- 1Department of Physiological Sciences, Piracicaba Dental School, University of Campinas, Piracicaba, Brazil
- 2Department of Biochemistry and Tissue Biology, Biology Institute, University of Campinas, Campinas, Brazil
- 3Department of Oral Biology, University of Florida College of Dentistry, Gainesville, FL, United States
- 4Faculty of Pharmaceutical Sciences, University of Campinas, Campinas, Brazil
Objective: The aim of the present study was to encapsulate vancomycin in different liposomal formulations and compare the in vitro antimicrobial activity against Staphylococcus aureus biofilms.
Methods: Large unilamellar vesicles of conventional (LUV VAN), fusogenic (LUVfuso VAN), and cationic (LUVcat VAN) liposomes encapsulating VAN were characterized in terms of size, polydispersity index, zeta potential, morphology, encapsulation efficiency (%EE) and in vitro release kinetics. The formulations were tested for their Minimum Inhibitory Concentration (MIC) and inhibitory activity on biofilm formation and viability, using methicillin-susceptible S. aureus ATCC 29213 and methicillin-resistant S. aureus ATCC 43300 strains.
Key Findings: LUV VAN showed better %EE (32.5%) and sustained release than LUVfuso VAN, LUVcat VAN, and free VAN. The formulations were stable over 180 days at 4°C, except for LUV VAN, which was stable up to 120 days. The MIC values for liposomal formulations and free VAN ranged from 0.78 to 1.56 µg/ml against both tested strains, with no difference in the inhibition of biofilm formation as compared to free VAN. However, when treating mature biofilm, encapsulated LUVfuso VAN increased the antimicrobial efficacy as compared to the other liposomal formulations and to free VAN, demonstrating a better ability to penetrate the biofilm.
Conclusion: Vancomycin encapsulated in fusogenic liposomes demonstrated enhanced antimicrobial activity against mature S. aureus biofilms.
Introduction
Staphylococcus aureus (S. aureus) is a Gram-positive microorganism responsible for the majority of nosocomial and community-acquired infections. Notably, S. aureus infections remain a global public health issue highly costly for the healthcare system, with increasing morbidity and mortality rates worldwide (Chakraborty et al., 2012; Honary et al., 2013; Elkhodairy et al., 2014; Holland et al., 2014). Today, over 90% of S. aureus strains are found to be resistant to methicillin (methicillin resistant S. aureus—MRSA), penicillin, aminoglycosides, macrolides, lincosamides, and other beta-lactams (Chakraborty et al., 2012; Muppidi et al., 2012; Sande et al., 2012; Elkhodairy et al., 2014; Shi et al., 2014).
In this scenario of microbial resistance, vancomycin (VAN) is considered a first-choice antibiotic for the treatment of methicillin-resistant S. aureus (MRSA) infections (Pumerantz et al., 2011; Honary et al., 2013; Holland et al., 2014; Men et al., 2016; Gudiol et al., 2017). While VAN remains a first-choice antibiotic for the treatment of MRSA infections, its therapeutic efficacy is limited due to its high molecular weight (1,449.2 g mol−1) and hydrophilicity restricting the drug interaction with bacterial cells and hindering its penetration into biofilms (Howden et al., 2010; Nicolosi et al., 2010; Butler et al., 2014; Moghadas-Sharif et al., 2015). In addition to that, VAN systemic side effects are another limiting factor, which include severe watery diarrhea, kidney failure (Pumerantz et al., 2011; Rose et al., 2012; Honary et al., 2013), ototoxicity, neutropenia, fever, anaphylaxis, thrombocytopenia, and phlebitis (McAuley, 2012).
Bacterial biofilms are characterized by the aggregation of specific bacterial species adhered to a substrate, forming highly organized microbial communities (Khameneh et al., 2014; McCarthy et al., 2015). Biofilm-forming bacteria display a differentiated phenotype compared to planktonic cells and have the ability to produce an extracellular polymeric matrix composed mainly of polysaccharides (Khameneh et al., 2014; Dong et al., 2015; McCarthy et al., 2015). This scaffold provides an extremely robust defense mechanism, which hinders antibiotic penetration into the biofilm structure, substantially reducing the susceptibility of bacterial cells to exogenous agents (Dong et al., 2015; McCarthy et al., 2015; Moghadas-Sharif et al., 2015).
The shortcomings of VAN traditional treatment along with the increased microbial resistance rates, and difficulty to treat biofilms have encouraged the development of drug-carrier systems such as VAN-loaded liposomal formulations (Kadry et al., 2004; Drulis-Kawa et al., 2009; Nicolosi et al., 2010; Lankalapalli et al., 2015). It has been shown that the liposomal sustained release of VAN (i) enhances antibacterial efficacy due to higher interaction of the antibiotic molecule with bacterial cells (Kim and Jones, 2004); (ii) improves pharmacokinetics (Ma et al., 2011); (iii) reduces toxicity (Sande et al., 2012); and (iv) increases the antimicrobial spectrum of action against Gram-negative bacteria (Nicolosi et al., 2010). Furthermore, liposomes can facilitate antibiotic penetration into bacterial cells and, therefore, increase drug concentration in the biofilm inner layers (Moghadas-Sharif et al., 2015). Despite these reports, only a few studies have evaluated the effects of liposomal formulations on the inhibition of biofilm development and viability, particularly Staphylococcus biofilms (Ma et al., 2011; Moghadas-Sharif et al., 2015).
The liposome composition can be specifically modulated in terms of morphology to favor the adsorption onto, or fusion with, the microbial cell membrane. Likewise, vesicle surfaces can be changed based on the characteristics of the infectious agent (Nicolosi et al., 2010). Among some types of liposomes with the ability of interacting with bacterial biofilm cells are fusogenic and cationic liposomes (Kim et al., 1999; Nicolosi et al., 2010). Fusogenic liposomes are vesicles that may fuse with biological membranes, thereby increasing drug contact and delivery into cells. They consist of lipids, such as dioleoyl-phosphatidylethanolamine (DOPE) and cholesterol hemisuccinate (CHEMS), which provide increased fluidity to the lipid bilayer and may destabilize biological membranes (Nicolosi et al., 2010; Aoki et al., 2015; Nicolosi et al., 2015). Because of their composition, fusogenic liposomes are normally in the liquid crystalline phase and, under specific chemical conditions, e.g., acidic milieu or in the presence of cations (Forier et al., 2014) they can lose the bilayer arrangement and fuse. Cationic liposomes are composed of lipids with a positive residual charge, such as stearylamine (SA), dimethyldioctadecylammonium bromide (DDBA), dimethylaminoethane carbamoyl cholesterol (DC-chol), and dioleoyltrimethylammoniumpropane (DOTAP), which provides specific electrostatic interaction with bacterial cell wall and biofilms, both negatively charged (Kim et al., 1999; Torchilin, 2012; Zhang et al., 2014; Moghadas-Sharif et al., 2015).
