bandwidth (500 ksps minimum sampling rate), average DR of around 90 dB (covered by 16-bit I/Q ADCs), 50% receive duty cycle, and baseband demodulation to keep the resulting data rate and component power consumption low. Our estimation is in line with other published values [43, 44], only differing in terms of assumed ADC bit resolution, receive duty cycle, or number of receive elements.
Wireless Transmission Technologies and Protocols
Wireless transmission setups have been investigated for their usability in MRI, testing only the wireless link with “synthetic” MR image data without RF coil or signal conditioning components. Except early work on analog wireless MR signal transmission with carriers in the low gigahertz range (<3 GHz [45–47]), research was mostly oriented toward digital wireless MR signal transceivers following IEEE Wi-Fi standards. For digital wireless communication in MRI, apart from achievable data rate and power consumption, lossless spatial transmission is an important criterion. First MR data transfer tests based on the 802.11b [48] or 802.11n [49] standards revealed that long range (>10 m) comes at the cost of low achievable data rates as well as large and power-consuming antennas. These approaches are clearly impractical for wireless MRI. More recent attempts were conducted with higher carriers in the 5 GHz band (802.11ac Wi-Fi protocol), showing reliable in-bore operation of client and router antennae during an MRI scan at data rates around 90 Mbps [44]. This Wi-Fi approach is interesting as small client routers, used in most portable devices nowadays, are available, providing sufficient spatial range for MRI. Efficient data throughput could be improved up to 350 Mbps, suitable for low-channel and low-bandwidth MRI. However, power consumption for only one transmitter antenna can exceed 1 W [50], which can be problematic with limited wireless on-coil power supply, as explained in section “On-Coil Power Supply”. Aiming for enhanced data rate capability and reduced power consumption, subsequent work focused on even higher carriers−60 GHz “WiGig” links—included in the 802.11ad Wi-Fi protocol. At 1.5 T, without the presence of RF pulses or gradients, data rates up to 500 Mbps over 10–65 cm were achieved using a miniature transceiver that can achieve up to 2.5 Gbps with only 14 mW DC power per wireless transmitter [43]. Recently, out-of-bore experiments with shielded WiGig dongles [51] have shown transmission rates of 187–665 Mbps over 3–5.5 m distance. This Wi-Fi standard meets our estimated minimum data rate requirement for a modern clinical MRI setup and is therefore viable for wireless coil arrays. Also, the shorter spatial transmission range of one of the presented 60 GHz links [43] is sufficient for some transceiver positioning variants (see Figure 1B).
Optical wireless communication (OWC) [52, 53] with visible, infrared, or ultraviolet light carriers (i.e., several 100 THz) could be an attractive alternative to Wi-Fi with distinct benefits [54]: large license-free bandwidth, small and low-power components, immunity to electromagnetic interference, and the possibility for integration into available illumination infrastructure; moreover, OWC can operate well below light intensities considered dangerous for the human eye. Data rates over 3 Gb/s in visible light communication have been shown using a single LED [55]. An MR-compatible OWC front end has been tested for 2 m analog positron emission tomography detector signal transmission [56], but the technology has not yet been exploited for MR signals. Unlike Wi-Fi, high-speed OWC mostly requires a direct line of sight between transceivers, although some systems can even communicate via diffuse light reflections [57]. Suitable components for Li-Fi (Light-Fidelity, i.e., high-speed optical wireless networking [58]) in MRI still remain to be identified and tested on-coil in future studies.
Authors' Opinion on a Wireless MR Receive Signal Chain
Wireless digital MR signal transmission appears feasible with current Wi-Fi strategies under the condition that appropriate measures for data rate reduction prior to wireless transmission are implemented on-coil, e.g., analog baseband demodulation, if possible even combined with further digital data compression methods. Wi-Fi protocol-dependent or component-related drawbacks, e.g., the trade-off between achievable data rate, spatial transmission range, and required power as well as questionable full MR compatibility, restrict the usability of today's Wi-Fi technologies. WiGig (60 GHz) seems to be a promising strategy because of high data rate capability, sufficient transmission range, and low power consumption, although full functioning of WiGig hardware on-coil during an MR scan and the effect on image quality still have to be examined. Also, the final interfacing of the chosen wireless (WiGig) transceiver to a digital RF coil still has to be demonstrated and can be challenging, as it requires the smooth interaction of various on-coil components. So far, Wi-Fi technology benefited from rapid development pushed by the portable device industry; therefore, we think that the implementation of future high-performance Wi-Fi transceivers in RF coils is an aspect to be followed up by the research community. Alternatively, OWC strategies could be investigated for wireless MR signal transmission. With OWC, the wireless transmission of uncompressed, directly digitized MR signals could be envisioned, which is advantageous with respect to miniaturized device size and low system complexity but is questionable concerning a limited on-coil power budget.
