- 1Laboratory of Molecular Genetics of Intracellular Transport, Institute of Gene Biology, Russian Academy of Sciences, Moscow, Russia
- 2Department of Biophysics, Faculty of Biology, Lomonosov Moscow State University, Moscow, Russia
Since cell nucleus is one of the most vulnerable compartments, the maximum therapeutic effect from a variety of locally acting agents, such as photosensitizers, alfa-emitters, Auger electron emitters, will be expected when they get there. Therefore, the targeted delivery of these agents into the nuclei of target tumor cells is necessary for their anticancer effects and minimization of side effects. Modular nanotransporters (MNT) are artificial polypeptides comprising several predefined modules that recognize target cell, launching their subsequent internalization, escape from endosomes, and transport the drug load to the nucleus. This technology significantly enhances the cytotoxicity of locally acting drugs in vitro and in vivo. Epidermal growth factor receptors (EGFR) are useful molecular targets as they are overexpressed in glioblastoma, head-and-neck cancer, bladder cancer, and other malignancies. Here, we examined the possibility of using internalizable anti-EGFR affibody as an EGFR-targeting MNT module for drug transport into the cancer cell nuclei. It binds to both murine and human EGFR facilitating preclinical studies. We showed that MNT with affibody on the N-terminus (MNTN-affibody) effectively delivered the Auger electron emitter 111In to target cell nuclei and had pronounced cytotoxic efficacy against EGFR-overexpressing human A431 epidermoid carcinoma cells. Using EGFR-expressing human adenocarcinoma MCF-7 cells, we demonstrated that in contrast to MNT with N-terminal epidermal growth factor (EGF), MNTN-affibody and MNT with EGF on the C-terminus did not stimulate cancer cell proliferation.
Introduction
Today a great interest in pharmacology sphere is the design of drugs that have a local damaging effect and are able to selectively uptake by the target cells (Rosenblum et al., 2018; Sobolev, 2018). Locally acting cytotoxic agents, in particular Auger electron emitters, have great therapeutic potential that can be used to antitumor treatment. These agents are able to damage biomolecules within a few tens of nanometers from their location but have no effect at large distances. Different cell compartments have different resistance to certain damaging agents; the cell nucleus is the most vulnerable compartments to many of them. It determines the importance of development targeted systems for their delivery to reach a significant effect without damaging non-target cells. This approach would minimize side effects and increase the effectiveness of treatment with reducing the minimum required concentration of the drug. Research in this area, in particular, is aimed at creating different intranuclear delivery systems conjugated with locally acting cytotoxic compounds (such as Auger electron emitters, photosensitizers, etc.) (Pan et al., 2018). One such approach is the creation of conjugates with EGF, one of the natural ligands to EGFR (Reilly et al., 2000; Cornelissen et al., 2013).
EGFR, also known as HER1 and ErbB1, has received much attention as a marker, growth driver, and therapeutic target for cancers (Nicholson et al., 2001; Ciardiello and Tortora, 2008; Xu et al., 2017; Sigismund et al., 2018). EGF and several other natural ligands activate EGFR and its downstream signaling pathways (Wang, 2017). Induction of these pro-oncogenic pathways stimulates cancer cell proliferation, migration, survival, DNA-double strand break repair, and hypoxia tolerance, mediates resistance to therapy and inhibits apoptosis (Rodemann et al., 2007; Bussink et al., 2008, Sigismund et al., 2018). Upregulation of this receptor may cause malignization as EGFR plays an important role in the regulation of cell division (Wang, 2017). Abnormal EGFR overexpression and signaling are associated with malignant tumors of the lung (Hirsch et al., 2009), pancreas (Lemoine et al., 1992; Ueda et al., 2004), brain (Hicks et al., 2006), bladder (Chow et al., 1997; Hashmi et al., 2018), breast (Wang et al., 2017), prostate (Peraldo-Neia et al., 2011), and other cancers (Mendelsohn and Baselga, 2000; Ciardiello and Tortora, 2008). EGFR, upon binding to its ligand, is not only capable to activate other signaling proteins, but is also transported inside endosomes from the surface of the cell membrane (Sorkin and Goh, 2009). It has been shown for tumor cells that part of ligand-bound EGF translocates into the cell nuclei (Reilly et al., 2000; Hicks et al., 2006; De Angelis Campos et al., 2011; Sobolev, 2018). Based on the data obtained, numerous attempts have been made to create a drug where EGFR would be used as a vehicle for various agents, such as, for example, Auger-electron emitter 111In, Staphylococcal enterotoxin A, etc. Several therapeutic approaches exploiting EGFR for this purpose have been evaluated in vitro and in vivo (Chen et al., 2002; Song et al., 2016; Zahaf et al., 2017; Liu et al., 2018).
However, since the part of the EGF transported to the nucleus is extremely small (about 7–8%) compared to the cell-bound EGF within 4 h after adding (Reilly et al., 2000), another method should be developed to increase the efficiency of delivery of the cytotoxic agent to the cell nuclei. One of these developments is the modular nanotransporters (MNT) designed in our laboratory (Gilyazova et al., 2006; Rosenkranz et al., 2018). MNT were designed to deliver locally acting drugs such as photosensitizers and radionuclide-emitting short-range particles to the nuclei of the target cells (Sobolev, 2008; Sobolev et al., 2016; Sobolev, 2018). We used Auger electron emitters, because they combine two important characteristics. On the one hand, Auger electrons have a high linear energy transfer, which leads to multiple damage of macromolecules, and on the other hand, their path length is extremely small and in most cases does not exceed several tens of nanometers, which greatly reduces the cytotoxicity of Auger electron emitters for tissues if decay occurs outside the cell nucleus (Kassis and Adelstein, 2005).
We designed an EGFR-recognizing modular nanotransporter consisting of EGF as a ligand module for selective recognition of target cells overexpressing EGFR, an endosomolytic module based on the translocation domain of the diphtheria toxin, a module containing an optimized nuclear localization sequence (NLS) of the SV40 large T antigen for active nuclear transport by the importin-α/β carrier protein complex (Goldfarb et al., 2004), and a carrier module based on the E. coli hemoglobin-like protein HMP (Rosenkranz et al., 2008). Scheme of the MNT transport into the cell nucleus of a target cell is depicted in Figure 1.
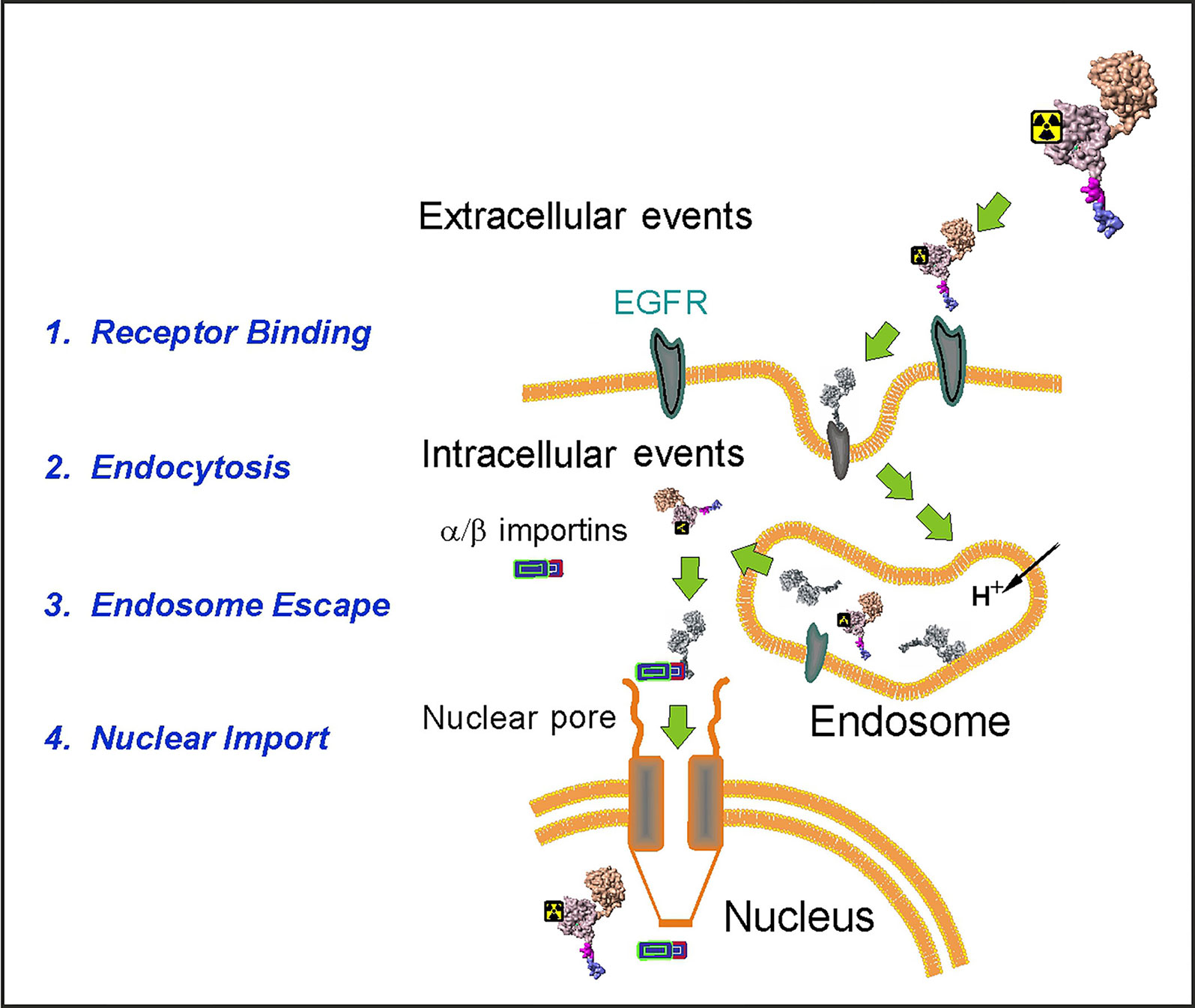
Figure 1 A schematic diagram depicting the stages of the MNT transport to the cell nucleus of the EGFR-expressing target cells. (Reproduced with slight modification from Sobolev, 2008 Bioessays. 2008 Mar;30(3):278-87. doi: 10.1002/bies.20715, with permission).
This modular nanotransporter is named DTox-HMP-NLS-EGF (or, MNTC-EGF, where C-EGF indicates that EGF is localized to the C-terminal in the MNT). The experiments with this MNT were carried out on several cell lines in particular on human A431 epidermoid carcinoma cells. It was shown that MNTC-EGF efficiently delivered drugs to the cell nucleus of the target cells and enhanced their cytotoxic efficacy in vitro (Gilyazova et al., 2006; Rosenkranz et al., 2008; Slastnikova et al., 2012a; Koumarianou et al., 2014; Slastnikova et al., 2017b; Rosenkranz et al., 2018) and in vivo (Slastnikova et al., 2012a; Slastnikova et al., 2012b; Slastnikova et al., 2017b; Rosenkranz et al., 2018). Thus, in in vitro experiments it was shown that the concentration of the drug agent (Auger electron emitters 111In, 67Ga, 125I, or alpha-particle emitter 211At or photosensitizers bacteriochlorin p or chlorin e6) corresponding to 50% survival of cancer cells was up to 3000 times higher for free chlorin e6 or corresponding to 37% survival up to 4000 times for 125I compared to MNT-agent conjugates (Gilyazova et al., 2006; Rosenkranz et al., 2008; Slastnikova et al., 2012a; Koumarianou et al., 2014; Slastnikova et al., 2017b). In vivo experiments on tumor-bearing mice proved that locoregional injection of MNTC-EGF conjugated with 111In resulted in significant tumor growth inhibition compared to tumor-bearing animals receiving a corresponding dose of non-labeled MNT or free 111In (Rosenkranz et al., 2018).
When EGF is used as a ligand in drug delivery vehicles, it can affect cellular signaling and stimulates events leading to enhances cancer cell proliferation (Chen et al., 2002; Kim et al., 2015). EGF and other natural ligands induce dimerization and autophosphorylation of EGFR (Schlessinger, 2002) and subsequent internalization (Friedman and Stahl, 2009) of the receptor. Downstream activation of the phosphatidylinositol-3-kinase (PI3-K)/protein kinase B (AKT) (PI3-K/AKT pathway), Ras and STAT signaling cascades (Citri and Yarden, 2006; Rodemann et al., 2007) leads to stimulation of proliferation, inhibit apoptosis, and promote migration (Seshacharyulu et al., 2012). In order to avoid ligand-mediated activation of the downstream cascades researchers developed alternative EGFR ligands such as EGFt, a truncated form of human EGF (hEGF) lacking the eight C-terminal amino acids (Panosa et al., 2015), artificial EGFR ligands such as small antibody-like protein on the basis of Z-domain of A-protein: anti-EGFR affibody (ZEGFR:1907) (Kim et al., 2012; Stahl et al., 2017), and anti-EGFR nanobody (Roovers et al., 2007).
