- 1Institute of Space Physics, Luoyang Normal University, Luoyang, China
- 2Henan Key Laboratory of Electromagnetic Transformation and Detection, Luoyang, China
Coronal mass ejections (CMEs) are intense solar explosive eruptions and have significant impact on geomagnetic activities. It is important to understand how CMEs evolve as they propagate in the solar-terrestrial space. In this paper, we studied the coalescence of magnetic flux ropes embedded in five interplanetary coronal mass ejections (ICMEs) observed by both ACE and Wind spacecraft. The analyses show that coalescence of magnetic flux ropes could persist for hours and operate in scale of hundreds of earth radii. The two merging flux ropes could be very different in the axial orientation and the plasma density and temperature, which should complicate the progress of coalescence and have impact on the merged structures. The study indicates that coalescence of magnetic flux ropes should be an important factor in changing the magnetic topology of ICMEs.
Key Points
1. Coalescence of magnetic flux ropes within five interplanetary coronal mass ejections was studied.
2. The process of coalescence could be steady and large-scaled.
3. The process of coalescence is an important factor in changing the magnetic topology of interplanetary coronal mass ejections.
Introduction
Coronal mass ejections (CMEs) are large-scale solar explosive eruptions and their counterparts in the interplanetary space, interplanetary coronal mass ejections (ICMEs), are known to be an important cause of intense geomagnetic disturbances [1–3]. The geomagnetic effectiveness of ICMEs has strongly relation with their magnetic structures. For example, Magnetic Clouds (MCs), a subset of ICMEs, are found to be more effective than non-MC ICMEs in causing intense geomagnetic storms [4]. CMEs are thought to originally be of magnetic flux rope structures (e.g., [5–7]). However, ICMEs appearing as flux rope (i.e., MCs) only account for 30−40% of ICMEs observed at 1 AU [8, 9]. Therefore, understanding how CMEs evolve as they propagate in the solar-terrestrial space is very important for the space weather forecasting.
As an ICME propagates in the interplanetary space, its interaction with ambient solar wind or being caught up by other ICMEs from behind can cause the change of its magnetic topology [10–17]. Multiple rope-like substructures have been detected within ICMEs [18–22]. Feng et al. [22] reported observations of three merging flux ropes within an ICME and they thought that the coalescence would lead to the formation of a bigger rope. However, the potential of coalescence of flux ropes in altering the magnetic topology (e.g., the scale of coalescence in space and time) is still unclear.
Phan et al. [23] made a statistical study of extended reconnection X-lines in the solar wind at 1 AU with the combined observations of ACE and Wind spacecraft. In the work presented here, we surveyed the reconnection current sheet listed in Phan et al. [23] and found five of them were formed during coalescence of magnetic flux ropes embedded in ICMEs. The analyses show that the operation of coalescence can extend hundreds of earth radii and persist for several hours. The two merging flux ropes could be very different in some aspects. We think that coalescence of flux ropes should play important roles in the evolution of ICMEs.
Data
The data used in this paper are obtained from several instruments onboard ACE and Wind spacecraft. Wind magnetic field data and plasma data with time resolution of 3 s are taken from the Fluxgate Magnetometer experiment and the 3DP instrument, respectively [24, 25]. ACE magnetic field data (1 and 16 s resolution) are from MAG and plasma data (64 s resolution) are from SWEPAM instrument [26, 27]. If not specified, the GSE coordinate system (the Geocentric Solar Ecliptic coordinate system in which the x-axis directs from the Earth to the Sun, the z-axis points north, perpendicular to the ecliptic plane, the y-axis completes the right-handed coordinate system) is used in this paper.
Observations
In this section we first show one example to illustrate the identification of ICMEs and the merging flux ropes, then the procedure for estimating the X-line length formed during the coalescence progress and the other four cases are presented.
Figure 1 shows observations made by ACE (black) and Wind (red) from Oct. 3rd, 2000 to Oct. 5th, 2000. For clarity, the time series of ACE are shifted 110 min forward. During the whole interval showed in Figure 1, the data curves of the magnetic field and plasma at the two spacecraft were generally similar. From ~12:00 on Oct. 3rd (the first vertical line), the magnetic field became smoother and its strength gradually increased (Figures 1a–d). In the meantime, the proton temperature and the plasma beta values dropped (Figures 1i,j). At ~03:00 on Oct. 5th (the second vertical line), the speed of the plasma, the proton temperature and the plasma beta values suddenly increased (Figures 1e,i,j). Based on the above observations, we think the spacecraft encountered an ICME during the interval bounded by the two dashed vertical lines.