Control Signaling
Striving for full removal of coil cabling, a bidirectional wireless link is indispensable as signals must be sent not only from the coil to the MR scanner but also from the scanner control unit to the coil, mainly for triggering, synchronization, and in some cases, control of B0 shimming.
Active Detuning
Trigger signals need to be distributed to the coil electronics, e.g., to bias PIN diodes for detuning receive coils during RF transmission. Wireless detuning triggers transmitted via a 418 MHz antenna during an MRI scan at 1.5 T have been investigated [59], involving power-efficient replacement of PIN diodes by field-effect transistors (FETs) [60]. Presumably, these trigger signals could also be applied to activate power-consuming components (preamplifiers, ADCs) only during signal reception.
Synchronization
A stable clock, phase-synchronous with the MRI, controlling on-coil electronics (such as ADC or down-conversion), is critical. Clock jitter, which decreases the effective number of ADC bits and creates image artifacts, must be limited. For synchronization of MR unit and in-bore receivers, one method is to physically transmit the MRI master clock to the receiver, which has been demonstrated with 1.6, 2.4, and 3.5 GHz carriers [34, 61]. This requires additional on-coil clocking electronics (e.g., a phase-locked loop, PLL) and a wireless back channel from the MR unit to the coil. In contrast, on-coil clock generators can be used but are particularly impaired by gradient induction; therefore, free-running oscillator information has to be sent to the MR system alongside sampled data to detect and correct for frequency and phase errors as well as time offsets, i.e., to synchronize the two clocks by software. This often requires both additional hardware and software in the wireless receive system [62–65].
On-Coil B0 Shimming
Several MRI applications benefit from localized on-coil B0 shimming with DC currents on the RF coil elements compensating for B0 inhomogeneities [66]. High shim currents themselves cannot be wirelessly transmitted but can be wirelessly controlled, which has been successfully demonstrated by 2.4 GHz Wi-Fi communication [67], using the RF coil itself as a wireless transponder.
Authors' Opinion on Wireless Control Signaling
Overall, less stringent requirements concerning data rate and DR apply to wireless control signals, but correct timing and reliable, simultaneous operation to other wireless paths, especially the MR signal transmission, play a crucial role. Wireless control of active detuning and on-coil B0 shimming circuits is feasible with existing technologies and has been implemented during an MRI scan in combination with a wired or battery power supply and MR signal transmission via coaxial cables. Solutions for the synchronization of LO signals or ADC sampling clocks to the MR system clock, crucial to avoid image artifacts and signal degradation, were presented but not demonstrated with a realistic wireless MR receive chain yet because implementation in practice seems challenging. Patient movement and coil vibrations can become an issue for synchronization, but to date, physical system clock transmission via a wireless back-channel appears to be a quite robust solution for wireless MRI. The long-term stability of the external reference clock might be combined with further clock correction in post-processing. Also, a possibility for software synchronization with a free-running oscillator might be included in any case as a fallback strategy if the physical clock transmission fails.
On-Coil Power Supply
The electric power required on-coil is of major concern for wireless RF coil development. In wired coils, generally only components for preamplification and detuning (plus B0 shimming in some applications) have to be supplied with DC power. In contrast, wireless digital MR signal transmission will add on-coil power requirements for ADCs, potential down-conversion, and wireless transceivers. In this case, the power budget can easily exceed 1–2 W per channel, especially with high-speed ADCs. Power requirements scale with the number of receive channels and depend on multiplexing strategies, i.e., if one ADC and/or wireless transceiver is used for one or multiple coil element(s). For a 64-channel coil and one direct sampling ADC per channel, the power requirement could thus exceed 100 W, which is not feasible with current wireless power supply strategies in MRI as detailed below. Therefore, the first step to implement power supply for wireless coils is to reduce power consumption. Realizable low-power solutions for digitization, detuning, and wireless transceivers have been investigated in studies cited above [21, 43, 60] and could be further improved employing passive components whenever possible, e.g., passive mixers for down-conversion. Assuming a low power consumption in the range of hundreds of milliwatts per receive channel, for arrays up to 64 channels, this still results in on-coil power requirements of tens of watts.
Batteries
The use of non-magnetic rechargeable batteries could be envisioned, although available battery power capacities are limited, and as a consequence, the need for recharging limits scan time. Li-ion batteries (e.g., 5,000 mAh, 7.2 V [21]) or, more specifically, Lithium-ion polymer batteries, e.g., used for motion sensors (250 mAh, 3.7 V, 6.5 × 18 × 25 mm3 [68, 69]), themselves are generally non-magnetic. However, care must be taken because voltage conversion circuits often include ferrite core transformers not suitable for use in MRI. Typically, an increase in power capacity means bigger battery pack size (e.g., 6,000 mAh, 3.7 V, 5.8 × 58 × 138 mm3 [69]), and it is therefore obvious that with higher channel count, battery power supply becomes cumbersome and suboptimal for use in-bore or on-coil with limited space.
