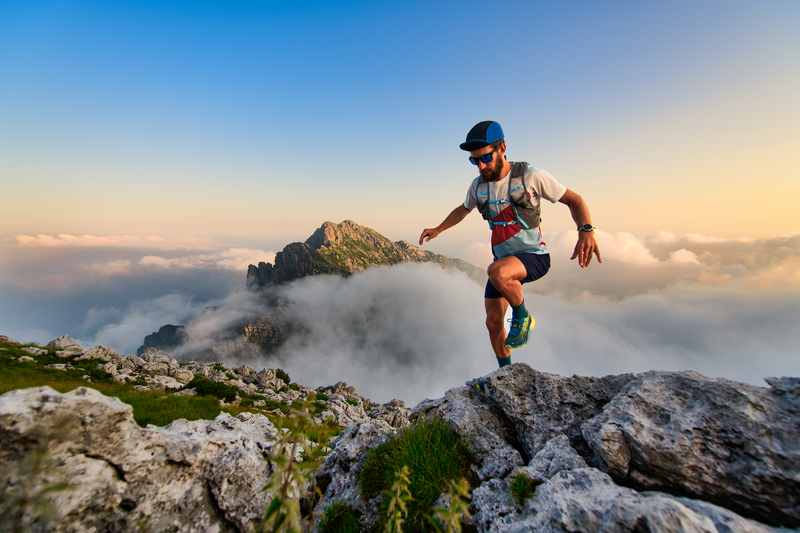
94% of researchers rate our articles as excellent or good
Learn more about the work of our research integrity team to safeguard the quality of each article we publish.
Find out more
ORIGINAL RESEARCH article
Front. Phys. , 14 May 2019
Sec. Interdisciplinary Physics
Volume 7 - 2019 | https://doi.org/10.3389/fphy.2019.00074
This article is part of the Research Topic Physics of Porous Media View all 15 articles
Wettability alteration was proposed as one of the enhanced oil recovery (EOR) mechanisms for nanoparticle fluid (nanofluid) flooding. The effect of nanoparticle adsorption on wettability alteration was investigated by wettability index measurement of Berea sandstone core injected with nanofluids and by contact angle measurement of a glass surface treated with nanofluids. Nanoparticle adsorption was studied by single phase coreflooding with nanofluids in Berea sandstone. The adsorption isotherm and the impact of adsorption on the effective permeability were investigated by measuring the effluent nanoparticle concentration and differential pressure across the core. Results showed that hydrophilic nanoparticles (e.g., fumed silica) made the core slightly more water wet, and hydrophobic nanoparticles (e.g., silane modified fumed silica) delayed spontaneous imbibition but could not alter the original wettability. It was found that hydrophilic nanoparticles treatment reduced contact angle between oil and water by about 10 to 20 degree for a glass surface. Results also showed that different types of nanoparticle have different adsorption and desorption behavior and different ability to impair the permeability of Berea sandstones cores.
The wettability of reservoir rock plays an important role in oil field development. It controls the relative permeability, capillary pressure, and residual oil distribution. Altering wettability to a favorable status will lead to more oil production. It has been reported that oil recovery is optimum at slightly water-wet to neutral wet reservoir [1]. Traditionally, surfactant flooding was utilized to alter reservoir rock wettability to more water wet, which can enhance spontaneous imbibition and increase oil recovery during water injection [2, 3]. Hammond and Unsal [4] discussed the possible mechanisms of wettability alteration with surfactants. They include surfactant adsorption onto rock surface and surfactant complexing with contaminant molecules of crude oil that are adsorbed on the rock surface and stripping them off. Salehi et al. studied the mechanisms of wettability alteration using surfactant in naturally fractured reservoirs, they found that both ion-pair formation and adsorption of surfactant molecules are the two main mechanisms responsible for altering the rock wettability to more water-wet, and surfactants with higher charge density on the head groups were more effective in altering wettability to a more water-wet state [2].
Recently, nanoparticles were proposed as wettability alteration agents to change the wettability of reservoir rock [5, 6]. A nanoparticle is a particle with size ranging from 1 to 100 nm. A nanoparticle suspension is called a nanofluid. It has been applied in many different disciplines including heat transfer [7], biomedicines [8], and soil remediation [9]. Besides EOR and wettability alteration, nanofluids have been proposed for applications on exploration, drilling and production, equipment manufacturing, refining and processing [10]. The EOR mechanisms of a nanofluid were discussed by Li et al. [11]. They include interfacial tension reduction, wettability alteration, pore channels plugging, disjoining pressure, and emulsification.
