- 1Science and Technology Innovation Center, Guangzhou University of Chinese Medicine, Guangzhou, China
- 2Department of Endocrinology, Fourth Clinical Medical College, Guangzhou University of Chinese Medicine, Shenzhen, China
- 3Department of Cancer Immunology and Virology, Dana-Farber Cancer Institute, Boston, MA, United States
- 4Department of Medicine, Harvard Medical School, Boston, MA, United States
- 5Department of Clinical Laboratory, The Third Affiliated Hospital of Guangzhou Medical University, Guangzhou, China
- 6Department of Encephalopathy First Affiliated Hospital of Henan University of Chinese Medicine, Zhengzhou, China
- 7Institute of Clinical Pharmacology, Guangzhou University of Chinese Medicine, Guangzhou, China
Alzheimer's disease (AD) is a complex neurodegenerative disease characterized by cognitive dysfunction. Kai-Xin-San (KXS) is a traditional Chinese medicine (TCM) formula that has been used to treat AD patients for over a thousand years in China. However, the therapeutic mechanisms of KXS for treating AD have not been fully explored. Herein, we used a comprehensive network pharmacology approach to investigate the mechanism of action of KXS in the treatment of AD. This approach consists of construction of multiple networks and Gene Ontology enrichment and pathway analyses. Furthermore, animal experiments were performed to validate the predicted molecular mechanisms obtained from the systems pharmacology-based analysis. As a result, 50 chemicals in KXS and 39 AD-associated proteins were identified as major active compounds and targets, respectively. The therapeutic mechanisms of KXS in treating AD were primarily related to the regulation of four pathology modules, including amyloid beta metabolism, tau protein hyperphosphorylation process, cholinergic dysfunction, and inflammation. In scopolamine-induced cognitive dysfunction mice, we validated the anti-inflammatory effects of KXS on AD by determining the levels of inflammation cytokines including interleukin (IL)-6, IL-1β, and tumor necrosis factor (TNF)-α. We also found cholinergic system dysfunction amelioration of KXS is correlated with upregulation of the cholinergic receptor CHRNB2. In conclusion, our work proposes a comprehensive systems pharmacology approach to explore the underlying therapeutic mechanism of KXS for the treatment of AD.
Introduction
Alzheimer's disease (AD), as the most common form of dementia, has become one of the leading causes of morbidity and mortality in the aged population. According to the World Alzheimer Report, up to 2019, there were over 50 million people living with dementia (Cao et al., 2018b; Gaugler and Al, 2019). Patients with AD suffer from a decline in learning and memory, cognitive deficits, and behavioral/personality changes, which lead to a heavy public health burden (Jia et al., 2018; Kumar and Tsao, 2019). As a complex multifactorial disease, AD is driven by extracellular deposition of beta amyloid (Aβ) and intracellular accumulation of tau protein. Current treatments can only provide limited symptomatic-relief benefits but fail to stop or reverse disease progression. Moreover, adverse effects, including diarrhea, nausea, and nightmares, further restrict the clinical treatment of AD (Masters et al., 2015). Therefore, there is an urgent need to discover novel therapeutic drugs with new mechanisms of action (MOAs) for treating AD.
Traditional Chinese medicine (TCM), which embraces centuries of knowledge and practical experience, has been used to treat many complex diseases in China for over 2,000 years (Cooper and Ma, 2017; Jiang et al., 2017). TCM has advantages for multi-targeting in intervention and treatment and has provided comprehensive prospects for understanding physiopathology and drug development for neurodegenerative diseases, including AD (Ho et al., 2010; Law et al., 2017). Kai-Xin-San (KXS) is a widely used TCM formula initially recorded in Beiji Qianjin Yaofang for treating dementia and depression in China since the Tang Dynasty. It is comprised of four herbs: Panax ginseng C. A. Mey (RENSHEN, RS), Polygala tenuifolia Willd (YUANZHI, YZ), Acorus tatarinowii (SHICHANGPU, SCP), and Poria (FULING, FL) (Cao et al., 2018a). Previous studies of KXS mainly focused on the mechanism of a single target-oriented pathway or neurotransmitter regulation, which cannot comprehensively illuminate the therapeutic effects and mechanism of action (MOA) of KXS for AD treatment (Lu et al., 2017; Wang et al., 2017; Cao et al., 2018a; Gao et al., 2018). Herein, there is a need to investigate the overall beneficial effects of KXS for treating AD using advanced approaches.
Systems pharmacology is a cutting-edge methodology that combines computational and experimental tools toward discovering novel therapeutic agents and understanding the therapeutic mechanisms of complex diseases. (Fang et al., 2017a; Fang et al., 2019). In recent years, systems pharmacology-based approaches have provided new insights into elucidating the mechanisms of TCM in the treatment of diseases such as cardiovascular diseases and AD (Zhou and Wang, 2014; Fang et al., 2017a; Cai et al., 2018). In this study, we employed a systems pharmacology approach to identify potential compounds, candidate targets, and therapeutic mechanisms of KXS against AD disease from a holistic prospect (Figure 1). Briefly, we first determined the comprehensive AD-associated genes and ingredients of KXS after integrating different data sources. We further predicted candidate targets based on a balanced substructure-drug-target network-based inference approach (bSDTNBI). Subsequently, the targets of KXS were mapped onto AD-relevant genes to determine their biological functions and corresponding AD pathways. Furthermore, we performed multiple level data analyses to reveal the MOA of KXS on AD treatment. Finally, we validated the proposed pharmacological mechanism of KXS in a scopolamine (SCOP)-induced AD mouse model.
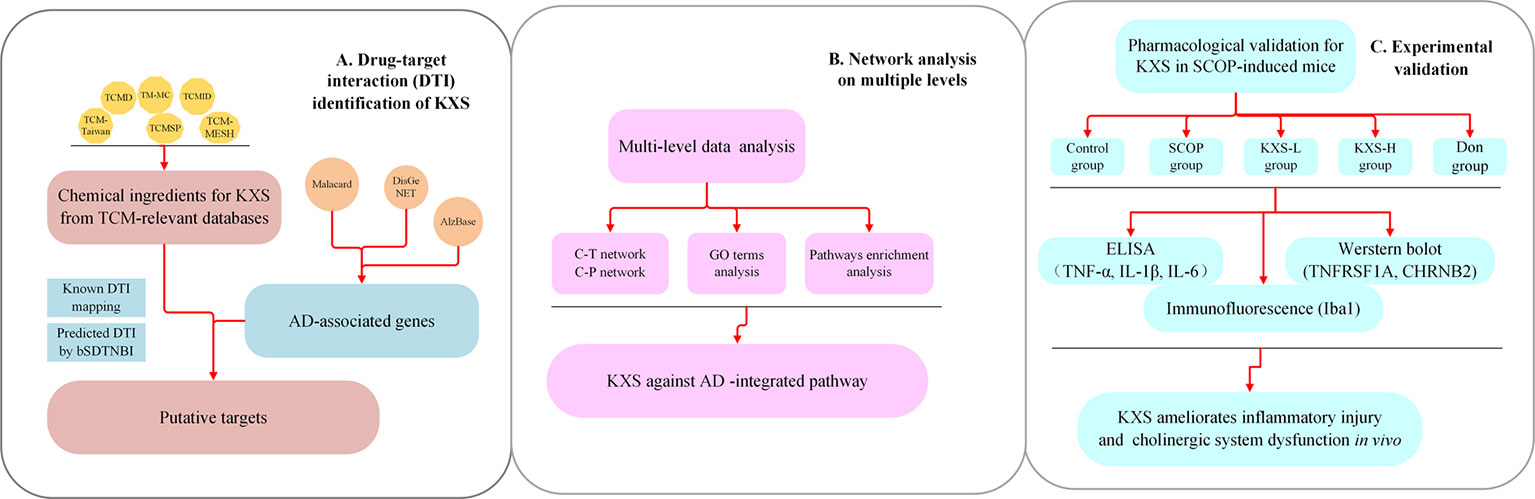
Figure 1 Flowchart of the systems pharmacology approach for deciphering the therapeutic mechanisms of action of Kai-Xin-San (KXS) on Alzheimer's disease (AD). (A) Drug-target interaction (DTI) identification. (B) Network analysis of multiple data to investigate the therapeutic mechanisms of KXS on AD. (C) Experimental validation in vivo to explore the pharmacological mechanisms of KXS on AD.
Materials and Methods
AD-Associated Gene Collection
Genes related to AD were collected from several public disease gene-related databases, including Malacard (https://www.malacards.org), DisGeNet database, GWAS catalog, HGMD (Pinero et al., 2017), AlzBase database (http://alz.big.ac.cn/alzBase/summary/Gene), and AlzPlatform. AlzPlatform is an AD-specific chemogenomics knowledgebase for target identification and drug discovery (Liu et al., 2014). Ultimately, a total of 447 AD-associated genes were obtained (Supplementary Datasheet S1).
KXS Ingredient Collection
All ingredients in KXS (4 herbs) were collected from six TCM-related databases, including TCMID (Xue et al., 2013), TCM-Taiwan (Chen, 2011), TCMD (He et al., 2001), TCMSP (Ru et al., 2014), TM-MC (Kim et al., 2015), and TCM-MESH (Zhang et al., 2017). For each database, we extracted the chemical structures of each herb as an SDF file. Subsequently, six SDF files were merged to a single SDF, which contained all of the chemical structures from the six data sources. SMILES as well as InChIKey were generated by Open Babel (O'boyle et al., 2011) for each ingredient. After removing the duplicates, 1,118 ingredients in KXS were finally obtained.
Target Identification for KXS
In this work, the known targets of KXS were extracted from our previous integrated database (Fang et al., 2017b), which contains 7,314 drug-target interactions (DTIs) connecting 751 targets and 2,388 natural products.
In a previous study, we developed predictive network models to identify new targets of natural products via the bSDTNBI approach (Fang et al., 2017b), which prioritized potential targets for known drugs and new chemical entities (NCEs) by resource-diffusion processes of the substructure drug-target network. During the course, two parameters including α and β were imported to balance the initial resource allocation of different node types as well as the weighted values of different edge types. Moreover, parameter γ was also utilized to balance the influence of hub nodes. The final parameters (α = β =0.1, γ = –0.5, and k = 2) of bSDTNBI were adopted from a previous study (Wu et al., 2016). Among the four network models developed using different types of fingerprints, bSDTNBI_KR performed best with the highest value of P (0.049), R (0.752), eP (27.02), eR (27.24), and AUC (0.959). Thus, bSDTNBI_KR was utilized to predict new targets of natural products in the global network.