Wireless Power Transfer
Optical wireless power transfer (WPT) has been suggested for recharging medical implants (<10 mW [70]) or portable devices [71] and could be used in analogy to power-over-fiber approaches previously employed in MRI [12, 72]. To satisfy the power budget for an MR receiver array, it is likely that multiple free-space lasers with high optical powers in combination with efficient photodetectors would be required, possibly resulting in solutions that—depending on optical powers and wavelengths—are not eye-safe [73] and would require sophisticated alignment mechanisms.
For MRI, WPT in the RF range and energy harvesting have been investigated as attractive alternatives. The latter converts energy from electromagnetic fields present during an MR examination, namely the transmit RF field (tens of kilowatts) and gradient fields, into DC power, using inductive coupling in resonant “harvesting” loops [74–77]. Harvesting loops rely on induction at the Larmor frequency, and thus, to avoid system interferences, the size and placement of the loops cannot be chosen freely; further, variations in harvested power depending on the imaging sequence have to be taken into account, which limits the achievable power supply (tens of milliwatts). RF WPT implies the construction of a dedicated system consisting of primary (e.g., in the patient table) and secondary (close to the receive coil) loops for the sole purpose of power delivery by inductive coupling. Byron et al. [78, 79] propose an MR-compatible WPT system operating at 10 MHz transferring up to 13 W over a few centimeters' distance in a 1.5 T system.
Authors' Opinion on Wireless On-Coil Power Supply
The analysis of existing approaches for wireless on-coil power supply leads us to the conclusion that this aspect is still a bottleneck, currently preventing completely wireless MRI. Limitations due to available on-coil power reappear in every subsystem, e.g., concerning the choice of digitization components, analog/digital compression steps, and wireless transceivers. To overcome this bottleneck, ideally, solutions should be found to reduce the total power consumption per wireless MR channel to around 200 mW, so that a 13 W RF WPT system would be sufficient to supply DC power for a 64-channel coil array. Further advances in wireless power development are also desirable to increase available on-coil power budget and therefore alleviate related restrictions. Batteries are currently the only solution for a simple implementation of on-coil power supply, but considering weight, size, and uncertain MR compatibility in some cases, this approach should not remain the only accessible strategy in the future. Out of the other existing strategies, we believe that RF WPT is currently the most sophisticated and promising wireless power supply solution for receive arrays including electronics, as it is capable of supplying a high amount of DC power with negligible impact on MRI performance. A drawback of RF WPT is that the developed system is not yet optimized for on-coil (secondary loop) or in-bore (primary loop) integration. Power transfer distance should ideally be increased and system size and complexity reduced to yield an easily reproducible and efficient WPT solution. Perhaps, another alternative DC power source in MRI might be a technology based on the magnetoelectric effect, using a piezoelectric material between magnetostrictive layers [80]. However, this technology has not been adapted for MRI conditions yet and will, as we believe, rather be suitable for power delivery in the milliwatt range, similar to existing harvesting techniques, as it is now employed for medical implant charging.
Discussion and Conclusion
In this paper, we summarized the status quo of wireless RF coil development and analyzed existing strategies for the adaptation of the three subsystems of wireless RF coils: the MR receive signal chain, control signaling, and on-coil power supply. We reviewed the benefits of current technology as well as technological challenges or limitations encountered in their development and suggest some future directives.
Over the last years, considerable progress has been made investigating wireless MR and control signal transmission. Feasible strategies exist for on-coil digitization, wireless in-bore signal transmission, cordless active detuning, synchronization to the MR system, and B0 shim control. However, regarding the numerous requirements for a complete removal of coil cabling in high-density coil arrays, there is still a need for improvement. Solutions described in this work have limitations concerning data rate capabilities and spatial transmission distance as well as power consumption and device size. In addition, full MR compatibility is often questionable. Despite required innovations, we think that future work should focus on the first demonstration of a complete bidirectional wireless MR and control signal chain. This implies the connection of an RF coil with on-coil digitization to a suitable wireless transceiver and the inclusion of wireless active detuning and synchronization circuitry on-coil (leaving out B0 shimming in a first step, reserved for some specific applications). An important aspect is to thoroughly test this assembly under realistic MRI scan conditions, i.e., with B0, RF, and gradient fields present and patient movement or coil vibrations possibly impairing component functioning, especially wireless links, and MRI performance. For a proof of concept, only a low number of RF receive elements could be targeted to circumvent high system complexity and high demands in terms of system miniaturization, required data rate, and on-coil power.