Miranda et al. [12] reported that silica nanoparticles have many advantages as EOR agents, such as: (1) 99.8% of silica nanoparticle is silicon dioxide, which is the main component of sandstone, thus making it an environmentally friendly material; (2) properties such as thermal conductivity, stress–strain relationship and rheology strongly depend on size and shape of the nanoparticles, which can be tailor designed during the manufacturing process; (3) the chemical behavior of nanoparticles can be controlled by changing surface coating; (4) the price of silica nanoparticle is cheaper than most of EOR chemicals.
The ability of nanoparticles to alter the wettability has been extensively studied. Contact angle measurements show that different types of nanoparticle (silicon dioxide, iron oxide, aluminum oxide and titanium dioxide) can reduce contact angle between oil and water, making surface more water wet regardless of initial surface wettability [13–16]. Microfluidic flooding experiments show that nanofluid flooding can alter wettability of glass grains. Nanoparticle adsorption on glass grains leading to wettability change from oil wet to water wet was observed in microfluidic experiments [13]. Lu et al. [17] reported imbibition of nanofluids into a capillary channel that changes wettability to strongly water wet, so the residual oil formed spherical droplets and can move freely with low resistance. Disjoining pressure is proposed as another mechanism of wettability alteration by nanofluids. Various researchers have investigated the ability of a nanofluid to displace oil from a solid surface due to disjoining pressure [18–20]. The phenomenon of nanoparticles ordering themselves into a confined wedge between an oil drop and a solid substrate is a consequence of entropy increase of the overall nanofluids by permitting greater freedom for nanoparticles in the bulk liquid. These ordered microstructures exert an excess pressure separating the two surfaces confining the nanofluids. This excess pressure is defined as a structural disjoining pressure [20]. The particles that are present in this three-phase contact region tend to form a wedge-like structure and force themselves between the discontinuous phase and the substrate. Particles present in the bulk fluid exert a pressure forcing the particles in the confined region to move forward. The energies that drive this mechanism are Brownian motion and electrostatic repulsion between the particles. The force imparted by a single particle is extremely weak, but when large amounts of small particles are present, the pressure can be upwards of 50,000 Pa at the vertex. Particle size and the associated particle charge density also affect the strength of this force. The smaller the particle size, the higher is the charge density, and the larger is the electrostatic repulsion between those particles. When this force is confined to the vertex of the discontinuous phase, displacement occurs in an attempt to regain equilibrium. As with any colloidal system, particle size, temperature, salinity and surface characteristics of the substrate affect the magnitude of the disjoining force [21].
Adsorption and transport behavior of nanoparticles inside porous media play a very critical role for wettability alteration. Normally, when hydrophilic nanoparticles are injected into a porous medium, five phenomena can occur: adsorption, desorption, blocking, transport and aggregation of nanoparticles. Five forces dominate the interactions between nanoparticles and pore walls: the attractive potential force of van der Waals, repulsion force of electric double layers, Born repulsion, acid-base interaction, and hydrodynamics [22]. When the total force of these five forces is negative, the attraction is larger than repulsion between nanoparticles and pore walls, which leads to adsorption of nanoparticles on the pore walls. Otherwise desorption of nanoparticles from the pore walls will occur. Adsorption and desorption is a dynamic balance process controlled by the total force between nanoparticles and pore walls. Zhang et al. [23] discussed that both reversible and irreversible adsorption of nanoparticles occur during transport through porous media. Blocking will take place if the diameter of the particle or nanoparticle aggregate is larger than the size of pore throat. Aggregation of nanoparticles happens if the previous equilibrium system breaks up and nanoparticles form clusters [24].
In this paper, in order to study the effect of nanoparticles (hydrophilic and hydrophobic) adsorption on wettability alteration, several commonly used silica nanoparticles were used. Two approaches were used in wettability alteration experiments. First, overall wettability change of a core plug treated with nanoparticles was studied by measuring the wettability index. Second, the contact angle between oil and water was measured on a glass surface soaked in a nanofluid. The results from these two approaches were analyzed comprehensively. Since the nanoparticle adsorption is crucial for wettability alteration of the core, the adsorption behavior of nanoparticles inside porous media was investigated by single phase coreflooding of a nanofluid. Nanoparticles adsorption curves and differential pressure curves were plotted to analyzed nanoparticles adsorption and transport behavior inside porous media.
This study is novel in several aspects. First, this is the first quantitative study of the ability of nanoparticles to change the wettability of a core using the wettability index, which can provide an overall wettability state for an oil reservoir. Second, this is the first reported study of the transport of nanoparticles in Berea sandstone cores giving the transport behavior of nanoparticles in such porous medium with complex pore structures.
