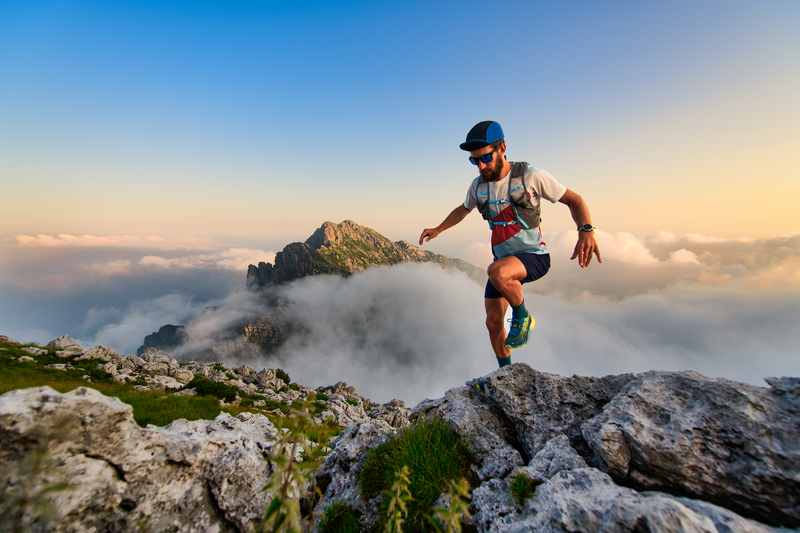
95% of researchers rate our articles as excellent or good
Learn more about the work of our research integrity team to safeguard the quality of each article we publish.
Find out more
OPINION article
Front. Pharmacol. , 16 October 2020
Sec. Inflammation Pharmacology
Volume 11 - 2020 | https://doi.org/10.3389/fphar.2020.570698
This article is part of the Research Topic Coronavirus Disease (COVID-19): Molecular Mechanisms, Translational Approaches and Therapeutics View all 118 articles
The recent outbreak of the COVID-19 has posed an unprecedented challenge to the health care system. Effective and immediate therapeutics are urgently required to control SARS-CoV-2 infection, which is manifested by aberrant immunopathology. Hyper activation of macrophages and neutrophils contributes to acute respiratory distress syndrome, respiratory failure, and subsequent death of COVID-19 cases. Due to the short life span of neutrophils, tempering macrophage plasticity is relevant for the management of COVID-19 cases. In this context, we here propose that either exchange or in situ reprogramming of derailed Th17+ alveolar macrophages/ Slan+ DC with Th1 programmed counterpart would potentially mitigate or abolish pulmonary fibrosis. This is a new pathology, and our knowledge about this disease is limited, therefore, a great deal of work is required before any therapeutics/vaccine could potentially be launched for its eradication. Several recent and compelling studies have proposed several aspects of the pathological basis of the disease (Zhou F. et al., 2020), which enhanced have our understanding of how SARS-CoV-2 is interacting with alveolar and peripheral tissues and causing the death of some infected patients.
SARS-CoV-2 induces pathogenic inflammation, pulmonary fibrosis, acute respiratory distress syndrome (ARDS), and nephropathy which can lead to the death of some infected patients within 2-3 weeks (Zhou F. et al., 2020). Hyper-activation of committed macrophages, in what is termed macrophage activation syndrome (MAS) (Giamarellos-Bourboulis et al., 2020), is associated with ARDS (Konig et al., 2020), which causes respiratory failure and in some cases the death of COVID-19 patients. Autopsy reports and single-cell RNA sequencing (Liao et al., 2020) have revealed the presence of monocyte‐derived FCN1 + macrophages and other immune cells like neutrophils (Liao et al., 2020; Yao et al., 2020) in the bronchoalveolar lavage fluid (BALF) of COVID‐19 patients with severe ARDS. However, due to the abundance of macrophages over other immune cells and MAS in COVID 19 patients, these cells seem to be important for pathogenesis.