While fusogenic and cationic liposomes have proven advantages in interacting with bacterial cells and formed biofilms, there is still no consensus on the ideal composition of liposome-encapsulated VAN formulations able to prolong drug release and increase its antimicrobial efficacy. Thus, in the present study we developed and characterized large unilamellar vesicles of conventional (LUV VAN), fusogenic (LUVfuso VAN), and cationic (LUVcat VAN) liposomes encapsulating vancomycin hydrochloride. The in vitro antimicrobial activity of these formulations on S. aureus biofilms was further determined and compared.
Materials and Methods
Materials
VAN hydrochloride was kindly provided by Teuto/Pfizer Laboratory (Anápolis, GO, Brazil). HEPES buffer, cholesterol (Chol), alpha-tocopherol (α-T) and egg phosphatidylcholine (EPC) were purchased from Sigma-Aldrich (St. Louis, MO, USA) and chloroform was obtained from Merck (Darmstadt, Germany). Dioleoylphosphatidylethanolamine (DOPE), dipalmitoylphosphatidylcholine (DPPC), cholesterol hemisuccinate (CHEMS) and stearylamine (Sa) were purchased from Avanti Polar Lipids Inc. (Alabaster, AL USA).
Preparation of Liposomal Formulations
Conventional (LUV VAN), fusogenic (LUVfuso VAN) and cationic (LUVcat VAN) liposomal formulations were prepared containing 10 mg/ml VAN. Plain, VAN-free formulations were used as negative controls in the experiments (LUV, LUVfuso, and LUVcat). All liposomal formulations were prepared with 10 mM lipid concentration, with the following composition: LUV–EPC : Chol:α-T (4:3:0.07, mol%) (Cereda et al., 2006); LUVfuso–DOPE : DPPC : CHEMS:α-T (4:2:4:0.07, mol%) (Nicolosi et al., 2010); LUVcat–EPC : Sa:Chol:α-T (1:0.5:0.5:0.07, mol%) (Kadry et al., 2004), respectively. All formulations were prepared in HEPES buffer (80 mM) containing 150 mM NaCl (pH 7.4).
Preparation of liposomal formulations was carried out as previously described, with modifications (Cereda et al., 2006). Briefly, the lipids were dissolved in chloroform, evaporated under nitrogen flow to obtain the lipid film, and vacuumed for 2 h to ensure complete solvent removal. Subsequently, the film was hydrated in HEPES buffer with or without VAN hydrochloride solution. Then the suspension was vortexed for 5 min to form large multilamellar vesicles (MLVs). The suspensions were extruded under nitrogen flow at high pressure (Extruder Emulsiflex C5, Avestin, Inc., Ottawa, ON, Canada) 12 times using polycarbonate membrane initially with 400 nm pores, and then, with 100 nm pores, to obtain small unilamellar vesicles. The extrusion of LUVfuso formulation was performed in water bath at 50°C, which is higher than the DPPC phase transition temperature (Nicolosi et al., 2010).
Characterization of Liposomal Formulations
Morphological Analysis
The morphology of the different types of VAN-containing liposomes or plain liposomes was analyzed by Transmission Electron Microscopy (TEM) (906 LEO-ZEISS, Jena, Germany) at 80 kV. Briefly, one drop of each formulation was added to a copper-coated grid with 200 mesh for 10 s (Electron Microscopy Sciences, Fort Washington, PA). Subsequently, uranyl acetate aqueous solution (2%, w/v) was added and kept at room temperature for 4 h.
Determination of Size, Polydispersity Index, Zeta Potential and Stability of Liposomes
Liposomal vesicles were diluted in deionized water for evaluation of the average size (nm), polydispersity index (PDI), and zeta potential (mV) by the dynamic light scattering using Nano ZS equipment (Malvern Instruments Ltd., Worcestershire, UK, England) at 25°C in triplicate. To evaluate stability of liposomes, these parameters were monitored during 180 days at 4°C.
Vancomycin Encapsulation Efficiency
The encapsulation efficiency (%EE) of VAN into liposomal formulations was determined by the ultrafiltration–centrifugation method (Da Silva et al., 2016) (35). Unencapsulated VAN was separated from encapsulated VAN by ultracentrifugation (Optima L-90K Ultracentrifuge, Beckman Coulter Inc. Pasadena, California, USA) at 120,000g for 2 h at 10°C. Aliquots from the supernatant were diluted in deionized water and analyzed spectrophotometrically at 280 nm (Varian Cary® 50 UV-vis, Varian Inc., Palo Alto, CA, USA). The %EE was calculated based on the concentration of unencapsulated VAN over the concentration of VAN in solution, using the formula as follows:
Evaluation of Vancomycin Release In Vitro
The drug release assay was performed using the Franz vertical diffusion cell (Franz, 1975), which consists of two compartments—one donor and one receptor—separated by a regenerated cellulose membrane (Spectra/Por® 2) with molecular exclusion limit of 12,000–14,000 Da (Spectrum Laboratories Inc., Rancho Dominguez, CA, USA) (de Araujo et al., 2008; Da Silva et al., 2016). An aliquot of 1 ml of the liposomal suspensions was added to the donor compartment, while the receptor compartment was filled with 4 ml of buffer (pH 7.4), maintained at 37°C and 400 rpm agitation. Aliquots of the receptor medium were removed throughout the 10-hour experiment and analyzed by spectrophotometry at 280 nm (Varian Cary® 50 UV-Vis, Varian Inc., Palo Alto, CA, USA). The collected volume was replaced with fresh medium due to the dilution effect.
