- 1Department of Chemistry, Nagoya University, Nagoya, Japan
- 2Research Center for Functional Materials, National Institute for Materials Science, Tsukuba, Japan
- 3Faculty of Pure and Applied Sciences, University of Tsukuba, Tsukuba, Japan
- 4Department of Physics, Tokyo Metropolitan University, Hachioji, Japan
We have investigated atomic and electronic structure of grain boundaries in monolayer MoS2, where relative angles between two different grains are 0 and 60 degree. The grain boundaries with specific relative angle have been formed with chemical vapor deposition (CVD) growth on graphite and hexagonal boron nitride flakes; van der Waals interlayer interaction between MoS2 and the flakes restricts the relative angle between two different grains of MoS2. Through scanning tunneling microscopy (STM) and spectroscopy measurements, we have found that the perfectly stitched structure between two different grains of MoS2 was realized in the case of the 0 degree grain boundary. We also found that even with the perfectly stitched structure, valence band maximum (VBM) and conduction band minimum (CBM) shows significant blue shift, which probably arise from lattice strain at the boundary.
Introduction
A post-graphene material, transition metal dichalcogenide (TMD), has recently attracted a great deal of attention. TMDs have a long research history, but research on properties of monolayer TMDs, three-atom-thick atomic layers, has only recently been started [1–3]. One of the most distinct in TMDs from graphene is that TMDs can have sizable bandgap (~2 eV), leading to electronic and optoelectronic applications of TMD atomic layers [4]. In fact, various TMD-based devices, including high-performance FET devices, light-emitting transistors, and photodetectors, have actually been demonstrated [5–7]. In conjunction with the flexibility arising from the ultrathin structure, flexible electronic and optoelectronic devices can also be made [8, 9]. In addition, monolayer TMDs in 2H form can have valley-degree-of-freedom, which may lead to future novel electronic devices based on valleytronics [10, 11].
For future applications of TMDs for electronic and optoelectronic devices, wafer-scale monolayer TMDs grown by chemical vapor deposition (CVD) are indispensable [12, 13]. Top-down approaches, such as mechanical exfoliation, are not compatible with wafer-scale monolayer TMDs, and a bottom-up approach is required for that purpose [14]. Crystal growth by CVD is a bottom-up approach to obtain thin films, having been successfully applied to grow various atomic layers, such as graphene, hexagonal boron nitrides (hBN), and TMDs [15–20]. In typical CVD growth of TMDs, solid sources such as metal oxides and elemental sulfur are used, and monolayer TMDs film with a lateral size of millimeters have been reported [19, 21]. Recently, the growth of TMDs by metal-organic CVD (MOCVD) with volatile liquid sources has been successfully demonstrated, and MOCVD is a promising method to realize wafer-scale TMDs that are compatible with device applications [22–24].
In CVD-grown large-area TMDs, grain boundaries (GBs) are inevitably formed, which can significantly alter the electronic and optical properties of TMDs [25–28]. During the CVD growth of TMDs, nuclei form at the beginning of the CVD process, growing to form a large-area continuous sheet of TMD with GBs. Because the orientation of nuclei is normally random, a wide variety of GB structures can be formed. For example, a GB with 7-5 and 8-4-4 membered rings forms in a CVD-grown MoS2, where midgap boundary states appear [25, 29–31]. The existence of GB-induced midgap states significantly affects electronic transport across the boundary, leading to reduction of carrier mobility via additional carrier scattering at the GB [25]. Therefore, control of GB structure by controlling grain orientation and understanding the boundary-oriented electronic structure provide a basis for the realization of future TMD-based devices.
In this work, we have focused on orientation-limited growth of a TMD and investigation of localized boundary states using scanning tunneling microscopy (STM) and scanning tunneling spectroscopy (STS); the STS is a powerful tool to investigate domain boundaries [32, 33]. The key for the successful control of crystal orientation in CVD growth of TMDs is the interaction between TMDs and the substrates used in CVD processes. In conventional CVD growth of TMDs, SiO2/Si substrates with amorphous surfaces are used, leading to random crystal orientations of grown TMDs. In contrast, substrates with crystalline structures can limit the crystal orientation of grown TMDs through TMD-substrate interactions [20, 29, 34, 35]. For the control of crystal orientation, we used hBN and graphite as substrates for CVD growth of TMDs. The atomically flat surfaces with three-fold (hBN) and six-fold rotation (graphite) symmetries successfully limited crystal orientations of grown TMD flakes; only two different orientations were observed. GBs between MoS2 flakes with different orientations (relative angle of 60°) shows boundary states localized at specific location near the Fermi level. On the other hand, a GB between MoS2 flakes with the same orientation shows a perfectly-stitched structure without any defects in scanned areas in STM images. We also found that both the conduction band minimum (CBM) and the valence band maximum (VBM) shift to the higher energy side at the GB even with a perfectly-stitched structure. This means that the GB state does not arise from defects but from strain at the GB, and strain formed at the growth process cannot be released even with the low friction coefficient between MoS2 and graphite.