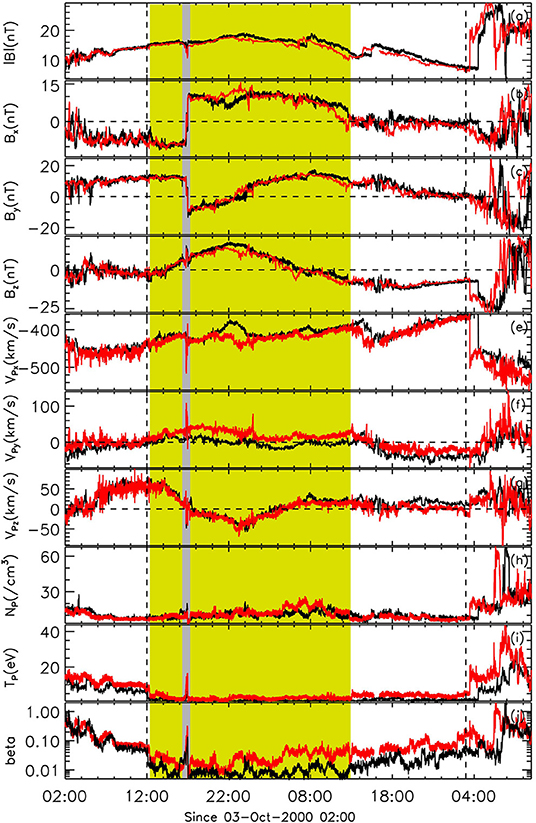
Figure 1. Measurements of ACE (black) and Wind (red) from 02:00 UT on Oct 03 to 11:00 UT on Oct 05, 2000. The observations of ACE have been shifted 110 min for forward. (a–d) Magnitude and three components of the magnetic field. (e–g) Three components of plasma velocity. (h–j) Proton density, temperature and proton plasma beta values. The two vertical dashed lines indicates the boundary of the ICME. the two orange regions denote the two merging flux ropes. The shadow region denotes the reconnection current sheet intermediating the calescence.
During the two intervals covered by the orange color, the magnetic field rotated. For the first orange region, Bz gradually increased from−2 nT to 7 nT (Figure 1d). For the second orange region, By gradually increased from −6 to 15 nT (Figure 1c) and Bz first increased to 15 nT, then decreased to −8 nT (Figure 1d). Along with the rotation, the strength of the magnetic field enhanced. The rotation of the magnetic field and the concurrent enhancement in its strength indicated that the two orange regions corresponded to crossing of two flux ropes. With the assumption of two dimension and quasi-steady state, the axis of a flux rope can be determined by Grad-Shafranov (G-S) reconstruction method [28]. According to the G-S equation, the thermal pressure and the magnetic pressure are constant along one magnetic field line in the plane perpendicular to the axial direction [28]. Applying the G-S reconstruction method to the data of the two orange regions, the obtained axis of the two flux ropes was (φ = 116.08, θ = 19.45) for the earlier, and (φ = 1.82, θ = 29.88) for the latter, where φ and θ are the longitude and latitude with respect to the ecliptic plane.
In the intermediate region (the shadow region) between the two magnetic flux ropes, the spacecraft detected steep changes in Bx and By with Bx jumping from −9 to 11 nT and By dropping from 12 to −10 nT (Figures 1b,c). Meanwhile, the plasma velocity in the Vx and Vy component locally peaked (Figures 1e,f). The proton temperature and the plasma beta values also showed a local peak (Figures 1i,j). The above observations indicated that the spacecraft might cross exhaust of magnetic reconnection, which can be more clearly in Figure 2.