In our previous experiments we used MNT with EGF at the C-terminus (MNTC-EGF) and revealed that it had no effect on cell growth (Rosenkranz et al., 2018). However, as in vivo degradation of the EGF-containing MNT potentially can lead to EGFR-activating products, we examined the possibility of using a new MNT with the affibody to EGFR. Affibody Z1907 does not influence on cell proliferation (Ekerljung et al., 2012), so we tested it as a ligand module. Based on the findings of earlier studies (Kim et al., 2012; Kim et al., 2013), we placed the affibody on the N-terminus of the MNT. Therefore, another reason of creating the new MNT was a palette extension, which would expand the possibility of modification of the MNT with additional functional modules. MNTC-EGF and the new MNT with EGF at the N-terminus (MNTN-EGF) served as controls. The effects of these MNT on the proliferation of cells overexpressing surface EGFR were investigated. We also explored the ability of these MNT to deliver the cytotoxic agent 111In, an Auger-electron emitter, to target cell nuclei.
Materials and Methods
Materials
Human A431 epidermoid carcinoma and MCF-7 adenocarcinoma cells were obtained from the American Type Culture Collection (ATCC; Manassas, VA, USA). They were passaged in the laboratory less than 30 times. All media, cell culture components, and 3-[4,5-dimethylthiazol-2-yl]-2,5 diphenyltetrazolium bromide (MTT) were purchased from Paneco (Moscow, Russia). Escherichia сoli C3029 cells were purchased from New England Biolabs (Ipswich, MA, USA). Ni-NTA agarose was purchased from Qiagen (Hilden, Germany). Alexa Fluor 647 succinimidyl ester was purchased from Molecular Probes (Eugene, OR, USA). The bifunctional chelator 2-S-(4-isothiocyanatobenzyl)-1,4,7-triazacyclononane-1,4,7-triacetic acid (p-SCN-BnNOTA) was obtained from Macrocyclics (Plano, TX, USA). The 111InCl3 was obtained from Zavod Medradiopreparat (Moscow, Russia). The 125I was acquired from the Khlopin Radium Institute (Saint Petersburg, Russia). The 1,4-dithiothreitol (DTT) was purchased from Fisher Scientific (Schwerte, Germany). Mini-Protean® TGX Any kD™ gels were purchased from Bio-Rad Laboratories (Hercules, CA, USA). LB Broth Miller-Novagen, a granulated E. coli cultivation medium, was purchased from Helicon (Moscow, Russia). EGTA and EDTA were obtained from Serva (Heidelberg, Germany). Isopropyl β-D-1-thiogalactopyranoside (IPTG) and Amicon Ultracel-30K centrifugal filter units were procured from Merck (Darmstadt, Germany). Antibodies were purchased from Abcam (Cambridge, UK). SYBR Green was purchased from Lumiprobe (Moscow, Russia). 1,3,4,6-Tetrachloro-3α,6α-diphenylglycouril (Iodogen), KCl, NaHCO3, MgCl2, and CaCl2 were purchased from Sigma-Aldrich (St. Louis, MO, USA). Calcein was obtained from Fluka (Munich, Germany).
Methods
Cell Culture
Human A431 epidermoid carcinoma and MCF-7 adenocarcinoma cells overexpressing EGFR were maintained in Dulbecco’s modified Eagle’s medium (DMEM) supplemented with 10% calf fetal serum (CFS) and 50 μg ml−1 gentamicin at 37°C under a 5% CO2 humidified atmosphere.
Plasmid Construction and Protein Purification
A DNA fragment encoding EGF was amplified by PCR from the 6His-DTox-HMP-NLS-EGF (MNTC-EGF) gene and subcloned into a plasmid encoding 6His-DTox-HMP-NLS (Gilyazova et al., 2006). The product was EGF-6His-DTox-HMP-NLS (MNTN-EGF). The anti-EGFR affibody gene was synthesized by General Biosystems (Morrisville, NC, USA) based on a published amino acid sequence (Stahl et al., 2017), and subcloned into 6His-DTox-HMP-NLS. The product was the affibody-6His-DTox-HMP-NLS (MNTN-affibody) plasmid.
E. coli C3029 cells were transformed with MNTC-EGF, MNTN-EGF, or MNTN-affibody plasmids and grown on LB Broth Miller-Novagen with ampicillin (100 μg ml−1) to A600 = 0.6 at 37°C. They were induced overnight with 0.2 mM IPTG at 18°C. Proteins were purified with Ni-NTA from the soluble fraction of E. coli lysate as previously described (Gilyazova et al., 2006).
Binding of the MNT to the EGFR
Binding of the new MNT to EGFR was assessed on A431 cells by a competitive radioligand binding assay with 125I-labeled EGF as previously described using Iodogen (Rosenkranz et al., 2008; Slastnikova et al., 2012a).
EGF and 40 MBq of radioiodide in 0.05 M sodium borate buffer (pH 8.5) were incubated in glass vials coated with 10 μg of Iodogen for 15 min on ice. Radioiodinated EGF was purified by gel-filtration through a PD-10 column (GE Healthcare, Chicago, IL, USA) that was eluted with phosphate-buffered saline (pH 7.5).
The competitive radioligand analysis MNT binding was accomplished with A431 cells in 48-well plates overnight at 4°C with 1 nM of 125I-EGF and indicated concentrations of MNT in the DMEM medium without sodium bicarbonate supplemented with 10 mg/ml of bovine serum albumin and 20 mM 4-(2-hydroxyethyl)-1-piperazineethanesulfonic acid (pH 7.5). The cells were washed four times with the same medium on ice, lysed in 1 M NaOH for 30 min, and the radioactivity associated with the cell lysates was measured using RiaGamma counter 1271 (LKB Wallac, Sollentuna, Sweden).
Functional Activity of the Endosomolytic Module
The ability of the new MNT to provide liposome leakage was demonstrated on unilamellar liposomes from egg lecithin (Khimpharmzavod, Kharkov, Ukraine) loaded with the fluorescent dye calcein (Fluka, Munich, Germany) in accordance with a previously published protocol (Khramtsov et al., 2008). In brief, the unilamellar liposomes loaded with fluorescent calcein up to the concentration of fluorescence quenching (100 mM) were prepared by sonicating fresh lipid suspension in 20 mM HEPES, 20 mM MES, 20 mM citrate, 150 mM NaCl, pH 7.4 (liposome buffer) until clear, using a W-181-T sonicator (Finnsonik, Lahti, Finland; 40 kHz, 90 W, 0°C, 30 min), and passed 10 times through Durapore filters with 0.22 μm pore diameter (Millipore, Burlington, MA, USA) to standardize liposomes sizes. The liposomes were stored under an argon atmosphere at 4°C for several months. PD-10-purified liposomes were incubated with 100 nM MNT for 30 min in liposome buffer at indicated pH (3–7.5) in triplicates after that samples were diluted tenfold in liposome buffer, pH 7.5 and fluorescence of leaked calcein was measured at 520 nm at excitation wavelength 490 nm. As a positive control (100% calcein leakage) we used addition Triton X-100 up to 0.5%. The samples without MNT were used as background leakage.
Conjugation of Alexa Fluor 647 to MNT
Freshly prepared 9.8 mM Alexa Fluor 647 succinimidyl ester was added in 5:1 molar excess to MNTN-EGF, MNTN-affibody, MNTC-EGF, or ligand-free MNT solutions in carbonate buffer at pH 8.6. After overnight incubation with gentle stirring at 4°C, the Alexa Fluor 647-labeled MNT were separated from the unreacted fluorophore by five cycles of Amicon Ultracel-30K ultrafiltration. The quantification of Alexa Fluor 647 MNT labeling (near 3.5 Alexa residues per MNT for all of them) was carried out by spectrophotometry. Alexa Fluor 647 extinction coefficient ϵ650nm = 270,000 M–1 cm–1. The MNT protein concentration was determined by the Bradford assay.
Flow Cytometry Studies of Alexa Fluor 647-labeled MNT Internalization in EGFR-expressing Cells
EGFR-expressing A431 cells were seeded in 24-well plates (2.5 × 104 cells/well), a couple days afterward the medium was changed for a fresh one and AlexaFluor 647 labeled MNT were added (n = 4 per each point) to a final concentration of 100 nM. After 18-h incubation at 37°C in a 5% CO2 humidified atmosphere, medium containing unbound MNT was removed and the cells were washed thrice, trypsinized to detach and remove cell-surface bound MNT, harvested, dissolved in Hanks’ solution with CFS and analyzed by flow cytometry using an Epics Altra Flow Cytometer (Beckman Coulter, Miami, Florida, USA). The Alexa Fluor 647 dye was excited at 633 nm, and emission was detected at 675 nm. A total of 1 × 104 gated events were collected per sample. To assess the nonspecific uptake parallel wells (n = 4 per each point) with free EGF excess (2 μM) were processed in the same way. Untreated cells were used as an autofluorescence control.
Confocal Laser-Scanning Microscopy Imaging
EGFR-expressing A431 cells were seeded in 24-well cell imaging black plates with glass bottoms (1.5 × 104 cells well−1). After 2 d, the medium was changed, and Alexa Fluor 647-labeled MNT were added to final concentrations of 50 nM.
Prior to imaging, SYBR Green (1:10,000 dilution) was added to the cells to visualize the nuclei. After incubation with Alexa Fluor 647-labeled MNT, the cells were examined under the LSM-510 Meta NLO multiphoton laser scanning microscope fitted with a Plan-Apochromat ×63/1.4 Oil DIC lens (Carl Zeiss, Oberkochen, Germany). SYBR Green fluorescence was recorded at an excitation wavelength of 488 nm and an emission wavelength bandpass of 500 to 530 nm. Alexa Fluor 647 fluorescence was recorded at an excitation wavelength of 633 nm and an emission wavelength bandpass of 650 to 710 nm. The mean intranuclear fluorescence at 7 and at 48 h of Alexa 647-labeled MNT toward SYBR Green were calculated using multiphoton laser scanning microscope software.
Interactions of the MNT with α/β Importins Evaluated by Thermophoresis
Importins α and β were obtained as previously described (Gilyazova et al., 2006). Importin β was conjugated with Cy3 dye and separated from unbound dye on a PD-10 column (GE Healthcare, Chicago, IL, USA). Importins were diluted in importin buffer (pH 7.4) containing 20 mM HEPES, 110 mM KCl, 5 mM NaHCO3, 5 mM MgCl2, 0.1 mM CaCl2, 1 mM EGTA, and 1 mM DTT. Importin heterodimers were used at equimolar concentrations of 50 nM. MNTN-EGF, MNTc-EGF, and MNTN-affibody were serially diluted in buffer with α/β importins. The initial concentrations were 1 µM for MNTN-EGF and 4 µM for MNTN-affibody and MNTC-EGF. The strengths of the interactions between the MNT and the α/β importins were measured with a MonolithNT.115 instrument (NanoTemper Technologies, Munich, Germany). The binding affinities (Kd) were automatically interpolated from a fitted curve by MonolithNT.115 Instruments software (NanoTemper Technologies, Munich, Germany).
Labeling MNT With 111In Using p-SCN-Bn-NOTA
MNTC-EGF, MNTN-affibody, and MNTN-EGF were labeled in accordance with a previously published protocol (Slastnikova et al., 2017a). MNT were incubated with 10-fold molar excess of the bifunctional p-SCN-Bn-NOTA chelator in conjugation carbonate buffer at pH 8.6 (Hens et al., 2009) for 20 h at room temperature with final concentrations of MNT ≥1.5 mg/ml. The chelator-MNT conjugate was concentrated and separated from excess chelator by five cycles of ultrafiltration using Amicon Ultracel-30K. During this process, the conjugation buffer was gradually replaced with 10 mM 4-(2-hydroxyethyl)-1-piperazineethanesulfonic acid (HEPES), 15 mM NaCl, pH 7.4. All buffers used for chelator conjugation and labeling procedures were passed through Chelex-100 resin (200–400 mesh; Bio-Rad) to minimize adventitious metal ion contamination.
For 111In labeling NOTA-MNTC-EGF, NOTA-MNTN-affibody and NOTA-MNTN-EGF (0.2 mg, 0.04 mg, and 0.04 mg, respectively) in 10 mM HEPES, 15 mM NaCl, pH 7.5, was mixed with 1 M HEPES, pH 7.5, 0.1 M citrate, pH 6.7, 1% SDS, and 0.25 M HCl (Ultrapure Grade, Merck, Darmstadt, Germany); then 111InCl3 in 0.048 M HCl was added. The reaction mixture was incubated at 37°C for 1 h, and then the reaction was stopped by adding 3 μl 0.05 M EDTA, pH 8.0, followed by gentle mixing and incubation for 10 min at 37°C. Finally, the pH was neutralized with 1 M NaOH. The initial specific radioactivity of either FR-targeted 111In-MNT, obtained using this protocol, was 2.7 GBq mg−1. As a control, 111In was treated following the same procedures except that the NOTA-MNT was omitted in the reaction mixture. Radiochemical yields and 111In-MNT integrity were analyzed by Laemmli SDS-PAGE using Mini-Protean TGX Any kD gels. Radioactivity was detected on a Storm 865 phosphor imager (GE Healthcare, Uppsala, Sweden). Images were analyzed by ImageQuant TL v. 5.0 software (Bio-Rad Laboratories, Hercules, CA, USA).