Transcriptional analysis of BALF further revealed high levels of IFN‐ϒ induced protein-10 (IP‐10) and various chemokines like MCP‐1, CCL2, and CCL7 in BALF of severe COVID-19 patients, which correlated with increased infiltration of monocyte/Macrophages (Xiong et al., 2020) in their lungs. Cytokine profiling of critical COVID-19 patients reflects MAS with high levels of pro-inflammatory cytokines and chemokines such as IL-6, IL-7, Tumour Necrosis Factor (TNF-α), CCL2, CCL3, CXCL10, and IL-2 receptor. This demonstrates dysregulated activation of the mononuclear phagocyte system, hyper-inflammatory response (Mehta et al., 2020), and MAS (Schulert and Grom, 2015) which ultimately leads to the death of patients.
Apart from macrophages, the activation of neutrophils has also been associated with ARDS and cytokine storm in COVID-19 patients. Cytokine profiling, blood clots, and MPO activity in patients reveal infiltration of activated neutrophils in the lungs, which is indicative of early defense mechanism against SARS – CoV-2 virus. This was made evident by the presence of Neutrophils extracellular traps (NET), which are used by activated neutrophils to trap the pathogens. Excessive infiltration and activation of neutrophils (Barnes et al., 2020) are manifested in neutrophilia, which is sufficient to predict poor outcomes in COVID-19 cases. Furthermore, the neutrophil-to-lymphocyte ratio has been associated with a high-risk factor for severe disease (Zhang et al., 2020). The accumulation of neutrophils in the lungs of COVID-19 patients was associated with acute capillaritis with fibrin deposition, extravasation of neutrophils into the alveolar space, and neutrophilic mucositis (Zhang et al., 2020). These findings potentially indicate the role of activated neutrophils also in the pathogenesis of COVID-19 cases.
During the early phase of infection in COVID-19 patients, NETs activate macrophages to secrete IL-1 and TGF beta, further activating neutrophils infiltration and activation. Therefore, both macrophages and neutrophils represent potential targets for interventions. However, due to the sensitive nature of neutrophils for ROS, RNI mediated death during pneumonitis, and pulmonary fibrosis, these cells undergo death. In COVID-19 patients, these dead neutrophils are then phagocytosed by the surrounding inflammatory macrophages, which induce the release of IL-1 beta and TGF beta, inducing the anti-inflammatory programming of macrophages (Greenlee-Wacker, 2016) and Th2 programming of the pulmonary compartment. The macrophage remains active and viable for an extended period over the neutrophils and is likely to be more proficient than neutrophils in controlling SARS-CoV-2 infection. Due to this activation and lack of neutrophils, interventions targeting these cells are particularly challenging at the moment. Therefore, macrophages are a prudent target and approach to controlling the infection.
Both activation and increased infiltration of macrophages (Giamarellos-Bourboulis et al., 2020; Wang et al., 2020) and neutrophils (Morris et al., 2020; Middleton et al., 2020) during infection leads to ARDS and cytokine storm in COVID-19 patients. During SARS-CoV-2 infection, activated CD4+ T lymphocytes secrete granulocyte-macrophage colony-stimulating factor which further stimulates committed macrophage to secrete pro-inflammatory cytokines, thereby continuing the vicious cycle of the cytokine storm.
SARS‐CoV‐2 activates alveolar, splenic, and renal macrophages through angiotensin-converting enzyme 2 (ACE2) and enhances the secretion of IL‐6, TNF-α, IL‐10, and PD‐1 (Wang et al., 2020). Both ARDS and MAS contribute to the significant depletion of CD8+ CTLs which are associated with disease severity (Luo et al., 2020) in COVID-19 patients. SARS-CoV-2 infects type 2 alveolar cells, epithelial cells, and podocytes in the lungs and kidney respectively and interacts with them via ACE2 receptors which facilitate the attachment and entry of this virus into host cells (Hoffmann et al., 2020; Wrapp et al., 2020). ACE-2/Angiotensin-II receptors are known to activate the Sphingosine-1-phosphate receptor 1 (S-1P receptor 1) which is known to mediate IL-6 induced myopathy and fibrosis (Ohkura et al., 2017). S-1P R-1 signaling is associated with Th2/17 responses (Schroder et al., 2011; Schulze et al., 2011), hypoxia, and allergic manifestations (Saluja et al., 2017), which altogether promote Th2 bias in the infected patients. Therefore it is rational to presume that the S-1P receptor 1 signaling would enhance the pathogenesis of COVID-19 cases. Taking this into account, blocking S-1P signaling either by application of S-1P lyase (Vijayan et al., 2017) or FTY-720/Fingolimod (Penuelas-Rivas et al., 2005; Papadopoulos et al., 2010), could modulate the pathogenesis of the novel COVID-19 disease. Considering this, FTY-720 is being explored in a Phase-2 clinical trial of COVID-19 patients (NCT04280588, MRCTA, ECFAH of FMU), the results of which have not yet been published. However, due to its immunosuppressive nature, FTY-720 is expected to only lower the hyper-inflammatory response, providing symptomatic relief in COVID-19 cases but not the clearance of the SARS-CoV-2 infection (Walsh et al., 2010).