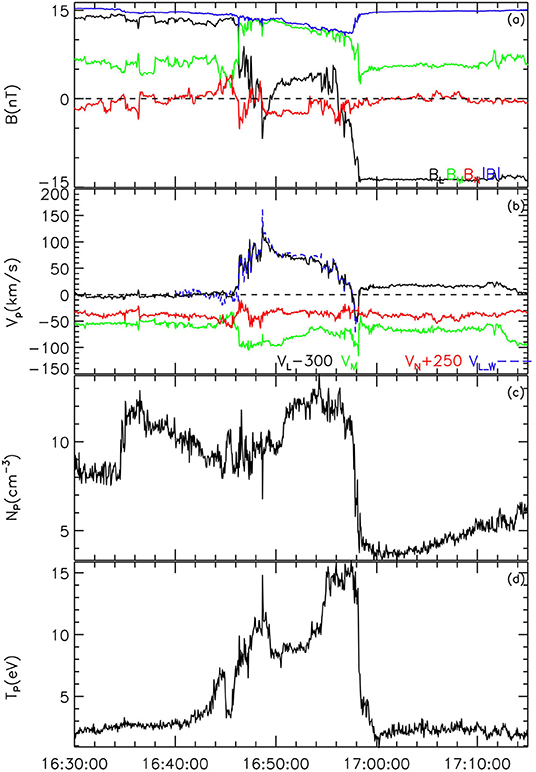
Figure 2. The enlarged vision of current sheet (the shadow region in Figure 1) in the LMN coordinate system. (a) Magnitude (blue) and three components of the magnetic field, L (black), M (green), and N (red). (b) Three components of plasma velocity. The dashed blue line represent the velocity predicted by walen relation. Note that the origin data curves have been shifted up or down for clarity. (c–d) The proton density and temperature.
In Figure 2, all vectors are presented in a local LMN coordinate system, where L was assumed to be along the reconnection outflow direction, M along the X-line direction and N along the normal direction of the reconnection current sheet. N was determined by minimum variance analysis of the magnetic field across the current sheet [29] and that M was chose so that the M components of the in-plane asymptotical magnetic field in both sides of the current sheet are same [30]. L = M × N forms the right-hand coordinate system. The most remarkable feature of the magnetic field is the two-step decrease in BL (Figure 2a), which corresponded to a bifurcated current sheet. Within the current sheet, the proton temperature increased (Figure 2d). The changes in VL (Figure 2b) during the crossing of the current sheet were consistent with these predicted (the dashed lines) by walén relation, which were calculated from the following equation [31, 32]:
Note that the pressure anisotropy factor, α, was assumed to be zero. Therefore, we thought that the spacecraft crossed exhaust of magnetic reconnection [33]. Detection of the reconnection current sheet between two flux ropes indicated that the two flux ropes were merging [22].
Adopting a similar procedure as that in Phan et al. [32], the extent of the X-line associated with the coalescence was estimated. The reconnection current sheet intermediating the coalescence was assumed to be planar and its normal direction obtained by minimum variance analysis was (φ = 41.19, θ = −17.41). The separation of the two ships was [193, 223, −3.8] RE in GSE. Using this normal direction and the planar assumption, the predicted temporal delay from ACE to Wind was 103 min which was close to the observed temporal delay, 110 min. This agreement indicated that the obtained normal direction and the planar assumption were valid. The direction of the X-line, M was (φ = 52.55, θ = 72.26) [30]. With the knowledge of N, M and the separation of the two ships, the distance along the X-line between the locations where the two ships intersected the current sheet was calculated to be 14 RE, which meant that the extend of the coalescence in space was at least 14 RE. The temporal delay between the two ships was ~110 min and the interval covered by the reconnection current sheet was ~12 min. Therefore, the progress of coalescence at least operated for 122 min.
With similar procedure, another four events of coalescence of flux ropes within an ICME were analyzed. The four events and the reconnection current sheet intermediating the coalescence are, respectively, presented in Figures 3, 4. Some of the five ICMEs have been studied by other researches [21, 22]. The details of the five cases are listed in Table 1. These cases were different in some aspects. The interval of the two merging flux ropes only occupied a small portion (~23%) of the whole duration of the ICME in Apr. 2000 (Figure 3B). However, for the other four cases, the two merging flux ropes occupied most of the ICME that they were embedded in Figures 1, 3A,C,D. The angle formed by the axes of the two merging flux ropes varied from case to case with a range from ~70° to ~160° (Table 1, in column Fr2-to-fr1). The plasma carried by the merging flux ropes could also be different in temperature and density (Figures 3Ah,Ci,Dh). For example, the plasma density was much higher in the latter flux ropes than that in the former one for the case in Mar. 1998 (Figure 3Ah). There were also significant differences in the estimated mini duration of the magnetic reconnection and length of X-line associated with the coalescence (Table 1, in columns len and dur). For example, the duration and the length were 34 min and 8 RE, respectively, for the case in Apr. 2004, while for the case in Feb. 2002, the values were 150 min and 393 RE, respectively. Finally, the density and temperature of plasma were not symmetric on both side of the reconnection current sheet in some cases (e.g., Figures 4Ac,Cd,Dc).
