- 1Institute of Laser Technology, Tianshui Normal University, Tianshui, China
- 2Baoji Engineering Technology Research Center on Ultrafast Laser and New Materials, Baoji, China
- 3School of Physics and Optoelectronics Technology, Baoji University of Arts and Science, Baoji, China
A passively Q-switched mode-locked all-solid-state Tm:CaYAIO4 (Tm:CYA) laser with a MoS2 saturable absorber is demonstrated for the first time. Employing a typical X-type cavity and output coupling mirror of 9%, when the laser diode pump power is higher than 8.5 W, the laser enters into a stable Q-switched mode-locked operation, further increasing the pump power into a dual-wavelength operating state; the corresponding typical center wavelengths are 1,863 and 1,877 nm. When the pump power reaches 20 W, the maximum output power is 1.15 W, the frequency of mode-locked pulse is 103.7 MHz, and the modulation depth of mode-locked pulses in the Q envelope is close to 100%. The results prove that MoS2 saturable absorber is suitable for 2-μm high-power mode locking.
Introduction
The 2-μm band is located at the absorption peak of water and the window band of atmosphere, which is a safe area for the human eye. Based on the above characteristics, 2 μm band laser is widely used in high-precision surgery [1], free space communication [2], and other fields. In particular, high-power dual-wavelength mode-locked lasers can generate coherent terahertz radiation by difference frequency [3, 4], which is widely used in differential radar and remote space detection [5].
Passive mode locking is one of the common methods to obtain ultrashort pulse. In general, the mode-locked technique needs to have saturable absorber (SA), non-linear loop mirror, or non-linear polarization rotating [6]. At present, many materials have been employed as SA for passively mode-locked lasers, such as semiconductor saturable absorption mirrors, carbon nanotubes, graphene, and black phosphorus [7, 8]. An excellent SA should possess the properties of higher damage threshold, ultrafast recovery time, and lower saturation intensity [9, 10]. Among them, due to the complex process, narrow working bandwidth, and high cost of semiconductor saturable absorption mirrors, we need to look for other materials. Carbon nanotubes are widely used in fiber lasers [11, 12], but their performance is poor in 2 μm solid-state lasers due to their unique structures and properties. Graphene is widely used in 1 μm, but its absorption efficiency at 2 μm is weak, which limits its ability to adjust light, and black phosphorus is easily oxidized in the presence of oxygen and water, leading to its instability in the experiment [13]. As such, MoS2 has attracted our attention with its universality and good stability. MoS2, as a SA, was first widely used in fiber lasers [14–16]. Later, it is slowly used in solid-state lasers to realize Q-switching or mode-locked operation. In 2015, a passively Q-switched mode-locked (QML) Tm:LiLuF4 (LLF) laser with a MoS2 SA is demonstrated for the first time [17]. In 2017, the maximum peak power of 4.33 W is obtained using MoS2 as SA in Er:LUAG laser [18]. Next year, Li et al. first used MoS2 to realize Q-switched operation and obtained an average output power of 3.3 W in Tm,Ho:YAP lasers [19]. We also use MoS2 SA to realize QML operation in Tm,Ho:LLF [20] and Tm:LuAG [21] lasers, respectively. In short, MoS2 has great potentiality according to the current experimental progress.
CYA crystals are widely used because of their excellent mechanical strength, chemical stability, and high thermal conductivity [22]. As early as 1997, Tm:CYA crystal was pumped by Ti:Sapphire laser to realize continuous wave (CW) operation, in which the output power and slope efficiency were 50 mW and 18%, respectively [23]. In 2015, Kong et al. demonstrated a CW mode-locked Tm:CYA laser with a maximum output power of 830 mW and obtained dual-wavelength output of 1,958.9 and 1960.6 nm [24]. The next year, Yao et al. pumped Tm:CYA with a Raman fiber laser and obtained output of up to 6.8 W under CW operation [25]. Recently, Lan et al. used MoS2 for the first time to achieve Q-switched operation in Tm:CYA laser, with the maximum output power of 0.49 W [26].
In this paper, we first use MoS2 as SA to realize a Watt-level dual-wavelength QML operation in Tm:CYA laser. The output power of QML laser is 1.153 W, and the dual-wavelength is 1,863 and 1,877 nm, respectively. The dual-wavelength laser will have a broad application prospect in the field of optical communication and sensing.