Methodology
We grew monolayer MoS2 on hBN and graphite (Kish graphite, type C, Covalent Materials) flakes exfoliated on quartz substrates with a multi-furnace CVD apparatus. We prepared hBN and graphite flakes by the mechanical exfoliation method with adhesive tape (Scotch tape, 3M). As precursors for growth of MoS2, we used molybdenum trioxide (Sigma-Aldrich, 99.5% purity) and sulfur powder (Sigma-Aldrich, 99.98% purity). Furnace temperatures at the locations where molybdenum trioxide and elemental sulfur were placed were set to 1,029 K and 473 K, respectively, and the growth of MoS2 was carried out at 1,373 K for 20 min under Ar flow with a flow rate of 200 sccm. Atomic force microscope (AFM) observations were performed by the Veeco AFM system (Dimension 3100SPM, Nanoscope IV) operated at a scanning rate of 1 Hz. We measured photoluminescence (PL) spectra by a microspectroscopy system with a confocal microscope (Jobin Yvon HR-800, Horiba) with an excitation laser wavelength of 488 nm. For PL imaging, an LED light source (Mightex GCS-6500-15) was used to illuminate samples, and PL intensity (λ > 600 nm) was imaged with CCD (Princeton Instruments PIXIS-1024BR-eXelon). We formed electrical contacts to samples for STM/STS measurements by deposition of gold though a shadow mask or patterning conductive silver paste. After making the electrical contact, samples were introduced to an ultrahigh vacuum (UHV) environment and degassed at 473 K. The STM/STS measurements were conducted using a scanning tunneling microscope (Omicron LT–STM) in constant current mode operated at 90 K with an electrochemically etched W tip coated with PtIr (UNISOKU Co., Ltd.). A numerical derivative was used to acquire dI/dV curves, and WSxM software was used to process the STM images [36].
Results and Discussion
PL imaging and spectroscopy have clearly shown that the quality of the present samples is high. Figure S1 shows a typical PL image of MoS2/hBN and typical PL spectrum of MoS2/hBN and MoS2/graphite. As clearly seen, the PL image shows bright and uniform contrast, which clearly demonstrates high quality of samples we use. The observed FWHM values of PL spectra are 35~45 meV, which are much smaller than those of samples exfoliated onto SiO2 substrates [37, 38]. These PL spectra clearly demonstrate that quality of our sample is high.
The crystal orientations of MoS2 grown on hBN and graphite are limited to two orientations due to the van der Waals interactions between MoS2 and hBN. Figure 1A shows an AFM image of monolayer MoS2 crystals grown on a hBN flake. As clearly seen, all crystals possess a hexagonal shape with long and short facets, and their orientations are limited to only two different ones, where 60° rotation of one orientation matches the other orientation. The observed long and short facets in the crystals correspond to chalcogen and metal zigzag edges; the relationship between crystal shape and crystallographic orientation was investigated with transmission electron microscopy and electron diffraction (additional information is given in Figure S2). Figure 1B shows structural models of hexagonal MoS2 flakes with the two different orientations. The limited orientations of MoS2 flakes are also observed in MoS2 flakes grown on graphite substrates. This clearly demonstrates that the orientation-dependent potential arising from crystalline substrates is crucial to limiting the crystal orientation of grown MoS2.