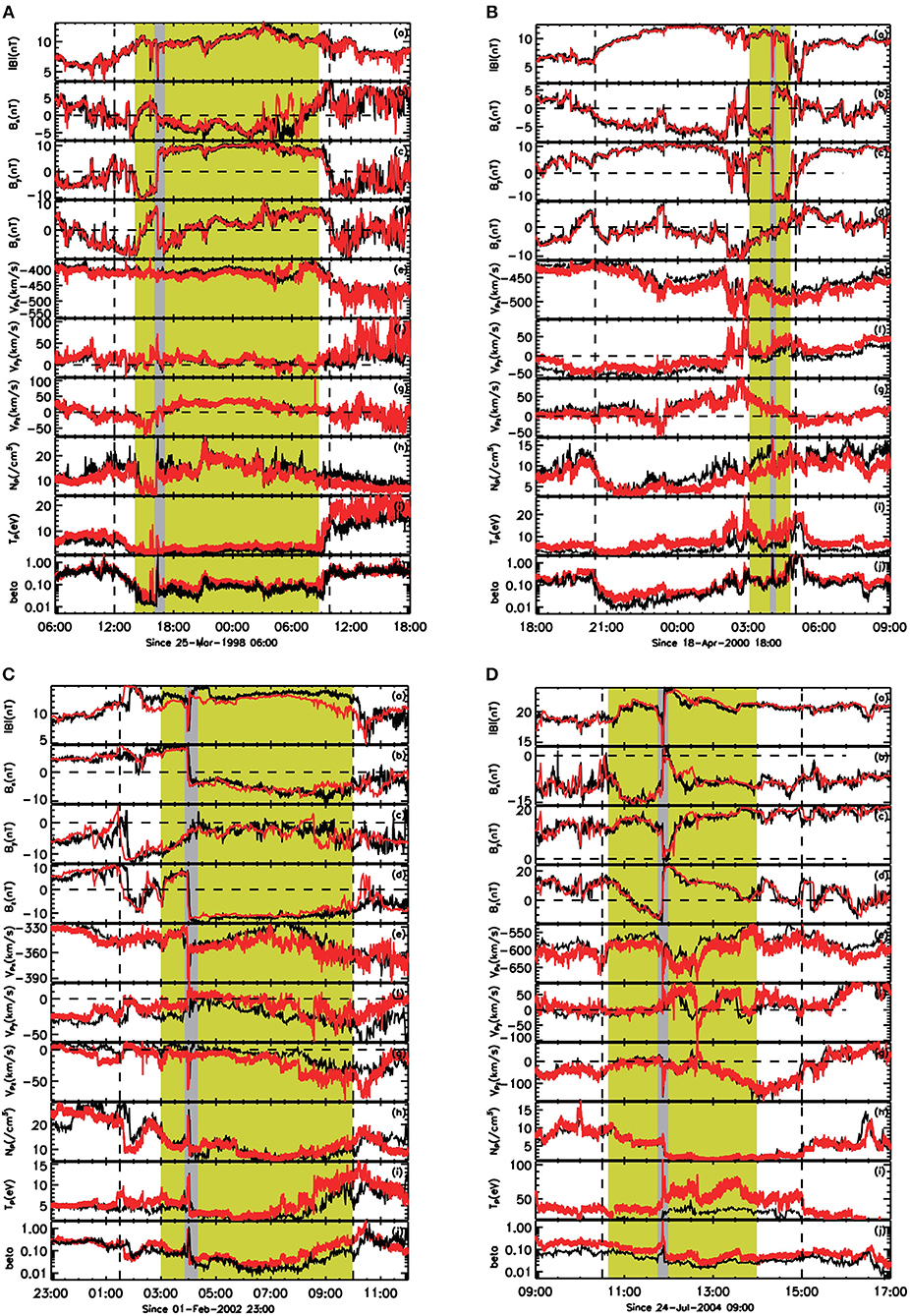
Figure 3. The other four ICMEs in Mar. 1998 (A), Apr. 2000 (B), Feb. 2002 (C), and Jul. 2004 (D). The observations of ACE have been shifted 5 min for case A (28 min for case B, 147 min for case C, −13 min for case D) forward. For each case, the figure format is similar to Figure 1.