Like any of the other new interventions that have been proposed since the start of the pandemic, validating a new hypothesis in a pre-clinical model is essential before its clinical application in patients. Both inbred Balb/c & C57BL/6 mice represent a suitable model of testing vaccines, antiviral drugs, and disease pathogenesis (Subbarao and Roberts, 2006). Furthermore, due to the dependency of SARS-CoV-2 on Ace-2 (Imai et al., 2005), Tmprss2 (Graham et al., 2012), and Stat-1 (Zhou P. et al., 2020) protein for manifesting disease, knockout mice of these proteins also represent potential models for studying COVID-19 disease. Most interestingly, the floxed strain of these mice could be used and backcrossed with LysM Cre mice to address macrophages related phenotype. To demonstrate the anti-COVID 19 potentials of M1 macrophages, the most suitable strategy would be to either use clinical isolate of SARS-CoV-2 or express major spike proteins of this virus in the VeroE6 cell line and co-culture them with THP/CD14+/CD11b+ macrophages to evaluate the influence of the various adjuvants (Table 1 and Figure 1) on the innate and adaptive immune response of both macrophages and CD8+CTL. Several aspects like maturation, the M1/Th1 programming of macrophages, and subsequent killing of the virus or expression of Spike proteins can be used to correlate the M1 programming of RAW, THP, CD14+, and CD11b+ primary macrophages. T cell response could be measured by co-culturing T cells with infected epithelial and macrophages co-culture. Various parameters like TARC/CCL17, RANTES STAT-1, 6, calcineurin, IRF - 4, and 8 factors Th1 and Th17 response could be evaluated to address the impact of M1 programmed macrophage on T cell activation and killing off infected epithelial cells. These preclinical experiments are essential for validating the anti-COVID-19 impact of M1 and CD8+CTL. These approaches represent the most suitable ways of proving the concept at preclinical levels and need further investigation.
Table 1 Clinical trials targeting Activated macrophages for indicated inflammatory and tumor Diseases.
Figure 1 Schematic representation of augmenting SARS-Co-V2 specific adaptive immunity. Shown here are the most potent immune mediate (perspective) and/or pharmaceutical/palliative approaches for augmenting adaptive immune responses of derailed Macrophages or Slan DC for tuning effector T cell response for the treatment and recovery of COVID-19 patients. These approaches are a paramount requirement (in conjunction with other therapies) and are expected to help in managing COVID-19 disease.
Given the above facts, mitigating pathogenic inflammation (Th2/17 responses) and pulmonary fibrosis are paramount for the management of COVID-19 infection. In this context, our previous studies have demonstrated the potential significance of iNOS+M1 effector macrophages and CD8+CTL in controlling respiratory pathogens (Schulert and Grom, 2015; Mehta et al., 2020; Xiong et al., 2020), which are associated with community-acquired pneumonia. Based on these studies, we believe that tweaking these effector immune cells may be also sufficient to control the replication of SARS-CoV2 infection in COVID-19 cases. This could be achieved through in situ reprogramming of Th2 and Th17 programmed macrophages or by exchanging Th2/17 programmed macrophages with Th1 primed iNOS+CD14+M1 macrophages and CD8+CTL, which are in the neo-adjuvant setting. Based on our previous studies (Barnes et al., 2020; Zhang et al., 2020), we also believe that the exchange of Th17+ macrophages with Th1+ M1 macrophages may provide protective immunity against SARS-CoV-2 infection in cases of COVID-19. This approach could improve the prospects of severely infected patients but needs to be commissioned and explored further before clinical application.