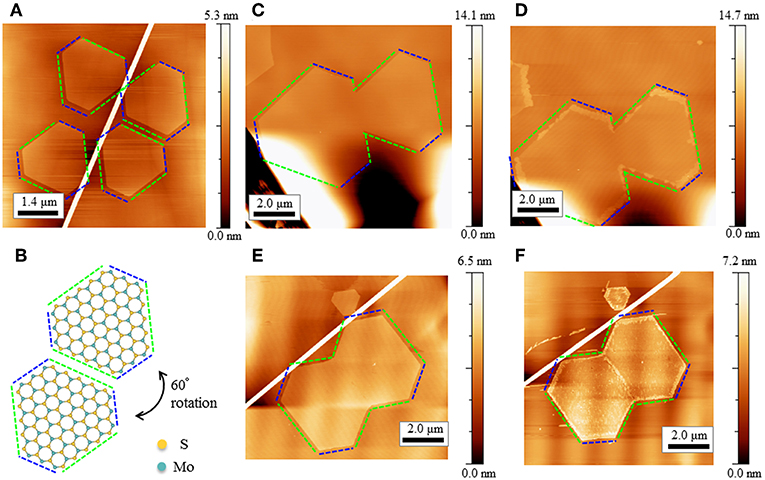
Figure 1. (A) An AFM image of monolayer MoS2 grown on h BN. Long and short edges of MoS2 flakes are marked by green and blue dotted line, respectively. (B) Structural model of the grown MoS2 with relative angle of 60°. (C,D) An AFM image of MoS2 before and after the oxidation. The relative angle between the two grains in this case is 0°. (E,F) An AFM image of MoS2 before and after the oxidation. The relative angle between the two grains in this case is 60°. White linear contrasts in Figures 1A,C,F are wrinkles in hBN flakes.
Because the crystal orientation of MoS2 on hBN is limited, the resulting structure of the GBs should also be limited: GBs between grains with the same orientation (GB-0°) and grains with 60° mutual orientation (GB-60°). To investigate if defects exist at such GBs, we investigated the reactivity for an oxidation reaction. MoS2 flakes with GBs were heated at 573 K under a flow of dry air. It has been shown that defects are sensitive to oxidation and reactions under the conditions above lead to the formation of oxides. Because oxidation from MoS2 to the corresponding oxides heightens the pristine structure, position-sensitive detection of oxidation of MoS2 can easily be done through AFM height images. Figures 1C–F are AFM images of pristine (oxidized) MoS2 flakes that have GB-0° and GB-60°, respectively. As clearly seen in Figures 1D,F, oxidation at Mo zigzag edges (shorter edges) is faster than that at S zigzag edges (longer edges) [39, 40]. We also found that GB-60° is oxidized as edges are oxidized, whereas GB-0° essentially retains its pristine structure. This means that GB-0° does not have defects that are sensitive to oxidation reactions, indicating that, unlike GB-60°, GB-0° has a well-stitched structure.
To investigate the structure and local electronic structure of GBs, we performed STM/STS measurements around the GBs. For this purpose, we use MoS2 grown on graphite, where the same orientation-limited growth of MoS2 occurs. Figure 2A is a STM image of a MoS2 grown on graphite, where positions of GB-0° are highlighted by arrows; we confirmed the monolayer structure by a line profile analysis at the edge (Figure S3). As can be seen, the GB-0° image is slightly darker than its peripheral place, which indicates that GB-0° has different local density of states from its peripheral place. For detailed investigations of structure and electronic structure, atomic-resolution STM observation of the GB-0° was carried out. Figure 2B is a STM image of the GB-0° at high magnification, showing the triangular array of sulfur atoms as bright spots. Based on a close inspection of the STM image, the misorientation angle between the two domains is almost zero (Figures S4, S5). GB-0° is imaged as slightly darker than its peripheral place at the middle of the STM topographic image, and we observed no defects at the GB-0°; neither vacancies nor insertion of atomic rows are seen. The well-stitched structure of GB-0° revealed by STM observation is consistent with its observed low reactivity toward oxidation reactions. It should be noted that a translational mismatch should exist at the boundary even with orientation matching between two grains. This result, however, clearly demonstrates that GB-0° has a stitched structure without defects, indicating that most of the translational mismatch can be relaxed through sliding on the graphite plane. The ultraflat surfaces of graphite and MoS2 may lead to ultralow friction between them, which should facilitate the sliding [41–43].