Preparation of MoS2 SA
In this experiment, MoS2 SA is prepared by liquid phase stripping method. First, the powdered MoS2 is added to the water-based solution with a concentration of ~1 mg/ml. Then, a glass substrate is prepared by the following procedure. First, the glass substrate is immersed in deionized water. Second, it is treated with ultrasonic cleaning apparatus for ~10 min. Third, the surface dust of substrate is removed by ultrasound in alcohol for 10 min. Fourth, the treated glass piece is placed in a mixed solution of sulfuric acid and hydrogen peroxide for ~1 h before hydrophilic treatment. Finally, the glass piece is soaked in the glass in clean deionized water for ~10 min. Then, the glass substrate is prepared, and then the prepared MoS2 solution was dropped on it directly. Figure 1a is the electron micrograph of MoS2, which proves that MoS2 has been spun on the glass substrate. Figure 1b shows the Raman spectrum of MoS2. The phonon vibration modes of MoS2 mainly include (in plane) and A1g (out of plane), and the two main vibration modes change with the thickness. Specifically, the A1g mode shifted blue, while the E2g mode shifted red. In the figure, E2g and A1g are located at 383 and 402 cm−1, respectively. It can be seen that MoS2 is a small layer structure indeed. The prepared MoS2 SA material is shown in Figure 1c.
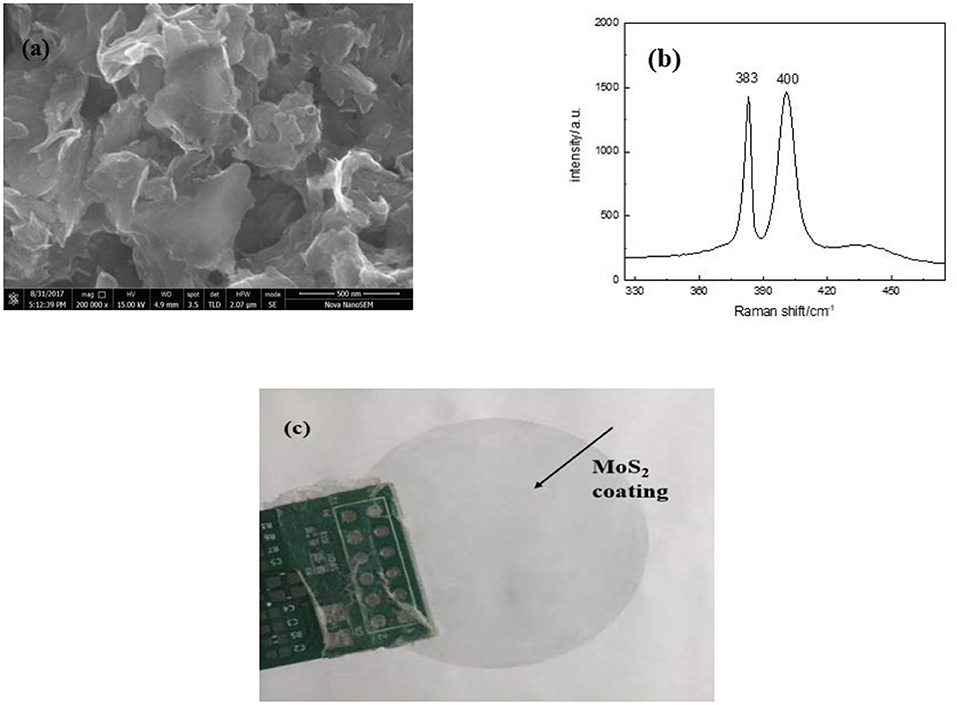
Figure 1. (a) Electron micrograph of MoS2. (b) The Raman spectrum of MoS2. (c) MoS2 saturable absorber.
Experimental Setup
The experiment setup of dual-wavelength QML Tm:CYA laser is shown in Figure 2. In this experiment, a 790 nm laser diode (LD) with a maximum output power of 30 W is used as the pump source. The Tm:CYA crystal is cut at the Brewster angle, and the two end faces are polished. The doped concentration of Tm3+ is 4%, and the size is 3.8 × 4 × 4 mm. The crystal was wrapped with indiu mfoil and tightly mounted in a water-cooled copper block system with stable temperature holding at 11°C. L is the pump focusing and collimating system with a ratio of 1:0.8 and a transmittance of 95% for 790 nm. Its working distance is 49.2 mm, and external diameter is 37.5 mm. In the laser cavity, M1 and M2 are flat concave mirrors with curvature radius of 75 and 100 mm, respectively. Both have high transmissivity to pump light and highly reflective to 1,850–2,100 nm. M3 is a flat-concave mirror with a curvature radius of 100 mm and has a reflectivity of more than 99.9% for wavelength from 1,850 to 2,100 nm, whose main function is to focus the oscillating light to the SA and start QML operation. M4 is a flat mirror with the same reflective film as M3. In the experiment, plane mirrors with 3, 5, and 9% transmissivity are used as the output couplers (OC).