Evaluation of Antimicrobial Activity
Microorganisms and Growth Conditions
Methicillin-susceptible S. aureus (MSSA) ATCC 29213 and methicillin-resistant S. aureus (MRSA) ATCC 43300 strains were used in this study. Microorganisms were maintained in Tryptone Soy Broth (TSB) (Difco®, New Jersey, USA) with 20% glycerol at −80°C, and cultivated onto Tryptone Soy Agar (Difco®, New Jersey, USA) plates at 37°C. Mueller Hinton Broth (MHB) (Difco®, New Jersey, USA) was used in the MIC assay, while Brain Heart Infusion (Difco®, New Jersey, USA) plus 1% D-glucose (Sigma-Aldrich, St. Louis, MO, USA) was used in the biofilm killing assays.
Experimental Groups
Test formulations consisted of VAN-containing and VAN-free LUV, LUVfuso and LUVcat. The experimental groups were set as follows: A—culture medium, test formulation and inoculum; B—culture medium, control formulation and inoculum; C—culture medium, free VAN solution and inoculum; D—culture medium, HEPES buffer (vehicle) and inoculum; E—culture medium and test formulation; F—culture medium and inoculum; and G—culture medium alone.
Minimum Inhibitory Concentration (MIC)
The MIC was determined by the microdilution method, as previously described by the CLSI (2012), using Mueller-Hinton Broth. The formulations were added to 96-well microplates and serially diluted to obtain concentrations ranging from 0.025 to 50 µg/ml. From 18–24 h agar cultures, three to five colonies of S. aureus were dispersed into saline solution and bacterial inoculum was adjusted using a spectrophotometer (λ 625nm, OD 0.1, 1 to 2 × 108 CFU/ml). Then, the inoculum was diluted and transferred to the wells at a final concentration of 5 × 104 CFU/ml. The plates were incubated at 37°C for 24 h and the absorbance was read at 620 nm (Biochrom ASYS UVM 340, Biochrom, Cambridge, England). The MIC was defined as the lowest concentration of the formulation which inhibited visible bacterial growth. The experiments were performed in six replicates.
Inhibitory Effects on Biofilm Formation
The liposomal formulations were tested for their ability to inhibit biofilm formation and adherence according to the protocol proposed by Graziano et al. (2015) and Wu et al. (2013). First, BHI medium supplemented with 1% glucose and S. aureus cell suspension (final concentration of 5 × 104 CFU/ml) were added to 96-well U-bottom microplates. Right after, the test formulations were added to the wells and plates were then incubated for 24 h, at 37°C. After this period, the supernatant was removed, and the wells were washed three times with distilled water to remove loosely bound or non-adhered cells. Biofilms were stained with 0.4% crystal violet, solubilized with 98% ethanol and read in a microplate reader at 575 nm (Asys UVM 340, Biochrom, Cambridge, England).
Inhibitory Effects on Biofilm Viability
The liposomal formulations were next tested for their inhibitory effects on biofilm viability, as previously described (Graziano et al., 2015) (39). Cellulose acetate membranes (25 mm diameter, 0.2 µM pores) (Sartorius Stedim GmbH, Guxhagen, Hessen, Germany) were used as substrates for S. aureus biofilm formation. The membranes were placed in 6-well plates containing BHI medium supplemented with 1% glucose and bacterial suspension (approximately 1 × 106 CFU/ml in each well). The plates were incubated at 37°C for 24 h. Then the membranes were transferred to new plates containing fresh BHI plus 1% glucose, and biofilms were treated with the formulations at 1 × MIC, 10 × MIC, and 50 × MIC for 24 h. Treated biofilm-coated membranes were gently washed (three times) through immersion into 5 ml of 0.9% NaCl. Then, the membranes were transferred to other tubes containing freshly 5 ml of 0.9% NaCl and then sonicated with six pulses of 9.9 s, 5 s time-interval, 5% amplitude (VibraCell 400W, Sonics & Materials Inc., Newtown, CT, USA) and vortexed at 3,800 rpm for 30 s. Ten-microliter aliquots were collected from each tube, serially diluted, and plated for CFUs onto TSA. The plates were incubated at 37°C for 24 h.
Statistical Analysis
The data distribution was analyzed using the Shapiro–Wilks test. The variables size, PDI, zeta potential, and %EE, were compared using unpaired t-test. Stability parameters for liposomes and the biofilm data were compared using analysis of variance (ANOVA) followed by Tukey’s post-hoc test. The drug release profile was analyzed by two-way ANOVA followed by Tukey’s post-hoc test. Statistical analyses were performed on Origin 8.0 (Microcal TM Software Inc., EUA) and GraphPad Prism 6.0 (San Diego, California, USA). The data were presented as mean and standard deviation (SD), with a 5% significance level. All data are representative of three independent experiments.
Results
Characterization of Liposomal Formulations
TEM images confirmed that the liposomal vesicles had spherical shape with clear edges. Vesicle size in all formulations ranged between 100 and 200 nm. Micrographs of all liposomal formulations are presented in Figure 1. As exemplified in Figures 1C, D, some fusogenic vesicles were found to merge with each other, which typically characterizes this type of liposome.