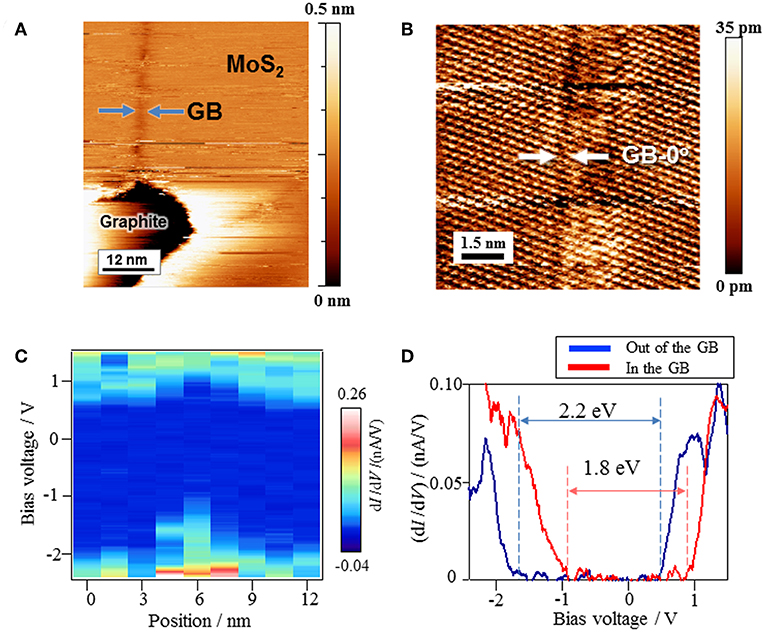
Figure 2. (A) An STM image of MoS2 grown on graphite. The blue arrows indicate the position of GB. (B) A magnified STM image around the GB-0°. The GB-0° can be imaged as a dark linear contrast, which are indicated by the arrow. (C) dI/dV mapping across the GB-0°. (D) STS spectra measured at a place out of the GB and on the GB.
As demonstrated by darker contrasts in the STM image, even though GB-0° has a well-stitched structure, the local electronic structure at GB-0° is different from that of its peripheral places. To see the differences in the electronic structures, we carried out STS and dI/dV mapping to visualize the local density of states. Figure 2C shows a dI/dV map across the GB-0°, which is located at a lateral position of around 6 nm in Figure 2C. As clearly seen in the figure, both CBM and VBM show upward shifts at the GB-0°. Figure 2D is a STS spectrum at GB-0°, showing that the upward shift at VBM (0.8 eV) is larger than that at CBM (0.4 eV). This results in a reduction of the bandgap at the GB-0° from 2.2 to 1.8 eV; the observed bandgap of pristine monolayer MoS2 (2.2 eV) is consistent with the value reported previously: 2.15–2.4 eV [44–46].
Because GB-0° has a stitched structure, this upward shift cannot be explained by formation of defects-mediated midgap states and can probably be explained by the local strain at the GB-0°. The bandgap of monolayer MoS2 is very sensitive to strain, and strain causes bandgap narrowing through upward/downward shift of VBM/CBM [46]. The observed modulation of bands, however, is upward shift in both CB and VB, which probably originates from accumulation of electrons at the boundary. This discrepancy can be understood if piezoelectric charge is taken into account [47]. As monolayer MoS2 has a non-centrosymmetric structure, the local strain can induce charge accumulation at the GB-0°, leading to the observed upward shift of CBM and VBM. One important implication is that a small strain, which probably arises from residual translational mismatch even after the sliding-based relaxation, remains at GB-0°, where the local electronic structure is strongly altered.
To investigate the degree of strain at GB-0°, we performed detailed image analyses with the high-resolution STM image shown in Figure 2B. Figure S4 shows a contrast-enhanced STM image after applying high-pass filter to filter out the low-frequency noise. It is clear that there are no atomic defects at GB-0°. A line profile along the yellow line clearly demonstrates that location of bright spots in the STM image align periodically without noticeable distortion. In addition, we performed fast Fourier transform (FTT) analysis on the STM image shown in Figure 2B. As shown in Figure S5, a FFT image at GB-0° shows spots with 6-fold symmetry, which is consistent with a triangular lattice of the sulfur array. The 6-fold symmetric pattern in the FFT image of GB-0° is almost identical to a FFT image at a corresponding peripheral place; line profiles along the green arrows in the FFT image at GB-0° and the peripheral place also coincide well. This means that the difference in lattice constants at the GB-0° and its peripheral place is less than the experimental resolution (2%). As discussed above, the observed difference in bandgap at GB-0° and peripheral places is 0.4 eV. Even though we assume that the difference in bandgap originates only from lattice strain, the strain should be comparable to the experimental resolution, and it is difficult to image the strain directly [44]. These analyses mean that small distortion less than the experimental resolution can remain at the GB-0°, and significant bandgap modulation can occur even in the case of GB-0°. This suggests that it is important to grow large single crystal of TMDs without any boundaries for future application with high-mobility TMD films.