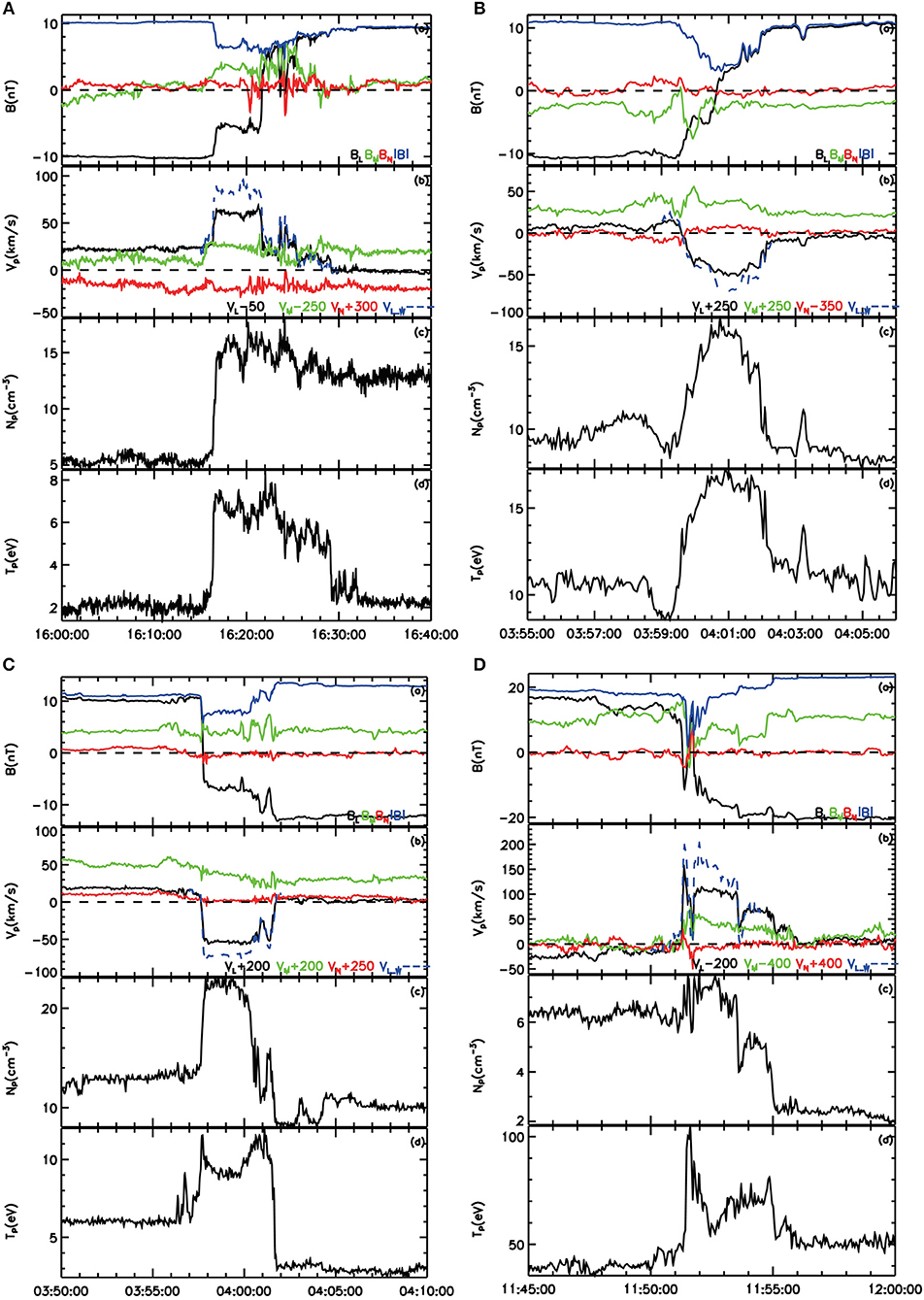
Figure 4. The reconnection current sheet intermediating the coalescence of flux ropes in ICME (A–D). For each case, the figure format is similar to Figure 2.