Network Construction
To comprehensively understand the complex interactions among herbs, compounds, targets, and pathways, herb-target and compound-target networks were constructed by Cytoscape (version 3.2.1) and Gephi (version 0.9.2). In the graphical network, the nodes represent compounds, targets, or herbs, while the edges denote links among them. The quantitative property “degree” was calculated as the number of edges linked to each node, indicating the importance of a given node in a network.
Gene Ontology (GO) Enrichment Analysis
The biological significance of protein targets can be interpreted via GO enrichment analysis. In this work, we conducted a biological process (BP) interpretation by mapping AD-related genes (with a degree greater than 2) of KXS to the DAVID database (https://david.ncifcrf.gov/home.jsp) (Huang Da et al., 2009). DAVID is an integrated biological knowledgebase and analytic tool aimed at systematically extracting the biological meaning from large gene/protein lists.
Pathway Construction and Analysis
To investigate the roles of protein targets in the pathophysiological network of AD and how KXS acts on AD by regulating certain pathways, an “AD-integrated pathway” was proposed based on our present understanding of AD pathology and target identification. In brief, the protein targets were first mapped to the Kyoto Encyclopedia of Genes and Genomes database (KEGG, http://www.genome.jp/kegg/) to obtain the potential pathways. Subsequently, pathways related to AD pathological processes were selected and incorporated into an “AD-integrated pathway” to analyze the therapeutic mechanisms of KXS for treating AD.
Experimental Validation
KXS Preparation
The four herbs in KXS (RS, FL, SCP, and YZ) were obtained from the First Affiliated Hospital of Guangzhou University of Chinese Medicine (Guangzhou, China) and were mixed at a ratio of 3:3:2:2 referenced the previous studies (Cao et al., 2012; Xu et al., 2019). The extraction process and quality control were implemented according to our previous study (Xu et al., 2019). Briefly, SCP was added to six parts of water as a solvent for 2 h, followed by heat reflux extraction for 8 h. Volatile oil and SCP dregs were saved. RS was extracted twice by 60% ethanol as solvent for each time with 1 hour. The extracts were combined and filtrated, and the RS dregs were saved. The dregs of SCP, RS, FL, and YZ were added to 10 parts of water and extracted twice, for 1 h each. All extracts were combined and evaporated on a rotary evaporator. Finally, volatile oil of SCP and original liquid after concentrated were mixed and refrigerated at −20°C for usage.
AD Model and Drug Treatments
Kun-Ming mice (8 weeks old) weighing 30–35 g were obtained from Sibeifu Biotechnology (Beijing, China) and housed at 22 ± 2°C, with a relative humidity of 55% ± 5%, a 12 h light/dark cycle and ad libitum access to food and water. All animal procedures were performed in accordance with the principles and guidelines of the National Institutes of Health Guide for the Care and Use of Laboratory Animals and approved by the Guangzhou University of Chinese Medicine Animal Ethics Committee.
AD model was induced by intraperitoneally injected SCOP (3 mg/kg) for a consecutively week. In this week, Morris water maze and new objection recognition test were performed daily after 30 min of SCOP injection to evaluate animal model. In this study: mice were randomly allotted to five groups: Control group (n=10; 0.9% saline p.o.+ 0.9% saline, i.p.), SCOP group (n=10; 0.9% saline p.o.+ SCOP 3 mg/kg/d i.p.), low-dose KXS group (n=10; KXS 1.4 g/kg/d p.o.+ SCOP 3 mg/kg/d i.p.), high-dose KXS group (n=10; KXS 2.8 g/kg/d p.o.+ SCOP 3 mg/kg/d i.p.), and Don group (n=10; donepezil 3 mg/kg/d p.o.+ SCOP 3 mg/kg/d i.p.). The mice were orally administered 0.9% saline, KXS, or donepezil mice for 14 continuous days. Memory impairment was induced by SCOP treatment (3 mg/kg body weight) for 7 days.
Western Blot Analysis
The hippocampus and cortex were homogenized in SDS lysis buffer containing a protease inhibitor and phosphatase inhibitor mixture (Sigma-Aldrich). After sonication, lysates were centrifuged at 3,000×g and 4°C for 15 min. Supernatants were collected, and protein concentrations were determined by the Bradford assay (Bio-Rad). The same amounts of proteins were resolved on SDS-PAGE gels, transferred to PVDF membranes (Millipore), and probed with primary antibodies overnight at 4°C. Rabbit anti-TNFRSF1A (1:1,000, Abcam), anti-CHRNB2 (1:1,000, Abcam), and anti-β-actin (1:10,000, Abcam) were used as the primary antibodies. Immunoblots were visualized by the ECL western blot detection kit (Millipore) and quantified by densitometry and ImageJ software (National Institutes of Health) (Lee et al., 2017).
Immunofluorescence
Mice were transcardially perfused with 0.9% saline. The mouse brains were fixed in 4% paraformaldehyde (PFA) for 72 h, followed by dehydration with different concentrations of ethanol and paraffin embedding. A paraffin microtome was used to cut 5-μm-thick coronal brain sections.
For immunofluorescence, sections were deparaffinized by ethanol and incubated with 3% H2O2. Antigen retrieval was performed by heating the sections in sodium citrate buffer (10 mM trisodium citrate, 0.5% Tween-20 in H2O, pH 6.0) at 70°C for 30 min. Sections were then permeabilized and incubated with blocking solution containing the Iba-1 antibody (1:100, Abcam) overnight at 4°C. The sections were then stained with a fluorophore-conjugated secondary antibody (1:1,000, CST) for 1 h, followed by 4′6-diamino- 2-phenylindole (DAPI, Sigma) staining for another 1 h. Images were acquired by a fluorescence microscope Model DMi8 (Leica, Germany) and quantified using ImageJ software (National Institutes of Health) (Muhammad et al., 2019).
ELISA Analysis
Mice cortical and hippocampal tissues were sequentially homogenized in ice-cold PBS, and the supernatants were centrifuged at 21,000×g for 20 min at 4°C. The levels of IL-1β, IL-6, and TNF-α were measured using an enzyme-linked immunosorbent assay (ELISA, Biological Technology, Jiangsu) (Karthivashan et al., 2018) according to the manufacturer's instructions.
Statistical Analysis
All data were expressed as means ± SEM of at least three independent experiments. Statistical analyses were performed using SPSS (version 20.0, IBM, Armonk, NY). Statistical tests between multiple datasets were carried using a one-way analysis of variance (ANOVA) followed by Dunnett's post hoc test to determine statistical significance, as appropriate. A P value < 0.05 was considered statistically significant.
Results and Discussion
Collection of Chemical Ingredients in KXS
In the present study, a total of 1,118 compounds in KXS were collected from specific TCM-relevant databases after removing the duplicate structures. The numbers of ingredients for each herb in KXS were 628 (RS), 237 (YZ), 119 (FL), and 210 (SCP). Among the 1,118 chemical ingredients, there were 70 compounds that existed in more than one herb. For example, compound M449 (palmitic acid) could be found in all four herbs in the KXS formula. Candidate ingredients were defined if there were known targets or putative targets via bSTDNBI for a certain ingredient. Ultimately, 1,113 candidate compounds were obtained (Supplementary Datasheet S1).
Identification of Putative Targets for the Ingredients in KXS
After merging the known DTIs and predicted DTIs by bSTDNBI, we identified 439 target proteins for 1,113 candidate compounds. We further identified 39 AD-associated targets for KXS by overlapping 439 potential targets into the curated human AD-associated 447 genes. Detailed information of the 39 AD-associated targets can be found in Supplementary Datasheet S1.
Analysis of the Synergetic Actions of KXS Against AD
The TCM theory “Jun-Chen-Zuo-Shi” serves as the guide for physicians when formulating herbal prescriptions. Among the herbs in KXS, RS and FL serve as the “Jun” and “Chen” herbs to treat the major symptoms and signs of AD, while YZ and SCP act as the “Zuo” and “Shi” herbs for improving the therapeutic effects of the “Jun” and “Chen” herbs and guiding the herbs to the disease targets (Cao et al., 2018a). Herein, we investigated the distribution of AD-relevant targets among the four herbs (18 from RS, 9 from FL, 16 from SCP, 15 from YZ) via a Venn analysis (Supplementary Datasheet S1).
The four herbs covered all 39 AD-associated targets (Figure S1). Among the four herbs, RS covered the largest number (18) of AD targets for the “Jun” herb in KXS for treating AD, demonstrating the consistency of TCM theory. To exploit the synergistic MOAs of the KXS formula at the individual herb level, we constructed a herb-target network (Figure 2, H-T network). We found that the four herbs in KXS shared six common targets, suggesting that KXS could exert its magnifying effects by targeting these key targets. These targets include acetylcholinesterase (ACHE), beta-secretase 1 (BACE1), hydroxysteroid 17-beta dehydrogenase 10 (HSD17B10), mitogen-activated protein kinase 1 (MAPK1), peroxisome proliferator-activated receptor gamma (PPARG), and tumor necrosis factor (TNF). BACE plays a key role in neurotoxic Aβ generation (Moussa-Pacha et al., 2019). Tumor necrosis factor-α (TNF-α) has been confirmed to advance Aβ production through enhancing the expression of BACE1 and suppressing the clearance of Aβ (Yamamoto et al., 2007; Decourt et al., 2017). Moreover, ACHE regulates acetylcholine in the cholinergic system, which plays a role in the learning process in AD patients (Ferreira-Vieira et al., 2016). Therefore, it is likely that the four herbs in KXS are able to regulate several important AD-associated pathological processes to exert advanced or synergistic effects in AD intervention and treatment.