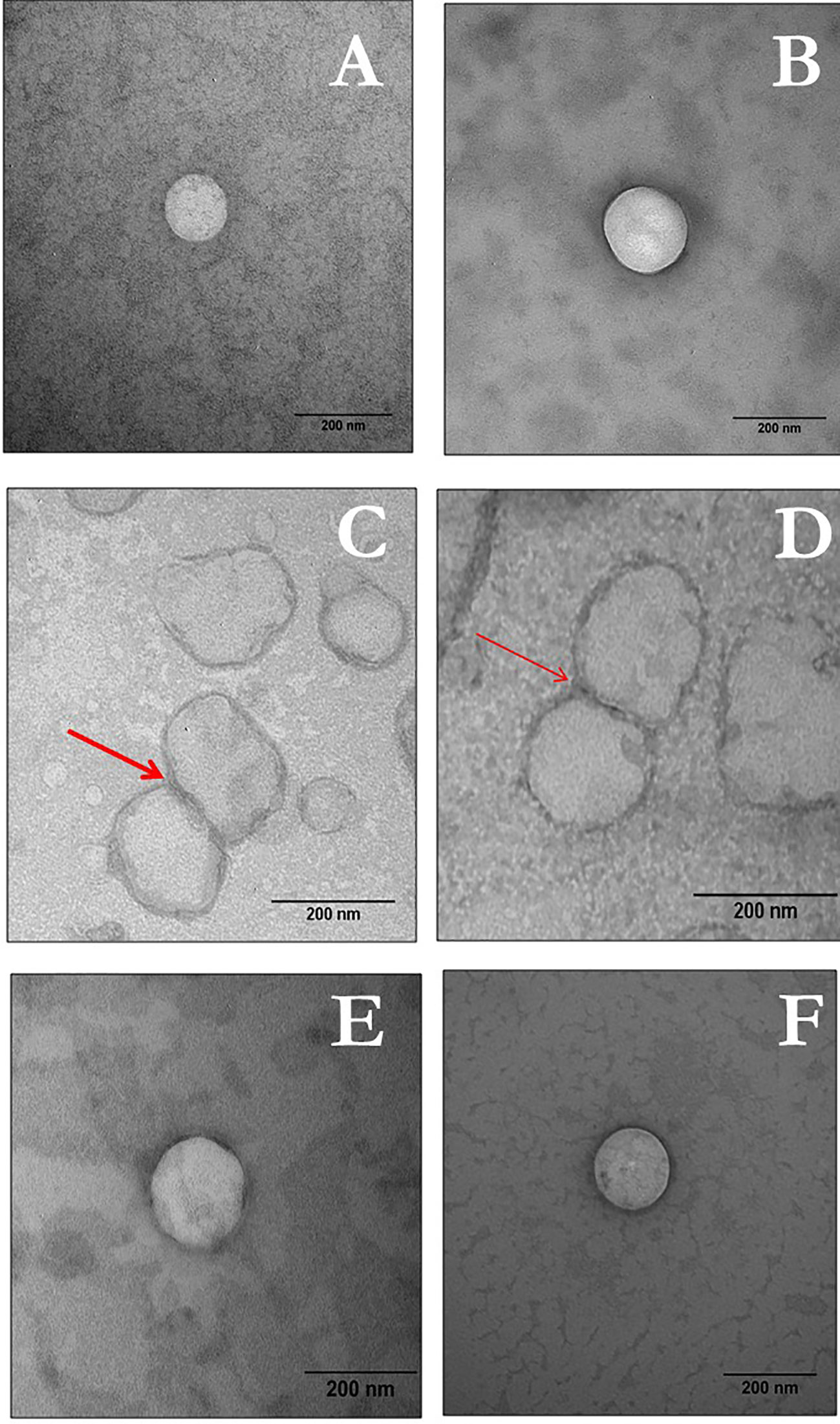
Figure 1 TEM images of LUV VAN, LUVfusoVAN, and LUVca VAN.The left panel represents plain vesicles and the right panel indicated VAN-containing LUVs as follows: (A, B) LUV; (C, D) LUVfuso; and (E, F) LUVcat. Bars indicate 200 nm, with 100,000× magnification).
The means and standard deviations of size, PDI, zeta potential and %EE of the liposomal formulations are given in Table 1. Comparisons were made between plain and VAN-containing liposomes. No differences in size were found while PDI values increased for VAN-containing formulations in comparison to plain controls (p < 0.05). Moreover, as expected, the zeta potential values confirmed the presence of negative charges on LUV and LUVfuso liposomes and positive charges on LUVcat. The encapsulation decreased the negative zeta potentials of LUVfuso VAN liposomes (p < 0.05), while it increased the positive zeta potential in LUVcat VAN, as compared to their respective controls (p < 0.05). Higher %EE values were observed for LUV VAN, followed by LUVfuso VAN and LUVcat VAN.
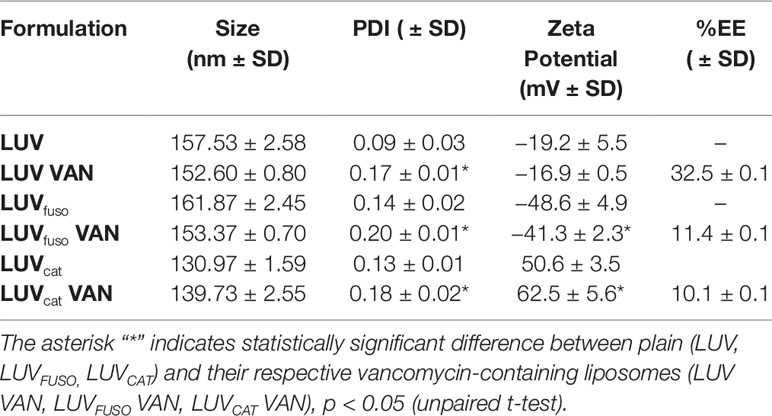
Table 1 Mean ( ± SD) of the size (nm), polydispersity index (PDI), zeta potential (mV) and encapsulation efficiency (%EE) of the liposomal formulations developed in this study.
The stability of the formulations was determined from measurements of size, PDI and zeta potential (Figures 2A–C) during storage at 4°C. In general, LUVcat VAN, LUVfuso VAN and LUV VAN kept their size during the 180-day experimental period (p > 0.05). LUV VAN showed an increase in size after 7 days of storage (p < 0.05) but kept their size in the other time points. LUVcat VAN also changed in size after 60 and 90 days (p < 0.05). However, these alterations were no greater than 10% of the initial size. No significant changes in PDI and Zeta values were found during the experiment (p > 0.05). It is also worth noting that, although with an increasingly trend, PDI values were found to be under 0.2, as required for a monodisperse size distribution.