Hydrophilic fumed silica nanoparticles (FNP), dimethyldichlorosilane modified hydrophobic fumed silica nanoparticles (FNP-O) and hydrophilic silica colloidal nanoparticles (CNP) were provided by Evonik Industries and employed in this study. They were supplied by Evonik Industries. FNP and FNP-O were manufactured as solid powder and CNP were provided as a highly concentrated suspension. FNP and CNP have been characterized with Cryo-Transmission Electron Microscope (Cryo-TEM) and Scanning Electron Microscopy (SEM) and images are shown in Figures 1, 2, respectively. FNP has irregular shape in suspension and CNP is seen as separate spherical particles. FNP and FNP-O have an average primary particle size of 7 nm and specific surface area of 300 m2/g. However, they may aggregate to form bigger particles in dispersion. CNP have an average single particle size of 18 nm and specific surface areas of 350 m2/g. They do not aggregate in dispersion.
Based on the experience of previous flooding experiments [11, 25], three nanoparticle concentrations (0.05, 0.2, and 0.5 wt.%) were used in this study. A 3 wt.% Sodium chloride (NaCl) brine was used as dispersion fluid for FNP and CNP, and n-decane was used as a dispersion fluid for FNP-O. For nanofluids preparation, solid powder nanoparticles were weighed, and then were mixed with dispersing fluid with a high-speed magnetic stirrer for 5–10 min and continued agitation by using a sonicator for 20–30 min at room temperature. The CNP nanofluids were diluted from a concentrated dispersion. Fluid properties of each hydrophilic nanofluids measured at room temperature are shown in Table 1. Nanoparticle size distribution in suspension was measured with dynamic light scattering (DLS) and are shown in Figure 3. The particle size for FNP and CNP is 153.3 and 17.5 nm, respectively. The results of DLS also were used to check the homogeneity of nanoparticles suspension.
Figure 3. Size distribution measured by DLS: (A) FNP; (B) CNP. The Y axis is light scattering intensity.
The oil used in this experiment was n-decane and crude oil from North Sea. The density was 0.73 and 0.82 g/ml, respectively. The API gravity value of crude oil was 41.06°.
Berea sandstone core plugs were used. Twenty short core plugs and six long core plugs drilled from the same block of Berea Sandstone were used for the wettability index (WI) measurement and nanoparticles transport experiments. The average porosity and permeability are 18.3% and 316 mD, respectively. The diameter was 3.83 cm and the length was 3 cm for the short cores and 8 cm for the long cores. All core plugs were cleaned with methanol before experiment.
Amott cells and centrifuge were used to measure WI of core.
Figure 4 shows schematic of coreflooding setup. The pump injected Exxol D-60 as pumping fluid to push the piston located inside the reservoir, which was filled with brine and nanofluids. The differential pressure across the core plug during nanoparticle transport experiments was recorded by precision pressure gauge.
Figure 4. Nanoparticles coreflooding apparatus. (1) Pump fluid (Exxol D60); (2) injection line; (3) Quzix Pump; (4) Valve; (5) Pump Fluid in reservoir; (6) Piston plate; (7) Brine; (8) Nanofluids; (9) Nanofluids; (10) Oil line; (11) Brine/Nanofluids line; (12) Bypass Valve; (13) Hassler Core Cell; (14) Core plug; (15) Pressure gauge; (16) Sleeve pressure; (17) connection cable; (18) Computer; (19) Accumulator; (20) electrical resistivity meter.
A total of eighteen short core plugs (designated as S3-S20) were treated with nanoparticles. Two cores (S1 and S2) were used for wettability index measurement of core without nanoparticle treatment. Three pairs of short cores (S3, S4; S5, S6 and S7, S8) were injected with three concentrations (0.05, 0.2, and 0.5 wt. %) of FNP nanofluids and another three pairs (S9, S10; S11, S12 and S13, S14) were injected with three concentrations (0.05, 0.2, and 0.5 wt. %) of CNP nanofluids. The rest of core plugs (S15, S16; S17, S18 and S19, S20) were injected with three concentrations (0.05, 0.2, and 0.5 wt. %) of FNP-O nanofluids. First, each pair of core plugs was saturated with nanofluids by using a vacuum container, and then the same nanofluids were injected into the core for 20 pore volumes (PV), inlet and outlet were exchanged at 10 PVs. Afterwards, deionized water/n-decane was injected to flush free nanoparticles out. Finally core plugs were dried in a heating cabinet and used for wettability index measurement.