While the application of antiviral drugs like Remedesvir and Tocilizumab is sufficient to control the infection, it is still questionable whether these drugs are adequate to augment immunogenic inflammation and clear infected cells from the infected organ. In this context, we propose the potential role of Ceramide-1 phosphate as immune adjuvants for promoting the Th1 programming of infected cells/tissue and subsequent clearance of virus infected cells from the tissue. Given the potential involvement of S-1P in fibrosis and Th1 bias, FTY-720 based interventions are presently under Phase-2nd clinical trial (NCT04280588) based on trapping effector T cells in the lymph node for effective control of the infection and mitigating cytokine storm. However, due to its immunosuppressive nature, we believe that FTY-720 may not help patients. Other studies have also highlighted the significance of the BCG vaccination and dexamethasone on increased resistance to the infection (Escobar et al., 2020) and reduced mortality (Lammers et al., 2020) in COVID-19 patients. Since these interventions are targeted to reduce the inflammatory score and are anticipated to provide only symptomatic relief, other more robust and stable interventions are required for managing the disease.
Several of the reports mentioned above have provided compelling evidence that hyper-activated macrophages are critical for ARDS and in causing the subsequent death of patients. It is worth considering combining macrophage directed strategies with other available approaches (as adjuncts), which may be decisive for the effective management of severely infected cases. Out of several available options, the most relevant strategy would be to promote in situ reprogramming of Th2/17 macrophages toward M1 by employing various neo adjuvants, summarized in Table 1.
In the past, we have identified several neo adjuvants, such as PDE4b and AC mimetic, which are capable of modulating the Th17 response in human CD14+ monocyte and Slan DC (Oehrl et al., 2017). This reflects the results of another study (Konig et al., 2020), which highlighted the potential of androgenic receptors in modulating cytokine storm. This indicates that PDE4/AC modulators (Maier et al., 2017) could also potentially modulate and mitigate macrophage activation syndrome and help in COVID-19 cases.
Apart from these, we have validated several other immune adjuvants, such as low dose Gamma Radiation (Klug et al., 2013; Prakash et al., 2016), S-1P (Nadella et al., 2019), and Smac mimetics (Nadella et al., 2017), which indicate the potential of the Th1 programming of macrophages in a pre-clinical model system. These adjuvants are likely to create immunity against SARS-CoV-2 infection in the animal models discussed above. Various other adjuvants are included in Table 1, each of which targets refractory macrophages that can also potentially be utilized for tempering macrophage plasticity and could effectively control SARS-CoV-2 infection.
Since macrophages are a relatively plastic component of the body and could help with tissue regeneration and homeostasis after completion of therapy. These aspects are critical for the expected outcome of the proposed immune and pharmacological interventions against infection. M2 or refractory macrophages are also known to promote the activity of fibroblast and mesenchymal stem cells and fibroblasts (Yu et al., 2016; Zhang et al., 2018), which can potentially neutralize any potentially adverse impacts of therapy. Both MSC and macrophages secrete various factors (Boxman et al., 1996; Broughton et al., 2012) that are involved in wound healing. On account of the regenerative potential of macrophages (Chamoto et al., 2012; Hoegl et al., 2016) and their close association with mesenchymal stem cells (Spiller and Koh, 2017; Myneni et al., 2019), these cells can potentially be utilized in a palliative approach (Granata et al., 2010; Almatroodi et al., 2014) for the accelerated recovery of patients. This warrants the use of macrophages and MSC as whole-cell infusions or their products as palliative components for enhancing the recovery of COVID-19 patients after therapeutic interventions.
The proposed interventions depicted in Figure 1 are candid approaches to tuning pathogenic inflammation, including the Th1 reprogramming of derailed Macrophages and enhancing the MHC-I dependent presentation of the viral antigens CD8+ CTL. These approaches provide potential means of augmenting the adaptive immunity of the host in fighting SARS-CoV-2 infection: a novel immunotherapeutic approach that is translationally viable. The approach harbors the potential for the effective management of SARS-CoV-2 infection in severely infected COVID-19 patients. We anticipate that it could be employed as a potential adjunct to placid/antiviral therapies in effectively managing COVID-19 disease, both prospective and therapeutic, in patients and which requires immediate further investigation for its clinical efficacy.