Already with low channel counts, wireless on-coil power supply seems to be the main bottleneck, currently preventing fully wireless MRI. Other than bulky rechargeable batteries, no easily accessible WPT technology exists. We believe that reduction of on-coil component power consumption will be achieved and more efficient technologies for WPT will be developed that can be more easily integrated in existing MR systems.
In conclusion, based on our investigations of the state of the art, we predict that completely wireless RF coils will be feasible in the future. Their final implementation will require the combination of already-available technologies and the investigation of alternative promising strategies. Ultimately, with innovations especially required for wireless technologies (e.g., OWC for MRI), MR-compatible components, as well as wireless power supply, efficient solutions for each of the subsystems could be assembled. The realization of wireless RF coils would lead to a significant improvement in coil usability, image quality, patient safety, and comfort.
In the future, wireless RF coils could also follow the trend of additional sensor integration, providing a multitude of complementary information during MRI, e.g., patient motion [81, 82], to further improve image quality and physiological monitoring. While wireless sensor data transmission often relaxes data rate constraints, efficient power supply and reliable data transmission still have to be ensured.
Author Contributions
RF-K initiated the work. LN, RF-K, EL, and J-CG contributed to the literature search. LN, RF-K, and EL generated the figures. All authors contributed to writing and proofreading the manuscript.
Funding
This work was funded by the Austrian/French FWF (Austrian Science Fund)/ANR grant, No. I-3618 BRACOIL, and the Austrian/French OeAD WTZ grant FR 03/2018.
Conflict of Interest
The authors declare that the research was conducted in the absence of any commercial or financial relationships that could be construed as a potential conflict of interest.
References
1. Moser E. Ultra-high-field magnetic resonance: why and when? World J Radiol. (2010) 2:37–40. doi: 10.4329/wjr.v2.i1.37
2. Moser E, Laistler E, Schmitt F, Kontaxis G. Ultra-high field NMR and MRI—the role of magnet technology to increase sensitivity and specificity. Front Phys. (2017) 5:33. doi: 10.3389/fphy.2017.00033
3. Roemer PB, Edelstein WA, Hayes CE, Souza SP, Mueller OM. The NMR phased array. Magn Reson Med. (1990) 16:192–225. doi: 10.1002/mrm.1910160203
4. Sodickson DK, Manning WJ. Simultaneous acquisition of spatial harmonics (SMASH): fast imaging with radiofrequency coil arrays. Magn Reson Med. (1997) 38:591–603. doi: 10.1002/mrm.1910380414
5. Pruessmann KP, Weiger M, Scheidegger MB, Boesiger P. SENSE: sensitivity encoding for fast MRI. Magn Reson Med. (1999) 42:952–62.
6. Konings MK, Bartels LW, Smits HFM, Bakker CJG. Heating around intravascular guidewires by resonating RF waves. J Magn Reson Imaging. (2000) 12:79–85. doi: 10.1002/1522-2586(200007)12:1<79::aid-jmri9>3.0.co;2-t
7. Armenean C, Perrin E, Armenean M, Beuf O, Pilleul F, Saint-Jalmes H. RF-induced temperature elevation along metallic wires in clinical magnetic resonance imaging: influence of diameter and length. Magn Reson Med. (2004) 52:1200–6. doi: 10.1002/mrm.20246
8. International Electrotechnical Commission (IEC). International Standards. Medical Electrical Equipment – Part 2-33: Particular Requirements for the Basic Safety and Essential Performance of Magnetic Resonance Equipment for Medical Diagnosis (IEC-60601-2-33). 3.1. Geneva (2013).
9. Peterson DM, Beck BL, Duensing GR, Fitzsimmons JR. Common mode signal rejection methods for MRI: reduction of cable shield currents for high static magnetic field systems. Concepts Magn Reson Part B Magn Reson Eng. (2003) 19:1–8. doi: 10.1002/cmr.b.10090
10. Seeber DA, Jevtic J, Menon A. Floating shield current suppression trap. Concepts Magn Reson Part B Magn Reson Eng. (2004) 21:26–31. doi: 10.1002/cmr.b.20008
11. Yuan J, Wei J, Shen GX. A 4-channel coil array interconnection by analog direct modulation optical link for 1.5-T MRI. IEEE Trans Med Imaging. (2008) 27:1432–8. doi: 10.1109/TMI.2008.922186
12. Memis OG, Eryaman Y, Aytur O, Atalar E. Miniaturized fiber-optic transmission system for MRI signals. Magn Reson Med. (2008) 59:165–73. doi: 10.1002/mrm.21462
13. Fandrey S, Weiss S, Müller J. A novel active MR probe using a miniaturized optical link for a 1.5-T MRI scanner. Magn Reson Med. (2012) 67:148–55. doi: 10.1002/mrm.23002
14. Koste GP, Nielsen MC, Tolliver TR, Frey RL, Watkins RD. Optical MR receive coil array interconnect. In: Proceedings of the International Society for Magnetic Resonance in Medicine 13. Miami Beach (2005). p. 411.