The wettability indexes of all core plugs were measured at room temperature (25°C). The core treated with nanoparticles was saturated with n-decane and then placed into an Amott cell surrounding with brine. Brine was allowed to imbibe into the core displacing oil out until equilibrium was reached, and the volume of water imbibed (Vo1) was measured. Then the remaining oil in the core was reduced to residual oil saturation by using a centrifuge. The volume of oil displaced (Vo2) was determined by weight measurements. The core saturated with water at residual oil saturation was returned to the Amott cell and surrounded with n-decane. Oil was allowed to imbibe into the core displacing water out of core. The volume of water displaced (Vw1) was measured after equilibrium was reached. The remaining water in the core was forced out by displacement in a centrifuge. The volume of water displaced (Vw2) was measured. The wettability index of the core was defined and calculated by the following equation:
During brine imbibition process, oil production volume vs. time was measured to plot the spontaneous imbibition curve. The WI is a generally accepted quantitative measure of the wettability of a reservoir core sample. A WI of −1.0 indicates a strongly oil-wet core, whereas a WI of +1.0 indicates a strongly water-wet core.
Contact angle measurements were performed under room temperature (25°C) Small glass chips cut from microscope slides were used as the solid phase in this experiment. First, glass chips were cleaned and soaked in FNP or CNP nanofluids for about 1 day, and then the captive drop method [26] was applied to measure the contact angle between 3 wt.% brine and crude oil on glass chip. Three concentrations 0.05, 0.2, and 0.5 wt.% of nanofluids were used in this experiment. For each case three measurements were performed to obtain an average value. Using glass chips as substrate provides a smooth surface for contact angle measurement, since glass is 100% silicon dioxide so it cannot mimic other minerals in Berea sandstone.
Single phase nanofluids flooding was performed for nanoparticles transport experiment. Six long core plugs (8 cm length) were employed in this experiment for FNP and CNP. Different salt concentrations of brine were used as a tracer. First, core plugs were saturated with a 2.5 wt.% brine by using a vacuum pump and then injected with one PV of the same brine for measuring the absolute water permeability. Afterwards, about 4 PVs of nanofluids with 3 wt.% brine as dispersing phase were injected into the core plug to investigate the effect of nanoparticle adsorption and retention on permeability. Finally, 4 PVs of 2.5 wt.% brine were injected as a post-flush to observe the desorption of nanoparticles. A constant flow rate of 2 ml/min was used. The resistivity of the effluent was measured to determine the breakthrough time of the tracer. Differential pressure across the core was recorded during the whole injection process. Effluent fluid was collected every 4 ml (about 1/4 PV), and nanoparticle concentration was measured by using a UV-VIS spectrophotometer.
In this section, we first present the results of wettability alteration for core plugs treated with nanoparticles. One pair of core plugs was used for each case to test repeatability. The average wettability index for untreated core plugs (S1 and S2) was 0.795. Since all core plugs were drilled from the same block of Berea sandstone, we assumed that the original wettability index for all cores plugs before nanoparticles treatment was also 0.795. Based on wettability classification (Figure 5), the wettability for original core plugs was classified as water wet.
Figure 5. Wettability classification based on Amott-Harvey wettability index [27].
Three pairs of core plugs (S3, S4; S5, S6 and S7, S8) were treated with injection of FNP nanofluids with concentration of 0.05, 0.2, and 0.5 wt.%, and another three pairs of core plugs (S9, S10; S11, S12, and S13, S14) were treated with injection of CNP nanofluids with concentration of 0.05, 0.2, and 0.5 wt.%. After treatment with nanoparticles, core plugs were put into an Amott cell. During spontaneous imbibition oil production volume vs. time was recorded and spontaneous imbibition curves were plotted and are shown in Figure 6. Both FNP and CNP have similar spontaneous imbibition performance. Most of the oil was displaced at the beginning of the spontaneous imbibition, and oil volume remained constant when it reached to maximum. This meant that core plugs treated with hydrophilic nanofluids were still strongly water wet, and the oil was produced rapidly because of capillary force. However, compare to the core plugs without treatment, the core plugs treated with both FNP and CNP had slower spontaneous imbibition rate and less final oil production volume, which might due to permeability impairment during nanofluids injection and drying process. The results of wettability index measurement are shown in Figure 7 (detail data in Supplementary Tables 1, 2). There is no significant change of wettability observed for the core plugs treated with both FNP and CNP. For both 0.05 and 0.2 wt.% FNP and CNP cases, core plugs changed to slightly more water wet from original wetting state, because adsorption of hydrophilic nanoparticles on pore wall made core more water wet. For core plugs treated with 0.5 wt.% nanofluids, they should have the similar wettability index value with 0.05 and 0.2 wt.% cases, while the results showed that they were slightly less water wet than the original cores. This might be because of the permeability impairment (pore channels were plugged during drying process). Overall, there is no significant difference between the core plugs treated with hydrophilic CNP or FNP. Both have potential to alter the wettability of core plugs slightly to more water wet.