HP conceived the idea and supervised the study. DT, AJ, SK, HM, and VS contributed to the research tool. DT and VT undertook the critical analysis. HP and AJ wrote the manuscript. All authors contributed to the article and approved the submitted version.
The authors declare that the research was conducted in the absence of any commercial or financial relationships that could be construed as a potential conflict of interest.
Almatroodi, S. A., McDonald, C. F., Pouniotis, D. S. (2014). Alveolar Macrophage Polarisation in Lung Cancer. Lung Cancer Int. 2014, 721087. doi: 10.1155/2014/721087
Barnes, B. J., Adrover, J. M., Baxter-Stoltzfus, A., Borczuk, A., Cools-Lartigue, J., Crawford, J. M., et al. (2020). Targeting potential drivers of COVID-19: Neutrophil extracellular traps. J. Exp. Med. 217 (6), 1–7. doi: 10.1084/jem.20200652
Boxman, I. L., Ruwhof, C., Boerman, O. C., Lowik, C. W., Ponec, M. (1996). Role of fibroblasts in the regulation of proinflammatory interleukin IL-1, IL-6 and IL-8 levels induced by keratinocyte-derived IL-1. Arch. Dermatol. Res. 288 (7), 391–398. doi: 10.1007/BF02507108
Broughton, B. R., Lim, R., Arumugam, T. V., Drummond, G. R., Wallace, E. M., Sobey, C. G. (2012). Post-stroke inflammation and the potential efficacy of novel stem cell therapies: focus on amnion epithelial cells. Front. Cell Neurosci. 6, 66. doi: 10.3389/fncel.2012.00066
Chamoto, K., Gibney, B. C., Ackermann, M., Lee, G. S., Lin, M., Konerding, M. A., et al. (2012). Alveolar macrophage dynamics in murine lung regeneration. J. Cell Physiol. 227 (9), 3208–3215. doi: 10.1002/jcp.24009
Escobar, L. E., Molina-Cruz, A., Barillas-Mury, C. (2020). BCG vaccine protection from severe coronavirus disease 2019 (COVID-19). Proc. Natl. Acad. Sci. U. S. A. 117 (30), 17720–17726. doi: 10.1073/pnas.2008410117
Giamarellos-Bourboulis, E. J., Netea, M. G., Rovina, N., Akinosoglou, K., Antoniadou, A., Antonakos, N., et al. (2020). Complex Immune Dysregulation in COVID-19 Patients with Severe Respiratory Failure. Cell Host Microbe 992–1000.e3. doi: 10.1016/j.chom.2020.04.009
Graham, R. L., Becker, M. M., Eckerle, L. D., Bolles, M., Denison, M. R., Baric, R. S. (2012). A live, impaired-fidelity coronavirus vaccine protects in an aged, immunocompromised mouse model of lethal disease. Nat. Med. 18 (12), 1820–1826. doi: 10.1038/nm.2972
Granata, F., Frattini, A., Loffredo, S., Staiano, R. I., Petraroli, A., Ribatti, D., et al. (2010). Production of vascular endothelial growth factors from human lung macrophages induced by group IIA and group X secreted phospholipases A2. J. Immunol. 184 (9), 5232–5241. doi: 10.4049/jimmunol.0902501
Greenlee-Wacker, M. C. (2016). Clearance of apoptotic neutrophils and resolution of inflammation. Immunol. Rev. 273 (1), 357–370. doi: 10.1111/imr.12453
Hoegl, S., Zwissler, B., Eltzschig, H. K., Vohwinkel, C. (2016). Acute respiratory distress syndrome following cardiovascular surgery: current concepts and novel therapeutic approaches. Curr. Opin. Anaesthesiol. 29 (1), 94–100. doi: 10.1097/ACO.0000000000000283
Hoffmann, M., Kleine-Weber, H., Schroeder, S., Kruger, N., Herrler, T., Erichsen, S., et al. (2020). SARS-CoV-2 Cell Entry Depends on ACE2 and TMPRSS2 and Is Blocked by a Clinically Proven Protease Inhibitor. Cell 271–280.e8. doi: 10.1016/j.cell.2020.02.052
Imai, Y., Kuba, K., Rao, S., Huan, Y., Guo, F., Guan, B., et al. (2005). Angiotensin-converting enzyme 2 protects from severe acute lung failure. Nature 436 (7047), 112–116. doi: 10.1038/nature03712