Experimental Results and Discussion
The output power as a function of the pump power is plotted in Figure 2. Contrast experiments are carried out with OCs with different transmissivities of 3, 5, and 9%, respectively. At first, the CW laser performance is discussed without MOS2 SA in the cavity. The threshold power of 3% OC is 2.4 W, the slope efficiency is 11%, and the maximum output power of 1,857 mW is obtained at 20 W pump power. The threshold power of 5 and 9% OC are 2.6 and 2.8 W, respectively. When pump power is 20 W, the maximum output power is increased to 2,380 and 2,600 mW, respectively. To obtain high power output, we theoretically simulate the optimal transmissivity parameters of the OC.
The relationship between the best transmissivity of the cavity and the pump power is shown in Equation (1), where σ is the crystal emission cross-section, τf is the emission life, λP is the pump wavelength, Pin is the pump power, αp is absorption coefficient, L is the crystal length, δO is the cavity loss, is the average pump spot radius, and WO is the oscillating spot radius. Through the simulation Equation (1), the curve of Figure 3 is obtained. It can be seen from the figure that when the pump power is 20 W, the optimal transmissivity T of the cavity is ~0.088. Considering the actual situation of optical glass coating in processing, it is more appropriate to choose an output mirror with a transmittance of 0.09.
When the MoS2 SA is inserted into the cavity, the laser oscillation is obtained with the threshold power of 3, 3.2, and 3.5 W under 3, 5, and 9% OC, respectively. After entering the stable QML operation, the corresponding maximum output power is 506, 894, and 1,153 mW. The relationship between average output power and pump power in CW and QML operation is shown in Figures 4, 5, respectively. Through the comparison of the different OCs mentioned above, it is found that the QML output power of 9% OC is up to 1.15 W, which is the highest output power among all OCs. This is also consistent with the theoretical simulation above. This kind of QML pulse has potential application in remote detection.
The spectrum of the QML pulse is measured using an optical spectrum analyzer (AvaSpec-NIR256-2.5 TEC). As shown in Figure 6, the dual-wavelength synchronous output is obtained with the center wavelength of 1,863 and 1,877 nm and the spectrum of half-width of 11 and 8 nm, respectively. It is found that the central wavelength and the spectral width of the spectrum do not change with the output power, and the output spectrum is stable.
The pulses of QML laser were recorded by a fast photodiode (ET-500) with a digital oscilloscope (RIGOL, DS4034). The typical QML pulse trains in 500 μs, 10 ns, and 2 ns timescales are shown in Figure 7. The QML pulses have a repetition frequency of 103.7 MHz, corresponding to the laser cavity length of 1.45 m.
Since the autocorrelator is only suitable for measuring the pulse width of CW mode-locked pulse, the Q-switched mode-locked pulse cannot obtain autocorrelation envelope because of the envelope modulation of kilohertz, so we can only estimate the pulse duration roughly in theory. Here, Equation (2) is used to estimate the width of the QML pulse [27].
Here, tm is the rising edge time of the measured mode-locked pulse, tr is the rising edge time of the actual mode-locked pulse, tp is the rising edge time of the photodetector, and to is the rising edge time of the oscilloscope. In the experiment, the rising edge time of the mode-locked pulse is ~2.06 ns, and the rising edge time of the photodetector is ~35 ps. to can be estimated as 1,900 ps using the Equation (2) as follows.
Among them, Wbis the bandwidth of the oscilloscope, which is 200 MHz in the experiment. Thus, it can be calculated that the rise time of the mode-locked pulse is ~795 ps. Since the actual mode-locked pulse width is ~1.25 times of the rising edge time, it is calculated as 994 ps.
Conclusion
In conclusion, we first use MoS2 SA to realize a Watt-level dual-wavelength Q-switched mode locked operation in the Tm:CYA laser. Using a typical “X” type five mirror folded cavity, a maximum CW output power of 2.6 W is obtained under 9% output coupling mirror. After the laser enters into stable dual-wavelength QML operation, the maximum output power reaches 1.15 W with central wavelengths of 1,863 and 1,877 nm. The repetition frequency of QML is 103.7 MHz, and the modulation depth of mode-locked pulses in the Q envelope is close to 100%. The results show that MoS2 SA is suitable for 2 μm high-power mode locking and has potential application value in high-power mode locking. Next step, we will strive to achieve high-power CW mode-locked operation by increasing the pump power and reducing the loss of MoS2. The LD pumped high-power mode-locked laser has the advantage of low cost and will be widely used in industrial production.