Cytotoxicity Studies
A431 cells were seeded in 24-well plates (2 × 104 cells well−1). After 2 d, the media were refreshed and various dilutions of 111In-NOTA-MNT (0–6.5 MBq ml−l; 0–32 μg ml−l) were added. The 111In (0–20 MBq mv−l) was used as a control. The cells were incubated for 48 h at 37°C under a 5% CO2 atmosphere. Medium containing unbound radioactivity was removed. The cells were washed by Versene solution, trypsinized, harvested, and resuspended in 1 ml fresh medium with 10% (w/v) CFS. For a colony-forming assay, the cells were seeded in 25-cm2 flasks (2,000 cells flask−1) containing DMEM/F12 supplemented with 10% (w/v) CFS. After 8 d, the colonies were stained with crystal violet and counted. Fitting was made in accordance with «one phase decay» algorithm using GraphPad Prism 5 software.
111In-NOTA-MNT Accumulation in the Nuclei
A431 cells were seeded in 12-well plates (5 × 105 cells well−1) containing DMEM supplemented with 10% CFS. After 2 d, the media were refreshed and various dilutions of 111In-NOTA-MNT (0.6 MBq ml−1; 1.9 μg ml−1) were added. The cells were incubated for 2 h at 37°C under a 5% CO2 atmosphere. The plates were cooled and the media containing unbound radioactivity were removed. The cells were washed by Versene solution, trypsinized, and harvested. The wells were washed with ice-cold DMEM containing 10% (w/v) CFS, and the rinsate was added to the cells samples. The cells were then centrifuged in an Eppendorf centrifuge 5415R at 200 × g and 4°C for 7 min, resuspended in 500 μl cold fresh medium with 10% (w/v) CFS, and re-centrifuged. The supernatant was removed, and the cells were resuspended in 300 μl ice-cold hypotonic buffer (25 mM Tris-HCl (pH 7.5), 5 mM KCl, 0.5 mM DTT, 1 mM PMSF, and 0.15 U ml−1 aprotinin) and expanded on ice for 20 min. The mixture was homogenized on ice with a Dounce homogenizer (15 strokes). Nuclei were pelleted by centrifugation at 600 × g and 4°C for 12 min. Pelleted nuclei were washed 5× in 300 μl·wash−1·tube−1 ice-cold isotonic buffer (0.25 M sucrose, 6 mM MgCl2, 10 mM Tris-HCl (pH 7.4) 0.5% (w/v) Triton X-100, 1 mM PMSF, and 0.15 U ml−1 aprotinin) to remove cytoplasmic membranes.
Nuclear purity was confirmed by microscopic evaluation. The nuclei were resuspended in a Versene solution and incubated on ice for 15 to 30 min to reduce clumping. The suspension was centrifuged at 600 × g and 4°C for 12 min, and the nuclear yield was determined using counting chambers.
Sample radioactivity was measured with a RiaGamma counter 1271 (LKB Wallac, Sollentuna, Sweden). The percentages of activity in the nuclei relative to the total intracellular activity were calculated for all 111In-NOTA-MNT.
Nuclear purity was evaluated by western blot using antibodies against α-tubulin (a cytoplasmic marker) and nibrin (NBS-1; a nuclear marker).
Cell Proliferation
MCF-7 cells were seeded in 24-well plates (8 × 103 cells well−1) at day 0 in DMEM supplemented with 0.5% (w/v) CFS. After 1 d, the medium was refreshed with 2 ml DMEM and 0.5% CFS per well. MNTC-EGF, MNTN-affibody, MNTN-EGF, MNT without ligand module (MNTw/l), and human EGF were added to the cells to a final concentration of 100 nM. The media were not changed during the experiment. Cell growth at the indicated time points was estimated using 0.2 ml of MTT. The resultant formazan crystals were solubilized in 2 ml of 96° ethanol:DMSO (1:1) per well. Absorbances and the background were read at 570 and 650 nm, respectively, on a Biotek Synergy 4 microplate reader (Winooski, VT, USA).
Statistics
The data were analyzed using GraphPad Prism 5 software (GraphPad Software Inc., San Diego, CA, USA). Data on the plots represent mean values, with bars indicating the standard error of the mean of repetitive values. The significance of the difference was evaluated using the Mann–Whitney U-test or Tukey multiple comparison test. The differences were significant when P < 0.05.
Results
Here, we produced and characterized MNTN-affibody, the new EGFR-binding MNT. Within the previously created and characterized MNTC-EGF (Gilyazova et al., 2006), we transferred its ligand module from C-terminus to N-terminus and replaced EGF with the Z1907 affibody (Kim et al., 2012). MNTN-EGF was also derived from the MNTC-EGF (Figure 2A). The sequences of these MNT are shown in Supplements 1.
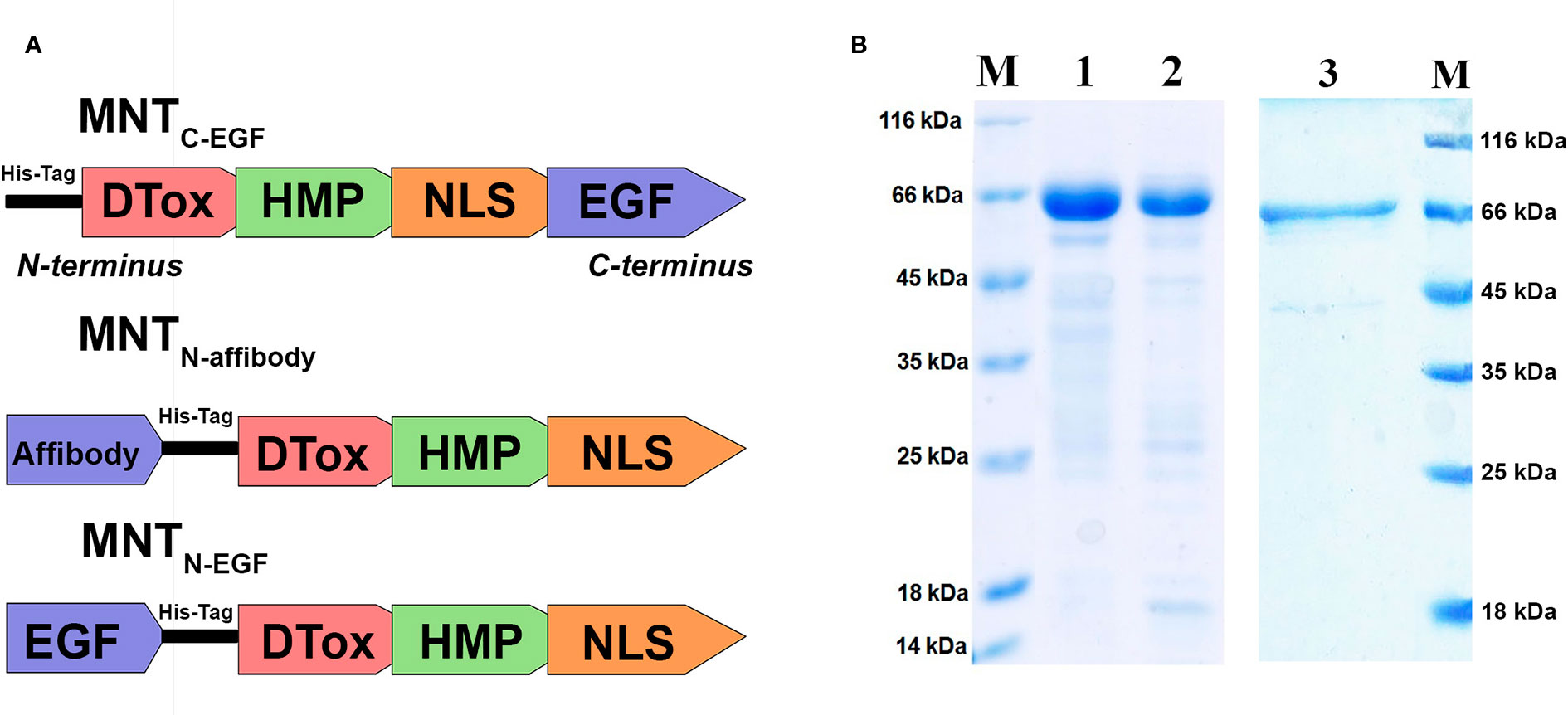
Figure 2 Characteristics of the MNT. (A) A schematic diagram depicting structure of the new MNTN-affibody and MNTN-EGF and previously described MNTC-EGF. (B) SDS-PAGE gels of MNTN-affibody (lane 1), MNTN-EGF (lane 2), MNTC-EGF (lane 3) and unstained protein markers (lane M).
Purity of isolated and purified MNTN-affibody, MNTC-EGF and MNTN-EGF was evaluated by Laemmli SDS PAGE, it was > 85% for all MNT. SDS-PAGE gel of MNTN-affibody and MNTN-EGF was shown in Figure 2B.
The binding of MNT to EGFR was evaluated with an EGFR-expressing human A431 epidermoid carcinoma cell line. The dissociation constants for MNTN-EGF and MNTN-affibody were interpolated from displacement curves (Figure 3) and were 37.5 ± 5.9 nM and 34.7 ± 4.1 nM, respectively. These values are close to those of the prototypical MNTC-EGF (29.3 nM) (Rosenkranz et al., 2008) and indicate the ability of the new MNT to specifically bind to the EGFR, that is necessary for receptor-specific recognition of the target cells.
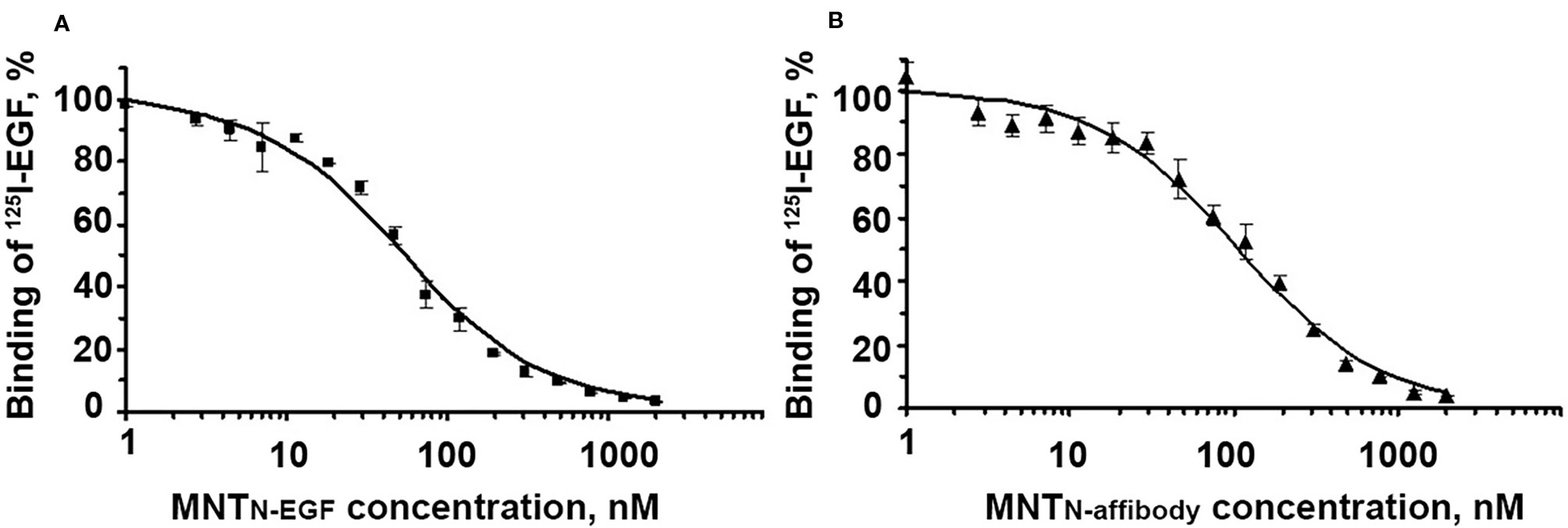
Figure 3 Displacement curves for [125I]iodo-EGF by MNTN-EGF (A) and MNTN-affibody (B) from EGFR on A431 cells. [125I]iodoEGF (1 nM) and MNT at the indicated concentrations were added to the cells and incubated at 4°C for 20 h. Error bars represent standard errors of the mean (SEM; n = 3).
The propensity of MNT to make membrane pores via the endosomolytic module was tested using calcein-loaded liposomes at various pH. Maximum pH-dependent calcein leakage (Figure 4) was observed at pH = 5.5 for all three assessed MNT. This weakly acidic internal pH corresponds to that of the endosome (Canton and Battaglia, 2012). Accordingly, relocation and change of the ligand module do not influence endosomolytic activity of the DTox module, and the new MNT are potentially able to escape from endosomes before fusion of late endosomes with lysosomes and subsequent lysosomal degradation.