Klug, F., Prakash, H., Huber, P. E., Seibel, T., Bender, N., Halama, N., et al. (2013). Low-dose irradiation programs macrophage differentiation to an iNOS(+)/M1 phenotype that orchestrates effective T cell immunotherapy. Cancer Cell 24 (5), 589–602. doi: 10.1016/j.ccr.2013.09.014
Konig, M. F., Powell, M., Staedtke, V., Bai, R. Y., Thomas, D. L., Fischer, N., et al. (2020). Preventing cytokine storm syndrome in COVID-19 using alpha-1 adrenergic receptor antagonists. J. Clin. Invest. 130 (7), 3345–3347. doi: 10.1172/JCI139642
Lammers, T., Sofias, A. M., van der Meel, R., Schiffelers, R., Storm, G., Tacke, F., et al. (2020). Dexamethasone nanomedicines for COVID-19. Nat. Nanotechnol. 15 (8), 622–624. doi: 10.1038/s41565-020-0752-z
Liao, M., Liu, Y., Yuan, J., Wen, Y., Xu, G., Zhao, J., et al. (2020). Single-cell landscape of bronchoalveolar immune cells in patients with COVID-19. Nat. Med. 26 (6), 842–844. doi: 10.1038/s41591-020-0901-9
Luo, M., Liu, J., Jiang, W., Yue, S., Liu, H., Wei, S. (2020). IL-6 and CD8+ T cell counts combined are an early predictor of in-hospital mortality of patients with COVID-19. JCI Insight 5 (13), 1–12. doi: 10.1172/jci.insight.139024
Maier, C., Ramming, A., Bergmann, C., Weinkam, R., Kittan, N., Schett, G., et al. (2017). Inhibition of phosphodiesterase 4 (PDE4) reduces dermal fibrosis by interfering with the release of interleukin-6 from M2 macrophages. Ann. Rheum Dis. 76 (6), 1133–1141. doi: 10.1136/annrheumdis-2016-210189
Mehta, P., McAuley, D. F., Brown, M., Sanchez, E., Tattersall, R. S., Manson, J. J. (2020). COVID-19: consider cytokine storm syndromes and immunosuppression. Lancet 395 (10229), 1033–1034. doi: 10.1016/S0140-6736(20)30628-0
Middleton, E. A., He, X. Y., Denorme, F., Campbell, R. A., Ng, D., Salvatore, S. P., et al. (2020). Neutrophil Extracellular Traps (NETs) Contribute to Immunothrombosis in COVID-19 Acute Respiratory Distress Syndrome. Blood. 1169–1179. doi: 10.1182/blood.2020007008
Morris, G., Bortolasci, C. C., Puri, B. K., Olive, L., Marx, W., O’Neil, A., et al. (2020). The pathophysiology of SARS-CoV-2: A suggested model and therapeutic approach. Life Sci. 258, 118166. doi: 10.1016/j.lfs.2020.118166
Myneni, V. D., McClain-Caldwell, I., Martin, D., Vitale-Cross, L., Marko, K., Firriolo, J. M., et al. (2019). Mesenchymal stromal cells from infants with simple polydactyly modulate immune responses more efficiently than adult mesenchymal stromal cells. Cytotherapy 21 (2), 148–161. doi: 10.1016/j.jcyt.2018.11.008
Nadella, V., Mohanty, A., Sharma, L., Yellaboina, S., Mollenkopf, H. J., Mazumdar, V. B., et al. (2017). Inhibitors of Apoptosis Protein Antagonists (Smac Mimetic Compounds) Control Polarization of Macrophages during Microbial Challenge and Sterile Inflammatory Responses. Front. Immunol. 8, 1792. doi: 10.3389/fimmu.2017.01792
Nadella, V., Sharma, L., Kumar, P., Gupta, P., Gupta, U. D., Tripathi, S., et al. (2019). Sphingosine-1-Phosphate (S-1P) Promotes Differentiation of Naive Macrophages and Enhances Protective Immunity Against Mycobacterium tuberculosis. Front. Immunol. 10, 3085. doi: 10.3389/fimmu.2019.03085
Oehrl, S., Prakash, H., Ebling, A., Trenkler, N., Wolbing, P., Kunze, A., et al. (2017). The phosphodiesterase 4 inhibitor apremilast inhibits Th1 but promotes Th17 responses induced by 6-sulfo LacNAc (slan) dendritic cells. J. Dermatol. Sci. 87 (2), 110–115. doi: 10.1016/j.jdermsci.2017.04.005