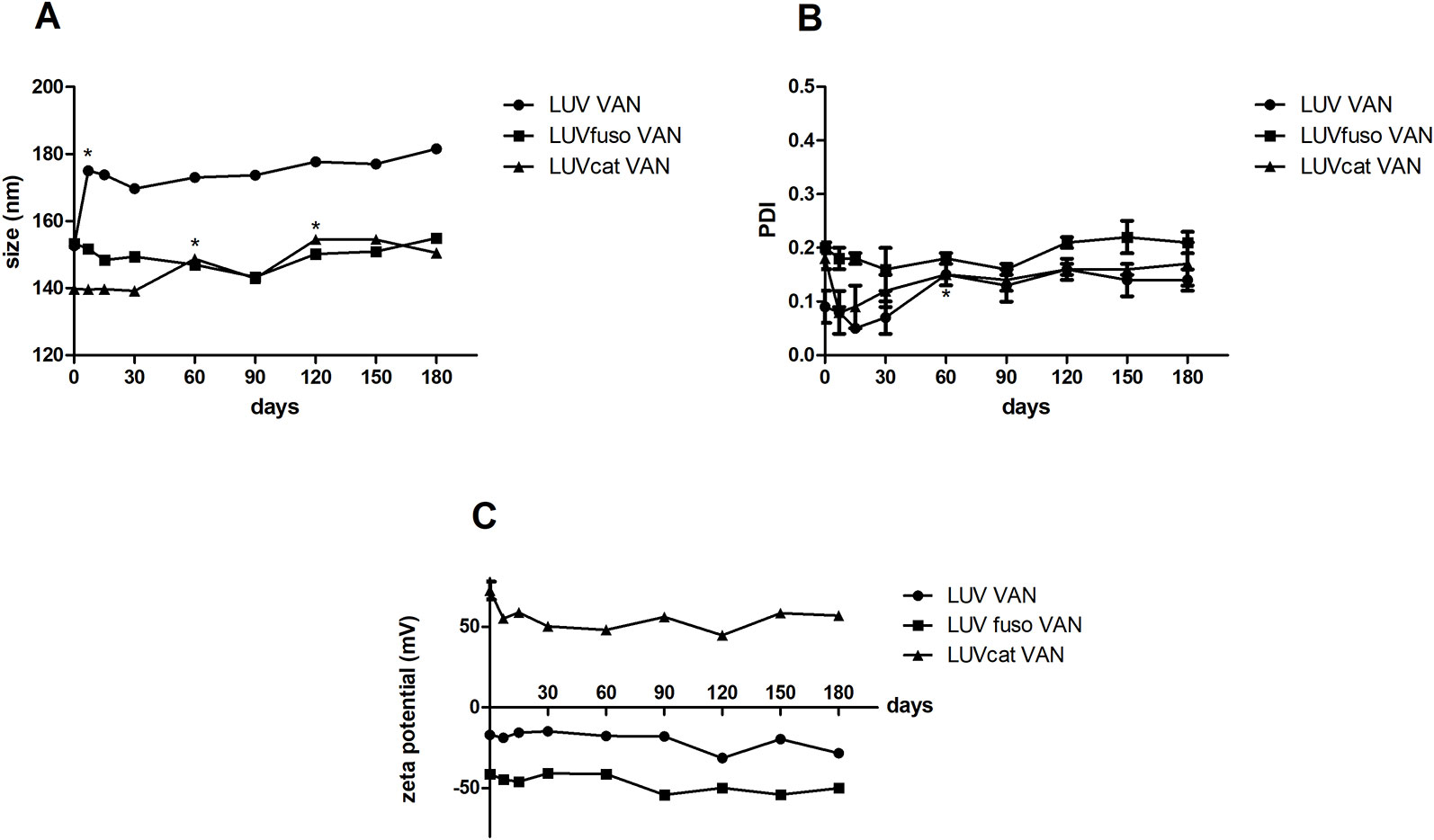
Figure 2 Size (A), PDI (B) and Zeta potential (C) for VAN liposomal formulations analyses during 180 days. The asterisk “*” indicates statistically significant difference between the drug treatment and its respective untreated control at p < 0.05 (One-way ANOVA, followed by Tukey’s post-hoc test).
The release kinetics of VAN in solution and encapsulated in the liposomal formulations was determined in vitro. As seen in Figure 3, encapsulated VAN formulations showed prolonged releases overtime as compared to free VAN (p < 0.05).
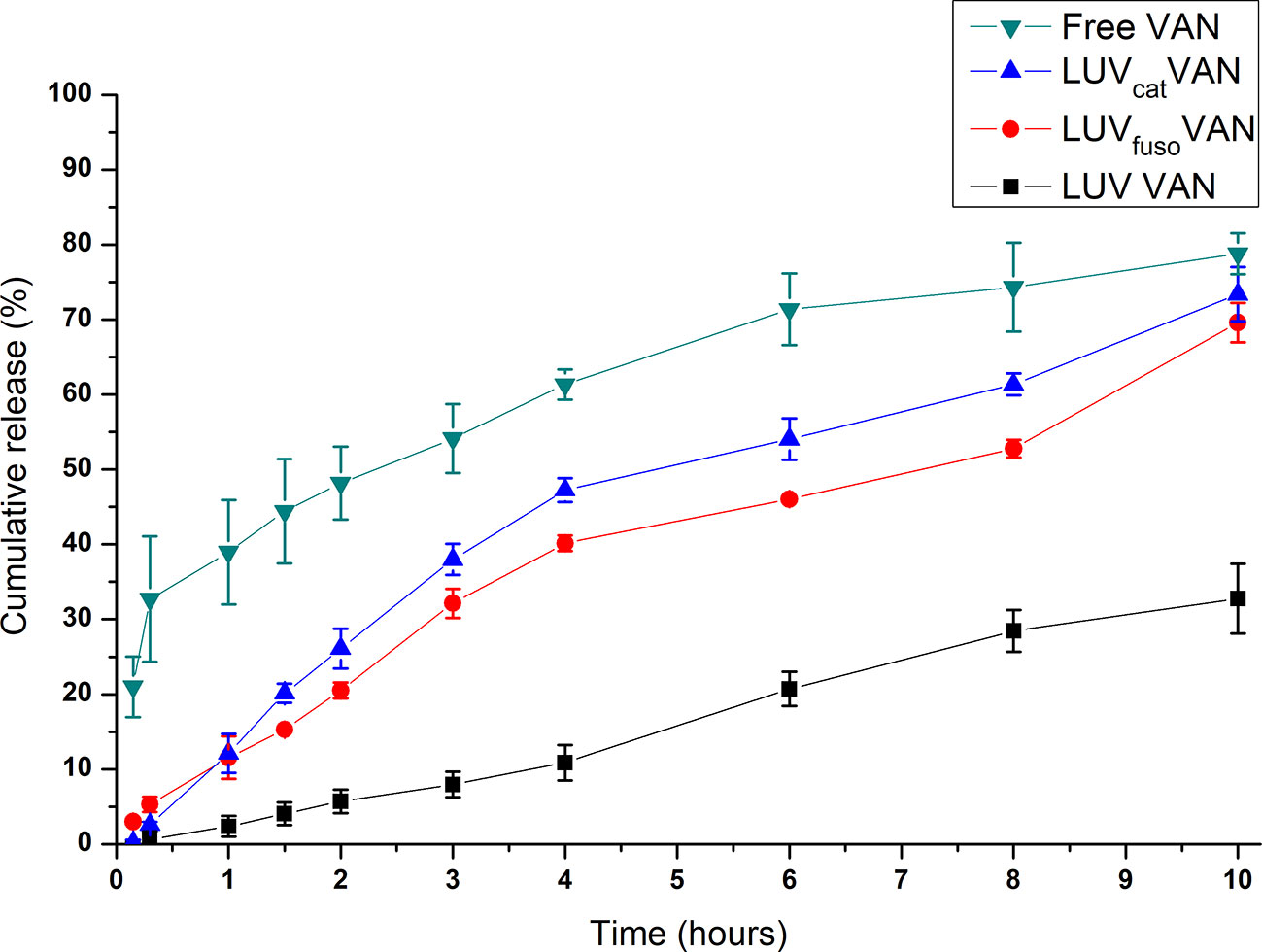
Figure 3 In vitro release kinetics of VAN in solution and encapsulated in the liposomal formulations, at 37°C. Two-way ANOVA, Tukey, P < 0.05. There were statistical difference between groups, as follows: LUV VAN × LUVfuso VAN—from 1 to 10 h; LUV VAN × LUVcat VAN—from 1 to 10h; LUV VAN × free VAN—from 0.15 to 10 h; LUVfuso VAN × free VAN—from 0.15 to 8 h; LUVcat VAN × free VAN—from 0.15 to 8 h.