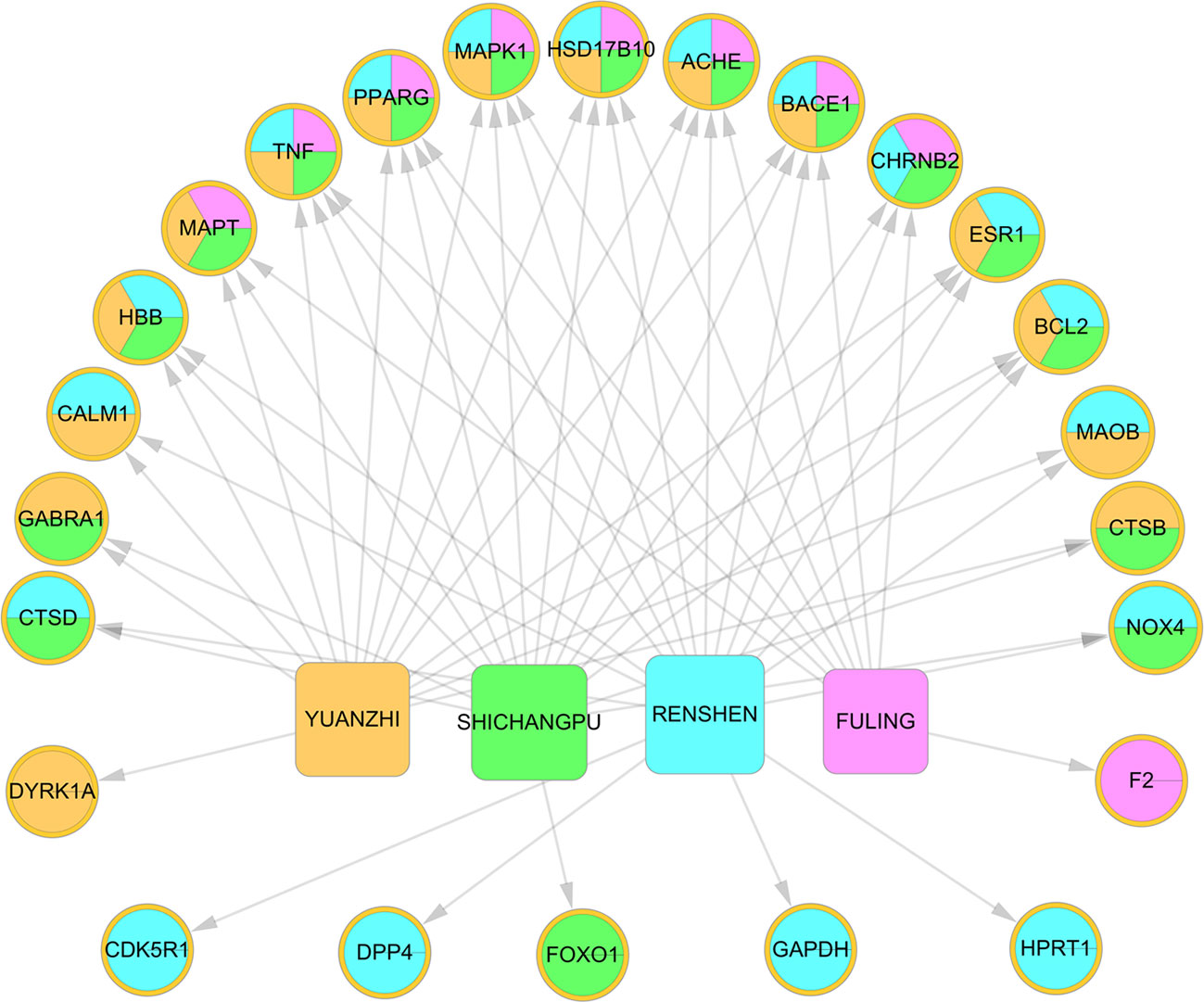
Figure 2 Herb-target network of Kai-Xin-San (KXS). The ellipses represent the targets of KXS. The round rectangle indicates the herbs in KXS.
Compound-Target (C-T) Network Analysis
To further decipher the therapeutic mechanism of KXS against AD, we constructed a AD-specific compound-target (C-T) network consisting of 1,936 C-T interactions (Figure 3). The C-T network comprises 40 known CTIs and 1,896 predicted CTIs connecting 1,021 compounds to 39 AD target proteins. Among the 1,021 ingredients, nine have a target degree (N) higher than five, including M909 (apigenin, N=9), M638 (4-aminobutyric acid, N=6), M326 (aspidinol, N=5), M429 (dodecanal, N=5), M621 (harmine, N=5), M8 (spinacen, N=5), and M903 (DL-catechin, N=5). For the 39 AD targets, five were targeted by more than 100 compounds (D): ACHE (D=454), MAPK1 (D=372), TNF (D=346), PPARG (D=261), and BACE1 (D=174). Previous studies have demonstrated that these targets are beneficial for AD patients. For example, inhibitors of ACHE (e.g., donepezil) can provide modest symptomatic relief for AD patients (Arvanitakis et al., 2019). Recently, both preclinical and clinical studies have indicated that PPARG agonists can improve learning and memory abilities in AD patients (Khan et al., 2019). BACE1 inhibitors have also been reported to repress the generation of neurotoxic amyloid protein (Dobrowolska Zakaria and Vassar, 2018; Moussa-Pacha et al., 2019). Overall, this AD-specific C-T network contributes to uncovering the MOA of chemicals in KXS against AD. The detailed information of the C-T network is provided in Supplementary Datasheet S2.
GO BP Enrichment Analysis
To illustrate the related signaling pathways involved in the treatment of AD with KXS, GO BP enrichment analysis was performed using ClueGO, a plug in of the Cytoscape software package. Only the top 20 significantly enriched (adjusted P < 0.05) signaling pathways were preserved for further analysis. Multiple signaling pathways were involved in the treatment of AD with KXS, including reactive oxygen species metabolic process, positive regulation of neuron death (apoptotic process), regulation of calcium ion transport into the cytosol, and nitric oxide biosynthetic process, etc. (Figure 4). These signaling pathways seem to play vital roles in AD-associated pathological processes. For instance, Aβ-induced neuron death is considered a central pathway in the pathogenesis of AD through oxidative stress, inflammation, apoptosis, or autophagy (Cao et al., 2018b; Kumar and Tsao, 2019).
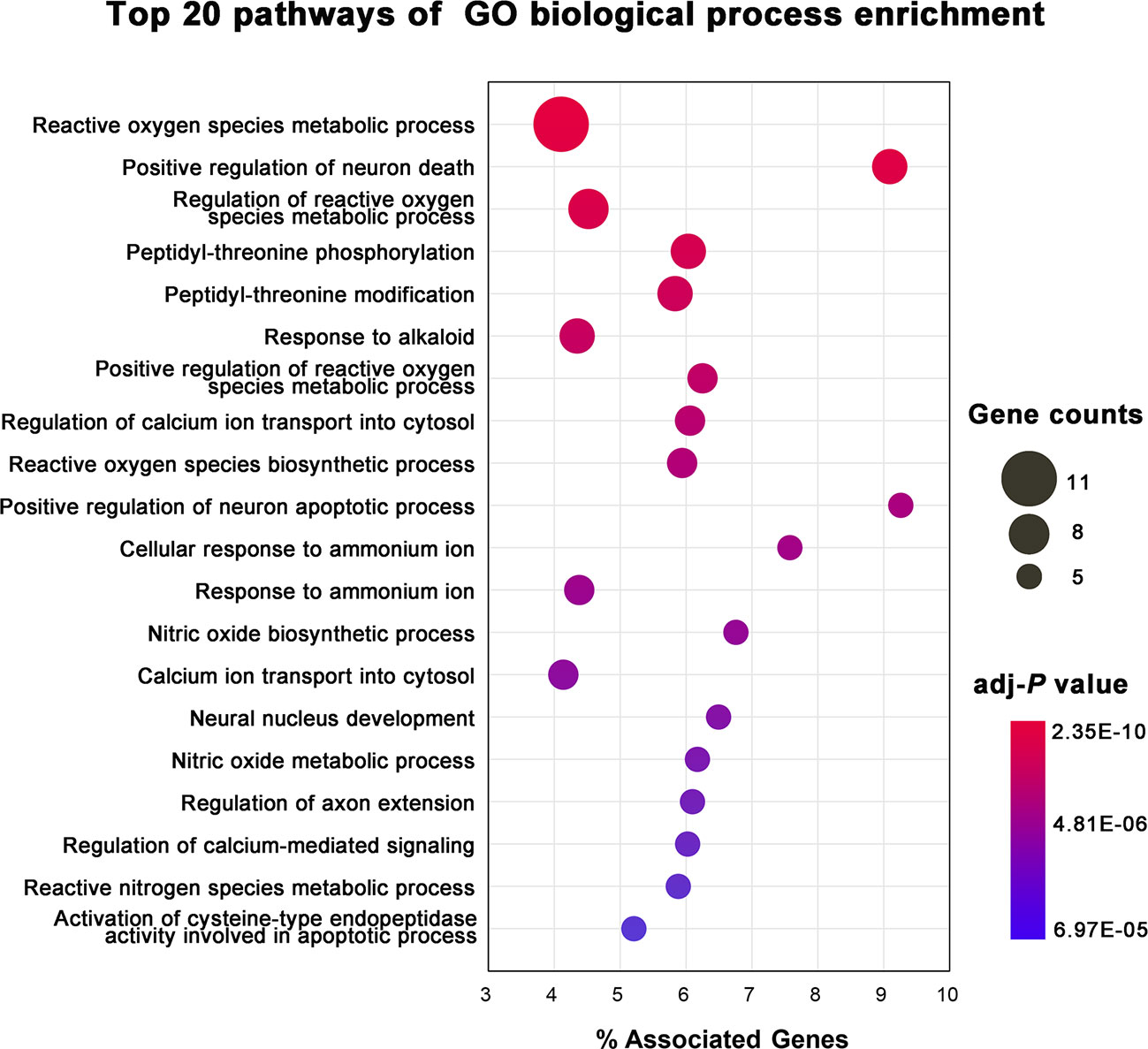
Figure 4 Gene Ontology (GO) biological process enrichment analysis of targets of Kai-Xin-San (KXS) for the treatment of Alzheimer's disease (AD).
Integrated Pathway Analysis
In this analysis, pathways directly related to AD were incorporated into an “AD-integrated pathway” based on target identification for KXS and AD pathology, including the glucose homeostasis signaling pathway, ACHE-associated signaling pathway, Aβ-associated signaling pathway, tau hyperphosphorylated signaling pathway, and TNF-induced inflammation signaling pathway. As depicted in Figure 5, several pathophysiological modules were involved in this integrated pathway, such as glycolysis, cell death, survival, and synaptic plasticity. Here, four representative modules will be discussed to illustrate the underlying therapeutic mechanisms of KXS for AD treatment.