Ohkura, S. I., Usui, S., Takashima, S. I., Takuwa, N., Yoshioka, K., Okamoto, Y., et al. (2017). Augmented sphingosine 1 phosphate receptor-1 signaling in cardiac fibroblasts induces cardiac hypertrophy and fibrosis through angiotensin II and interleukin-6. PLoS One 12 (8), e0182329. doi: 10.1371/journal.pone.0182329
Papadopoulos, D., Rundle, J., Patel, R., Marshall, I., Stretton, J., Eaton, R., et al. (2010). FTY720 ameliorates MOG-induced experimental autoimmune encephalomyelitis by suppressing both cellular and humoral immune responses. J. Neurosci. Res. 88 (2), 346–359. doi: 10.1002/jnr.22196
Penuelas-Rivas, G., Dominguez-Perles, R., Brinkmann, V., Del Rio, M. L., Munoz-Luna, A., Ramirez-Romero, P., et al. (2005). FTY720 inhibits TH1-mediated allogeneic humoral immune response. Transplant. Proc. 37 (9), 4124–4126. doi: 10.1016/j.transproceed.2005.09.184
Prakash, H., Klug, F., Nadella, V., Mazumdar, V., Schmitz-Winnenthal, H., Umansky, L. (2016). Low doses of gamma irradiation potentially modifies immunosuppressive tumor microenvironment by retuning tumor-associated macrophages: lesson from insulinoma. Carcinogenesis 37 (3), 301–313. doi: 10.1093/carcin/bgw007
Saluja, R., Kumar, A., Jain, M., Goel, S. K., Jain, A. (2017). Role of Sphingosine-1-Phosphate in Mast Cell Functions and Asthma and Its Regulation by Non-Coding RNA. Front. Immunol. 8, 587. doi: 10.3389/fimmu.2017.00587
Schroder, M., Richter, C., Juan, M. H., Maltusch, K., Giegold, O., Quintini, G., et al. (2011). The sphingosine kinase 1 and S1P1 axis specifically counteracts LPS-induced IL-12p70 production in immune cells of the spleen. Mol. Immunol. 48 (9-10), 1139–1148. doi: 10.1016/j.molimm.2011.02.007
Schulert, G. S., Grom, A. A. (2015). Pathogenesis of macrophage activation syndrome and potential for cytokine- directed therapies. Annu. Rev. Med. 66, 145–159. doi: 10.1146/annurev-med-061813-012806
Schulze, T., Golfier, S., Tabeling, C., Rabel, K., Graler, M. H., Witzenrath, M., et al. (2011). Sphingosine-1-phospate receptor 4 (S1P(4)) deficiency profoundly affects dendritic cell function and TH17-cell differentiation in a murine model. FASEB J. 25 (11), 4024–4036. doi: 10.1096/fj.10-179028
Spiller, K. L., Koh, T. J. (2017). Macrophage-based therapeutic strategies in regenerative medicine. Adv. Drug Deliv. Rev. 122, 74–83. doi: 10.1016/j.addr.2017.05.010
Subbarao, K., Roberts, A. (2006). Is there an ideal animal model for SARS? Trends Microbiol. 14 (7), 299–303. doi: 10.1016/j.tim.2006.05.007
Vijayan, M., Xia, C., Song, Y. E., Ngo, H., Studstill, C. J., Drews, K., et al. (2017). Sphingosine 1-Phosphate Lyase Enhances the Activation of IKKepsilon To Promote Type I IFN-Mediated Innate Immune Responses to Influenza A Virus Infection. J. Immunol. 199 (2), 677–687. doi: 10.4049/jimmunol.1601959
Walsh, K. B., Marsolais, D., Welch, M. J., Rosen, H., Oldstone, M. B. (2010). Treatment with a sphingosine analog does not alter the outcome of a persistent virus infection. Virology 397 (2), 260–269. doi: 10.1016/j.virol.2009.08.043
Wang, C., Xie, J., Zhao, L., Fei, X., Zhang, H., Tan, Y., et al. (2020). Alveolar macrophage dysfunction and cytokine storm in the pathogenesis of two severe COVID-19 patients. EBioMedicine 57, 102833. doi: 10.1016/j.ebiom.2020.102833