Figure 6. Spontaneous imbibition curves: (A) core plugs treated with FNP; (B) core plugs treated with CNP.
The rest of the three pairs of core plugs (S15 S16, S17 S18, and S19 S20) were treated with injection of hydrophobic FNP nanofluids with concentration 0.05, 0.2, and 0.5 wt.%, respectively. Spontaneous imbibition rate was also recorded and plotted in Figure 8. Unlike hydrophilic nanoparticle cases, the imbibition rate of core plugs treated with hydrophobic nanoparticle was very slow at beginning. For core plugs treated with 0.5 wt.% there was no oil production during first 40 min meaning they were oil wet at beginning. After some delay oil was produced at a low rate and gave the similar ultimate oil production volume as the hydrophilic nanoparticles cases (Figure 6). The wettability index measurement results of core plugs treated with hydrophobic nanoparticle are shown in Figure 9 (detail data in Supplementary Table 3). It can be seen that there was almost no change of wettability index after core treatment with hydrophobic nanoparticle. The reasons for this result and delay of spontaneous imbibition might be the adsorption of hydrophobic nanoparticle on water wet pore wall was weak. So some adsorbed nanoparticle desorbed and dispersed in decane, and those hydrophobic nanoparticles were transported out of core together with the oil. Thus, the wettability of core restored to what it was originally.
The contact angle between crude oil and brine on glass chips was measured and the results are shown in Figure 10. Glass chips were soaked in nanofluids for about 1 day before measurement. Unlike some contact angle measurements conducted before, in this experiment 3 wt.% brine was utilized as aqueous phase rather than nanofluids, which is similar to the condition of wettability index measurement. As shown in Figure 10, both soaking in FNP and CNP nanofluids reduced the contact angle and change the glass surface to more water wet. For CNP nanofluids, a concentration higher than 0.1 wt.% cannot reduce contact angle anymore, while for FNP nanofluids the higher the concentration of nanoparticles, the smaller was the contact angle. We also observed that for glass chips soaked in higher concentration nanofluids, due to nanoparticles adsorption, oil was not sticky on the glass surface anymore and an oil drop can easily slip on the surface. This indicated existence of a strongly hydrophilic surface.
Both wettability index and contact angle were measured at room temperature, which gave good stability to nanoparticles suspension but cannot show the performance of nanoparticles at realistic reservoir condition, for instance, high temperature may make nanoparticle suspension less stable.
Hydrophilic nanoparticles transport experiments were conducted to study nanoparticle adsorption behavior inside core and its influence on the permeability. Differential pressure across the core plug was recorded and effluent was collected during flooding. Effluent nanoparticle concentrations were measured after flooding. Six long core plugs were used in this experiment. Three of them were injected with FNP nanofluids with concentrations of 0.05, 0.2, and 0.5 wt.%. Another three long cores were injected by CNP nanofluids with concentrations of 0.05, 0.2, and 0.5 wt.%.
Figure 11 shows the differential pressure during nanoparticles transport experiments for FNP and CNP nanofluids with different concentrations. FNP and CNP nanofluids exhibited a totally different differential pressure behavior. For all FNP nanofluids injection (Figure 11A) the differential pressure increased after 1 PV of nanofluids injection and the higher the concentration, the faster differential pressure climbed. At the end of nanofluids injection, the pressure was still increasing and seemed far away from reaching its ultimate value. During the post-flush brine injection, due to residual nanofluids in inlet tubing and pores, differential pressure still increased to a high value and peaked until the end of injection. This indicated that nanoparticle retention cannot be flushed out of core and the higher concentration, the higher the plateau. Figure 11B shows that there is no big differential pressure change between CNP nanofluids injection and post-flush injection for all injection cases, which means that CNP adsorption does not impair permeability of the core. The ratio of permeability of core before nanofluids injection to after nanofluids injection was calculated and is shown in Table 2. FNP injection impairs permeability significantly at all concentrations, while CNP injection has no effect on permeability.
Figure 11. Differential pressure curves for nanoparticles transport experiment: (A) core plugs treated with FNP; (B) core plugs treated with CNP.