In the case of GB-60°, structural defects exist and the local electronic structure is strongly modified. Figure 3A is a STM image of MoS2 on graphite near the GB-60°. The vertical linear contrast at the middle of the image corresponds to an impurity attached at GB-60°, where strong binding sites for impurities should exist. Figure 3B and Figure S6 are magnified STM images of clean GB-60°, where the atomic structure can be seen. Based on close investigation of the STM image, we found that the angle between GB-60° and the zigzag edge of MoS2 is about 20°. Figure 3C shows the STS spectral mapping along the blue line in Figure 3B. As clearly seen in Figure 3C, the electronic structure is significantly modulated at GB-60°, where both CBM and VBM upshift to reduce the bandgap from 2.3 to 1.9 eV. To investigate the spatial distribution of the boundary state at GB-60°, we performed dI/dV mapping at three different bias voltages of 0.83, −0.78, and −1.54 V. Figures 3D–F show the observed dI/dV mappings at bias voltages of 0.83, −0.78, and −1.54 V, respectively. As clearly seen, the boundary state strongly localizes at GB-60°. In addition, the boundary state corresponding to a bias voltage of −0.78 V shows a dotted distribution rather than a linear uniform distribution, and this means that the boundary state originates from a specific defect site existing at the GB-60°.
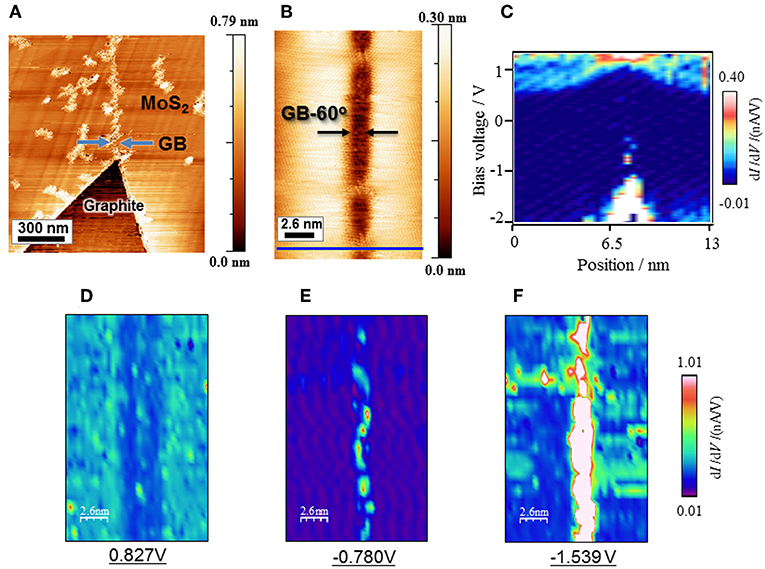
Figure 3. (A) An STM image of MoS2 grown on graphite with a grain boundary of 60°. (B) A magnified STM image around the GB-60°. (C) dI/dV mapping across the GB-60° measured along the blue line in (B). (D–F) STS spectral image of the GB-60° measured with different bias voltage of 0.827, −0.780, and −1.539 V, respectively.
Conclusion
In this paper, electronic properties and defect densities in two types of GBs in MoS2 grown by the CVD process were investigated. The orientations of MoS2 grown on hBN and graphite by the CVD process are limited to two directions and the misorientation angles of the two flakes are 0° and 60°. It is confirmed that two grains are stitched completely in the GB-0°, but have an upshift of band structure due to the local stress and charge accumulation. In the GB-60°, the structure of GB is clearly imaged by STM/STS without the disturbance of adsorbates on GB. The band structure in GB-60° upshifts and localized states appear. In the case of two grains of MoS2 stitched at the same angle, the electronic structure of GB-0° is modified due to local stress and carrier accumulation. It will be a challenge to make a MoS2 sheet without modulation of the electronic state or the structure.
Author Contributions
TN prepared MoS2 samples. TN, KM, and SY performed STM/STS measurements. YK and YM performed optical characterizations of MoS2 samples. TT and KW synthesized hBN crystals. RK designed experiments and wrote the paper. OT, HidS, and HisS discussed the results and reviewed the manuscript.
Funding
This work was supported by JSPS KAKENHI Grant numbers JP16H06331, JP16H03825, JP16H00963, JP15K13283, JP25107002, and JST CREST Grant Number JPMJCR16F3. KW and TT acknowledge support from the Elemental Strategy Initiative conducted by the MEXT, Japan and the CREST (JPMJCR15F3), JST.
Conflict of Interest Statement
The authors declare that the research was conducted in the absence of any commercial or financial relationships that could be construed as a potential conflict of interest.