The LUV VAN formulation showed slower release profile than the other liposomes (p < 0.05), whereas LUVfuso VAN and LUVcat VAN were found to have very similar release kinetics (p > 0.05). As expected, VAN-free formulations showed greater percent release at all timepoints, with a significant difference from the other liposomal formulations (p < 0.05).
Antimicrobial Activity
Free and encapsulated VAN LUV formulations affected bacterial growth in both MSSA (29213) and MRSA (43300) strains, with MIC values ranging between 0.78 and 1.56 µg/ml. These findings are in line with the information provided by the CLSI concerning S. aureus susceptibility to VAN (CLSI, 2012).
Next, the formulations were tested for their inhibitory effects on S. aureus ATCC 29213 biofilm adherence and formation. As shown in Figure 4, treatment with all formulations inhibited biofilm formation in a dose-dependent fashion. Free VAN was found to inhibit biofilm formation at MIC (1.56 µg/ml) and higher concentrations as compared to the untreated biofilm control, while the inhibitory effects of liposome-encapsulated VAN were only seen from 2 × MIC (3.13 µg/ml).
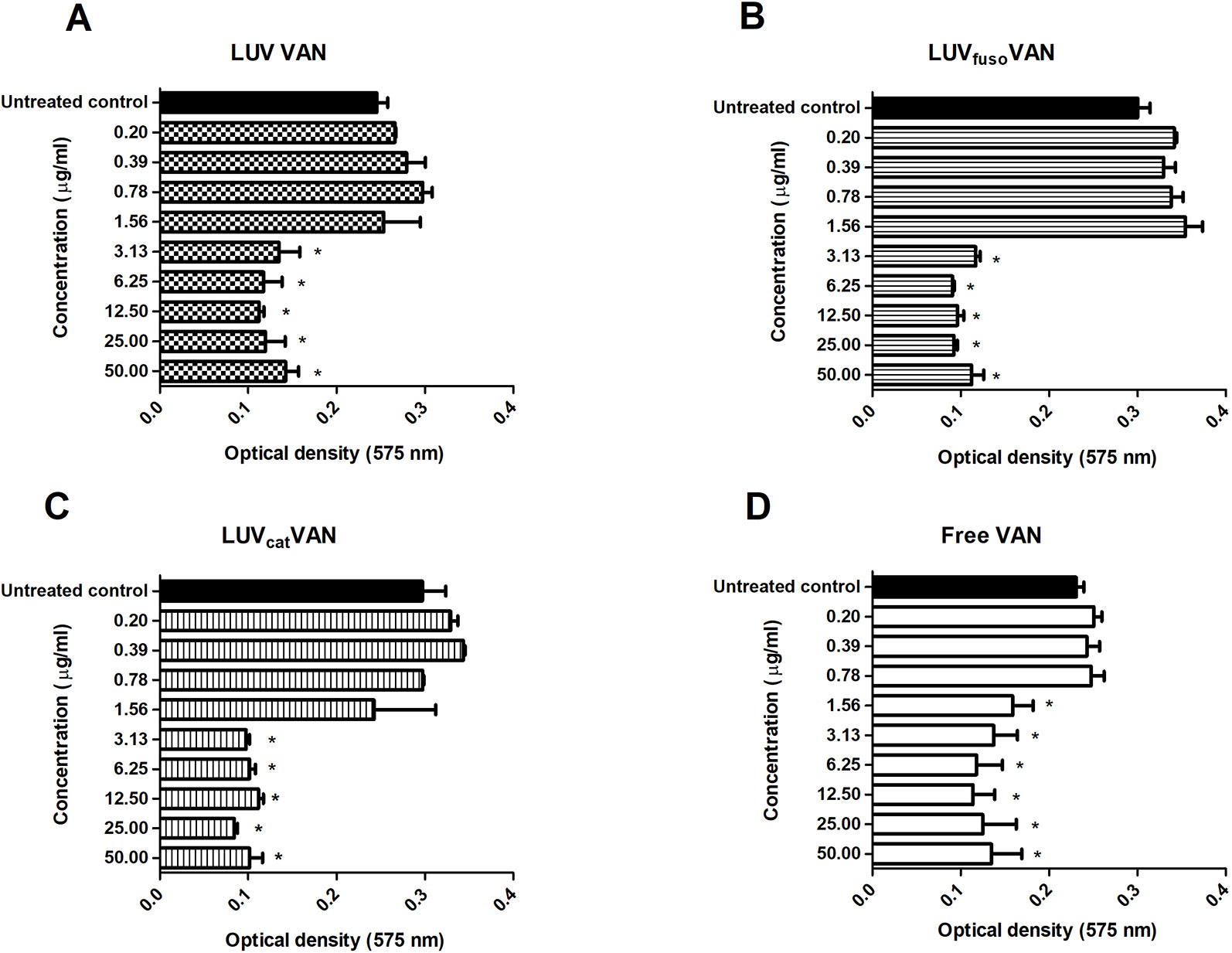
Figure 4 Free and liposome-encapsulated VAN formulations for their inhibitory effects on S. aureus ATCC 29213 biofilm adherence. Mean ( ± SD) optical density values of S. aureus biofilms treated with different concentrations of VAN encapsulated into LUV VAN (A), LUVfuso VAN (B), LUVcat VAN (C), or free VAN solution (D). The asterisk “*” indicates statistically significant difference between the drug treatment and its respective untreated control at p < 0.05 (One-way ANOVA, followed by Tukey’s post-hoc test).