15. Biber S, Baureis P, Bollenbeck J, Höcht P, Fischer H. Analog optical transmission of 4 MRI receive channels with high dynamic range over one single optical fiber. In: Proceedings of the International Society for Magnetic Resonance in Medicine 16. Toronto (2008). p. 1120.
16. Demir T, Delabarre L, Akin B, Adriany G, Ugurbil K, Atalar E. Optical transmission system for high field systems. In: Proceedings of the International Society for Magnetic Resonance in Medicine 19. Montréal (2011). p. 1865.
17. Du C, Yuan J, Shen GX. Comparison of FP, VCSEL and DFB laser diode in optical transmission for MR RF coil array. In: Proceedings of the International Society for Magnetic Resonance in Medicine 15. Berlin (2007). p. 1041.
18. Possanzini C, Van Liere P, Roeven H, Den Boef J, Saylor C, Van Eggermond J, et al. Scalability and channel independency of the digital broadband dStream architecture. In: Proceedings of the International Society for Magnetic Resonance in Medicine 19. Montréal (2011). p. 5103.
19. Possanzini C, Harvey PR, Ham K, Hoogeveen R. The Digital Revolution in MRI With dStream Architecture. (2016) Available online at: http://clinical.netforum.healthcare.philips.com/global/Explore/White-Papers/MRI/Ingenia-dStream-architecture-the-digital-revolution-in-MRI (accessed September 2, 2019).
20. Reber J, Marjanovic J, Brunner DO, Port A, Pruessmann KP. Scalable, in-bore array receiver platform for MRI. In: Proceedings of the International Society for Magnetic Resonance in Medicine 24. Singapore (2016). p. 2170.
21. Sporrer B, Wu L, Bettini L, Vogt C, Reber J, Marjanovic J, et al. A fully integrated dual-channel on-coil CMOS receiver for array coils in 1.5–10.5 T MRI. IEEE Trans Biomed Circuits Syst. (2017) 11:1245–55. doi: 10.1109/TBCAS.2017.2764443
22. Reber J, Marjanovic J, Brunner DO, Port A, Schmid T, Dietrich BE, et al. An in-bore receiver for magnetic resonance imaging. IEEE Trans Med Imaging. (2019). doi: 10.1109/TMI.2019.2939090. [Epub ahead of print].
23. Sporrer B, Bettini L, Vogt C, Mehmann A, Reber J, Marjanovic J, et al. Integrated CMOS receiver for wearable coil arrays in MRI applications. In: Design, Automation & Test in Europe Conference & Exhibition (DATE). (2015) p. 1689–94. doi: 10.7873/DATE.2015.1152
24. Port A, Reber J, Vogt C, Marjanovic J, Sporrer B, Wu L, et al. Towards wearable MR detection: a stretchable wrist array with on-body digitization. In: Proceedings of the International Society for Magnetic Resonance in Medicine 26. Paris (2018). p. 17.
25. Frass-Kriegl R, Navarro de Lara LI, Pichler M, Sieg J, Moser E, Windischberger C, et al. Flexible 23-channel coil array for high-resolution magnetic resonance imaging at 3 Tesla. PLoS ONE. (2018) 13:e0206963. doi: 10.1371/journal.pone.0206963
26. Mehmann A, Varga M, Vogt C, Port A, Reber J, Marjanovic J, et al. On the bending and stretching of liquid metal receive coils for magnetic resonance imaging. IEEE Trans Biomed Eng. (2019) 66:1542–8. doi: 10.1109/TBME.2018.2875436
27. De Zanche N. MR receive chain. In: Proceedings of the International Society for Magnetic Resonance in Medicine 27. Montréal (2019).
28. Gabr RE, Schär M, Edelstein AD, Kraitchman DL, Bottomley PA, Edelstein WA. MRI dynamic range and its compatibility with signal transmission media. J Magn Reson. (2009) 2:137–45. doi: 10.1016/j.jmr.2009.01.037
29. Behin R, Bishop J, Henkelman RM. Dynamic range requirements for MRI. Concepts Magn Reson Part B Magn Reson Eng. (2005) 26:28–35. doi: 10.1002/cmr.b.20042
30. Yuan J, Wei J, Du C, Shen GX. Investigation of dynamic range requirement for MRI signal transmission by optical fiber link. In: Proceedings of the International Society for Magnetic Resonance in Medicine 15. Berlin (2007). p. 995.