Data Availability Statement
All datasets generated for this study are included in the article/supplementary material.
Author Contributions
WL and QX designed the overall scheme. CC and RS carried out specific experiments. YZ provided experimental guidance.
Funding
This work was supported by the National Natural Science Foundation of China (Nos. 11774257, 61564008, 11647008, and 11504416), the National Key Research and Development Program (2017YFB045200), the International Science and Technology Cooperation and Exchanges Project of Shaanxi (No. 2014KW07-01), and the Key Sciences and Technology Project of Baoji City (No. 2015CXNL-1-3).
Conflict of Interest
The authors declare that the research was conducted in the absence of any commercial or financial relationships that could be construed as a potential conflict of interest.
References
1. Wang J, Sramek C, Paulus YM, Lavinsky D, Schuele G, Anderson D, et al. Retinal safety of near-infrared lasers in cataract surgery. J Biomed Optics. (2012) 17:95001. doi: 10.1117/1.JBO.17.9.095001
2. Chan VWS. Optical space communications. IEEE J Select Topics Quant Electr. (2000) 6:959–75. doi: 10.1109/2944.902144
3. Majkic A, Zgonik M, Petelin A, Jazbinsek M, Ruiz B, Medrano B, et al. Terahertz source at 9.4 THz based on a dual-wavelength infrared laser and quasi-phase matching in organic crystals OH1. Appl Phys Lett. (2014) 105:141115. doi: 10.1063/1.4897639
4. Chang JH, Wang TT, Zhang C, Zhang C, Ge YX, Tao ZH. Compact and tunable mid-IR light source based on a dual-wavelength fiber laser. Chin Phys Lett. (2013) 30:114206. doi: 10.1088/0256-307X/30/11/114206
5. Walsh BM. Review of Tm and Ho materials; spectroscopy and lasers. Laser Phys. (2009) 19:855–66. doi: 10.1134/S1054660X09040446
6. Liu XM. Hysteresis phenomena and multipulse formation of a dissipative system in a passively mode-locked fiber laser. Phys Rev A. (2010) 81:023811. doi: 10.1103/PhysRevA.81.023811
7. Liu XM, Popa D, Akhmediev N. Revealing the transition dynamics from Q Switching to mode locking in a soliton laser. Phys Rev Lett. (2019) 123:093901. doi: 10.1103/PhysRevLett.123.093901
8. Liu XM, Yao XK, Cui YD. Real-time observation of the buildup of soliton molecules. Phys Rev Lett. (2018) 121:023905. doi: 10.1103/PhysRevLett.121.023905
9. Kong LC, Xie GQ, Yuan P, Qian LJ, Wang H, Yu H, et al. Passive Q-switching and Q-switched mode-locking operations of 2 μm Tm: CLNGG laser with MoS2 saturable absorber mirror. Photon Res. (2015) 3:A47–50. doi: 10.1364/PRJ.3.000A47
10. Guo B. 2D noncarbon materials-based nonlinear optical devices for ultrafast photonics. Chin Optics Lett. (2018) 16:020004. doi: 10.3788/COL201816.020004
11. Liu XM, Pang M. Revealing the buildup dynamics of harmonic mode-locking states in ultrafast lasers. Laser Photon Rev. (2019) 13:1800333. doi: 10.1002/lpor.201800333
12. Liu XM, Han DD, Sun ZP, Zeng C, Lu H, Mao D, et al. Versatile multi-wavelength ultrafast fiber laser mode-locked by carbon nanotubes. Sci Rep. (2013) 3:2718. doi: 10.1038/srep02718
13. Sun XL, Nie HK, He JL, Zhao RW, Su XC, Wang YR, et al. Passively mode-locked 1.34 μm bulk laser based on few-layer black phosphorus saturable absorber. Opt Express. (2017) 25:20025–32. doi: 10.1364/OE.25.020025
14. Du J, Wang Q, Jiang G, Xu CW, Zhao CJ, Xiang YJ, et al. Ytterbium-doped fiber laser passively mode locked by few-layer Molybdenum Disulfide (MoS2) saturable absorber functioned with evanescent field interaction. Sci Rep. (2014) 4:6346–9. doi: 10.1038/srep06346
15. Luo ZQ, Huang YZ, Zhong M, Li YY, Wu JY, Xu B, et al. 1-, 1.5-, and 2-μm fiber lasers q-switched by a broadband few-layer mos2 saturable absorber. J Light Technol. (2014) 32:4077–84. doi: 10.1109/JLT.2014.2362147