These results corroborate those of the in vitro release kinetics assay (Figure 3), in which encapsulated VAN showed a late release profile as compared to free VAN. These can be attributed to the % amount of VAN encapsulated into the liposomes, so that just a fraction amount of VAN is available to immediately act. Thus, it is likely that a lower amount of VAN molecules was initially released from the liposomal formulations, thereby slowing up their overall antimicrobial effects.
The inhibitory effects of the formulations on biofilm viability were also investigated. Figure 5 shows the mean (± SD) CFU/ml (Log10) of biofilms treated for 24 h at 1 × MIC, 10 × MIC, and 50 × MIC. The data was compared among treatment groups and the untreated control. At 1 × MIC, only LUVcat VAN caused a significant decrease in the number of viable biofilm cells (p < 0.01). Nevertheless, at 10 × MIC and 50 × MIC all formulations showed significant inhibitory effects as compared to the untreated control (p < 0.05). Free VAN was not able to affect biofilm viability significantly at 10 × MIC (p > 0.05), but it did at 50 × MIC (p < 0.05). When liposomal formulations were compared among themselves, we observed that LUVfuso VAN had the most noticeable inhibitory potential on mature biofilms, followed by LUVcat VAN and LUV VAN, with significant differences among them (p < 0.05).
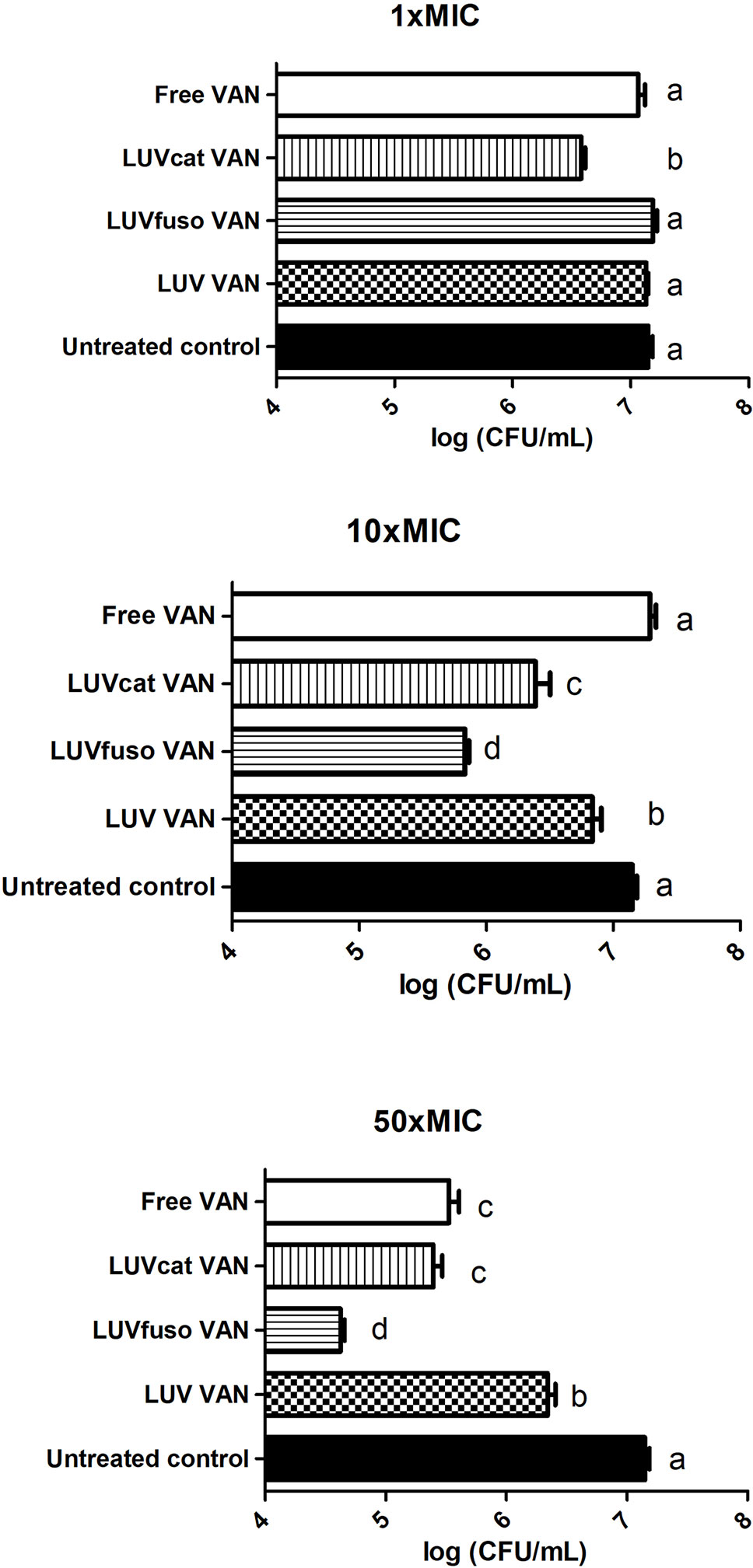
Figure 5 The inhibitory effects of the formulations on biofilm viability. Inhibitory effects of liposomal and plain formulations on S. aureus ATCC 29213 mature biofilm viability at 1 × MIC, 10 × MIC, and 50 × MIC. The values are expressed as mean ( ± SD) of CFU/ml. Different letters indicate significant differences between groups (p < 0.05 One-way ANOVA, with Tukey’s post-hoc test).
The effects on mature biofilms treated with LUVcat VAN and free VAN were found to be similar at 50 × MIC (p > 0.05) and greater than those promoted by LUV VAN (p < 0.05). LUVfuso VAN was the most active formulation against S. aureus biofilm viability when compared to the other groups (p < 0.05). LUVfuso VAN reduced biofilm viability by 3.5 Log10 CFU/ml (35×); LUVcat VAN and free VAN caused a reduction of 2.5 Log10 CFU/ml (25×), while LUV VAN reduced biofilm viability by 1 log10 CFU/ml (10×) in comparison to the control.