Supplementary Material
The Supplementary Material for this article can be found online at: https://www.frontiersin.org/articles/10.3389/fphy.2019.00059/full#supplementary-material
References
2. Radisavljevic B, Radenovic A, Brivio J, Giacometti V, Kis A. Single-layer MoS2 transistors. Nat Nanotechnol. (2011) 6:147–50. doi: 10.1038/nnano.2010.279
3. Mak KF, Lee C, Hone J, Shan J, Heinz TF. Atomically thin MoS2: a new direct-gap semiconductor. Phys Rev Lett. (2010) 105:136805. doi: 10.1103/PhysRevLett.105.136805
4. Liu GB, Xiao D, Yao YG, Xu XD, Yao W. Electronic structures and theoretical modelling of two-dimensional group-VIB transition metal dichalcogenides. Chem Soc Rev. (2015) 44:2643–63. doi: 10.1039/C4CS00301B
5. Pu J, Takenobu T. Monolayer transition metal dichalcogenides as light sources. Adv Mater. (2018) 30:1707627. doi: 10.1002/adma.201707627
6. Wang QH, Kalantar-Zadeh K, Kis A, Coleman JN, Strano MS. Electronics and optoelectronics of two-dimensional transition metal dichalcogenides. Nat Nanotechnol. (2012) 7:699–712. doi: 10.1038/nnano.2012.193
7. Jariwala D, Sangwan VK, Lauhon LJ, Marks TJ, Hersam MC. Emerging device applications for semiconducting two-dimensional transition metal dichalcogenides. Acs Nano. (2014) 8:1102–20. doi: 10.1021/nn500064s
8. Lee GH, Yu YJ, Cui X, Petrone N, Lee CH, Choi MS, et al. Flexible and transparent MoS2 field-effect transistors on hexagonal boron nitride-graphene heterostructures. Acs Nano. (2013) 7:7931–6. doi: 10.1021/nn402954e
9. Pu J, Yomogida Y, Liu KK, Li LJ, Iwasa Y, Takenobu T. Highly flexible MoS2 thin-film transistors with Ion gel dielectrics. Nano Lett. (2012) 12:4013–7. doi: 10.1021/nl301335q
10. Xiao D, Liu GB, Feng WX, Xu XD, Yao W. Coupled spin and valley physics in monolayers of MoS2 and other group-VI dichalcogenides. Phys Rev Lett. (2012) 108:196802. doi: 10.1103/PhysRevLett.108.196802
11. Schaibley JR, Yu HY, Clark G, Rivera P, Ross JS, Seyler KL, et al. Valleytronics in 2D materials. Nat Rev Mater. (2016) 1:16055. doi: 10.1038/natrevmats.2016.55
12. Kitaura R, Miyata Y, Xiang R, Hone J, Kong J, Ruoff RS, et al. Chemical vapor deposition growth of graphene and related materials. J Phys Soc Jpn. (2015) 84:121013. doi: 10.7566/JPSJ.84.121013
13. Shi YM, Li HN, Li LJ. Recent advances in controlled synthesis of two-dimensional transition metal dichalcogenides via vapour deposition techniques. Chem Soc Rev. (2015) 44:2744–56. doi: 10.1039/C4CS00256C
14. Novoselov KS, Jiang D, Schedin F, Booth TJ, Khotkevich VV, Morozov SV, et al. Two-dimensional atomic crystals. Proc Natl Acad Sci USA. (2005) 102:10451–3. doi: 10.1073/pnas.0502848102
15. Lee YH, Zhang XQ, Zhang WJ, Chang MT, Lin CT, Chang KD, et al. Synthesis of large-area MoS2 atomic layers with chemical vapor deposition. Adv Mater. (2012) 24:2320–5. doi: 10.1002/adma.201104798
16. Reina A, Jia XT, Ho J, Nezich D, Son HB, Bulovic V, et al. Large area, few-layer graphene films on arbitrary substrates by chemical vapor deposition. Nano Lett. (2009) 9:30–5. doi: 10.1021/nl801827v
17. Sinha S, Takabayashi Y, Shinohara H, Kitaura R. Simple fabrication of air-stable black phosphorus heterostructures with large-area hBN sheets grown by chemical vapor deposition method. 2d Mater. (2016) 3:035010. doi: 10.1088/2053-1583/3/3/035010
18. Okada M, Miyauchi Y, Matsuda K, Taniguchi T, Watanabe K, Shinohara H, et al. Observation of biexcitonic emission at extremely low power density in tungsten disulfide atomic layers grown on hexagonal boron nitride. Sci Rep. (2017) 7:322. doi: 10.1038/s41598-017-00068-0