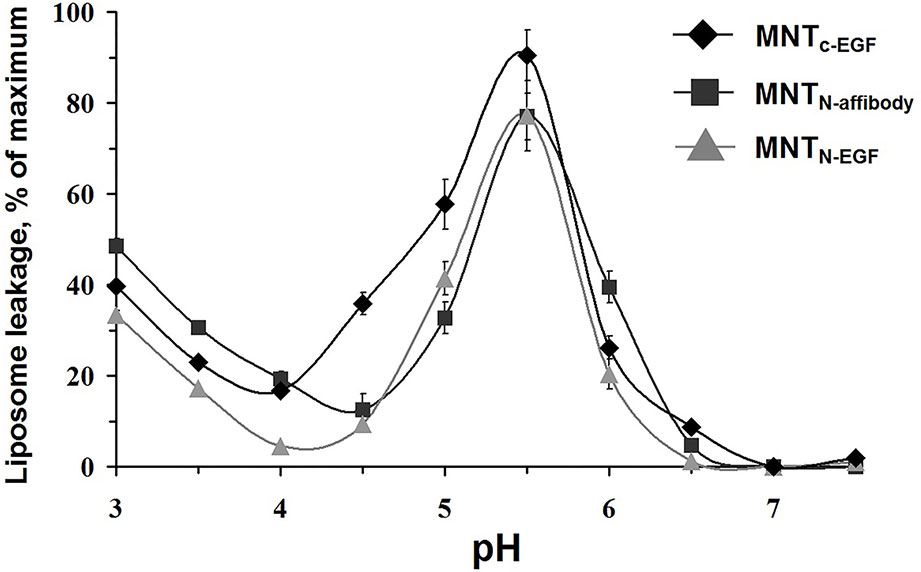
Figure 4 MNTC-EGF, MNTN-affibody, and MNTN-EGF induced leakage from egg yolk phosphatidyl choline liposomes loaded with fluorescent calcein to the fluorescence quenching concentration. The appearance of fluorescence indicates liposome leakage. Error bars are SEM (n = 4).
The ability of the Alexa Fluor 647 labeled MNT to internalize into the target EGFR-expressing cells was shown using the flow cytometry method. Since the cells incubated with MNT were washed and trypsinized, the detected fluorescence signal corresponded to internalized part of the MNT. For the cells incubated with EGFR-binding MNT, fluorescence intensity value corresponding to the maximum of cell amount significantly differs from fluorescence intensity value for non-incubated cells (autofluorescence). The smaller difference in the signal for MNTw/l from autofluorescence indicates that MNTw/l transport into the cell was significantly worse than that with MNTN-affibody, MNTN-EGF, and MNTC-EGF (Figure 5). Addition of 2 μM free EGF to the medium significantly reduced the average fluorescence intensity for EGFR-binding MNT that indicates the importance of the contribution of receptor-mediated transport. The significance of the difference was evaluated using the Mann–Whitney U-test.
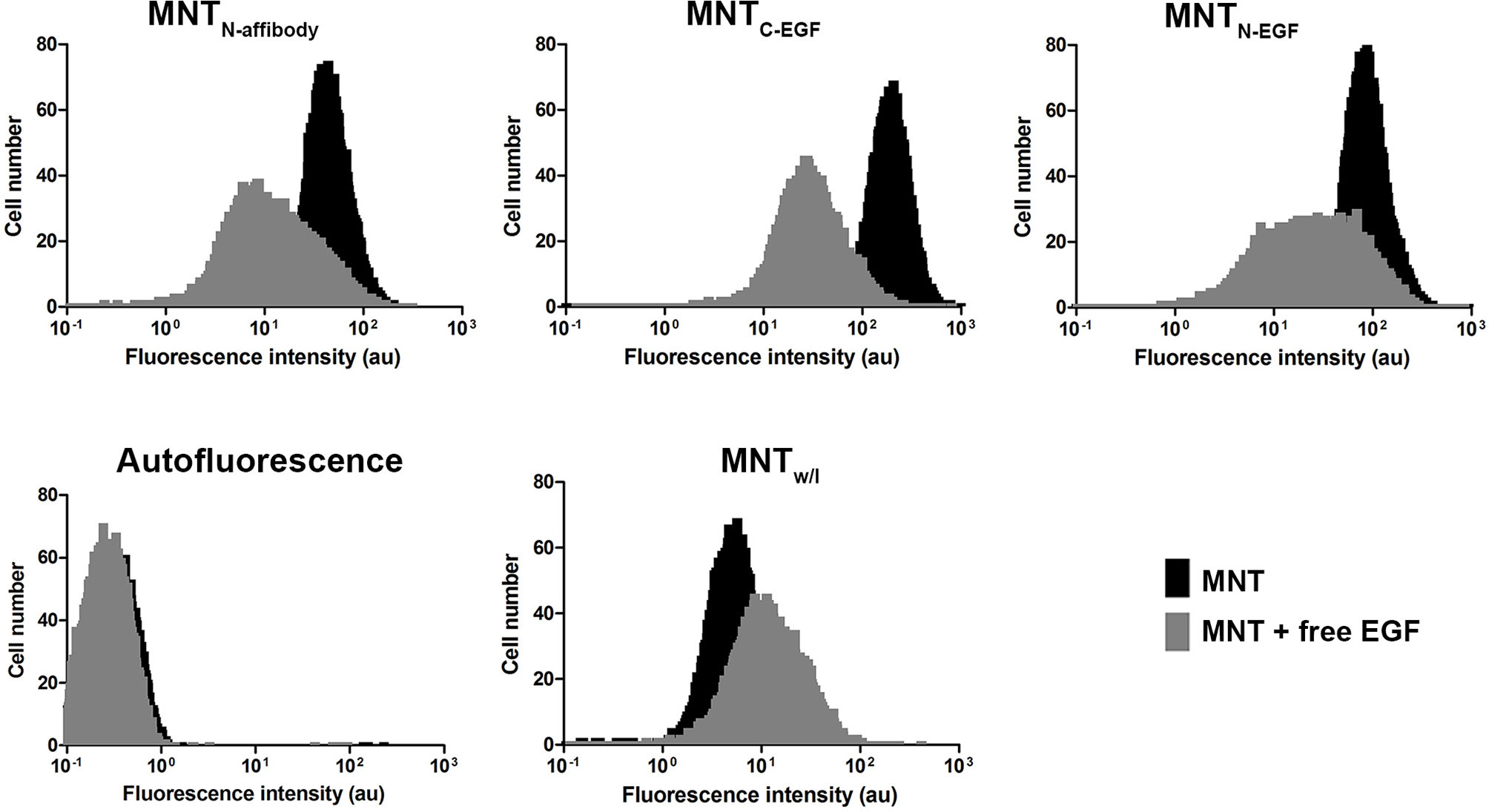
Figure 5 Intracellular accumulation of Alexa 647 labeled MNT measured by flow cytometry. Flow cytometry histograms of A431 cells, cells incubated with MNTN-affibody, MNTN-EGF, MNTC-EGF or with control MNTw/l for 18 h with or without free EGF (2 μM). Untreated cells served as autofluorescence controls. Error bars represent standard errors of mean (n = 4).
The intranuclear localizations of MNT labeled with Alexa Fluor 647 were viewed in A431 cells under confocal laser scanning microscopy. For all types of MNT with EGFR ligand modules, the Alexa Fluor 647 signal was visible within the nuclei from 7–48 h incubation. For MNTw/l, there was no substantial signal in the nuclei (Figures 6A, B). The intranuclear signal intensity increased from 7 h to 48 h for all EGFR-binding MNT (Figure 6C). The data obtained indicate the ability of new MNT to transport into the nuclei of the target cells and accumulate there in process of time.
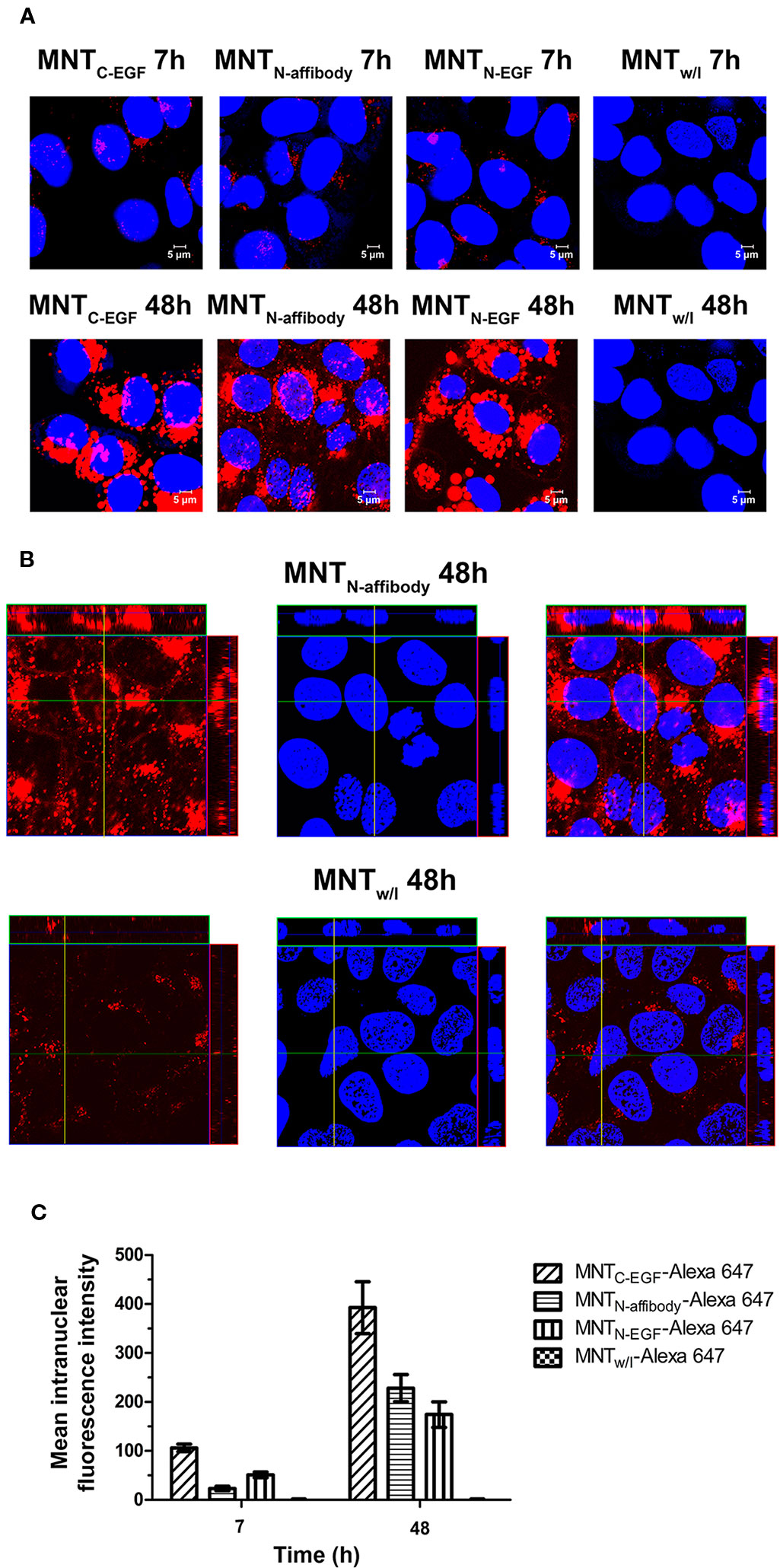
Figure 6 Intranuclear distribution of MNTC-EGF, MNTN-affibody, MNTN-EGF, and MNT without ligand module (MNTw/l), labeled with Alexa Fluor 647, and visualized by confocal laser scanning microscopy inside A431 cells. (A) Confocal laser scanning microscopy images across the nuclei of A431 cells incubated for 7 and 48 h with 50 nM Alexa 647-labeled MNT. Alexa Fluor 647 fluorescence corresponding to MNT is in red pseudo-color. Nuclei were counterstained with SYBR Green (blue pseudo-color). (B) Confocal laser scanning microscopy images across the nuclei of A431 cells incubated for 48 h with 50 nM Alexa 647-labeled MNTN-affibody and with MNTw/l as a control. Alexa Fluor 647 fluorescence corresponding to MNT is in red pseudo-color. Nuclei were counterstained with SYBR Green (blue pseudo-color). (C) Mean intranuclear fluorescence intensity of Alexa 647-labeled MNT following 7 and 48 h incubation. Error bars are SEM (n = 90–134).
The binding affinity of MNT for the importin-α/β carrier protein complex reflects the functional activity of the NLS module responsible for nuclear MNT import. The dissociation constant (Kd) for MNTN-affibody binding to the α/β importin heterodimer is 117 ± 29 nM (Figure 7A). It was automatically calculated from the interaction thermophoresis curve and closely approaches the value for MNTC-EGF (127 ± 15 nM; Figure 7C). In contrast, the value for MNTN-EGF is 15.8 ± 7.6 nM (Figure 7B).