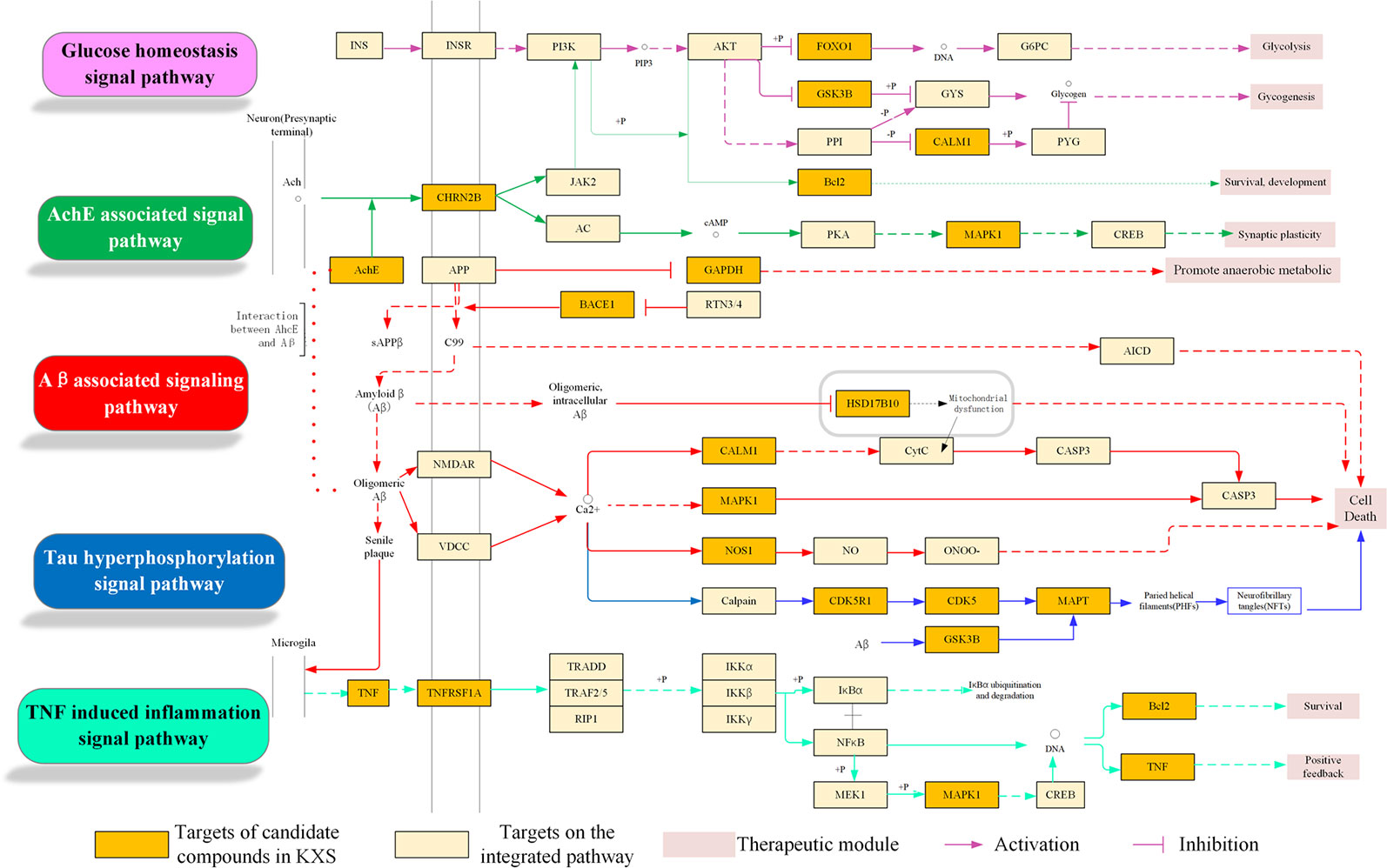
Figure 5 Integrated Alzheimer's disease (AD)-pathway and Kai-Xin-San (KXS) action in the therapeutic modules.
Aβ-Associated Pathological Signaling Pathway Regulation Module
It is well known that abnormal Aβ aggregation and accumulation plays a critical role in the pathogenesis of AD. Amyloid precursor protein (APP) is cleaved by α-secretase, β-secretase, and γ-secretase via non-amyloidogenic and amyloidogenic pathways. In the non-amyloidogenic pathway, proteolysis of APP by α- and γ-secretases yields nonpathogenic fragments, including sAPPα and C-terminal fragments. However, the amyloidogenic pathway involves cleavage by β- and γ-secretases, leading to the production of sAPPβ, C-terminal fragments, and Aβs (Barage and Sonawane, 2015; Selkoe and Hardy, 2016). Accumulated Aβ can further directly or indirectly trigger other pathological processes, such as mitochondrial dysfunction and inflammation, inducing neuronal death in AD (Mao et al., 2012). Thus, targeting β- and γ-secretases is regarded as a primary therapeutic approach for reducing Aβ production in AD patients. As shown in Figure 5, KXS targets key proteins implicated in the Aβ-associated signaling pathway, including BACE1 (β-secretase 1), GAPDH (glycolytic glyceraldehyde-3-phosphate dehydrogenase), and SD17B10 (hydroxysteroid 17-beta dehydrogenase 10). GAPDH (glycolytic glyceraldehyde-3-phosphate dehydrogenase), an abundantly expressed oxidoreductase for glucose metabolism, has been reported to possess diverse non-glycolytic functions, including interaction with Aβ (El Kadmiri et al., 2014; Sen et al., 2018). Target prediction suggested that M693 (citric acid) could bind with GAPDH, which might alleviate Aβ accumulation by disturbing the cross-talk between Aβ and GAPDH (Gerszon and Rodacka, 2018). In addition, HSD17B10 (hydroxysteroid 17-beta dehydrogenase 10), a hydroxysteroid dehydrogenase localized in the mitochondria (He et al., 2018), was the potential target of the compounds in KXS, including M150, M388, and M500. This target has been recognized as a putative intracellular mediator of Aβ neurotoxicity via its dozen proteins that bind to Aβ (Reddy et al., 2012). Thus, KXS could disturb the combination of Aβ and HSD17B10 to alleviate mitochondria dysfunction as it activates β-secretase and γ-secretase and facilitates Aβ generation (Yang et al., 2014). Taken together, KXS might prevent and treat AD by regulating Aβ generation and aggregation.
Neurofibrillary Tangles Regulation Module
NFTs (neurofibrillary tangles), aggregating by abnormal hyperphosphorylation of microtubule-associated protein tau (MAPT) in the brain, correlate well with the severity of cognitive deficits in AD patients (Simic et al., 2016; Jouanne et al., 2017). Tau protein can be hyperphosphorylated by various kinases including CDK5 (cyclin-dependent kinase 5) (Zhao et al., 2019) and GSK3B (glycogen synthase kinase 3beta) (Chu et al., 2017). Therefore, a reduction in tau protein levels (Wang and Mandelkow, 2015) as well as inhibition of tau protein hyperphosphorylation and aggregation are potential therapeutic avenues for AD treatment. As shown in Figure 5, KXS was predicted to target three crucial proteins (i.e., CDK5, GSK3B, and MAPT) involved in the regulation of NFTs. For example, the compounds M621, M622, and M909 were predicted to interact with CDK5 and GSK3B. Emerging evidence has revealed that inhibiting these kinases can prevent tau protein hyperphosphorylation and NFT formation (Congdon and Sigurdsson, 2018). Additionally, compounds such as M84, M257, and M686 were identified to bind to MAPT, suggesting that KXS might decrease tau protein levels to alleviate NFT formation and ameliorate Aβ-dependent neurotoxicity (Peters et al., 2019).
Cholinergic System Dysfunction Regulation Module
The loss of cholinergic neurons in AD patients leads to learning and memory deficits (Ferreira-Vieira et al., 2016). Cholinesterase inhibitors can prevent the breakdown of acetylcholine and preserve its activity at cholinergic synapses (Hampel et al., 2018). Figure 5 shows that the KXS formula regulates the cholinergic system signaling pathway by targeting ACHE (acetylcholinesterase) and CHRN2B (cholinergic receptor nicotinic beta 2 subunit). Four compounds, M21, M389, M597, and M944, interact with ACHE and CHRN2B, which might improve the level of acetylcholine and sustain its activity. Our previous study demonstrated that KXS could ameliorate cognitive function in SCOP-induced mice by regulating the cholinergic system through sustaining ACh levels, increasing ChAT activity, and decreasing AChE activity (Xu et al., 2019), which is in accordance with our systems pharmacology-based analysis.
Neuroinflammation Regulation Module
Neuroinflammation refers to the inflammatory response in the central nervous system secondary to neuronal insult (Calsolaro and Edison, 2016). Activating microglia and astrocytes in AD leads to the production and release of inflammatory cytokines, including interleukin-6 (IL-6), interleukin-1β (IL-1β), and TNF-α (Heneka et al., 2015; Kinney et al., 2018; Ozben and Ozben, 2019). Figure 5 suggests that KXS, a more specifically the compounds M230, M885, and M606, could modulate neuroinflammation in AD by regulating TNF-α and its receptor TNF-receptor superfamily 1A (TNFRSF1A). TNF-α can exacerbate Aβ burden by increasing γ-secretase activity and β-secretase production (Cheng et al., 2014; Chang et al., 2017) or bind to TNFRSF1A. This effect is partly mediated through MAPK1 signaling, which leads to the expression of molecules that participate in inflammation and amyloid genesis (Montgomery and Bowers, 2012; Decourt et al., 2017). Furthermore, IL-1β and IL-6 are able to enhance the amyloidogenic process of APP (Tachida et al., 2008) and the hyperphosphorylation of tau epitopes (Kitazawa et al., 2011). Suppression of either IL-1β or IL-6 can ameliorate neuroinflammation and neurodegeneration (Basu et al., 2004; Rothaug et al., 2016). In summary, KXS might prevent and treat AD by regulating neuroinflammation.
Experimental Validation of KXS in SCOP-Induced Mice
KXS Ameliorates Inflammatory Injury in SCOP-Induced Mice
The systems pharmacology analysis indicated that the therapeutic mechanism of KXS for AD involves several potential mechanisms, including the regulation of cholinergic system dysfunction and neuroinflammation.
Chronic neuroinflammation (sustained microglial activation and overproduction of pro-inflammatory cytokines) has been implicated in the pathophysiology of AD. To validate the mechanism of KXS on neuroinflammation in vivo, we measured proinflammatory cytokine levels in SCOP-induced mice treated with KXS. As shown in Figure 6, SCOP administration significantly increased the levels of TNF-α, IL-1β, and IL-6 compared to the non-treated control group (P < 0.05 or P < 0.01). However, KXS and Don significantly (P < 0.05) attenuated the upregulation of IL-1β, TNF-α, and IL-6 compared with the SCOP alone group both in the hippocampus and cortex.