Wrapp, D., Wang, N., Corbett, K. S., Goldsmith, J. A., Hsieh, C. L., Abiona, O., et al. (2020). Cryo-EM structure of the 2019-nCoV spike in the prefusion conformation. Science 367 (6483), 1260–1263. doi: 10.1126/science.abb2507
Xiong, Y., Liu, Y., Cao, L., Wang, D., Guo, M., Jiang, A., et al. (2020). Transcriptomic characteristics of bronchoalveolar lavage fluid and peripheral blood mononuclear cells in COVID-19 patients. Emerg. Microbes Infect. 9 (1), 761–770. doi: 10.1080/22221751.2020.1747363
Yao, X. H., Li, T. Y., He, Z. C., Ping, Y. F., Liu, H. W., Yu, S. C., et al. (2020). [A pathological report of three COVID-19 cases by minimal invasive autopsies]. Zhonghua Bing Li Xue Za Zhi 49 (5), 411–417. doi: 10.3760/cma.j.cn112151-20200312-00193
Yu, C. C., Chien, C. T., Chang, T. C. (2016). M2 macrophage polarization modulates epithelial-mesenchymal transition in cisplatin-induced tubulointerstitial fibrosis. Biomed. (Taipei) 6 (1), 5. doi: 10.7603/s40681-016-0005-5
Zhang, W., Ohno, S., Steer, B., Klee, S., Staab-Weijnitz, C. A., Wagner, D., et al. (2018). S100a4 Is Secreted by Alternatively Activated Alveolar Macrophages and Promotes Activation of Lung Fibroblasts in Pulmonary Fibrosis. Front. Immunol. 9, 1216. doi: 10.3389/fimmu.2018.01216
Zhang, B., Zhou, X., Zhu, C., Song, Y., Feng, F., Qiu, Y., et al. (2020). Immune Phenotyping Based on the Neutrophil-to-Lymphocyte Ratio and IgG Level Predicts Disease Severity and Outcome for Patients With COVID-19. Front. Mol. Biosci. 7, 157. doi: 10.3389/fmolb.2020.00157
Zhou, F., Yu, T., Du, R., Fan, G., Liu, Y., Liu, Z., et al. (2020). Clinical course and risk factors for mortality of adult inpatients with COVID-19 in Wuhan, China: a retrospective cohort study. Lancet 395 (10229), 1054–1062. doi: 10.1016/S0140-6736(20)30566-3
Keywords: COVID 19 infection, M1/ M2 macrophages, adoptive immunotheraoy, CD8+T cells, Anti viral activity
Citation: Toor D, Jain A, Kalhan S, Manocha H, Sharma VK, Jain P, Tripathi V and Prakash H (2020) Tempering Macrophage Plasticity for Controlling SARS-CoV-2 Infection for Managing COVID-19 Disease. Front. Pharmacol. 11:570698. doi: 10.3389/fphar.2020.570698
Received: 08 June 2020; Accepted: 21 August 2020;
Published: 16 October 2020.
Edited by:
Chen Liang, McGill University, CanadaReviewed by:
Paras K. Anand, Imperial College London, United KingdomCopyright © 2020 Toor, Jain, Kalhan, Manocha, Sharma, Jain, Tripathi and Prakash. This is an open-access article distributed under the terms of the Creative Commons Attribution License (CC BY). The use, distribution or reproduction in other forums is permitted, provided the original author(s) and the copyright owner(s) are credited and that the original publication in this journal is cited, in accordance with accepted academic practice. No use, distribution or reproduction is permitted which does not comply with these terms.
*Correspondence: Hridayesh Prakash, aHByYWthc2hAYW1pdHkuZWR1
Disclaimer: All claims expressed in this article are solely those of the authors and do not necessarily represent those of their affiliated organizations, or those of the publisher, the editors and the reviewers. Any product that may be evaluated in this article or claim that may be made by its manufacturer is not guaranteed or endorsed by the publisher.
Research integrity at Frontiers
Learn more about the work of our research integrity team to safeguard the quality of each article we publish.