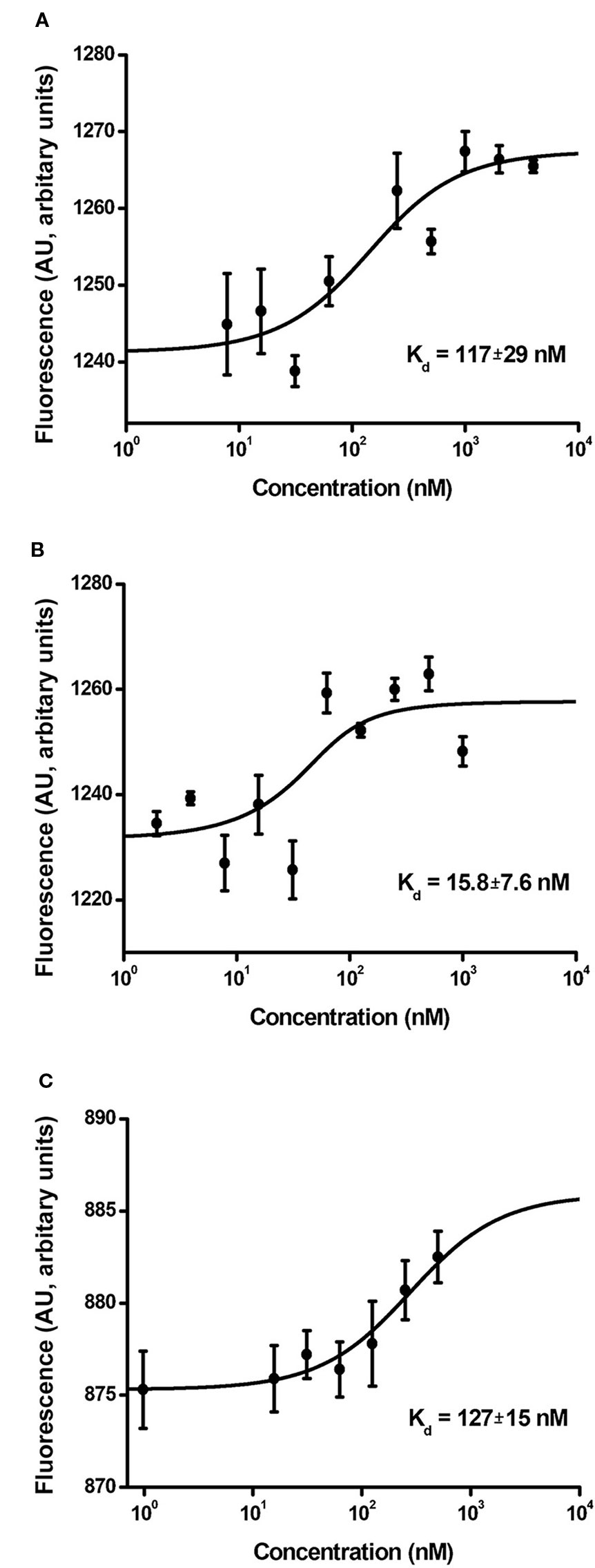
Figure 7 Interaction curves of MNTN-affibody (A), MNTN-EGF (B), and MNTC-EGF (C) with α/β importins. Binding of serial MNT dilutions with α/β importin heterodimer was measured by thermophoresis. Binding affinity (Kd) was automatically interpolated from a fitted curve by MonolithNT.115 Instruments software. Error bars are SEM (n = 3–9).
We demonstrated that the transfer and replacement of the ligand module did not affect to the functional activity of the remaining modules, and new transporters are able to reach the target compartment — the cell nucleus. On this basis, it seemed important to evaluate the cytotoxic effect of Auger electron emitter 111In, transported by new MNT to the nuclei of target cells. We used unbound 111In as a control. Figure 8 shows clonogenic survival plots of A431 cells after 48 h incubation with serial dilutions of 111In-NOTA-MNTC-EGF, 111In-NOTA-MNTN-affibody, 111In-NOTA-MNTN-EGF (0–6.5 MBq ml−1) and free 111In (0–20 MBq ml−1). The cytotoxicity of 111In delivered by any 111In-NOTA-MNT far exceeds that of control 111In. The 111In-NOTA-MNTC-EGF and 111In-NOTA-MNTN-affibody have similar cytotoxicity. The slopes of the curves for 111In-NOTA-MNTC-EGF and 111In-NOTA-MNTN-affibody differ significantly from the slopes of the curves for control 111In and 111In-NOTA-MNTN-EGF according to the Tukey multiple comparison test (P < 0.05). The 111In activity levels of 111In-NOTA-MNTC-EGF and 111In-NOTA-MNTN-affibody that reduced clonogenic efficiency to 37% (A37) of the control were 0.2± 0.05 MBq ml−1 and 0.15± 0.04 MBq ml−1, respectively. In contrast, that was 0.6 MBq ml−1 ± 0.05 for 111In-NOTA-MNTN-EGF A37 and 11.00 ± 2.00 MBq ml−1 for free 111In.
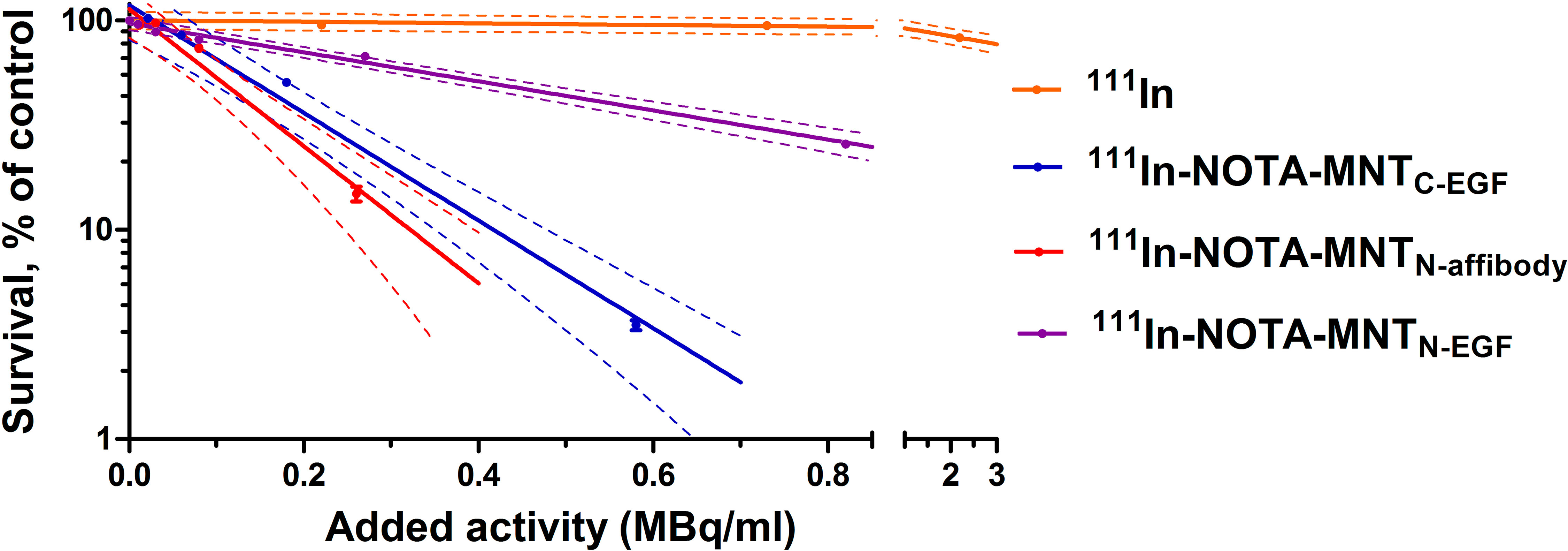
Figure 8 Cytotoxicity of 111In delivered by EGFR-targeted MNT. A431 cells were exposed for 48 h to various activity levels of 111In-NOTA-MNT or control 111In. Cells were seeded for a colony-forming assay at a density of 2,000 cells flask−1. After 8 d, the colonies were stained and counted. Solid lines represent data fitted to a mono-exponential model. Error bars are SEM (n = 3–6). Due to small values, in some cases, error bars merge with the symbols. Dotted lines represent 95% confidence intervals for each MNT and control 111In.
As 111In Auger electrons have extremely short ranges, their cytotoxicity was highest when they were localized in the nucleus (Li et al., 2015; Slastnikova et al., 2017a). Thus, we measured the ability of 111In-NOTA-MNT to accumulate in the nuclei. As shown in Figure 9, 111In was delivered to A431 nuclei by all EGFR-targeted MNT. The purity of the nuclear fractions was validated by western blot (Supplements 2).
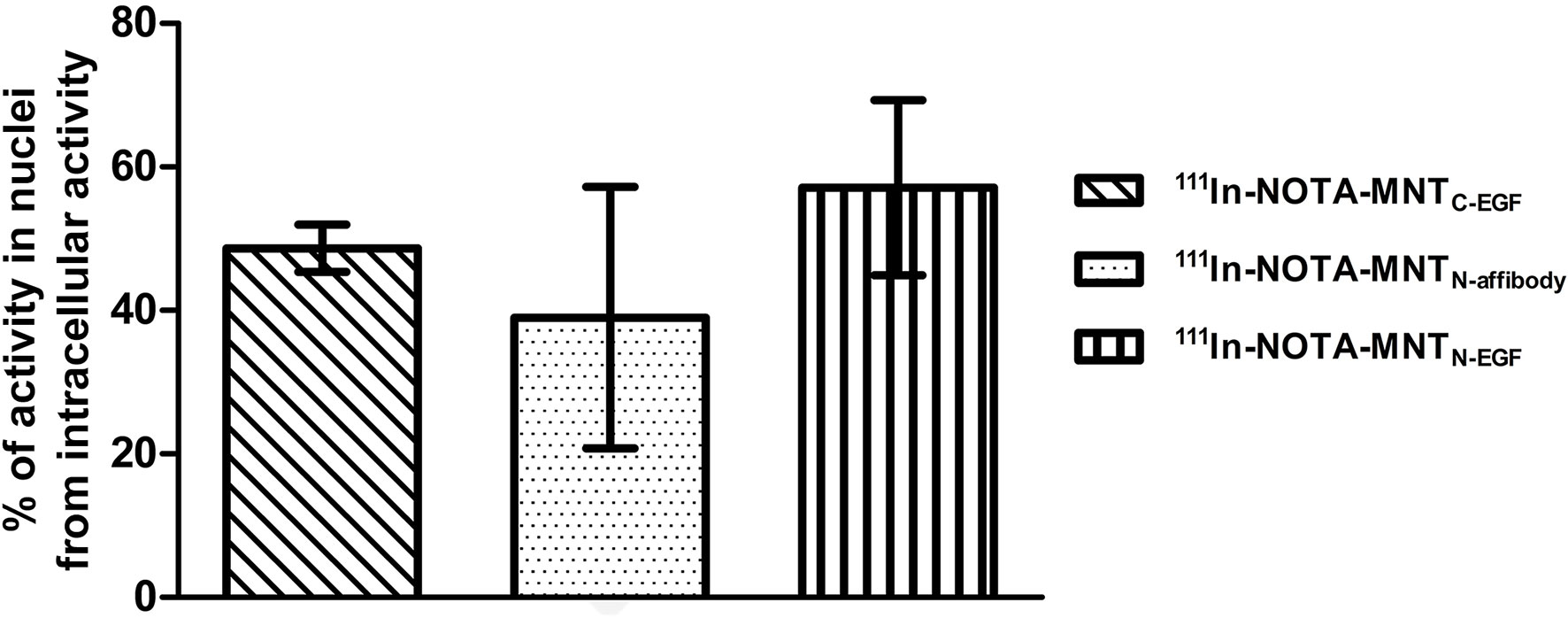
Figure 9 Intranuclear accumulation efficiency of 111In-NOTA-MNT. A431 cells were incubated with 111In-NOTA-MNT (0.6 MBq ml−1; 1.9 μg ml−1) for 2 h. Percentage of intranuclear accumulated activity relative to whole intracellular accumulated activity. Error bars are SEM (n = 3).
The percentages of total intracellular radioactivity in the nuclei slightly differed between the various 111In-NOTA-MNT. For 111In-NOTA-MNTN-affibody, it was 39 ± 18%, for 111In-NOTA-MNTC-EGF it was 49 ± 3%, and for 111In-NOTA-MNTN-EGF it was 57 ± 12% (Figure 9). The significance of the difference was evaluated using the Mann–Whitney U-test. No statistically significant difference between the MNT was found.
We investigated the effects of MNT on cell proliferation. It was previously shown that several cell lines, like A431 and MDA-MB-468 are atypical (Kawamoto et al., 1983; Armstrong et al., 1994; Bromberg et al., 1998). They have a feature that distinguishes them from most other EGFR-expressing cancer cell lines. For these cell lines activation of EGFR leads to inhibition of the tumor cell proliferation, whereas for other types of tumor cells, such as MCF-7, activation of EGFR, on the contrary, leads to the induction of cell growth. We used the cell line, that does not have the feature in order to examine the ability of the MNT to induce cell proliferation relative to the control free EGF. We showed that the growth rate of MCF-7 cancer cell line greatly increased after a few days incubation with EGF and MNTN-EGF compared to control cells. Therefore, these agents possessed pro-oncogenic effect. On the other hand, incubation with MNTN-affibody and MNTC-EGF had no statistically significant impact on MCF-7 proliferation (Figure 10).