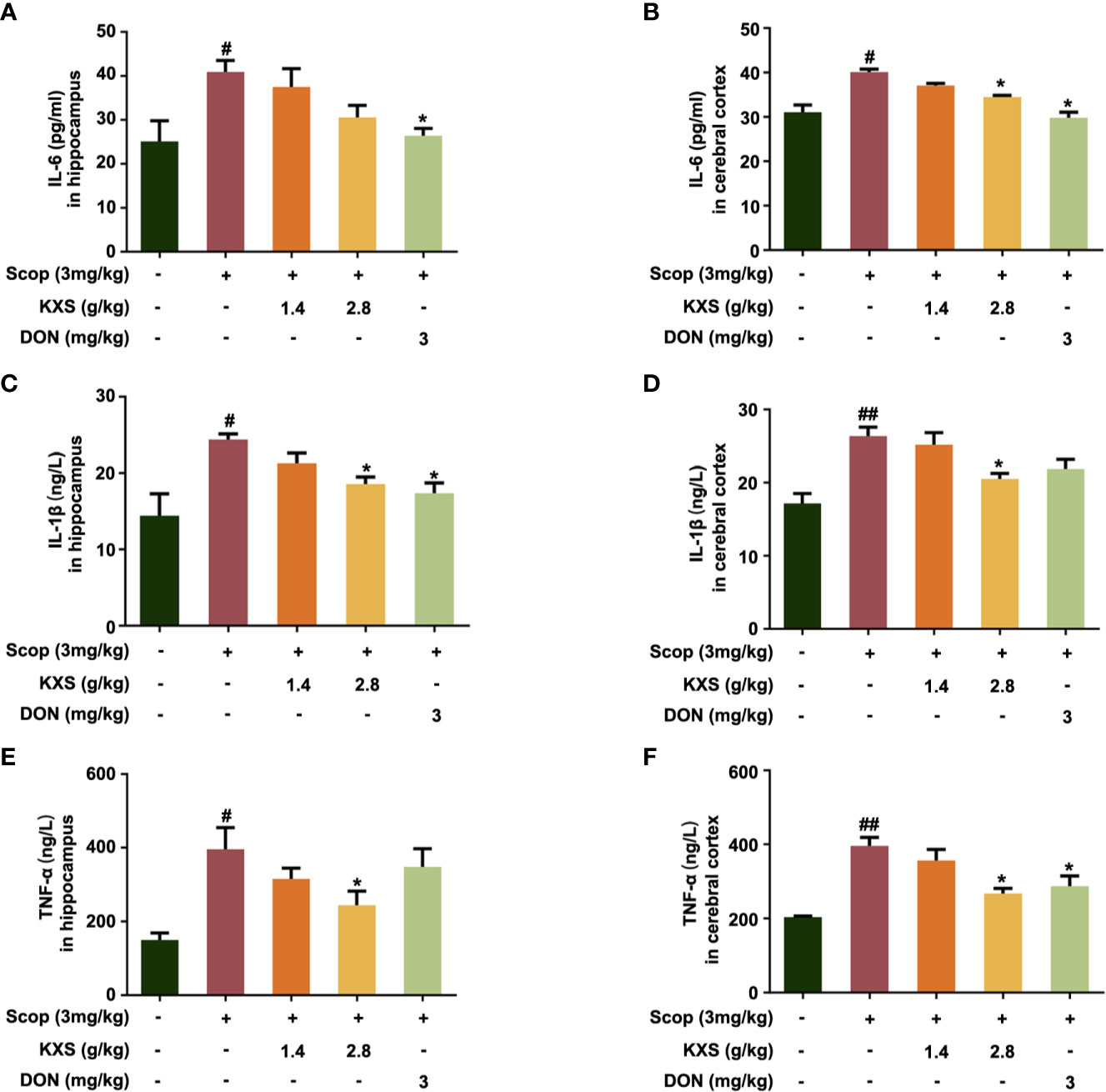
Figure 6 Kai-Xin-San (KXS) attenuates inflammatory injury in scopolamine (SCOP)-induced mice. The levels of interleukin (IL)-6, IL-1β, and tumor necrosis factor (TNF)-α in the hippocampus (A, C, E) and cortex (B, D, F) were determined by ELISA. Data are expressed as means ± SEM. Difference was calculated using one-way ANOVA followed by Dunnett's post hoc test (n=5, #P < 0.05, ##P < 0.01 the control group versus the SCOP group; *P < 0.05 versus the SCOP group).
TNFRSF1A is the main cell surface receptor for TNF. Next, we explored the expression of TNFRSF1A by western blotting. As shown in Figure 7, the protein expression of TNFRSF1A increased in the SCOP group in both the hippocampus and cortex (P < 0.01, vs. the control group). Both the low-dose and high-dose KXS groups and the DON wed group decreased TNFRSF1A expression (P < 0.05 or P < 0.01, vs. the SCOP group).
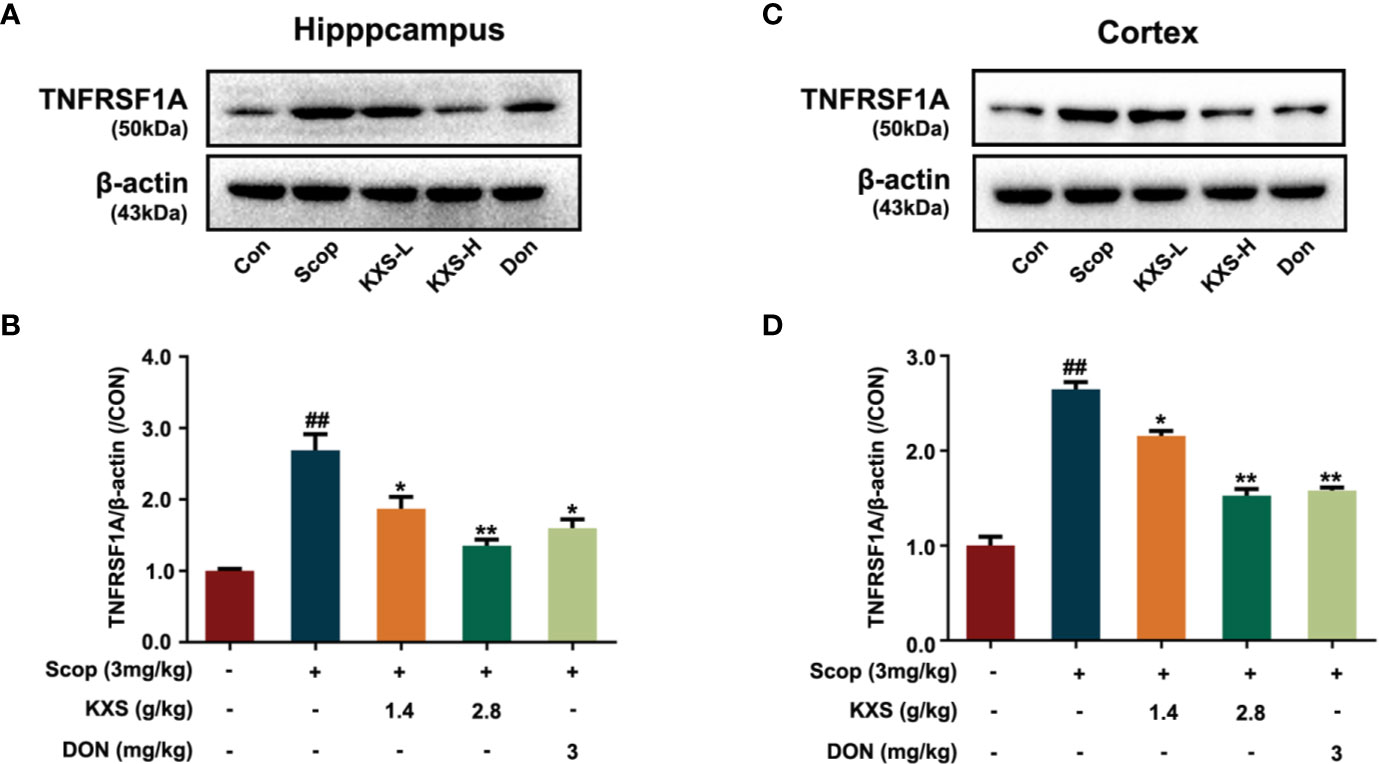
Figure 7 Kai-Xin-San (KXS) decreases the protein expression of tumor necrosis factor receptor superfamily member 1A (TNFRSF1A) in scopolamine (SCOP)-induced mice. Western blot analysis showing the protein expression levels of TNFRSF1A in the hippocampus (A) and cortex (C). Quantification of TNFRSF1A levels in panel (A, B) or (C, D) was normalized to that of actin. Data represent the means ± SEM (n=3, ##P < 0.01 versus the control group; *P < 0.05, **P < 0.01 versus the SCOP group).
Microglia secrete a number of inflammatory cytokines including TNF-α, IL-1β, and IL-6 in response to inflammatory stimuli (Ozben and Ozben, 2019). To further probe the effect of KXS on neuroinflammation, we detected the expression of Iba-1, a microglia activation marker, in the hippocampus and cortex using immunofluorescence. As displayed in Figure 8, elevated microglia activation was observed in the SCOP group in the hippocampus (CA1) and cortex, whereas KXS and donepezil markedly attenuated microglia activation. These results demonstrate that KXS inhibits neuroinflammation in SCOP-induced mice
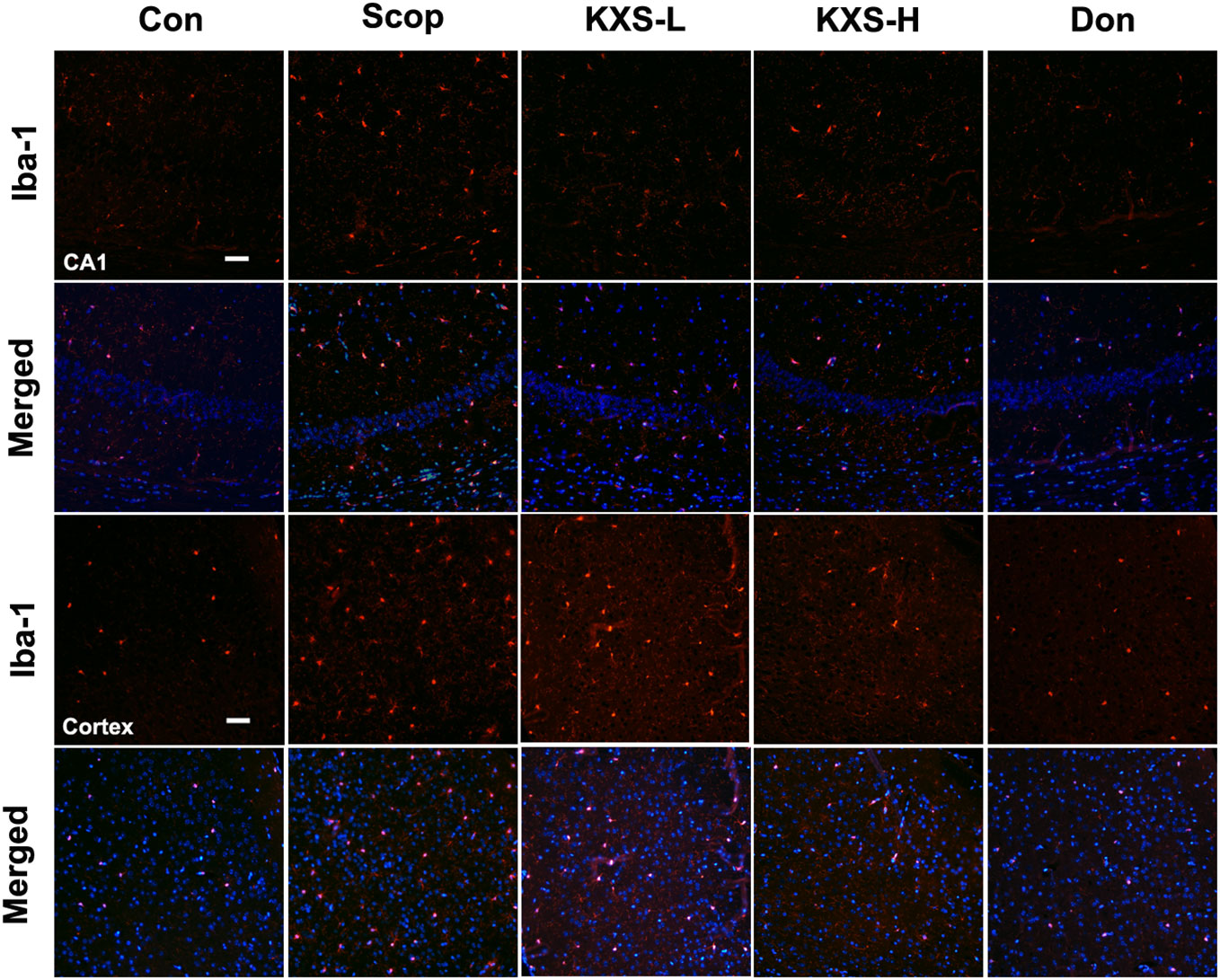
Figure 8 Kai-Xin-San (KXS) attenuates microglia activation in scopolamine (SCOP)-induced mice. Immunofluorescence analysis in the hippocampus (CA1) and cortex. Microglia were stained with anti-Iba-1 (red) and the nuclei were stained with DAPI (blue). Scale bar: 50 mm. Con, control group; SCOP, scopolamine; KXS-L, low-dose Kai-Xin San (1.4 g/kg); KSX-H, high-dose Kai-Xin San (2.8 g/kg); Don, donepezil.