Discussion and Conclusion
ICMEs consisting of multiple flux ropes have been reported [20, 21]. Recently, Feng et al. [22] reported observations of an ICME within which a series of merging flux ropes was detected. They thought the coalescence would lead to the formation of bigger ropes in the ICME. However, if the magnetic reconnection intermediating the coalescence is patchy and transient, the change made by coalescence in the magnetic topology of ICMEs will be localized in space. The cases presented here shows that coalescence of magnetic flux ropes can operate in scale of hundreds of Earth radii and persist for hundreds of minutes. Note that the presented values in scale and duration of coalescence were likely to be much underestimated. Therefore, the progress of coalescence should be an important factor in the evolution of CMEs, If CMEs originally are of magnetic flux rope structures.
Simulations show that coalescence of magnetic flux ropes with same axis direction will end up with one bigger rope [14, 34]. The case in the real interplanetary space shall be more complex. In Feng et al. [22], the axis of the first two merging flux ropes had nearly opposite directions, which they thought the coalescence may lead to the formation of a bigger rope with weak axial field. For the five case reported here, the axes of the two merging flux ropes were not parallel but formed an angle ranging from ~70° to ~160°. The direction of the X-line also formed big angles with the ropes' axis (the last column in Table 1). The relative attitude of the two merging flux ropes should have significant impact on the structures formed by the process of coalescence. Awasthi et al. [35] reported a non-MC ICME whose pre-eruptive structure consisted of multiple-braided flux ropes with different degrees and they thought reconnection occurring between these flux ropes was responsible for the complex structure of the ICME. The presented results are consistent with the observations in Awasthi et al. [35]. The difference in the plasma (e.g., in the temperature and density) carried by the merging flux ropes could cause asymmetric conditions at both side of the reconnection current sheet (Figures 4Ac,Cd,Dc), which may further complicate the progress of coalescence.
In summary, we reported five ICMEs observed by both ACE and Wind spacecraft, within which merging flux ropes were detected. The coalescence of magnetic flux ropes could be steady and large scale. The two merging ropes could be different in the axial orientation and the plasma density and temperature. The results showed here indicates that coalescence of magnetic flux ropes is an important factor for understanding of the evolution of CMEs.
Author Contributions
YZ drafted the manuscript and led the observational analysis. HF provided heuristic advice and revised the manuscript. QL and GZ conducted the G-S reconnection. All authors contributed to the interpretation of the results and helped draft the manuscript.
Conflict of Interest
The authors declare that the research was conducted in the absence of any commercial or financial relationships that could be construed as a potential conflict of interest.
Acknowledgments
We acknowledge supports from NSFC under grant Nos. 41674170, 41804162, and 41974197. We thank NASA/GSFC for the use of data from the Wind. These data can obtain freely from the Coordinated Data Analysis Web (http://cdaweb.gsfc.nasa.gov/cdaweb/istp_public/).