Dimensionless effluent nanoparticles concentration curves were plotted vs. time and are shown in Figure 12. The dimensionless nanoparticle concentration is defined as the ratio of effluent nanoparticle concentration to the injection nanoparticle concentration. Due to very weak responded signal during UV-VIS measurement, effluent nanoparticle concentration could not be measured for 0.05 wt.% CNP case. Therefore, the adsorption curve is absent from Figure 12B. In Figure 12A, nanoparticle concentration increased later than the tracer curve indicating nanoparticles adsorption and/or retention. After reaching the peak, FNP concentration started to decrease. It might be due to the “self-adsorption” of nanoparticles, which means that the previous adsorbed nanoparticles can adsorb nanoparticles injected afterwards resulting in multilayer adsorption and/or nanoparticles aggregation. If the nanoparticle aggregates are larger than one-seventh of the pore size [28], they can be retained in the porous medium thus causing plugging. This indicates that FNP adsorption is multilayer. In Figure 11A rapid differential pressure increase is observed after 2 PVs FNP nanofluids injection, which is another evidence of nanoparticles retention and core plugging. FNP concentration decreased rapidly during post-flush injection and earlier than the tracer curve indicating almost no desorption of nanoparticles. This is consistent with the results from differential pressure curve (no pressure decline during the post-flush in Figure 11A). In Figure 12B, CNP shows an almost ideal adsorption curve, where its concentration both increased and decreased later than tracer curve, indicating adsorption and desorption of CNP and effluent nanoparticle concentration kept at a plateau after nanoparticles adsorption on pore walls reached an equilibrium. It was observed that the 0.2 wt.% CNP curve increased and reached to plateau later than 0.5 wt.% CNP curve, which may indicate that amount of CNP adsorbed for the core plug is constant (Once pore walls of core were saturated with nanoparticles almost no nanoparticles will be adsorbed since adsorption and desorption reached a balance). So 0.2 wt.% CNP case takes a longer time to reach this amount. The CNP concentration decline curves for both concentrations are almost overlapped. Since the area between tracer and decline concentration curve indicate amount of nanoparticle desorbed, so the amount of CNP desorbed for the core plug is also constant (when adsorption and desorption reached an equilibrium).
Figure 12. Nanoparticles adsorption curves: (A) core plugs treated with FNP; (B) core plugs treated with CNP.
The percentage of nanoparticles adsorbed or trapped inside core plug was calculated for both FNP and CNP and is shown in Figure 13. More than 25% of FNP were adsorbed and trapped during the experiment, and the lower the concentration, the higher was the percentage. However, much less CNP was adsorbed compared to FNP. The adsorbed nanoparticles percentage of 0.2 wt.% case is 2.7 times that of the 0.5 wt.% case. Since this value is close to 2.5 (concentration ratio between 0.5 and 0.2 wt.%), it proves that adsorption capacity of the core plug for CNP is nearly constant and independent of concentration.
Hydrophilic nanoparticle adsorption and/or retention inside water wet core was observed in nanoparticle transport experiment. For FNP, it was easy to observe this phenomenon both with differential pressure curve and nanoparticle adsorption curve. However, since CNP injection did not influence differential pressure too much, so the adsorption and desorption of CNP could only be found in nanoparticle adsorption curve. This difference may indicate that two different kinds of nanoparticles have different adsorption behavior when they are injected into the core plug. This indirectly indicates that the adsorption and/or retention of FNP could be multilayer in nature whereas that of CNP is nearly monolayer.
In this study the effect of nanoparticle adsorption on wettability alteration was investigated by measuring wettability index of core plugs treated with nanoparticles and the contact angle between oil and brine on a glass surface treated with nanoparticles. Adsorption behavior of nanoparticle in porous media was investigated by coreflooding experiments with Berea sandstone core plugs. The results of wettability alteration experiment showed that treatment with hydrophilic nanoparticles altered wettability of sandstone slightly to more water wet and reduced the contact angle between oil and brine on a glass surface by about 10°. However, treatment with hydrophobic nanoparticles could not change wettability of core plug, but only delayed the spontaneous imbibition. For nanoparticle transport experiment, injection of FNP resulted in significant nanoparticle retention and permeability impairment, while injection of CNP had almost no influence on permeability. Nanoparticle adsorption curves indicated that FNP injection resulted in large amount of adsorption and/or retention, while no desorption was observed. However, compare to FNP cases, CNP injection only had less adsorption and significant desorption during post-flush was found. These results also suggested that FNP adsorption and/or retention inside core plug might be multilayer, while CNP adsorption is close to monolayer.