31. Hashimoto S, Kose K, Haishi T. Comparison of analog and digital transceiver systems for MR imaging. Magn Reson Med Sci. (2014) 13:285–91. doi: 10.2463/mrms.2013-0114
32. Texas Instruments. Magnetic resonance Imaging (MRI). Available online at: http://www.ti.com/solution/mri_magnetic_resonance_imaging (accessed September 3, 2019).
33. ADI's Magnetic Resonance Imaging (MRI) Solutions. Available online at: https://www.analog.com/media/cn/technical-documentation/apm-pdf/adi-mri-solution_en.pdf (accessed September 3, 2019).
34. Sekiguchi T, Akita K, Nakanishi T, Kato S, Adachi K, Okamoto K. Development of digital wireless transceiver for a MRI coil with clock synchronization. In: Proceedings of the International Society for Magnetic Resonance in Medicine 17. Honolulu (2009). p. 3048.
35. Bollenbeck J, Vester M, Oppelt R, Kroeckel H, Schnell W. A high performance multi-channel RF receiver for magnet resonance imaging systems. In: Proceedings of the International Society for Magnetic Resonance in Medicine 13. Miami Beach (2005). p. 860.
36. Giovannetti G, Hartwig V, Viti V, Gaeta G, Francesconi R, Landini L, et al. Application of undersampling technique for the design of an NMR signals digital receiver. Concepts Magn Reson Part B Magn Reson Eng. (2006) 29:107–14. doi: 10.1002/cmr.b.20065
37. Pérez P, Santos A, Vaquero JJ. Potential use of the undersampling technique in the acquisition of nuclear magnetic resonance signals. Magn Reson Mater Phys Biol Med. (2001) 13:109–17. doi: 10.1016/S1352-8661(01)00137-5
38. Tang W, Sun H, Wang W. A digital receiver module with direct data acquisition for magnetic resonance imaging systems. Rev Sci Instrum. (2012) 83:104701. doi: 10.1063/1.4755089
39. Tang W, Wang W, Liu W, Ma Y, Tang X, Xiao L, et al. A home-built digital optical MRI console using high-speed serial links. Magn Reson Med. (2015) 74:578–88. doi: 10.1002/mrm.25403
40. De Zanche N. MRI technology: circuits and challenges for receiver coil hardware. In: Iniewski K, editor. Medical Imaging: Principles, Detectors, and Electronics. Hoboken, NJ: John Wiley & Sons, Inc. (2009). p. 285–301.
41. Jutras JD, Fallone BG, De Zanche N. Efficient multichannel coil data compression: a prospective study for distributed detection in wireless high-density arrays. Concepts Magn Reson Part B Magn Reson Eng. (2011) 39:64–77. doi: 10.1002/cmr.b.20191
42. Marjanovic J, Reber J, Brunner DO, Engel M, Kasper L, Dietrich BE, et al. A reconfigurable platform for magnetic resonance data acquisition and processing. IEEE Trans Med Imaging. (2019). doi: 10.1109/TMI.2019.2944696. [Epub ahead of print].
43. Aggarwal K, Joshi KR, Rajavi Y, Taghivand M, Pauly JM, Poon ASY, et al. A millimeter-wave digital link for wireless MRI. IEEE Trans Med Imaging. (2017) 36:574–83. doi: 10.1109/TMI.2016.2622251
44. Vassos C, Robb F, Vasanawala S, Pauly J, Scott G. Characterization of In-Bore 802.11ac Wi-Fi performance. In: Proceedings of the International Society for Magnetic Resonance in Medicine 27. Montréal (2019). p. 1543.
45. Scott G, Yu K. Wireless transponders for RF coils: systems issues. In: Proceedings of the International Society for Magnetic Resonance in Medicine 13. Miami Beach (2005). p. 330.
46. Heid O, Vester M, Cork P, Hulbert P, Huish DW. Cutting the cord - wireless coils for MRI. In: Proceedings of the International Society for Magnetic Resonance in Medicine 17. Honolulu (2009). p. 100.
47. Riffe MJ, Heilman JA, Gudino N, Griswold MA. Using on-board microprocessors to control a wireless MR receiver array. In: Proceedings of the International Society for Magnetic Resonance in Medicine 17. Honolulu (2009). p. 2936.
48. Wei J, Liu Z, Chai Z, Yuan J, Lian J, Shen GX. A realization of digital wireless transmission for MRI signals based on 802.11b. J Magn Reson. (2007) 186:358–63. doi: 10.1016/j.jmr.2007.03.003
49. Shen GX, Wei J, Pang Y. Design of digital wireless transmission for 64 channel array using IEEE 802.11n. In: Proceedings of the International Society for Magnetic Resonance in Medicine 16. Toronto (2008). p. 1121.