KXS Attenuates Cholinergic System Dysfunction in SCOP-Induced Mice
In our previous study, we demonstrated that KXS could alleviate cholinergic system dysfunction in SCOP-induced mice by increasing ChAT activity and the Ach content, and decreasing AChE activity (Xu et al., 2019). However, the detailed mechanism remains unclear. In this study, a systems pharmacology-based analysis suggested that KXS could regulate the expression of the target protein CHRNB2 (a nicotinic acetylcholine receptor). CHRNB2 is lost in the brains of AD patients and knockout of the CHRNB2 gene impairs neuronal survival in aging (Cook et al., 2004). Therefore, we further investigated the effect of KXS on cholinergic dysfunction via CHRNB2 expression in SCOP-induced mice. As shown in Figure 9, SCOP significantly downregulated the expression of CHRNB2 compared to the control group in both the hippocampus and cortex (P < 0.05, P < 0.01, respectively). High-dose KXS and Donepezil remarkably increased the expression of CHRNB2 both in the hippocampus and cortex (P < 0.05, vs. the SCOP group). These data suggest that CHRNB2 may play an important role in the effects of KXS ameliorating cholinergic system dysfunction of SCOP-induced mice.
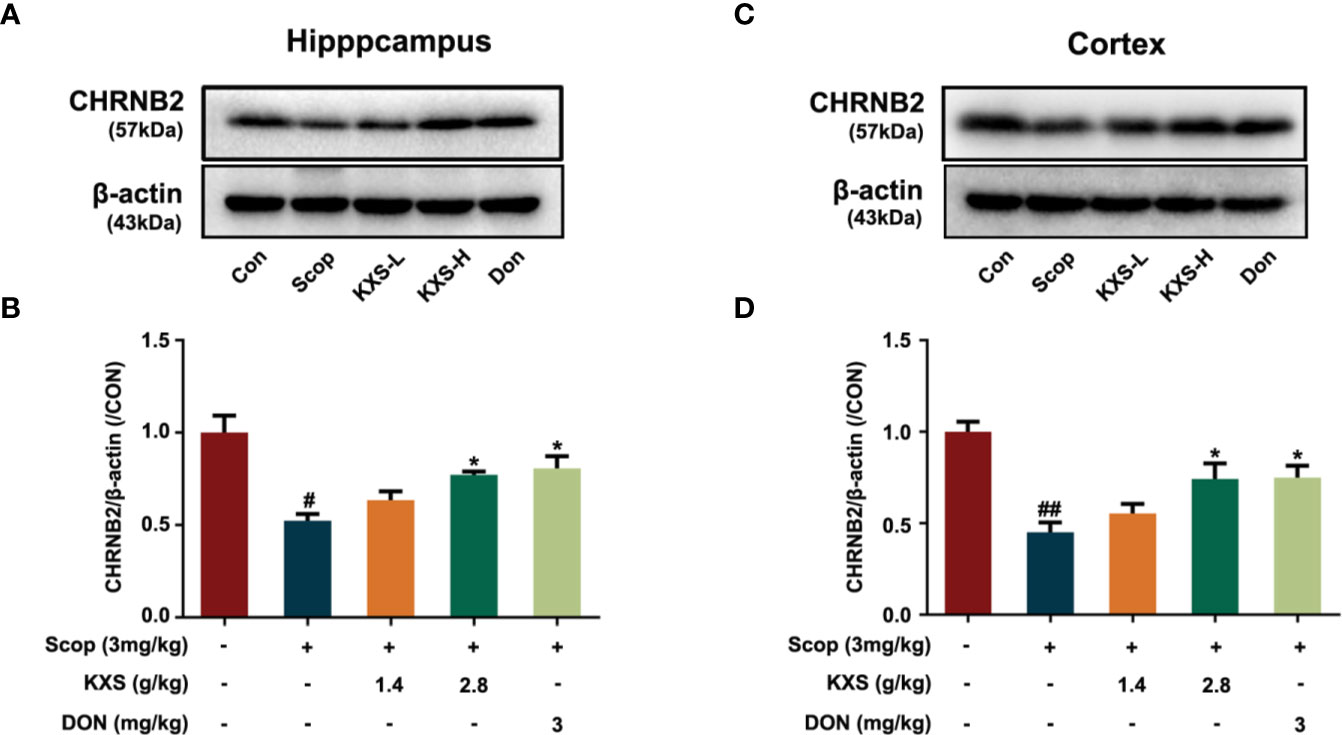
Figure 9 Kai-Xin-San (KXS) increases the expression of nicotinic acetylcholine receptor beta 2 (CHRNB2) in scopolamine (SCOP)-induce mice. Western blot analysis of the protein expression of CHRNB2 in the hippocampus (A) and cortex (C). Quantification of CHRNB2 level in panel (A, B) or (C, D) was normalized to that of actin. Data represent the means ± SEM (n=3, #P < 0.05, ##P < 0.01 versus the control group; *P < 0.05 versus the SCOP group).
Conclusion
To date, acetylcholinesterase inhibitors and NMDA receptor antagonists have been the primary choice for treating AD patients, showing marginal benefits for alleviating symptoms. The side effects are not ignorable and add to the ongoing AD burden. Therefore, it is urgent that novel and efficient curative remedies are discovered for AD patients. TCM efficacy in medical practice has been demonstrated for thousands of years. In contrast to western medicine, TCM formulas emphasize the regulation of interactions among all illness-associated elements within the abnormal body toward a balanced/normal condition. However, as TCM formulas contain hundreds of chemical components, the specific pharmacological mechanisms through which TCM prescriptions exert their effects against diseases are still difficult to illustrate.
KXS, a TCM formula that has been used for thousands of years in China to treat cognitive dysfunction, has been shown to improve learning and memory in animals studies. In this study, we developed an integrative systems pharmacology approach to illustrate the therapeutic mechanisms of KXS against AD. For the first time, we identified 39 AD-associated targets of KXS ingredients using known target mapping and in silico target prediction. Furthermore, we deciphered potential MOAs of KXS in AD treatment via a multiple data integration analysis, including an herb-target network analysis, compound-target network analysis, synergic action analysis, and integrated pathway analysis. Our systems pharmacology analysis was validated in in vivo experiments. The results showed that KXS could ameliorate cognitive dysfunction mainly through inhibiting the inflammation of microglia in SCOP-induced mice. More importantly, we found that the therapeutic effect of alleviating cholinergic system dysfunction involves the upregulation of the cholinergic receptor CHRNB2. Overall, these findings indicate that systems pharmacology could provide an alternative approach for exploring the complex MOAs of TCM and advance the comprehensive understanding of TCM.
Data Availability Statement
All datasets generated for this study are included in the article/Supplementary Material.
Ethics Statement
The animal study was reviewed and approved by Guangzhou University of Chinese Medicine Animal Ethics Committee.
Author Contributions
SF and JF conceived and designed the experiments. YuL and DL conducted the experiments and wrote the manuscript. QWu, YaL, CC, HL, HH, YX and XL provided and analyzed some of the data. HK, QWa, JF, and SF reviewed and revised the manuscript.
Funding
This work was supported by the National Natural Science Foundation of China (Nos. 81603318, 81673627, and 81904265), the Youth Scientific Research Training Project of GZUCM (No. 2019QNPY05), the Guangzhou Science Technology and Innovation Commission Technology Research Projects (No. 201805010005), and Key R & D and extension projects in Henan Province (No. 192102310166).
Conflict of Interest
The authors declare that the research was conducted in the absence of any commercial or financial relationships that could be construed as a potential conflict of interest.
Supplementary Material
The Supplementary Material for this article can be found online at: https://www.frontiersin.org/articles/10.3389/fphar.2020.00381/full#supplementary-material
Supplementary Datasheet S1 | The detailed information of compounds of KXS and their corresponding targe.
Supplementary Datasheet S2 | The detailed information of C-T network.