References
1. Tsurutani BT, Gonzalez WD, Tang F, Akasofu SI, Smith EJ. Origin of interplanetary southward magnetic fields responsible for major magnetic storms near solar maximum (1978–1979). J Geophys Res. (1988) 93:8519–31. doi: 10.1029/JA093iA08p08519
2. Gonzalez WD, Tsurutani BT, Clúa de Gonzalez AL. Interplanetary origin of magnetic storms. Space Sci Rev. (1999) 88:529–62. doi: 10.1023/A:1005160129098
3. Zhang J, Dere KP, Howard RA. Identification of Solar Sources of Major Geomagnetic Storms between 1996 and 2000. Astrophys J. (2008) 582:520. doi: 10.1086/344611
4. Gonzalez WD, Echer E, Clua-Gonzalez AL, Tsurutani BT. Interplanetary origin of intense geomagnetic storms (Dst < -100 nT) during solar cycle 23. Geophys Res Lett. (2007) 34:L06101. doi: 10.1029/2006GL028879
5. Rust DM, Kumar A. Evidence for helically kinked magnetic flux ropes in solar eruptions. Astrophys J. (2009) 464:L199. doi: 10.1086/310118
6. Liu R, Chang L, Wang S, Na D, Wang H. Sigmoid-to-flux-rope transition leading to a loop-like coronal mass ejection. Astrophys J Lett. (2010) 725:L84–90. doi: 10.1088/2041-8205/725/1/L84
7. Zhang J, Cheng X, Ding M. Observation of an evolving magnetic flux rope before and during a solar eruption. Nat. Commun. (2012) 3:747. doi: 10.1038/ncomms1753
8. Gosling J. Coronal mass ejections and magnetic flux ropes in interplanetary space. Phys Magn Flux Ropes. (1990) A92–31201:12–75. doi: 10.1029/GM058p0343
9. Mulligan T, Russell CT, Gosling J. On interplanetary coronal mass ejection identification at 1AU. In: AIP Conference Proceedings. Melville, NY: AIP (1999). p. 693–6. doi: 10.1063/1.58659
10. Farrugia C, Berdichevsky D. Evolutionary signatures in complex ejecta and their driven shocks. Ann Geophys. (2004) 22:3679–98. doi: 10.5194/angeo-22-3679-2004
11. Feng HQ, Wu DJ, Wang JM, Chao JK. Magnetic reconnection exhausts at the boundaries of small interplanetary magnetic flux ropes. Astron Astrophys. (2011) 527:A67. doi: 10.1051/0004-6361/201014473
12. Gopalswamy N, Yashiro S, Kaiser ML, Howard RA, Bougeret JL. Radio signatures of coronal mass ejection interaction: coronal mass ejection cannibalism?. Astrophy J. (2001) 548:L91–4. doi: 10.1086/318939
13. Gopalsamy N, Yashiro S, Kaiser ML, Howard RA, Bougeret JL. Interplanetary radio emission due to interaction between two coronal mass ejections. Geophys Res Lett. (2002) 29:1265–8. doi: 10.1029/2001GL013606
14. Odstrcil D, Vandas M, Pizzo VJ, MacNeice P. Numerical simulation of interacting magnetic flux ropes. In: Velli M, Bruno R, Malara F, editors. SOLARWIND 10, AIP Conference Proceedings. Melville, NY: AIP (2003). p. 699–702. doi: 10.1063/1.1618690
15. Temmer M, Vrsnak B, Amerstorfer T, Bein B. Characteristics of kinematics of a coronal mass ejection during the 2010 August 1 CME-CME interaction event. Astrophys J. (2012) 749:57. doi: 10.1088/0004-637X/749/1/57
16. Liu YD, Yang Z, Wang R, Luhmann JG, Richardson JD, Lugaz N. Sun-to-Earth characteristics of two coronal mass ejections interacting near 1 au: formation of a complex ejecta and generation of a two-step geomagnetic storm. Astrophys J. (2014) 793:L41–6. doi: 10.1088/2041-8205/793/2/L41
17. Ruffenach A, Lavraud B, Farrugia CJ, Demoulin P, Dasso S, Owens MJ, et al. Statistical study of magnetic cloud erosion by magnetic reconnection. J Geophys Res. (2015) 120:43–60. doi: 10.1002/2014JA020628
18. Fainberg J, Osherovich VA, Stone RC, MacDowall RJ. Ulysses observations of electron and proton components in a magnetic cloud and related wave activity. In: Winterhalter D, Gosling JT, Habbal SR, Kurth WS, Neugebauer M, editors. Proceeding Solar Wind 8 Conference, Dana Point. Melville, NY: AIP (1996). p. 554–7. doi: 10.1063/1.51513
19. Osherovich VA, Fainberg J, Stone RG. Multi-tube model for interplanetary magnetic clouds. Geophys Res Lett. (1999) 26:401–4. doi: 10.1029/1998GL900306