CNP has favorable injectivity into core plug compared with FNP, and it also has enough adsorption inside the core to alter wettability. FNP injection can change wettability to slightly more water wet, while a large amount of adsorption and/or retention result in unfavorable injectivity. FNP is not recommended for flooding without further surface modification. The wettability change during crude oil displacement with a nanofluid inside a core plug is interesting to investigate in the future.
SL: experimental work, results analysis, manuscript writing. OT: scientific advice, experimental suggestion, paper review. HL: paper review, scientific advice. NH: paper review. LS: scientific advice.
The authors declare that the research was conducted in the absence of any commercial or financial relationships that could be construed as a potential conflict of interest.
The authors would like to acknowledge Science and Engineering Research Council (SERC), A*STAR, Singapore for their financial support and the Research Council of Norway for their financial support through the Centers of Excellence funding scheme, project number 262644.
The Supplementary Material for this article can be found online at: https://www.frontiersin.org/articles/10.3389/fphy.2019.00074/full#supplementary-material
1. Norman RM. Wettability and its effect on oil recovery. J Petroleum Technol. (1990) 42:P1476. doi: 10.2118/21621-PA
2. Salehi M, Johnson SJ, Liang JT. Mechanistic study of wettability alteration using surfactants with applications in naturally fractured reservoirs. Langmuir. (2008) 24:14099–107. doi: 10.1021/la802464u
3. Zhang J, Nguyen QP, Flaaten AK, Pope GA. Mechanisms of enhanced natural imbibition with novel chemical. SPE Reservoir Eval. Eng. (2009) 12:912–20. doi: 10.2118/113453-PA
4. Hammond PS, Unsal E. Spontaneous imbibition of surfactant solution into an oil-wet capillary: wettability restoration by surfactant contaminant complexation. Langmuir. (2011) 27:4412–29. doi: 10.1021/la1048503
5. Karimi A, Fakhroueian Z, Bahramian A, Khiabani NP, Darabad JB, Azin R, et al. Wettability alteration in carbonates using zirconium oxide nanofluids: EOR implications. Energy Fuels. (2012) 26:1028–36. doi: 10.1021/ef201475u
6. Li S, Kaasa AT, Hendraningrat L, Torsæter. O. Effect of Silica Nanoparticles Adsorption on the Wettability Index of Berea Sandstone. In Presented at the International Symposium of the Society of Core Analysts (Napa valley, CA) (2013).
7. Ghadimi A, Saidur R, Metselaar HSC. A review of nanofuid stability properties and characterization in stationary conditions. Int J Heat Mass Transfer. (2011) 54:4051–68. doi: 10.1016/j.ijheatmasstransfer.2011.04.014
8. Rubilar O, Rai M, Tortella G, Diez MC, Seabra AB, Durán N. Biogenic nanoparticles: copper, copper oxides, copper sulphides, complex copper nanostructures and their applications. Biotechnol Lett. (2013) 35:1365–75. doi: 10.1007/s10529-013-1239-x
9. Tungittiplakorn W, Lion LW, Cohen C, Kim J. Engineered polymeric nanoparticles for soil remediation. Environ Sci Technol. (2004) 38:1605–10. doi: 10.1021/es0348997
10. Kong X, Ohadi MM. Applications of micro and nano technologies in the oil and gas industry - overview of the recent progress. In: Paper SPE 138241-MS Presented at Abu Dhabi International Petroleum Exhibition and Conference. Abu Dhabi (2010).
11. Li S, Hendraningrat L, Torsæter O. Improved oil recovery by hydrophilic silica nanoparticles suspension: 2-phase flow experimental studies. In: IPTC-16707-MS. Presented at the International Petroleum Technology Conference. Beijing (2013).
12. Miranda CR, De Lara LS, Tonetto BX. Stability and mobility of functionalized silica nanoparticles for enhanced oil recovery application. In: SPE 157033-MS presented at: SPE International Oilfield Nanotechnology Conference and Exhibition. Noordwijk (2012).
13. Li S, Hadia NJ, Lau HC, Torsaeter O, Stubbs LP, Ng QH. Silica nanoparticles suspension for enhanced oil recovery: stability behavior and flow visualization. In: Presented at the SPE Europec featured at the 80th EAGE Conference and Exhibition. Copenhagen (2018).