50. Saha SK, Deshpande P, Inamdar PP, Sheshadri RK, Koutsonikolas D. Power-throughput tradeoffs of 802.11n/ac in smartphones. In: 2015 IEEE Conference on Computer Communications (INFOCOM). Kowloon (2015). p. 100–8. doi: 10.1109/INFOCOM.2015.7218372
51. Ko Y, Bi W, Felder J, Shah NJ. Wireless digital data transfer based on WiGig/IEEE 802.11ad with self-shielded antenna gain enhancement for MRI. In: Proceedings of the International Society for Magnetic Resonance in Medicine 27. Montréal (2019). p. 1537.
53. Chu T-S, Gans M. High speed infrared local wireless communication. IEEE Commun Mag. (1987) 25:4–10. doi: 10.1109/MCOM.1987.1093675
54. Hou R, Chen Y, Wu J, Zhang H. A brief survey of optical wireless communication. In: 13th Australasian Symposium on Parallel and Distributed Computing (AusPDC 2015). Sydney (2015) p. 41–50.
55. Tsonev D, Chun H, Rajbhandari S, McKendry JJD, Videv S, Gu E, et al. A 3-Gb/s single-LED OFDM-based wireless VLC link using a gallium nitride μLED. IEEE Photonics Technol Lett. (2014) 26:637–40. doi: 10.1109/LPT.2013.2297621
56. Konstantinou G, Ali W, Chil R, Cossu G, Ciaramella E, Vaquero J. Experimental demonstration of an optical wireless MRI compatible PET/SPECT insert front-end. In: 2016 IEEE Nuclear Science Symposium, Medical Imaging Conference and Room-Temperature Semiconductor Detector Workshop (NSS/MIC/RTSD). Strasbourg (2016). p. 1–4. doi: 10.1109/NSSMIC.2016.8069524
57. Lu Z, Tian P, Fu H, Montes J, Huang X, Chen H, et al. Experimental demonstration of non-line-of-sight visible light communication with different reflecting materials using a GaN-based micro-LED and modified IEEE 802.11ac. AIP Adv. (2018) 8:105017. doi: 10.1063/1.5048942
58. Tsonev D, Videv S, Haas H. Light fidelity (Li-Fi): towards all-optical networking. In: Proceedings SPIE 9007, Broadband Access Communication Technologies VIII, 900702. San Francisco, CA (2014). doi: 10.1117/12.2044649
59. Lu JY, Robb F, Pauly J, Scott G. Wireless Q-spoiling of Receive Coils at 1.5T MRI. In: Proceedings of the International Society for Magnetic Resonance in Medicine 25. Honolulu (2017). p. 4297.
60. Lu JY, Grafendorfer T, Zhang T, Vasanawala S, Robb F, Pauly JM, et al. Depletion-mode GaN HEMT Q-spoil switches for MRI coils. IEEE Trans Med Imaging. (2016) 35:2558–67. doi: 10.1109/TMI.2016.2586053
61. Lu JY, Grafendorfer T, Robb F, Winkler S, Vasanawala S, Pauly JM, et al. Clock transmission methods for wireless MRI: a study on clock jitter and impact on data sampling. In: Proceedings of the International Society for Magnetic Resonance in Medicine 27. Montréal (2019). p. 1542.
62. Scott G, Robb F, Pauly J, Stang P. Software synchronization of independent receivers by transmit phase tracking. In: Proceedings of the International Society for Magnetic Resonance in Medicine 25. Honolulu (2017). p. 4311.
63. Reykowski A, Redder P, Calderon Rico R, Wynn T, Ortiz T, Dowling G, et al. High precision wireless clock recovery for on-coil MRI receivers using round-trip carrier phase tracking. In: Proceedings of the International Society for Magnetic Resonance in Medicine 26. Paris (2018). p. 27.
64. Scott G, Vasanawala S, Robb F, Stang P, Pauly J. Pilot tone software synchronization for wireless MRI receivers. In: Proceedings of the International Society for Magnetic Resonance in Medicine 26. Paris (2018). p. 25.
65. Reber J, Marjanovic J, Schildknecht C, Brunner DO, Pruessmann KP. Correction of gradient induced clock phase modulation for in-bore sampling receivers. In: Proceedings of the International Society for Magnetic Resonance in Medicine 25. Honolulu (2017). p. 1056.