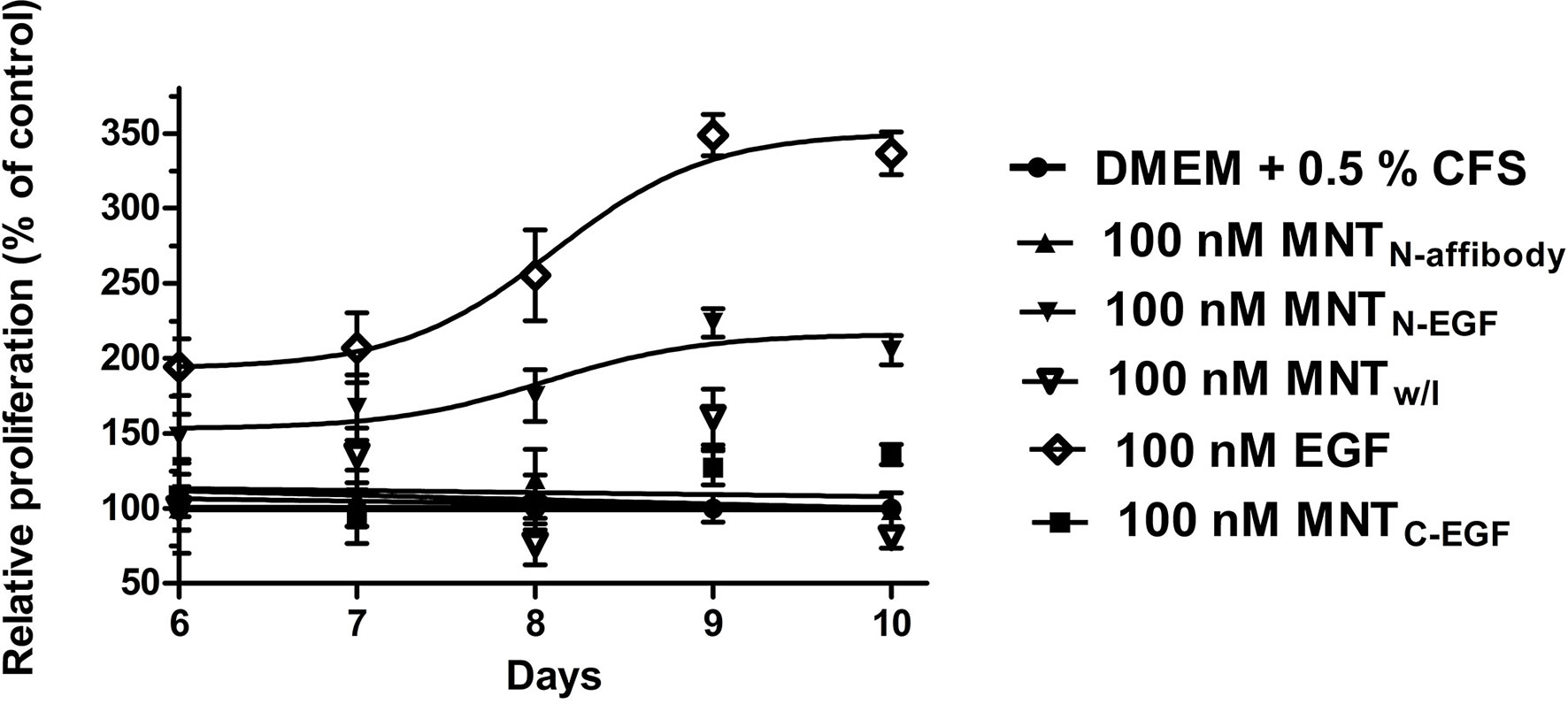
Figure 10 Effects of MNT on cell proliferation. MCF-7 cells were exposed for 5–9 d to 100 nM MNT or free EGF in medium with 0.5% serum. MNT and EGF were added 1 d after seeding. Cell proliferation was analyzed every 24 h by MTT assay starting at day 6. Proliferation values for untreated cells were set as 100% for each day. Other values were calculated as relative cell proliferation vs. corresponding values for untreated cells. Data points are represented as means ± standard deviation (SD) (n = 4).
Discussion
EGFRs are highly convenient for the targeted delivery of various drugs into cancer cells. A number of drug delivery vehicles were produced for this purpose and evaluated in vitro and in vivo [(Chen et al., 2002; Lu et al., 2005; Liu et al., 2010; Song et al., 2016; Slastnikova et al., 2017b; Zahaf et al., 2017; Rosenkranz et al., 2018), etc.]. The main areas of EGFR-targeted drug therapy include: (1) development of drugs based on anti-EGFR antibodies binding to the extracellular EGFR domain, preventing ligand binding, and interrupting signal cascades (Herbst, 2004; Friedman and Stahl, 2009; Scott et al., 2012); (2) tyrosine kinase inhibitors binding to the intracellular EGFR domain and inhibiting the downstream effects of EGFR ligand binding (Herbst, 2004; Dassonville et al., 2007; Gazdar, 2009); and (3) delivery of drugs to cancer cells by fusion constructs containing EGF (Scott et al., 2012). However, several factors reduce EGFR-dependent treatment efficacy.
Most preclinical studies are conducted on xenograft models using immunodeficient mice (Cai et al., 2007; Eiblmaier et al., 2008; Liu et al., 2010). The relative efficacies of drugs targeting EGFR and tested on xenograft models could significantly decrease in the transition to clinical trials (Troiani et al., 2008; Day et al., 2015). Antibodies often have low interspecies cross-reactivity. Poor translation of results from xenograft to clinical trials may be especially severe for monoclonal anti-EGFR antibodies (Weller et al., 1987; Burgess, 2008).
Anti-hEGFR antibodies bind to xenograft cells overexpressing hEGFR. When the drug is administered to humans, however, most of the antibodies bind to liver cells resulting in the decreased proportion of antibodies reaching the tumor cells (Burgess, 2008). Consequently, drug efficacy is compromised, the incidence and severity of side effects dramatically increase, and therapy is adversely affected. Moreover, drug efficacy may be relatively overstated in immunodeficient animals with xenografts (Frese and Tuveson, 2007; Cekanova and Rathore, 2014). Thus, there is a growing risk that newly developed drugs may fail the clinical trial stage (Sharpless and Depinho, 2006; Ocana et al., 2010). Attempts have been made to overcome these limitations such as the creation of the Cetuximab analogue anti-mouse EGFR mAb 7A7 (Garrido et al., 2004). In contrast, efforts have failed in the development of simple models for preclinical studies of drugs exhibiting the same properties as human analogues such as 7A7 (He et al., 2018).
An alternative remedial approach is to develop a drug that binds mouse and human EGFR equally well. It would facilitate the accurate assessment of drug pharmacokinetics (Haeri and Osouli, 2017). The hEGFs have high binding affinities for hEGFR and mEGFR (Groenen et al., 1994; Elleman et al., 2001). Thus, the use of hEGFR as a ligand module would improve and harmonize therapeutic agent biodistribution in preclinical and clinical studies.
However, upon its interaction with EGFR on tumor cells, EGF induces receptor dimerization and autophosphorylation which activate several downstream kinase cascades. This leads to complex of prooncogenic effects such as cancer cell proliferation, migration, survival, DNA-double strand break repair, hypoxia, mediates radioresistance and inhibits apoptosis (Rodemann et al., 2007; Bussink et al., 2008). Hence, we selected affibody (ZEGFR:1907) as a ligand for MNT.
The affibody has shown strong binding affinity to EGFR with good specificity (Qi et al., 2012); it is significantly smaller (~ 7 kDa) (Orlova et al., 2007: Tolmachev et al., 2009), compared to multidomain antibodies (~ 150 kDa), ScFv (~ 28 kDa) and single-domain antibodies - nanobodies (~ 15 kDa) (Slastnikova et al., 2018). It has equal affinity for both mEGFR and hEGFR (Friedman et al., 2008; Qi et al., 2012) and does not induce receptor autophosphorylation and cell proliferation (Beuttler et al., 2009; Nordberg et al., 2010; Ekerljung et al., 2012). In addition, affibody molecules tolerate modifications and conjugations with retained high-target affinity, and the absence of disulfide bridges in the structure make them convenient to be used as a part of complex molecules (Friedman et al., 2008).Therefore, the affibody can be successfully used to deliver cytotoxic agents to the nuclei of cancer cells with EGFR expression.
Although the affibody on its own does not induce receptor autophosphorylation and cell proliferation, it seemed important to verify this for MNTN-affibody. MNT with EGF at the N- and C-termini and free EGF were used as controls. We investigated the effects of MNT on cell proliferation on the non-atypical cell model MCF-7, for which activation of EGFR leads to mitogenic effect. We used A431 cell line for the other experiments, since the results for the previously described MNTC-EGF were obtained on this cell line (Gilyazova et al., 2006; Rosenkranz et al., 2008; Slastnikova et al., 2012a; Koumarianou et al., 2014; Slastnikova et al., 2017b; Rosenkranz et al., 2018), however for A431 activation of EGFR leads to inhibition of the tumor cell proliferation (Kawamoto et al., 1983; Bromberg et al., 1998). We showed that MNTN-affibody did not affect cell growth (Figure 10), that was consistent with the properties of this affibody (Friedman et al., 2008; Beuttler et al., 2009; Ekerljung et al., 2012). MNTC-EGF and MNTN-EGF presented with different effects on MCF-7 proliferation depending on the position of the EGF-ligand module. Similar effects were reported for other EGF-containing chimeric constructs (Kim et al., 2015). МNТN-EGF may interact with EGFR like an epigen ligand whose C-terminal sequence is long compared with that of EGF. The epigen initiates EGFR dimerization which is relatively unstable but induces cell proliferation (Kochupurakkal et al., 2005; Freed et al., 2017). Nevertheless, this comparison may be inaccurate as we did not study the steric mechanisms of MNT interaction with EGFR. Here, we focused on the characteristics of MNT that pertain to the development of targeted drug delivery platforms.
All of the MNT examined here specifically bound at similar Kd to EGFR and were then internalized by receptor-specific mechanism (Figures 3, 5, and 6). The MNT-receptor complex underwent endocytosis and the MNT was enclosed in the endosome. The endosomolytic module was active at pH ~5.5 according to the liposome-based experimental model (Figure 4). This pH corresponds to that previously obtained for MNTC-EGF (Gilyazova et al., 2006).
All MNT specifically bound to the α/β importin heterodimer responsible for nuclear import because of the activity of the NLS module. MNTN-affibody and MNTC-EGF had similar dissociation constants with the α/β importin heterodimer whereas that of MNTN-EGF was comparatively lower (Figure 7). We suggested that this may be due to steric features for these molecules. We also showed that the signal from all EGF-binding MNT molecules is detected inside target cell nuclei (Figures 6 and 9) and it changes over time (from 7 to 48 h incubation), while for MNTw/l it remains equally low (Figure 6C).
In this study, we selected the Auger electron emitter 111In as the cytotoxic agent, which has a local damaging effect. The range of its Auger electrons is several tens of nanometers. Thus, 111In is suitable for nucleus-targeted therapy (Kassis, 2004). When 111In was delivered into the nuclei of target cancer cells via MNT, its cytotoxicity was markedly greater than that of the free 111In used as a control. These data correspond to those previously reported (Slastnikova et al., 2017b; Rosenkranz et al., 2018). However, 111In-NOTA-MNTN-affibody and 111In-NOTA-MNTC-EGF demonstrates higher cytotoxicity than 111In-NOTA-MNTN-EGF (Figure 8). The lowest cytotoxicity of 111In-NOTA-MNTN-EGF may be explained by the oppositely directed effect of this type of MNT on the target cells, because of EGF in this version of MNT apparently may activate EGFR and induce signaling cascades, which leads to cell survival cell cycle progression, inhibition of apoptosis, etc. (Rodemann et al., 2007; Bussink et al., 2008, Sigismund et al., 2018). This is evidenced, in particular, by the stimulation of cell proliferation by this MNT.
Based on the results of the present study, we consider MNTN-affibody as a promising vehicle for anticancer agents for targeted therapy. It successfully delivers 111In inside target cell nuclei and result in cancer cell death.
The obtained data provide the basis for future experiments both in vitro and in vivo. In vitro study of further other possible types of cellular response to interaction with MNT, such as the level of double-strand DNA breaks (DSB) and apoptosis will be valuable. In vivo the effects of the new MNT should be evaluated. Prolonged intratumoral retention of 111In-NOTA-MNTC-EGF with t1/2 = 4.1 ± 0.5 days as well as significant dose-dependent tumor growth delay (up to 90% growth inhibition) after intratumoral administration of 111In-NOTA-MNTC-EGF (Rosenkranz et al., 2018) permits us to suggest the similar in vivo characteristics for this new MNT. Further modifications of the MNT aimed at reduction of its immunogenicity can provide a basis for efficient systemic use of the regarded MNT.
Conclusion
A new MNT with an affibody ligand was designed and characterized. MNTN-affibody specifically binds to EGFR. MNTN-affibody accumulated inside the target cells wherein it was transported into the nuclei. MNTN-affibody delivered the Auger electron emitter 111In into the target cell nuclei. The cytotoxicity of 111In delivered by MNTN-affibody was far greater than that of free 111In. Unlike EGF and MNTN-EGF, however, it does not induce target cell proliferation. Taken together, these results suggest that MNTN-affibody is a promising targeted drug delivery therapy against cancers characterized by EGFR overexpression.
Data Availability Statement
All datasets generated for this study are included in the article/Supplementary Material.
Author Contributions
AS, AU, and AR designed and evaluated the study. AU constructed plasmids of the new MNT by genetic engineering, and TL and TK made the proteins purification. TK, TS, AR, and YK carried out experiments to evaluate the activity of functional modules of the MNT and cell culture experiments. AS, AU, TS, AR, TL, YK, GG, and TK contributed to the discussion and interpretation of the results. TK took the lead in writing the manuscript. All authors agreed to be accountable for all aspects of the work and read and approved the final manuscript.
Funding
The research was supported by RSF (project No. 17-14-01304).
Conflict of Interest
The authors declare that the research was conducted in the absence of any commercial or financial relationships that could be construed as a potential conflict of interest.
Acknowledgments
Experiments were carried out with the use of equipment from Lomonosov Moscow State University Program of Development and the Center of Collective Usage of the Institute of Gene Biology. We thank Denis I. Zrelkin and Ksenia D. Ignatova for their assistance with the experiments.
Supplementary Material
The Supplementary Material for this article can be found online at: https://www.frontiersin.org/articles/10.3389/fphar.2020.00176/full#supplementary-material
Supplement 1 | Amino acid sequences of the MNT. Individual modules are highlighted in different colors. His-Tag is highlighted in red.