19. Kobayashi Y, Sasaki S, Mori S, Hibino H, Liu Z, Watanabe K, et al. Growth and optical properties of high-quality monolayer WS2 on graphite. Acs Nano. (2015) 9:4056–63. doi: 10.1021/acsnano.5b00103
20. Okada M, Sawazaki T, Watanabe K, Taniguch T, Hibino H, Shinohara H, et al. Direct chemical vapor deposition growth of WS2 atomic layers on hexagonal boron nitride. Acs Nano. (2014) 8:8273–7. doi: 10.1021/nn503093k
21. Zhan YJ, Liu Z, Najmaei S, Ajayan PM, Lou J. Large-area vapor-phase growth and characterization of MoS2 atomic layers on a SiO2 substrate. Small. (2012) 8:966–71. doi: 10.1002/smll.201102654
22. Eichfeld SM, Hossain L, Lin YC, Piasecki AF, Kupp B, Birdwell AG, et al. Highly scalable, atomically thin WSe2 grown via metal-organic chemical vapor deposition. Acs Nano. (2015) 9:2080–7. doi: 10.1021/nn5073286
23. Kim H, Ovchinnikov D, Deiana D, Unuchek D, Kis A. Suppressing nucleation in metal-organic chemical vapor deposition of MoS2 monolayers by alkali metal halides. Nano Lett. (2017) 17:5056–63. doi: 10.1021/acs.nanolett.7b02311
24. Kang K, Xie SE, Huang LJ, Han YM, Huang PY, Mak KF, et al. High-mobility three-atom-thick semiconducting films with wafer-scale homogeneity. Nature. (2015) 520:656–60. doi: 10.1038/nature14417
25. van der Zande M, Huang PY, Chenet DA, Berkelbach TC, You YM, Lee GH, et al. Grains and grain boundaries in highly crystalline monolayer molybdenum disulphide. Nat Mater. (2013) 12:554–61. doi: 10.1038/nmat3633
26. Ji HG, Lin YC, Nagashio K, Maruyama M, Solis-Fernandez P, Aji AS, et al. Hydrogen-assisted epitaxial growth of monolayer tungsten disulfide and seamless grain stitching. Chem Mater. (2018) 30:403–11. doi: 10.1021/acs.chemmater.7b04149
27. Elibol K, Susi T, O'Brien M, Bayer BC, Pennycook TJ, McEvoy N, et al. Grain boundary-mediated nanopores in molybdenum disulfide grown by chemical vapor deposition. Nanoscale. (2017) 9:1591–8. doi: 10.1039/C6NR08958E
28. Karvonen L, Saynatjoki A, Huttunen MJ, Autere A, Amirsolaimani B, Li S, et al. Rapid visualization of grain boundaries in monolayer MoS2 by multiphoton microscopy. Nat Commun. (2017) 8:15714. doi: 10.1038/ncomms15714
29. Yu H, Yang ZZ, Du LJ, Zhang J, Shi JN, Chen W, et al. Precisely aligned monolayer MoS2 epitaxially grown on h-BN basal plane. Small. (2017) 13:1603005. doi: 10.1002/smll.201603005
30. Najmaei S, Liu Z, Zhou W, Zou XL, Shi G, Lei SD, et al. Vapour phase growth and grain boundary structure of molybdenum disulphide atomic layers. Nat Mater. (2013) 12:754–9. doi: 10.1038/nmat3673
31. Zhou W, Zou XL, Najmaei S, Liu Z, Shi YM, Kong J, et al. Intrinsic structural defects in monolayer molybdenum disulfide. Nano Lett. (2013) 13:2615–22. doi: 10.1021/nl4007479
32. Nilius N, Kulawik M, Rust HP, Freund HJ. Defect-induced gap states in Al2O3 thin films on NiAl(110). Phys Rev B. (2004) 69:1214011. doi: 10.1103/PhysRevB.69.121401
33. Schmid M, Shishkin M, Kresse G, Napetschnig E, Varga P, Kulawik M, et al. Oxygen-deficient line defects in an ultrathin aluminum oxide film. Phys Rev Lett. (2006) 97:046101. doi: 10.1103/PhysRevLett.97.046101
34. Hotta T, Tokuda T, Zhao S, Watanabe K, Taniguchi T, Shinohara H, et al. Molecular beam epitaxy growth of monolayer niobium diselenide flakes. Appl Phys Lett. (2016) 109:133101. doi: 10.1063/1.4963178
35. Ago H, Endo H, Solis-Fernandez P, Takizawa R, Ohta Y, Fujita Y, et al. Controlled van der Waals epitaxy of mono layer MoS2 triangular domains on graphene. Acs Appl Mater Inter. (2015) 7:5265–73. doi: 10.1021/am508569m