References
Arvanitakis, Z., Shah, R. C., Bennett, D. A. (2019). Diagnosis and Management of Dementia: Review. JAMA 322, 1589–1599. doi: 10.1001/jama.2019.4782
Barage, S. H., Sonawane, K. D. (2015). Amyloid cascade hypothesis: Pathogenesis and therapeutic strategies in Alzheimer's disease. Neuropeptides 52, 1–18. doi: 10.1016/j.npep.2015.06.008
Basu, A., Krady, J. K., Levison, S. W. (2004). Interleukin-1: a master regulator of neuroinflammation. J. Neurosci. Res. 78, 151–156. doi: 10.1002/jnr.20266
Cai, H., Luo, Y., Yan, X., Ding, P., Huang, Y., Fang, S., et al. (2018). The Mechanisms of Bushen-Yizhi Formula as a Therapeutic Agent against Alzheimer's Disease. Sci. Rep. 8, 3104. doi: 10.1038/s41598-018-21468-w
Calsolaro, V., Edison, P. (2016). Neuroinflammation in Alzheimer's disease: Current evidence and future directions. Alzheimers Dement. 12, 719–732. doi: 10.1016/j.jalz.2016.02.010
Cao, Y., Hu, Y., Liu, P., Zhao, H. X., Zhou, X. J., Wei, Y. M. (2012). Effects of a Chinese traditional formula Kai Xin San (KXS) on chronic fatigue syndrome mice induced by forced wheel running. J. Ethnopharmacol. 139, 19–25. doi: 10.1016/j.jep.2011.08.030
Cao, C., Xiao, J., Liu, M., Ge, Z., Huang, R., Qi, M., et al. (2018a). Active components, derived from Kai-xin-san, a herbal formula, increase the expressions of neurotrophic factor NGF and BDNF on mouse astrocyte primary cultures via cAMP-dependent signaling pathway. J. Ethnopharmacol. 224, 554–562. doi: 10.1016/j.jep.2018.06.007
Cao, J., Hou, J., Ping, J., Cai, D. (2018b). Advances in developing novel therapeutic strategies for Alzheimer's disease. Mol. Neurodegener. 13, 64. doi: 10.1186/s13024-018-0299-8
Chang, R., Yee, K.-L., Sumbria, R. K. (2017). Tumor necrosis factor α Inhibition for Alzheimer's Disease. J. Cent. Nerv. Syst. Dis. 9, 1179573517709278. doi: 10.1177/1179573517709278
Chen, C. Y.-C. (2011). TCM Database@Taiwan: the world's largest traditional Chinese medicine database for drug screening in silico. PloS One 6, e15939. doi: 10.1371/journal.pone.0015939
Cheng, X., Shen, Y., Li, R. (2014). Targeting TNF: a therapeutic strategy for Alzheimer's disease. Drug Discovery Today 19, 1822–1827. doi: 10.1016/j.drudis.2014.06.029
Chu, J., Lauretti, E., Pratico, D. (2017). Caspase-3-dependent cleavage of Akt modulates tau phosphorylation via GSK3beta kinase: implications for Alzheimer's disease. Mol. Psychiatry 22, 1002–1008. doi: 10.1038/mp.2016.214
Congdon, E. E., Sigurdsson, E. M. (2018). Tau-targeting therapies for Alzheimer disease. Nat. Rev. Neurol. 14, 399–415. doi: 10.1038/s41582-018-0013-z
Cook, L. J., Ho, L. W., Taylor, A. E., Brayne, C., Evans, J. G., Xuereb, J., et al. (2004). Candidate gene association studies of the alpha 4 (CHRNA4) and beta 2 (CHRNB2) neuronal nicotinic acetylcholine receptor subunit genes in Alzheimer's disease. Neurosci. Lett. 358, 142–146. doi: 10.1016/j.neulet.2004.01.016
Cooper, E. L., Ma, M. J. (2017). Alzheimer Disease: Clues from traditional and complementary medicine. J. Tradit. Complement Med. 7, 380–385. doi: 10.1016/j.jtcme.2016.12.003
Decourt, B., Lahiri, D. K., Sabbagh, M. N. (2017). Targeting Tumor Necrosis Factor Alpha for Alzheimer's Disease. Curr. Alzheimer Res. 14, 412–425. doi: 10.2174/1567205013666160930110551
Dobrowolska Zakaria, J. A., Vassar, R. J. (2018). A promising, novel, and unique BACE1 inhibitor emerges in the quest to prevent Alzheimer's disease. EMBO Mol. Med. 10. e9717. doi: 10.15252/emmm.201809717
El Kadmiri, N., Slassi, I., El Moutawakil, B., Nadifi, S., Tadevosyan, A., Hachem, A., et al. (2014). Glyceraldehyde-3-phosphate dehydrogenase (GAPDH) and Alzheimer's disease. Pathol. Biol. (Paris) 62, 333–336. doi: 10.1016/j.patbio.2014.08.002
Fang, J., Wang, L., Wu, T., Yang, C., Gao, L., Cai, H., et al. (2017a). Network pharmacology-based study on the mechanism of action for herbal medicines in Alzheimer treatment. J. Ethnopharmacol. 196, 281–292. doi: 10.1016/j.jep.2016.11.034
Fang, J., Wu, Z., Cai, C., Wang, Q., Tang, Y., Cheng, F. (2017b). Quantitative and Systems Pharmacology. 1. In Silico Prediction of Drug-Target Interactions of Natural Products Enables New Targeted Cancer Therapy. J. Chem. Inf. Model 57, 2657–2671. doi: 10.1021/acs.jcim.7b00216
Fang, J., Cai, C., Chai, Y., Zhou, J., Huang, Y., Gao, L., et al. (2019). Quantitative and systems pharmacology 4. Network-based analysis of drug pleiotropy on coronary artery disease. Eur. J. Med. Chem. 161, 192–204. doi: 10.1016/j.ejmech.2018.10.020
Ferreira-Vieira, T. H., Guimaraes, I. M., Silva, F. R., Ribeiro, F. M. (2016). Alzheimer's disease: Targeting the Cholinergic System. Curr. Neuropharmacol. 14, 101–115. doi: 10.2174/1570159X13666150716165726
Gao, H. L., Zhang, A. H., Yu, J. B., Sun, H., Kong, L., Wang, X. Q., et al. (2018). High-throughput lipidomics characterize key lipid molecules as potential therapeutic targets of Kaixinsan protects against Alzheimer's disease in APP/PS1 transgenic mice. J. Chromatogr. B. Analyt. Technol. BioMed. Life Sci. 1092, 286–295. doi: 10.1016/j.jchromb.2018.06.032
Gaugler, J., Al, E. (2019). 2019 Alzheimer's disease facts and figures. Alzheimer's Dement. 15, 321–387. doi: 10.1016/j.jalz.2019.01.010
Gerszon, J., Rodacka, A. (2018). Oxidatively modified glyceraldehyde-3-phosphate dehydrogenase in neurodegenerative processes and the role of low molecular weight compounds in counteracting its aggregation and nuclear translocation. Ageing Res. Rev. 48, 21–31. doi: 10.1016/j.arr.2018.09.003
Hampel, H., Mesulam, M. M., Cuello, A. C., Farlow, M. R., Giacobini, E., Grossberg, G. T., et al. (2018). The cholinergic system in the pathophysiology and treatment of Alzheimer's disease. Brain 141, 1917–1933. doi: 10.1093/brain/awy132
He, M., Yan, X., Zhou, J., Xie, G. (2001). Traditional Chinese Medicine Database and Application on the Web. J. Chem. Inf. Comput. Sci. 41, 273–277. doi: 10.1021/ci0003101
He, X. Y., Isaacs, C., Yang, S. Y. (2018). Roles of Mitochondrial 17beta-Hydroxysteroid Dehydrogenase Type 10 in Alzheimer's Disease. J. Alzheimers Dis. 62, 665–673. doi: 10.3233/JAD-170974
Heneka, M. T., Carson, M. J., El Khoury, J., Landreth, G. E., Brosseron, F., Feinstein, D. L., et al. (2015). Neuroinflammation in Alzheimer's disease. Lancet Neurol. 14, 388–405. doi: 10.1016/S1474-4422(15)70016-5
Ho, Y. S., So, K. F., Chang, R. C. (2010). Anti-aging herbal medicine–how and why can they be used in aging-associated neurodegenerative diseases? Ageing Res. Rev. 9, 354–362. doi: 10.1016/j.arr.2009.10.001
Huang Da, W., Sherman, B. T., Lempicki, R. A. (2009). Systematic and integrative analysis of large gene lists using DAVID bioinformatics resources. Nat. Protoc. 4, 44–57. doi: 10.1038/nprot.2008.211
Jia, J., Wei, C., Chen, S., Li, F., Tang, Y., Qin, W., et al (2018). The cost of Alzheimer's disease in China and re-estimation of costs worldwide. Alzheimers Dement. 14, 483–491.
Jiang, Y., Gao, H., Turdu, G. (2017). Traditional Chinese medicinal herbs as potential AChE inhibitors for anti-Alzheimer's disease: A review. Bioorg. Chem. 75, 50–61. doi: 10.1016/j.bioorg.2017.09.004
Jouanne, M., Rault, S., Voisin-Chiret, A. S. (2017). Tau protein aggregation in Alzheimer's disease: An attractive target for the development of novel therapeutic agents. Eur. J. Med. Chem. 139, 153–167. doi: 10.1016/j.ejmech.2017.07.070
Karthivashan, G., Park, S. Y., Kweon, M. H., Kim, J., Haque, M. E., Cho, D. Y., et al. (2018). Ameliorative potential of desalted Salicornia europaea L. extract in multifaceted Alzheimer's-like scopolamine-induced amnesic mice model. Sci. Rep. 8, 7174. doi: 10.1038/s41598-018-25381-0
Khan, M. A., Alam, Q., Haque, A., Ashafaq, M., Khan, M. J., Ashraf, G. M., et al. (2019). Current Progress on Peroxisome Proliferator-activated Receptor Gamma Agonist as an Emerging Therapeutic Approach for the Treatment of Alzheimer's Disease: An Update. Curr. Neuropharmacol. 17, 232–246. doi: 10.2174/1570159X16666180828100002
Kim, S. K., Nam, S., Jang, H., Kim, A., Lee, J. J. (2015). TM-MC: a database of medicinal materials and chemical compounds in Northeast Asian traditional medicine. BMC Complement Altern. Med. 15, 218. doi: 10.1186/s12906-015-0758-5
Kinney, J. W., Bemiller, S. M., Murtishaw, A. S., Leisgang, A. M., Salazar, A. M., Lamb, B. T. (2018). Inflammation as a central mechanism in Alzheimer's disease. Alzheimers Dement. (N Y) 4, 575–590. doi: 10.1016/j.trci.2018.06.014
Kitazawa, M., Cheng, D., Tsukamoto, M. R., Koike, M. A., Wes, P. D., Vasilevko, V., et al. (2011). Blocking IL-1 signaling rescues cognition, attenuates tau pathology, and restores neuronal beta-catenin pathway function in an Alzheimer's disease model. J. Immunol. 187, 6539–6549. doi: 10.4049/jimmunol.1100620
Kumar, A., Tsao, J. W. (2019). “Alzheimer Disease,” in StatPearls (Treasure Island (FL): StatPearls Publishing LLC).