Supplement 2 | Purity of isolated nuclei verified by enhanced chemiluminescence (ECL) western blot. (α) Bands from PageRuler™ Prestained Protein Ladder. (β) Cytoplasmic fraction. (γ) Nuclear fraction. NBS-1 antibody recognizes PageRuler™ Prestained band at 95 kDa which represents NBS-1.
References
Armstrong, D. K., Kaufmann, S. H., Ottaviano, Y. L., Furuya, Y., Buckley, J. A., Isaacs, J. T., et al. (1994). Epidermal growth factor-mediated apoptosis of MDA-MB-468 human breast cancer cells. Cancer Res. 54, 5280–5283.
Beuttler, J., Rothdiener, M., Muller, D., Frejd, F. Y., Kontermann, R. E. (2009). Targeting of epidermal growth factor receptor (EGFR)-expressing tumor cells with sterically stabilized affibody liposomes (SAL). Bioconjug. Chem. 20, 1201–1208. doi: 10.1021/bc900061v
Bromberg, J. F., Fan, Z., Brown, C., Mendelsohn, J., Darnell, J. E., Jr. (1998). Epidermal growth factor-induced growth inhibition requires Stat1 activation. Cell Growth Differ. 9, 505–512.
Burgess, A. W. (2008). EGFR family: structure physiology signaling and therapeutic targets. Growth Factors 26, 263–274. doi: 10.1080/08977190802312844
Bussink, J., van der Kogel, A. J., Kaanders, J. H. (2008). Activation of the PI3-K/AKT pathway and implications for radioresistance mechanisms in head and neck cancer. Lancet Oncol. 9 (3), 288–296. doi: 10.1016/S1470-2045(08)70073-1
Cai, W., Chen, K., He, L., Cao, Q., Koong, A., Chen, X. (2007). Quantitative PET of EGFR expression in xenograft-bearing mice using 64Cu-labeled cetuximab, a chimeric anti-EGFR monoclonal antibody. Eur. J. Nucl. Med. Mol. Imaging 34, 850–858. doi: 10.1007/s00259-006-0361-6
Canton, I., Battaglia, G. (2012). Endocytosis at the nanoscale. Chem. Soc Rev. 41, 2718–2739. doi: 10.1039/c2cs15309b
Cekanova, M., Rathore, K. (2014). Animal models and therapeutic molecular targets of cancer: utility and limitations. Drug Des. Devel. Ther. 8, 1911–1921. doi: 10.2147/DDDT.S49584
Chen, P., Mrkobrada, M., Vallis, K. A., Cameron, R., Sandhu, J., Hendler, A., et al. (2002). Comparative antiproliferative effects of (111)In-DTPA-hEGF, chemotherapeutic agents and gamma-radiation on EGFR-positive breast cancer cells. Nucl. Med. Biol. 29, 693–699. doi: 10.1016/S0969-8051(02)00325-6
Chow, N. H., Liu, H. S., Lee, E. I., Chang, C. J., Chan, S. H., Cheng, H. L., et al. (1997). Significance of urinary epidermal growth factor and its receptor expression in human bladder cancer. Anticancer Res. 17, 1293–1296.
Ciardiello, F., Tortora, G. (2008). EGFR antagonists in cancer treatment. N. Engl. J. Med. 358, 1160–1174. doi: 10.1056/NEJMra0707704
Citri, A., Yarden, Y. (2006). EGF-ERBB signalling: towards the systems level. Nat. Rev. Mol. Cell Biol. 7, 505–516. doi: 10.1038/nrm1962
Cornelissen, B., Waller, A., Able, S., Vallis, K. A. (2013). Molecular radiotherapy using cleavable radioimmunoconjugates that target EGFR and gammaH2AX. Mol. Cancer Ther. 12, 2472–2482. doi: 10.1158/1535-7163.MCT-13-0369
Dassonville, O., Bozec, A., Fischel, J. L., Milano, G. (2007). EGFR targeting therapies: monoclonal antibodies versus tyrosine kinase inhibitors. Similarities and differences. Crit. Rev. Oncol. Hematol. 62, 53–61. doi: 10.1016/j.critrevonc.2006.12.008
Day, C. P., Merlino, G., Van, D. T. (2015). Preclinical mouse cancer models: a maze of opportunities and challenges. Cell 163, 39–53. doi: 10.1016/j.cell.2015.08.068
De Angelis Campos, A. C., Rodrigues, M. A., de, A. C., de Goes, A. M., Nathanson, M. H., Gomes, D. A. (2011). Epidermal growth factor receptors destined for the nucleus are internalized via a clathrin-dependent pathway. Biochem. Biophys. Res. Commun. 412, 341–346. doi: 10.1016/j.bbrc.2011.07.100
Eiblmaier, M., Meyer, L. A., Watson, M. A., Fracasso, P. M., Pike, L. J., Anderson, C. J. (2008). Correlating EGFR expression with receptor-binding properties and internalization of 64Cu-DOTA-cetuximab in 5 cervical cancer cell lines. J. Nucl. Med. 49, 1472–1479. doi: 10.2967/jnumed.108.052316
Ekerljung, L., Wallberg, H., Sohrabian, A., Andersson, K., Friedman, M., Frejd, F. Y., et al. (2012). Generation and evaluation of bispecific affibody molecules for simultaneous targeting of EGFR and HER2. Bioconjug. Chem. 23, 1802–1811. doi: 10.1021/bc3000645
Elleman, T. C., Domagala, T., McKern, N. M., Nerrie, M., Lonnqvist, B., Adams, T. E., et al. (2001). Identification of a determinant of epidermal growth factor receptor ligand-binding specificity using a truncated, high-affinity form of the ectodomain. Biochemistry 40, 8930–8939. doi: 10.1021/bi010037b
Freed, D. M., Bessman, N. J., Kiyatkin, A., Salazar-Cavazos, E., Byrne, P. O., Moore, J. O., et al. (2017). EGFR ligands differentially stabilize receptor dimers to specify signaling kinetics. Cell 171, 683–695. doi: 10.1016/j.cell.2017.09.017
Frese, K. K., Tuveson, D. A. (2007). Maximizing mouse cancer models. Nat. Rev. Cancer 7, 645–658. doi: 10.1038/nrc2192
Friedman, M., Stahl, S. (2009). Engineered affinity proteins for tumor-targeting applications. Biotechnol. Appl. Biochem. 53, 1–29. doi: 10.1042/BA20080287
Friedman, M., Orlova, A., Johansson, E., Eriksson, T. L., Hoiden-Guthenberg, I., Tolmachev, V., et al. (2008). Directed evolution to low nanomolar affinity of a tumor-targeting epidermal growth factor receptor-binding affibody molecule. J. Mol. Biol. 376, 1388–1402. doi: 10.1016/j.jmb.2007.12.060
Garrido, G., Sanchez, B., Rodriguez, H. M., Lorenzano, P., Alonso, D., Fernandez, L. E. (2004). 7A7 MAb: a new tool for the pre-clinical evaluation of EGFR-based therapies. Hybrid. Hybridomics. 23, 168–175. doi: 10.1089/1536859041224280
Gazdar, A. F. (2009). Activating and resistance mutations of EGFR in non-small-cell lung cancer: role in clinical response to EGFR tyrosine kinase inhibitors. Oncogene 28 Suppl 1, S24–S31. doi: 10.1038/onc.2009.198
Gilyazova, D. G., Rosenkranz, A. A., Gulak, P. V., Lunin, V. G., Sergienko, O. V., Khramtsov, Y. V., et al. (2006). Targeting cancer cells by novel engineered modular transporters. Cancer Res. 66, 10534–10540. doi: 10.1158/0008-5472.CAN-06-2393
Goldfarb, D. S., Corbett, A. H., Mason, D. A., Harreman, M. T., Adam, S. A. (2004). Importin alpha: a multipurpose nuclear-transport receptor. Trends Cell Biol. 14, 505–514. doi: 10.1016/j.tcb.2004.07.016
Groenen, L. C., Nice, E. C., Burgess, A. W. (1994). Structure-function relationships for the EGF/TGF-alpha family of mitogens. Growth Factors 11, 235–257. doi: 10.3109/08977199409010997
Haeri, A., Osouli, M. (2017). EGFR targeted nanocarriers for cancer diagnosis and therapy. Trends Pept. Protein Sci. 1, 41–55. doi: 10.22037/tpps.v1i2.13611
Hashmi, A. A., Hussain, Z. F., Irfan, M., Khan, E. Y., Faridi, N., Naqvi, H., et al. (2018). Prognostic significance of epidermal growth factor receptor (EGFR) over expression in urothelial carcinoma of urinary bladder. BMC. Urol. 18, 59. doi: 10.1186/s12894-018-0373-0
He, X., Cruz, J. L., Joseph, S., Pett, N., Chew, H. Y., Tuong, Z. K., et al. (2018). Characterization of 7A7, an anti-mouse EGFR monoclonal antibody proposed to be the mouse equivalent of cetuximab. Oncotarget 9, 12250–12260. doi: 10.18632/oncotarget.24242
Hens, M., Vaidyanathan, G., Welsh, P., Zalutsky, M. R. (2009). Labeling internalizing anti-epidermal growth factor receptor variant IIImonoclonal antibody with177Lu: in vitro comparison of acyclic andmacrocyclic ligands. Nucl. Med. Biol. 36 (2), 117–128. doi: 10.1016/j.nucmedbio.2008.11.001
Herbst, R. S. (2004). Review of epidermal growth factor receptor biology. Int. J. Radiat. Oncol. Biol. Phys. 59, 21–26. doi: 10.1016/j.ijrobp.2003.11.041
Hicks, D. G., Short, S. M., Prescott, N. L., Tarr, S. M., Coleman, K. A., Yoder, B. J., et al. (2006). Breast cancers with brain metastases are more likely to be estrogen receptor negative, express the basal cytokeratin CK5/6, and overexpress HER2 or EGFR. Am. J. Surg. Pathol. 30, 1097–1104. doi: 10.1097/01.pas.0000213306.05811.b9
Hirsch, F. R., Varella-Garcia, M., Cappuzzo, F. (2009). Predictive value of EGFR and HER2 overexpression in advanced non-small-cell lung cancer. Oncogene 28 Suppl 1, S32–S37. doi: 10.1038/onc.2009.199
Kassis, A. I., Adelstein, S. J. (2005). Radiobiologic principles in radionuclide therapy. J. Nucl. Med. 46 Suppl 1, 4S–12S.