14. Bayet AE, Junin R, Samsuri A, Piroozian A, Hokmabadi M. Impact of metal oxide nanoparticles on enhanced oil recovery from limestone media at several temperatures. Energy Fuels. (2014) 28:6255–66. doi: 10.1021/ef5013616
15. Jiang R, Li K, Horne R. A mechanism study of wettability and interfacial tension for eor using silica nanoparticles. In: Presented at the SPE Annual Technical Conference and Exhibition. San Antonio, TX (2017). doi: 10.2118/187096-MS
16. Joonaki E, Ghanaatian S. The application of nanofluids for enhanced oil recovery: effects on interfacial tension and coreflooding process. Petroleum Sci Technol. (2014) 32:2599–607. doi: 10.1080/10916466.2013.855228
17. Lu T, Li Z, Zhou Y, Zhang C. Enhanced oil recovery of low-permeability cores by SiO2 nanofluid. Energy Fuels. (2017) 31:5612–21. doi: 10.1021/acs.energyfuels.7b00144
18. Wasan DT, Nikolov A. Spreading of nanofluids on solids. J Nat. (2003) 423:156–9. doi: 10.1038/nature01591
19. Chengara A, Nikolov A, Wasan DT, Trokhymchuk A, Henderson D. Spreading of nanofluids driven by the structural disjoining pressure gradient. J Colloid Interf Sci. (2004) 280:192–201. doi: 10.1016/j.jcis.2004.07.005
20. Wasan DT, Nikolov A, Kondiparty K. The wetting and spreading of nanofluids on solids: Role of the structural disjoining pressure. Curr Opin Colloid Interf Sci. (2011) 16:344–9. doi: 10.1016/j.cocis.2011.02.001
21. McElfresh P, Holcomb D, Ector D. Application of Nanofluid Technology to Improve Recovery in Oil and Gas Wells. In Presented at the SPE International Oilfield Nanotechnology Conference and Exhibition. Noordwijk (2012). doi: 10.2118/154827-MS
22. Kartic KC, Fogler HS. Migrations of Fines in Porous Media. Dordrecht: Kluwer Academic Publishers (1998).
23. Zhang T, Murphy M, Yu H, Bagaria HG, Huh C, Bryant SL. Investigation of Nanoparticle Adsorption during Transport in Porous Media. In: Paper was Prepared for Presentation at the SPE ATCE. New Orleans (2013). doi: 10.2118/166346-MS
24. Ju B, Dai S, Luan Z, Zhu T, Su X, Qiu X. A study of wettability and permeability change caused by adsorption of nanometer structured polysilicon on the surface of porous media. In: Presented at the SPE Asia Pacific Oil and Gas Conference and Exhibition. Melbourne, VIC (2002).
25. Li S, Genys M, Wang K, Torsæter O. Experimental study of wettability alteration during nanofluid enhanced oil recovery process and its effect on oil recovery. In: Presented at the SPE Reservoir Characterisation and Simulation Conference and Exhibition. Abu Dhabi (2015).
26. Baek Y, Kang J, Theato P, Yoon J. Measuring hydrophilicity of RO membranes by contact angles via sessile drop and captive bubble method: a comparative study. Desalination. (2012) 303:23–8. doi: 10.1016/j.desal.2012.07.006
27. Anderson WG. Wettability literature survey –Part 2: Wettability measurement. J Pet Technol. (1986) 38:1246–62. doi: 10.2118/13933-PA
Keywords: wettability alteration, nanoparticle, adsorption and desorption, transport, contact angle
Citation: Li S, Torsæter O, Lau HC, Hadia NJ and Stubbs LP (2019) The Impact of Nanoparticle Adsorption on Transport and Wettability Alteration in Water-Wet Berea Sandstone: An Experimental Study. Front. Phys. 7:74. doi: 10.3389/fphy.2019.00074
Received: 20 December 2018; Accepted: 24 April 2019;
Published: 14 May 2019.
Edited by:
Antonio F. Miguel, University of Evora, PortugalReviewed by:
Farid B. Cortés, Universidad Nacional de Colombia, ColombiaCopyright © 2019 Li, Torsæter, Lau, Hadia and Stubbs. This is an open-access article distributed under the terms of the Creative Commons Attribution License (CC BY). The use, distribution or reproduction in other forums is permitted, provided the original author(s) and the copyright owner(s) are credited and that the original publication in this journal is cited, in accordance with accepted academic practice. No use, distribution or reproduction is permitted which does not comply with these terms.
*Correspondence: Shidong Li, TGlfU2hpZG9uZ0BpY2VzLmEtc3Rhci5lZHUuc2c=
Disclaimer: All claims expressed in this article are solely those of the authors and do not necessarily represent those of their affiliated organizations, or those of the publisher, the editors and the reviewers. Any product that may be evaluated in this article or claim that may be made by its manufacturer is not guaranteed or endorsed by the publisher.
Research integrity at Frontiers
Learn more about the work of our research integrity team to safeguard the quality of each article we publish.