Law, B. Y. K., Wu, A. G., Wang, M. J., Zhu, Y. Z. (2017). Chinese Medicine: A Hope for Neurodegenerative Diseases? J. Alzheimers Dis. 60, S151–s160. doi: 10.3233/JAD-170374
Lee, S., Park, H. J., Jeon, S. J., Kim, E., Lee, H. E., Kim, H., et al. (2017). Cognitive Ameliorating Effect of Acanthopanax koreanum Against Scopolamine-Induced Memory Impairment in Mice. Phytother. Res. 31, 425–432. doi: 10.1002/ptr.5764
Liu, H., Wang, L., Lv, M., Pei, R., Li, P., Pei, Z., et al. (2014). AlzPlatform: an Alzheimer's disease domain-specific chemogenomics knowledgebase for polypharmacology and target identification research. J. Chem. Inf. Model 54, 1050–1060. doi: 10.1021/ci500004h
Lu, C., Shi, Z., Sun, X., Pan, R., Chen, S., Li, Y., et al. (2017). Kai Xin San aqueous extract improves Abeta1-40-induced cognitive deficits on adaptive behavior learning by enhancing memory-related molecules expression in the hippocampus. J. Ethnopharmacol. 201, 73–81. doi: 10.1016/j.jep.2016.10.002
Mao, P., Manczak, M., Calkins, M. J., Truong, Q., Reddy, T. P., Reddy, A. P., et al. (2012). Mitochondria-targeted catalase reduces abnormal APP processing, amyloid beta production and BACE1 in a mouse model of Alzheimer's disease: implications for neuroprotection and lifespan extension. Hum. Mol. Genet. 21, 2973–2990. doi: 10.1093/hmg/dds128
Masters, C. L., Bateman, R., Blennow, K., Rowe, C. C., Sperling, R. A., Cummings, J. L. (2015). Alzheimer's disease. Nat. Rev. Dis. Primers 1, 15056. doi: 10.1038/nrdp.2015.56
Montgomery, S. L., Bowers, W. J. (2012). Tumor necrosis factor-alpha and the roles it plays in homeostatic and degenerative processes within the central nervous system. J. Neuroimmune Pharmacol. 7, 42–59. doi: 10.1007/s11481-011-9287-2
Moussa-Pacha, N. M., Abdin, S. M., Omar, H. A., Alniss, H., Al-Tel, T. H. (2019). BACE1 inhibitors: Current status and future directions in treating Alzheimer's disease. Med. Res. Rev. 40, 339–384. doi: 10.1002/med.21622
Muhammad, T., Ali, T., Ikram, M., Khan, A., Alam, S. I., Kim, M. O. (2019). Melatonin Rescue Oxidative Stress-Mediated Neuroinflammation/ Neurodegeneration and Memory Impairment in Scopolamine-Induced Amnesia Mice Model. J. Neuroimmune Pharmacol. 14, 278–294. doi: 10.1007/s11481-018-9824-3
O'boyle, N. M., Banck, M., James, C. A., Morley, C., Vandermeersch, T., Hutchison, G. R. (2011). Open Babel: An open chemical toolbox. J. Cheminf. 3, 33. doi: 10.1186/1758-2946-3-33
Ozben, T., Ozben, S. (2019). Neuro-inflammation and anti-inflammatory treatment options for Alzheimer's disease. Clin. Biochem. 72, 87–89. doi: 10.1016/j.clinbiochem.2019.04.001
Peters, F., Salihoglu, H., Pratsch, K., Herzog, E., Pigoni, M., Sgobio, C., et al. (2019). Tau deletion reduces plaque-associated BACE1 accumulation and decelerates plaque formation in a mouse model of Alzheimer's disease. EMBO J. 38, e102345. doi: 10.15252/embj.2019102345
Pinero, J., Bravo, A., Queralt-Rosinach, N., Gutierrez-Sacristan, A., Deu-Pons, J., Centeno, E., et al. (2017). DisGeNET: a comprehensive platform integrating information on human disease-associated genes and variants. Nucleic Acids Res. 45, D833–D839. doi: 10.1093/nar/gkw943
Reddy, P. H., Tripathi, R., Troung, Q., Tirumala, K., Reddy, T. P., Anekonda, V., et al. (2012). Abnormal mitochondrial dynamics and synaptic degeneration as early events in Alzheimer's disease: implications to mitochondria-targeted antioxidant therapeutics. Biochim. Biophys. Acta 1822, 639–649. doi: 10.1016/j.bbadis.2011.10.011
Rothaug, M., Becker-Pauly, C., Rose-John, S. (2016). The role of interleukin-6 signaling in nervous tissue. Biochim. Biophys. Acta 1863, 1218–1227. doi: 10.1016/j.bbamcr.2016.03.018
Ru, J., Li, P., Wang, J., Zhou, W., Li, B., Huang, C., et al. (2014). TCMSP: a database of systems pharmacology for drug discovery from herbal medicines. J. Cheminf. 6, 13. doi: 10.1186/1758-2946-6-13
Selkoe, D. J., Hardy, J. (2016). The amyloid hypothesis of Alzheimer's disease at 25 years. EMBO Mol. Med. 8, 595–608. doi: 10.15252/emmm.201606210
Sen, T., Saha, P., Sen, N. (2018). Nitrosylation of GAPDH augments pathological tau acetylation upon exposure to amyloid-beta. Sci. Signal 11, eaao6765. doi: 10.1126/scisignal.aao6765
Simic, G., Babic Leko, M., Wray, S., Harrington, C., Delalle, I., Jovanov-Milosevic, N., et al. (2016). Tau Protein Hyperphosphorylation and Aggregation in Alzheimer's Disease and Other Tauopathies, and Possible Neuroprotective Strategies. Biomolecules 6, 6. doi: 10.3390/biom6010006
Tachida, Y., Nakagawa, K., Saito, T., Saido, T. C., Honda, T., Saito, Y., et al. (2008). Interleukin-1 beta up-regulates TACE to enhance alpha-cleavage of APP in neurons: resulting decrease in Abeta production. J. Neurochem. 104, 1387–1393. doi: 10.1111/j.1471-4159.2007.05127.x
Wang, Y., Mandelkow, E. (2015). Tau in physiology and pathology. Nat. Rev. Neurosci. 17, 22. doi: 10.1038/nrn.2015.1
Wang, N., Jia, Y. M., Zhang, B., Xue, D., Reeju, M., Li, Y., et al. (2017). Neuroprotective mechanism of Kai Xin San: upregulation of hippocampal insulin-degrading enzyme protein expression and acceleration of amyloid-beta degradation. Neural Regener. Res. 12, 654–659. doi: 10.4103/1673-5374.205107
Wu, Z., Lu, W., Wu, D., Luo, A., Bian, H., Li, J., et al. (2016). In silico prediction of chemical mechanism of action via an improved network-based inference method. Br. J. Pharmacol. 173, 3372–3385. doi: 10.1111/bph.13629
Xu, Y.-M., Wang, X.-C., Xu, T.-T., Li, H.-Y., Hei, S.-Y., Luo, N.-C., et al. (2019). Kai Xin San ameliorates scopolamine-induced cognitive dysfunction. Neural Regener. Res. 14, 794–804. doi: 10.4103/1673-5374.249227
Xue, R., Fang, Z., Zhang, M., Yi, Z., Wen, C., Shi, T. (2013). TCMID: Traditional Chinese Medicine integrative database for herb molecular mechanism analysis. Nucleic Acids Res. 41, D1089–D1095. doi: 10.1093/nar/gks1100
Yamamoto, M., Kiyota, T., Horiba, M., Buescher, J. L., Walsh, S. M., Gendelman, H. E., et al. (2007). Interferon-gamma and tumor necrosis factor-alpha regulate amyloid-beta plaque deposition and beta-secretase expression in Swedish mutant APP transgenic mice. Am. J. Pathol. 170, 680–692. doi: 10.2353/ajpath.2007.060378
Yang, S. Y., He, X. Y., Isaacs, C., Dobkin, C., Miller, D., Philipp, M. (2014). Roles of 17beta-hydroxysteroid dehydrogenase type 10 in neurodegenerative disorders. J. Steroid Biochem. Mol. Biol. 143, 460–472. doi: 10.1016/j.jsbmb.2014.07.001
Zhang, R. Z., Yu, S. J., Bai, H., Ning, K. (2017). TCM-Mesh: The database and analytical system for network pharmacology analysis for TCM preparations. Sci. Rep. 7, 2821. doi: 10.1038/s41598-017-03039-7
Zhao, J., Li, X., Chen, X., Cai, Y., Wang, Y., Sun, W., et al. (2019). GRK5 influences the phosphorylation of tau via GSK3beta and contributes to Alzheimer's disease. J. Cell Physiol. 234, 10411–10420. doi: 10.1002/jcp.27709
Keywords: systems pharmacology, Kai-Xin-San, Alzheimer's disease, cholinergic system, neuroinflammation
Citation: Luo Y, Li D, Liao Y, Cai C, Wu Q, Ke H, Liu X, Li H, Hong H, Xu Y, Wang Q, Fang J and Fang S (2020) Systems Pharmacology Approach to Investigate the Mechanism of Kai-Xin-San in Alzheimer’s Disease. Front. Pharmacol. 11:381. doi: 10.3389/fphar.2020.00381
Received: 28 November 2019; Accepted: 12 March 2020;
Published: 03 April 2020.
Edited by:
Yonghua Wang, Northwest A&F University, ChinaReviewed by:
Jianxin Chen, Beijing University of Chinese Medicine, ChinaShiv Bahadur, GLA University, India
Copyright © 2020 Luo, Li, Liao, Cai, Wu, Ke, Liu, Li, Hong, Xu, Wang, Fang and Fang. This is an open-access article distributed under the terms of the Creative Commons Attribution License (CC BY). The use, distribution or reproduction in other forums is permitted, provided the original author(s) and the copyright owner(s) are credited and that the original publication in this journal is cited, in accordance with accepted academic practice. No use, distribution or reproduction is permitted which does not comply with these terms.
*Correspondence: Qi Wang, d2FuZ3FpQGd6dWNtLmVkdS5jbg==; Jiansong Fang, ZmFuZ2pzQGd6dWNtLmVkdS5jbg==; Shuhuan Fang, ZmFuZ3NodWh1YW5AZ3p1Y20uZWR1LmNu
†These authors have contributed equally to this work