16. Du TJ, Luo ZQ, Yang RH, Huang YZ, Ruan QJ, Cai ZP, et al. 1.2-W average-power, 700-W peak-power, 100-ps dissipative soliton resonance in a compact Er:Yb co-doped double-clad fiber laser. Optics Lett. (2017) 42:462–5. doi: 10.1364/OL.42.000462
17. Zou X, Leng YX, Li YY, Feng YY, Zhang PX, Hang Y, et al. Passively Q-switched mode-locked Tm:LLF laser with a MoS2 saturable absorber. Chin Opt Lett. (2015) 13:081405. doi: 10.3788/COL201513.081405
18. Zhang SY, Guo L, Fan MQ, Lou F, Gao P, Hao L, et al. Passively Q-switched Er:LuAG laser at 1.65 μm using MoS2 and WS2 saturable absorbers. IEEE Photon J. (2017) 9:1–7. doi: 10.1109/JPHOT.2017.2691740
19. Li LJ, Yang XN, Zhou L, Xie WQ, Wang YL, Shen YJ, et al. Active/passive Q-switching operation of 2 μm Tm,Ho:YAP laser with an acousto-optical Q-switch/MoS2 saturable absorber mirror. Photon Res. (2018) 6:614–9. doi: 10.1364/PRJ.6.000614
20. Ling WJ, Xia T, Dong Z, Zuo YY, Li K, You LF, et al. Passively Q-switched mode-locked Tm,Ho:LLF laser with a reflection-type MoS2 saturable absorber. In: International Conference on Optical Instruments and Technology: Advanced Laser Technology and Applications. Beijing (2018), p. 106190Q. doi: 10.1117/12.2295490
21. Ling WJ, Sun R, Chen C, Zhang YN, Kang CP, Xu Q, et al. Passively Q-switched mode-locked Tm:LuAG laser with reflective MoS2 saturable absorber. Chin J Lasers. (2019) 46:0808002. doi: 10.3788/CJL201946.0808002
22. Kustov EF, Petrov VP, Petrova DS, Udalov JP. Absorption and luminescence spectra of Nd3± and Er3± ions in monocrystals of CaYAlO4. Phys Status Solid. (1977) 41:379–83. doi: 10.1002/pssa.2210410204
23. Moncorgé R, Garnier N, Kerbrat P, Wyon C, Borel C. Spectroscopic investigation and two-micron laser performance of Tm3+:CaYAlO4 single crystals. Opt Commun. (1997) 141:29–34. doi: 10.1016/S0030-4018(97)00219-8
24. Kong LC, Qin ZP, Xie GQ, Xu XD, Xu J, Yuan P, et al. Dual-wavelength synchronous operation of a mode-locked 2-μm Tm: CaYAlO4 laser. Opt Lett. (2015) 40:356–8. doi: 10.1364/OL.40.000356
25. Yao WC, Wu F, Zhao YG, Chen H, Xu XD, Shen DY. Highly efficient Tm:CaYAlO4 laser in-band pumped by a Raman fiber laser at 1.7 μm. Appl Opt. (2016) 55:3730–3. doi: 10.1364/AO.55.003730
26. Lan JL, Zhang XY, Zhou ZY, Xu B, Xu HY, Cai ZP, et al. Passively Q-switched Tm:CaYAlO4 laser using a MoS2 saturable absorber. IEEE Photon Technol Lett. (2017) 29:515–9. doi: 10.1109/LPT.2017.2662699
Keywords: Tm:CYA laser, MoS2 saturable absorber, Q-switching and mode-locking, dual wavelength, diode pumped
Citation: Chen C, Ling W, Sun R, Xu Q and Zhang Y (2020) Watt-Level Dual-Wavelength Q-Switched Mode-Locked All-Solid-State Tm:CYA Laser. Front. Phys. 7:252. doi: 10.3389/fphy.2019.00252
Received: 11 November 2019; Accepted: 27 December 2019;
Published: 04 February 2020.
Edited by:
Xueming Liu, Zhejiang University, ChinaReviewed by:
Zhi-Chao Luo, South China Normal University, ChinaZhengqian Luo, Xiamen University, China
Copyright © 2020 Chen, Ling, Sun, Xu and Zhang. This is an open-access article distributed under the terms of the Creative Commons Attribution License (CC BY). The use, distribution or reproduction in other forums is permitted, provided the original author(s) and the copyright owner(s) are credited and that the original publication in this journal is cited, in accordance with accepted academic practice. No use, distribution or reproduction is permitted which does not comply with these terms.
*Correspondence: Weijun Ling, d2psaW5ndHNAc2luYS5jb20=; Qiang Xu, eHVxaWFuZ0Bzbm51LmVkdS5jbg==