20. Hu Q, Smith CW, Ness NF, Skoug RM. Multiple flux rope magnetic ejecta in the solar wind. J Geophys Res. (2004) 109:A03102. doi: 10.1029/2003JA010101
21. Chian AC-L, Feng HQ, Hu Q, Loew MH, Miranda RA, Muñoz PR, et al. Genesis of interplanetary intermittent turbulence: a case study of rope-rope magnetic reconnection. Astrophys J. (2016) 832:179. doi: 10.3847/0004-637X/832/2/179
22. Feng H, Zhao Y, Zhao G, Liu Q, Wu D. Observations on a series of merging magnetic flux ropes within an interplanetary coronal mass ejection. Geophys Res Lett. (2019) 146:5–10. doi: 10.1029/2018GL080063
23. Phan TD, Gosling JT, Davis MS. Prevalence of extended reconnection X-lines in the solar wind at 1 AU. Geophys Res Lett. (2009) 36:L09108. doi: 10.1029/2009GL037713
24. Lepping RP, Acuna MH, Burlaga LF, Farrell WM, Slavin JA, Schatten KH, et al. The wind magnetic field investigation, edited by Russell, C. T. Space Sci Rev. (1995) 71:207–29. doi: 10.1007/BF00751330
25. Lin RP, Anderson KA, Ashford S, Carlson C, Curtis D, Ergun R, et al. A three-dimensional plasma and energetic particle investigation for the wind spacecraft. Space Sci Rev. (1995) 71:125–53. doi: 10.1007/BF00751328
26. Mccomas DJ, Bame SJ, Barker P, Feldman WC, Phillips JL, Riley P, et al. Solar wind Electron Proton Alpha Monitor (SWEPAM) for the advanced composition explorer. Space Sci Rev. (1998) 86:563–612. doi: 10.1023/A:1005040232597
27. Smith CW, L'Heureux JL, Ness NF, Acuña MH, Burlaga LF, Scheifele J. The ace magnetic fields experiment. Space Sci. Rev. (1998) 86:613–32. doi: 10.1007/978-94-011-4762-0_21
28. Hu Q, Sonnerup BUÖ. (2002). Reconstruction of magnetic clouds in the solar wind: orientation and configuration. J Geophys Res. 107:10–5. doi: 10.1029/2001JA000293
29. Sonnerup BUÖ, Cahill LJ. Magnetopause structure and attitude from explorer 12 observations. J Geophys Res. (1967) 72:171–83. doi: 10.1029/JZ072i001p00171
30. Sonnerup BUÖ. Magnetopause reconnection rate. J Geophys Res. (1974) 79:1546–9. doi: 10.1029/JA079i010p01546
31. Paschmann G, Papamastorakis I, Baumjohann W, Sckopke N, Carlson CW, Sonnerup BUÖ, et al. The magnetopause for large magnetic shear: AMPTE/IRM observations. J Geophys Res. (1986) 91:11099–115. doi: 10.1029/JA091iA10p11099
32. Phan TD, Gosling JT, Davis MS, Skoug RM, Øieroset M, Lin RP, et al. A magnetic reconnection X-line extending more than 390 Earth radii in the solar wind. Nature. (2006) 439:175. doi: 10.1038/nature04393
33. Gosling JT, Skoug RM, McComas DJ, Smith CW. Direct evidence for magnetic reconnection in the solar wind near 1 AU. J Geophys Res. (2005) 110:A01107. doi: 10.1029/2004JA010809
34. Schmidt J, Cargill P. A numerical study of two interacting coronal mass ejections. Ann Geophys. (2004) 22:2245–54. doi: 10.5194/angeo-22-2245-2004
Keywords: interplanetary coronal mass ejection, magnetic flux rope, coalescence, magnetic reconnection, magnetic clouds
Citation: Zhao Y, Feng H, Liu Q and Zhao G (2019) Coalescence of Magnetic Flux Ropes Within Interplanetary Coronal Mass Ejections: Multi-cases Studies. Front. Phys. 7:151. doi: 10.3389/fphy.2019.00151
Received: 29 June 2019; Accepted: 19 September 2019;
Published: 04 October 2019.
Edited by:
Rui Liu, University of Science and Technology of China, ChinaReviewed by:
Qiang Hu, University of Alabama in Huntsville, United StatesFang Shen, National Space Science Center (CAS), China
Copyright © 2019 Zhao, Feng, Liu and Zhao. This is an open-access article distributed under the terms of the Creative Commons Attribution License (CC BY). The use, distribution or reproduction in other forums is permitted, provided the original author(s) and the copyright owner(s) are credited and that the original publication in this journal is cited, in accordance with accepted academic practice. No use, distribution or reproduction is permitted which does not comply with these terms.
*Correspondence: Hengqiang Feng, ZmVuZ2hxOTkyMUAxNjMuY29t