36. Horcas I, Fernandez R, Gomez-Rodriguez JM, Colchero J, Gomez-Herrero J, Baro AM. WSXM: a software for scanning probe microscopy and a tool for nanotechnology. Rev Sci Instrum. (2007) 78:013705. doi: 10.1063/1.2432410
37. Zafar A, Nan HY, Zafar Z, Wu ZT, Jiang J, You YM, et al. Probing the intrinsic optical quality of CVD grown MoS2. Nano Res. (2017) 10:1608–17. doi: 10.1007/s12274-016-1319-z
38. Kaplan D, Gong Y, Mills K, Swaminathan V, Ajayan PM, Shirodkar S, et al. Excitation intensity dependence of photoluminescence from monolayers of MoS2 and WS2/MoS2 heterostructures. 2d Mater. (2016) 3:015005. doi: 10.1088/2053-1583/3/1/015005
39. Yamamoto M, Dutta S, Aikawa S, Nakaharai S, Wakabayashi K, Fuhrer MS, et al. Self-limiting layer-by-layer oxidation of atomically thin WSe2. Nano Lett. (2015) 15:2067–73. doi: 10.1021/nl5049753
40. Lauritsen JV, Kibsgaard J, Helveg S, Topsoe H, Clausen BS, Laegsgaard E, et al. Size-dependent structure of MoS2 nanocrystals. Nat Nanotechnol. (2007) 2:53–8. doi: 10.1038/nnano.2006.171
41. Kobayashi Y, Taniguchi T, Watanabe K, Maniwa Y, Miyata Y. Slidable atomic layers in van der Waals heterostructures. Appl Phys Express. (2017) 10:045201. doi: 10.7567/APEX.10.045201
42. Mandelli D, Leven I, Hod O, Urbakh M. Sliding friction of graphene/hexagonal -boron nitride heterojunctions: a route to robust superlubricity. Sci Rep UK. (2017) 7:10851. doi: 10.1038/s41598-017-10522-8
43. Wang LF, Zhou X, Ma TB, Liu DM, Gao L, Li X, et al. Superlubricity of a graphene/MoS2 heterostructure: a combined experimental and DFT study. Nanoscale. (2017) 9:10846–53. doi: 10.1039/C7NR01451A
44. Li H, Contryman AW, Qian XF, Ardakani SM, Gong YJ, Wang XL, et al. Optoelectronic crystal of artificial atoms in strain-textured molybdenum disulphide. Nat Commun. (2015) 6:7381. doi: 10.1038/ncomms8381
45. Liu XL, Balla I, Bergeron H, Hersam MC. Point defects and grain boundaries in rotationally commensurate MoS2 on epitaxial graphene. J Phys Chem C. (2016) 120:20798–805. doi: 10.1021/acs.jpcc.6b02073
46. Huang YL, Chen YF, Zhang WJ, Quek SY, Chen CH, Li LJ, et al. Bandgap tunability at single-layer molybdenum disulphide grain boundaries. Nat Commun. (2015) 6:6298. doi: 10.1038/ncomms7298
Keywords: grain boundaries, transition metal dichalchogenides, scanning tunneling microscopy, boundary states, chemical vapor deposition (CVD)
Citation: Nakanishi T, Yoshida S, Murase K, Takeuchi O, Taniguchi T, Watanabe K, Shigekawa H, Kobayashi Y, Miyata Y, Shinohara H and Kitaura R (2019) The Atomic and Electronic Structure of 0° and 60° Grain Boundaries in MoS2. Front. Phys. 7:59. doi: 10.3389/fphy.2019.00059
Received: 25 January 2019; Accepted: 27 March 2019;
Published: 17 April 2019.
Edited by:
Sabina Botti, Italian National Agency for New Technologies, Energy and Sustainable Economic Development (ENEA), ItalyReviewed by:
Fausto Sirotti, École Polytechnique, FranceNiklas Nilius, University of Oldenburg, Germany
Copyright © 2019 Nakanishi, Yoshida, Murase, Takeuchi, Taniguchi, Watanabe, Shigekawa, Kobayashi, Miyata, Shinohara and Kitaura. This is an open-access article distributed under the terms of the Creative Commons Attribution License (CC BY). The use, distribution or reproduction in other forums is permitted, provided the original author(s) and the copyright owner(s) are credited and that the original publication in this journal is cited, in accordance with accepted academic practice. No use, distribution or reproduction is permitted which does not comply with these terms.
*Correspondence: Ryo Kitaura, ci5raXRhdXJhQG5hZ295YS11Lmpw