66. Truong T-K, Darnell D, Song AW. Integrated RF/shim coil array for parallel reception and localized B0 shimming in the human brain. Neuroimage. (2014) 103:235–40. doi: 10.1016/j.neuroimage.2014.09.052
67. Darnell D, Cuthbertson J, Robb F, Song AW, Truong TK. Integrated radio-frequency/wireless coil design for simultaneous MR image acquisition and wireless communication. Magn Reson Med. (2019) 81:2176–83. doi: 10.1002/mrm.27513
68. Chen B, Weber N, Odille F, Large-Dessale C, Delmas A, Bonnemains L, et al. Design and validation of a novel MR-compatible sensor for respiratory motion modeling and correction. IEEE Trans Biomed Eng. (2017) 64:123–33. doi: 10.1109/TBME.2016.2549272
69. PowerStream. Available online at: https://www.powerstream.com/non-magnetic-lipo.htm (accessed November 30, 2019).
70. Saha A, Iqbal S, Karmaker M, Fairose Zinnat S, Tanseer Ali M. A wireless optical power system for medical implants using low power near-IR laser. In: 2017 39th Annual International Conference of the IEEE Engineering in Medicine and Biology Society (EMBC). Seogwipo (2017). doi: 10.1109/EMBC.2017.8037238
71. Wi-Charge. The Future of Power. Available online at: https://wi-charge.com/ (accessed September 26, 2019).
72. Werthen JG, Cohen MJ, Wu T-C, Widjaja S. Electrically isolated power delivery for MRI applications. In: Proceedings of the International Society for Magnetic Resonance in Medicine 14. Seattle (2006). p. 1353.
73. International Electrotechnical Commission (IEC). International Standard IEC 60825-1:2014. Safety of Laser Products - Part 1: Equipment Classification and Requirements 3. Geneva (2014).
74. Riffe MJ, Heilman JA, Griswold MA. Power scavenging circuit for wireless DC power. In: Proceedings of the International Society for Magnetic Resonance in Medicine 15. Berlin (2007). p. 3278.
75. Höfflin J, Fischer E, Hennig J, Korvink JG. Energy Harvesting towards autonomous MRI detection. In: Proceedings of the International Society for Magnetic Resonance in Medicine 21. Salt Lake City (2013). p. 728.
76. Middelstaedt L, Foerster S, Doebbelin R, Lindemann A. Power electronics for an energy harvesting concept applied to magnetic resonance tomography. In: Progress in Electromagnetics Research Symposium. Prague (2015). p. 1419–23.
77. Byron K, Robb F, Vasanawala S, Pauly J, Scott G. Harvesting power wirelessly from MRI scanners. In: Proceedings of the International Society for Magnetic Resonance in Medicine 27. Montréal (2019). p. 1535.
78. Byron K, Robb F, Stang P, Vasanawala S, Pauly J, Scott G. An RF-gated wireless power transfer system for wireless MRI receive arrays. Concepts Magn Reson Part B Magn Reson Eng. (2017) 47B:e21360. doi: 10.1002/cmr.b.21360
79. Byron K, Winkler SA, Robb F, Vasanawala S, Pauly J, Scott G. An MRI compatible RF MEMs controlled wireless power transfer system. IEEE Trans Microw Theory Tech. (2019) 67:1717–26. doi: 10.1109/TMTT.2019.2902554
80. Rizzo G, Loyau V, Nocua R, Lourme JC, Lefeuvre E. Potentiality of magnetoelectric composites for wireless power transmission in medical implants. In: 13th International Symposium on Medical Information and Communication Technology (ISMICT). Oslo (2019). p. 1–4. doi: 10.1109/ISMICT.2019.8743873
81. Schildknecht CM, Brunner DO, Schmid T, Reber J, Marjanovic J, Pruessmann KP. Wireless motion tracking with short-wave radiofrequency. In: Proceedings of the International Society for Magnetic Resonance in Medicine 27. Montréal (2019). p. 66.
Keywords: magnetic resonance imaging, radio frequency coil, signal transmission, wireless technologies, wireless power
Citation: Nohava L, Ginefri J-C, Willoquet G, Laistler E and Frass-Kriegl R (2020) Perspectives in Wireless Radio Frequency Coil Development for Magnetic Resonance Imaging. Front. Phys. 8:11. doi: 10.3389/fphy.2020.00011
Received: 01 October 2019; Accepted: 09 January 2020;
Published: 21 February 2020.
Edited by:
Wouter van Elmpt, Maastricht University, NetherlandsReviewed by:
Simone Angela S. Winkler, Weill Cornell Medicine, Cornell University, United StatesRiccardo Lattanzi, Langone Medical Center, New York University, United States
Copyright © 2020 Nohava, Ginefri, Willoquet, Laistler and Frass-Kriegl. This is an open-access article distributed under the terms of the Creative Commons Attribution License (CC BY). The use, distribution or reproduction in other forums is permitted, provided the original author(s) and the copyright owner(s) are credited and that the original publication in this journal is cited, in accordance with accepted academic practice. No use, distribution or reproduction is permitted which does not comply with these terms.
*Correspondence: Roberta Frass-Kriegl, cm9iZXJ0YS5mcmFzc0BtZWR1bml3aWVuLmFjLmF0