Kassis, A. I. (2004). The amazing world of auger electrons. Int. J. Radiat. Biol. 80, 789–803. doi: 10.1080/09553000400017663
Kawamoto, T., Sato, J. D., Le, A., Polikoff, J., Sato, G. H., Mendelsohn, J. (1983). Growth stimulation of A431 cells by epidermal growth factor: identification of high-affinity receptors for epidermal growth factor by an anti-receptor monoclonal antibody. Proc. Natl. Acad. Sci. U. S. A 80, 1337–1341. doi: 10.1073/pnas.80.5.1337
Khramtsov, Y. V., Rokitskaya, T. I., Rosenkranz, A. A., Trusov, G. A., Gnuchev, N. V., Antonenko, Y. N., et al. (2008). Modular drug transporters with diphtheria toxin translocation domain form edged holes in lipid membranes. J. Control Release 128, 241–247. doi: 10.1016/j.jconrel.2008.03.011
Kim, D., Yan, Y., Valencia, C. A., Liu, R. (2012). Heptameric targeting ligands against EGFR and HER2 with high stability and avidity. PloS One 7, e43077. doi: 10.1371/journal.pone.0043077
Kim, D., Kim, S. K., Valencia, C. A., Liu, R. (2013). Tribody: robust self-assembled trimeric targeting ligands with high stability and significantly improved target-binding strength. Biochemistry 52, 7283–7294. doi: 10.1021/bi400716w
Kim, D. G., Kim, E. Y., Kim, Y. R., Kong, I. S. (2015). Construction of chimeric human epidermal growth factor containing short collagen-binding domain moieties for use as a wound tissue healing agent. J. Microbiol. Biotechnol. 25, 119–126. doi: 10.4014/jmb.1405.05073
Kochupurakkal, B. S., Harari, D., Di-Segni, A., Maik-Rachline, G., Lyass, L., Gur, G., et al. (2005). Epigen, the last ligand of ErbB receptors, reveals intricate relationships between affinity and mitogenicity. J. Biol. Chem. 280, 8503–8512. doi: 10.1074/jbc.M413919200
Koumarianou, E., Slastnikova, T. A., Pruszynski, M., Rosenkranz, A. A., Vaidyanathan, G., Sobolev, A. S., et al. (2014). Radiolabeling and in vitro evaluation of (67)Ga-NOTA-modular nanotransporter–a potential Auger electron emitting EGFR-targeted radiotherapeutic. Nucl. Med. Biol. 41, 441–449. doi: 10.1016/j.nucmedbio.2014.03.026
Lemoine, N. R., Hughes, C. M., Barton, C. M., Poulsom, R., Jeffery, R. E., Kloppel, G., et al. (1992). The epidermal growth factor receptor in human pancreatic cancer. J. Pathol. 166, 7–12. doi: 10.1002/path.1711660103
Li, H., Hasegawa, S., Kamada, T., Saga, T. (2015). Effective auger electron radioimmunotherapy using modified anti-HER2 antibody with nuclear localizing signal. Cancer Res. 75, 1814. doi: 10.1158/1538-7445.AM2015-1814
Liu, P., Li, Z., Zhu, M., Sun, Y., Li, Y., Wang, H., et al. (2010). Preparation of EGFR monoclonal antibody conjugated nanoparticles and targeting to hepatocellular carcinoma. J. Mater. Sci. Mater. Med. 21, 551–556. doi: 10.1007/s10856-009-3925-8
Liu, X., Zeng, L., Zhao, Z., Xie, Y., Wang, S., Zhang, J., et al. (2018). Construction, expression, and characterization of rSEA-EGF and in vitro evaluation of its antitumor activity against nasopharyngeal cancer. Technol. Cancer Res. Treat. 17, 1–11. doi: 10.1177/1533033818762910
Lu, J. X., Peng, Y., Meng, Z. F., Jin, L. Q., Lu, Y. S., Guan, M. X. (2005). Rational design of an EGF-IL18 fusion protein: implication for developing tumor therapeutics. Biochem. Biophys. Res. Commun. 334, 157–161. doi: 10.1016/j.bbrc.2005.06.076
Mendelsohn, J., Baselga, J. (2000). The EGF receptor family as targets for cancer therapy. Oncogene 19, 6550–6565. doi: 10.1038/sj.onc.1204082
Nicholson, R. I., Gee, J. M., Harper, M. E. (2001). EGFR and cancer prognosis. Eur. J. Cancer 37 Suppl 4, S9–15. doi: 10.1016/s0959-8049(01)00231-3
Nordberg, E., Ekerljung, L., Sahlberg, S. H., Carlsson, J., Lennartsson, J., Glimelius, B. (2010). Effects of an EGFR-binding affibody molecule on intracellular signaling pathways. Int. J. Oncol. 36, 967–972. doi: 10.3892/ijo_00000576
Ocana, A., Pandiella, A., Siu, L. L., Tannock, I. F. (2010). Preclinical development of molecular-targeted agents for cancer. Nat. Rev. Clin. Oncol. 8, 200–209. doi: 10.1038/nrclinonc.2010.194
Orlova, A., Feldwisch, J., Abrahmsén, L., Tolmachev, V. (2007). Affibody molecules for molecular imaging and therapy for cancer. Cancer Biother Radiopharm. 22 (5), 573–584. doi: 10.1089/cbr.2006.004-U
Pan, L., Liu, J., Shi, J. (2018). Cancer cell nucleus-targeting nanocomposites for advanced tumor therapeutics. Chem. Soc Rev. 47, 6930–6946. doi: 10.1039/c8cs00081f
Panosa, C., Fonge, H., Ferrer-Batalle, M., Menendez, J. A., Massaguer, A., De, L. R., et al. (2015). A comparison of non-biologically active truncated EGF (EGFt) and full-length hEGF for delivery of Auger electron-emitting 111In to EGFR-positive breast cancer cells and tumor xenografts in athymic mice. Nucl. Med. Biol. 42, 931–938. doi: 10.1016/j.nucmedbio.2015.08.003
Peraldo-Neia, C., Migliardi, G., Mello-Grand, M., Montemurro, F., Segir, R., Pignochino, Y., et al. (2011). Epidermal Growth Factor Receptor (EGFR) mutation analysis, gene expression profiling and EGFR protein expression in primary prostate cancer. BMC. Cancer 11, 31. doi: 10.1186/1471-2407-11-31
Qi, S., Miao, Z., Liu, H., Xu, Y., Feng, Y., Cheng, Z. (2012). Evaluation of four affibody-based near-infrared fluorescent probes for optical imaging of epidermal growth factor receptor positive tumors. Bioconjug. Chem. 23 (6), 1149–1156. doi: 10.1021/bc200596a
Reilly, R. M., Kiarash, R., Cameron, R. G., Porlier, N., Sandhu, J., Hill, R. P., et al. (2000). 111In-labeled EGF is selectively radiotoxic to human breast cancer cells overexpressing EGFR. J. Nucl. Med. 41, 429–438.
Rodemann, H. P., Dittmann, K., Toulany, M. (2007). Radiation-induced EGFR-signaling and control of DNA-damage repair. Int. J. Radiat. Biol. 83 (11-12), 781–791. doi: 10.1080/09553000701769970
Roovers, R. C., Laeremans, T., Huang, L., De, T. S., Verkleij, A. J., Revets, H., et al. (2007). Efficient inhibition of EGFR signaling and of tumor growth by antagonistic anti-EFGR Nanobodies. Cancer Immunol. Immunother. 56, 303–317. doi: 10.1007/s00262-006-0180-4
Rosenblum, D., Joshi, N., Tao, W., Karp, J. M., Peer, D. (2018). Progress and challenges towards targeted delivery of cancer therapeutics. Nat. Commun. 9, 1410. doi: 10.1038/s41467-018-03705-y
Rosenkranz, A. A., Vaidyanathan, G., Pozzi, O. R., Lunin, V. G., Zalutsky, M. R., Sobolev, A. S. (2008). Engineered modular recombinant transporters: application of new platform for targeted radiotherapeutic agents to alpha-particle emitting 211 At. Int. J. Radiat. Oncol. Biol. Phys. 72, 193–200. doi: 10.1016/j.ijrobp.2008.05.055
Rosenkranz, A. A., Slastnikova, T. A., Karmakova, T. A., Vorontsova, M. S., Morozova, N. B., Petriev, V. M., et al. (2018). Antitumor activity of auger electron emitter (111)In delivered by modular nanotransporter for treatment of bladder cancer with EGFR overexpression. Front. Pharmacol. 9, 1331. doi: 10.3389/fphar.2018.01331
Schlessinger, J. (2002). Ligand-induced, receptor-mediated dimerization and activation of EGF receptor. Cell 110, 669–672. doi: 10.1016/s0092-8674(02)00966-2
Scott, A. M., Wolchok, J. D., Old, L. J. (2012). Antibody therapy of cancer. Nat. Rev. Cancer 12, 278–287. doi: 10.1038/nrc3236
Seshacharyulu, P., Ponnusamy, M. P., Haridas, D., Jain, M., Ganti, A. K., Batra, S. K. (2012). Targeting the EGFR signaling pathway in cancer therapy. Expert. Opin. Ther. Targets. 16, 15–31. doi: 10.1517/14728222.2011.648617
Sharpless, N. E., Depinho, R. A. (2006). The mighty mouse: genetically engineered mouse models in cancer drug development. Nat. Rev. Drug Discovery 5, 741–754. doi: 10.1038/nrd2110
Sigismund, S., Avanzato, D., Lanzetti, L. (2018). Emerging functions of the EGFR in cancer. Mol. Oncol. 12, 3–20. doi: 10.1002/1878-0261.12155
Slastnikova, T. A., Koumarianou, E., Rosenkranz, A. A., Vaidyanathan, G., Lupanova, T. N., Sobolev, A. S., et al. (2012a). Modular nanotransporters: a versatile approach for enhancing nuclear delivery and cytotoxicity of Auger electron-emitting 125I. EJNMMI. Res. 2, 59. doi: 10.1186/2191-219X-2-59
Slastnikova, T. A., Rosenkranz, A. A., Gulak, P. V., Schiffelers, R. M., Lupanova, T. N., Khramtsov, Y. V., et al. (2012b). Modular nanotransporters: a multipurpose in vivo working platform for targeted drug delivery. Int. J. Nanomedicine. 7, 467–482. doi: 10.2147/IJN.S28249
Slastnikova, T. A., Rosenkranz, A. A., Khramtsov, Y. V., Karyagina, T. S., Ovechko, S. A., Sobolev, A. S. (2017a). Development and evaluation of a new modular nanotransporter for drug delivery into nuclei of pathological cells expressing folate receptors. Drug Des. Devel. Ther. 11, 1315–1334. doi: 10.2147/DDDT.S127270
Slastnikova, T. A., Rosenkranz, A. A., Morozova, N. B., Vorontsova, M. S., Petriev, V. M., Lupanova, T. N., et al. (2017b). Preparation, cytotoxicity, and in vivo antitumor efficacy of (111)In-labeled modular nanotransporters. Int. J. Nanomedicine. 12, 395–410. doi: 10.2147/IJN.S125359
Slastnikova, T. A., Ulasov, A. V., Rosenkranz, A. A., Sobolev, A. S. (2018). Targeted intracellular delivery of antibodies: the state of the art. Front. Pharmacol. 9, 1208. doi: 10.3389/fphar.2018.01208
Sobolev, A. S., Aliev, R. A., Kalmykov, S. N. (2016). Radionuclides emitting short-range particles and modular nanotransporters for their delivery to target cancer cells. Russian Chem. Rev. 85, 1011. doi: 10.1070/RCR4601
Sobolev, A. S. (2008). Modular transporters for subcellular cell-specific targeting of anti-tumor drugs. Bioessays 30, 278–287. doi: 10.1002/bies.20715
Sobolev, A. S. (2018). Modular nanotransporters for nuclear-targeted delivery of auger electron emitters. Front. Pharmacol. 9, 952. doi: 10.3389/fphar.2018.00952
Song, L., Falzone, N., Vallis, K. A. (2016). EGF-coated gold nanoparticles provide an efficient nano-scale delivery system for the molecular radiotherapy of EGFR-positive cancer. Int. J. Radiat. Biol. 92, 716–723. doi: 10.3109/09553002.2016.1145360
Sorkin, A., Goh, L. K. (2009). Endocytosis and intracellular trafficking of ErbBs. Exp. Cell Res. 315, 683–696. doi: 10.1016/j.yexcr.2008.07.029
Stahl, S., Graslund, T., Eriksson, K. A., Frejd, F. Y., Nygren, P. A., Lofblom, J. (2017). Affibody molecules in biotechnological and medical applications. Trends Biotechnol. 35, 691–712. doi: 10.1016/j.tibtech.2017.04.007
Tolmachev, V., Friedman, M., Sandstrom, M., Eriksson, T. L. J., Rosik, D., Hodik, . M., et al. (2009). Affibody molecules for epidermal growth factor receptor targeting in vivo: aspects of dimerization and labeling chemistry. J. Nucl. Med. 50 (2), 274–283. doi: 10.2967/jnumed.108.055525
Troiani, T., Schettino, C., Martinelli, E., Morgillo, F., Tortora, G., Ciardiello, F. (2008). The use of xenograft models for the selection of cancer treatments with the EGFR as an example. Crit. Rev. Oncol. Hematol. 65, 200–211. doi: 10.1016/j.critrevonc.2007.10.003
Ueda, S., Ogata, S., Tsuda, H., Kawarabayashi, N., Kimura, M., Sugiura, Y., et al. (2004). The correlation between cytoplasmic overexpression of epidermal growth factor receptor and tumor aggressiveness: poor prognosis in patients with pancreatic ductal adenocarcinoma. Pancreas 29, e1–e8. doi: 10.1097/00006676-200407000-00061
Wang, X., Reyes, M. E., Zhang, D., Funakoshi, Y., Trape, A. P., Gong, Y., et al. (2017). EGFR signaling promotes inflammation and cancer stem-like activity in inflammatory breast cancer. Oncotarget 8, 67904–67917. doi: 10.18632/oncotarget.18958
Wang, Z. (2017). ErbB receptors and cancer. Methods Mol. Biol. 1652, 3–35. doi: 10.1007/978-1-4939-7219-7_1
Weller, A., Meek, J., Adamson, E. D. (1987). Preparation and properties of monoclonal and polyclonal antibodies to mouse epidermal growth factor (EGF) receptors: evidence for cryptic EGF receptors in embryonal carcinoma cells. Development 100, 351–363.
Xu, M. J., Johnson, D. E., Grandis, J. R. (2017). EGFR-targeted therapies in the post-genomic era. Cancer Metastasis Rev. 36, 463–473. doi: 10.1007/s10555-017-9687-8
Keywords: affibody, cancer, epidermal growth factor receptors, modular nanotransporters, nuclear targeting, targeted drug delivery
Citation: Karyagina TS, Ulasov AV, Slastnikova TA, Rosenkranz AA, Lupanova TN, Khramtsov YV, Georgiev GP and Sobolev AS (2020) Targeted Delivery of 111In Into the Nuclei of EGFR Overexpressing Cells via Modular Nanotransporters With Anti-EGFR Affibody. Front. Pharmacol. 11:176. doi: 10.3389/fphar.2020.00176
Received: 21 October 2019; Accepted: 07 February 2020;
Published: 04 March 2020.
Edited by:
Herve Boutin, University of Manchester, United KingdomReviewed by:
Duncan Forster, University of Manchester, United KingdomCharles Truillet, Commissariat à l’Energie Atomique et aux Energies Alternatives (CEA), France
Rafal Zielinski, MD Anderson Cancer Center, United States
Copyright © 2020 Karyagina, Ulasov, Slastnikova, Rosenkranz, Lupanova, Khramtsov, Georgiev and Sobolev. This is an open-access article distributed under the terms of the Creative Commons Attribution License (CC BY). The use, distribution or reproduction in other forums is permitted, provided the original author(s) and the copyright owner(s) are credited and that the original publication in this journal is cited, in accordance with accepted academic practice. No use, distribution or reproduction is permitted which does not comply with these terms.
*Correspondence: Alexey V. Ulasov, al.ulasov@gmail.com