Discussion
Nosocomial and community-acquired MRSA infections remain a major concern in global health and have driven the adoption of public policies and medical research in this field (Honary et al., 2013; Elkhodairy et al., 2014; Holland et al., 2014). Evidence has shown the promising results of liposomal vesicles as drug carriers for pharmaceutical application (Kim et al., 1999; Nicolosi et al., 2010; Ma et al., 2011) (25, 26, 30). Herein, we report the development, characterization, and antimicrobial properties of experimental formulations containing VAN encapsulated into conventional, fusogenic and cationic liposomes. We compared the different formulations and demonstrated that the drug-delivery liposomes were more active than VAN in solution in reducing mature biofilm, with better efficacy for LUVfuso VAN.
Our goal when selecting the liposomal formulations was to achieve greater interaction with bacterial cells and, thereby, facilitate penetration into mature biofilms. Conventional (LUV) liposomes contain a mixture of EPC and cholesterol, which increases the rigidity and stability of the vesicles (de Paula et al., 2012). LUVfuso liposomes contain DOPE in their composition, which promotes destabilization of the lipid bilayer (towards inverse hexagonal structures) at acidic pH as it occurs in infected tissue. The use of DPPC was required for stabilization of the lipid bilayers due to the presence of DOPE. Additionally, EPC and CHEMS contribute to greater stability of the formulation (Aoki et al., 2015; Nicolosi et al., 2015). LUVcat liposomes contained stearylamine, EPC, and cholesterol in their composition. Sa is a positively charged lipid that facilitates, through electrostatic interactions, adsorption in the negatively charged bacterial biofilm (Balazs and Godbey, 2011). In order to prevent lipid oxidation, the antioxidant alpha-tocopherol was added to all liposomal formulations (de Paula et al., 2012).
The effect of VAN encapsulation was observed in changes in the Zeta values and size distribution in comparison to controls (Table 1). Such changes may have occurred because encapsulated VAN has a tendency to be located in the aqueous core or adjacent lipid-water interface near the polar head groups (Bozzuto and Molinari, 2015). This molecular location of VAN in liposomes could contribute to the reduction of size distribution homogeneity and enhance electrostatic attraction among liposomes, as VAN is positively charged. Similar results concerning vesicle size and PDI were also found with tetraether lipid liposomes (Uhl et al., 2017). Moreover, after 180 days of storage (4°C) the structural properties of the liposomes were maintained after VAN encapsulation, presenting desirable size and monodisperse distribution, as required for a drug delivery system.
LUV VAN and LUVcat VAN showed higher %EE (32.5% and 10.1%, respectively) than those already reported (2.0% and 5.0%, respectively) using equivalent (conventional, cationic) liposomes, but prepared by sonication and containing 20 mg/ml VAN (Kadry et al., 2004). On the other hand, Nicolosi et al. (2010) observed greater %EE (65.8%) for fusogenic liposomes (prepared by the reverse-phase evaporation method) as compared to our findings (11.4%) (Nicolosi et al., 2010). According to the authors, the preparation method and drug concentration in the liposomal suspension may have influenced the high upload (Muppidi et al., 2012).
In this study, no significant difference was observed in the release kinetics of VAN-containing LUVfuso and LUVcat. Both formulations released 12% of VAN after 1 h, whereas LUV VAN released 2% and free VAN 33%. The differences in the drug controlled release profile among the liposomal formulations may be a result of their diverse %EE (Lankalapalli et al., 2015; Liu et al., 2015). Recently (Lankalapalli et al., 2015), evaluated the release kinetics of VAN from conventional liposomes EPC : Chol liposomes with VAN (10 mg/ml), prepared by the ethanol injection method. The authors observed similar results to those found in our study regarding VAN release from LUV VAN liposomes, and different results with regard to release of free VAN, which was about 42% after 22 h. This divergence may be related to the free VAN concentration used in the donor compartment, which was 100 mg/ml in the study by Lankalapalli et al. and 10 mg/ml in our study.
It is also known that VAN exerts antibacterial action by inhibiting the synthesis of cell wall peptidoglycans (Howden et al., 2010). This drug has a high affinity to the D-Ala-D-Ala residue from the peptidoglycan precursor, lipid II, thereby blocking the addition of final precursors by transglycosylation and transpeptidation, which ultimately interrupts cell wall formation. In S. aureus, peptidoglycan biosynthesis takes place in the cell division septum in a specific site of the cytoplasmic membrane (Howden et al., 2010). Thus, in order to promote its effects on the cell wall, VAN molecules should penetrate approximately 20 layers of peptidoglycan to reach the division septum and bind to the protein fraction (L-lysine-D-alanyl-D-alanine) of murein monomers used as a substrate for glycosyltransferases. Depending on the bacterial cell cycle phase, the division septum can be completely formed or under formation (Nicolosi et al., 2010). Hence, the distance between the cell wall and the plasma membrane is shorter at the early phases of bacterial growth, which might have contributed to the bactericidal effects of free VAN. However, when bacterial growth reaches a final stage, the division septum is completely formed. As a result, the distance between the cell wall and the plasma membrane is wider, which may hinder the action of free VAN. In this case scenario, it is believed that encapsulated VAN could more effectively penetrate the cell wall and reach the periplasmic space, therefore promoting its antibacterial effects (Sande et al., 2012; Nicolosi et al., 2015). Such increased penetration can explain the improved antibiofilm activity observed in our study for the liposomal formulations.
The MIC values of liposome-encapsulated VAN on S. aureus ATCC 29213 observed in our study are in agreement with those found by Kadry et al. (2004). These authors reported MIC values of 0.75 µg/ml and 1.50 µg/ml for cationic and conventional liposomes, respectively. Another study found that encapsulation of VAN into conventional liposomes reduced by 2 the MIC against MRSA strains as compared to free VAN (Sande et al., 2012). This liposomal formulation was composed of DSPC : DCP:Chol (7:2:1, mol%) containing VAN at 50 mg/ml, which was 5 times higher than the VAN concentration used in our study.
Our findings indicate that free VAN at MIC had better inhibitory effects on early stages of biofilm formation than had the liposomal formulations. The latter inhibited biofilm adherence only from 2 × MIC, probably due to the encapsulation of vancomycin into the liposomes, with less free drug available to interact with forming biofilm. On the other hand, the liposomal formulations showed improved antibacterial activity than free VAN against mature biofilms, particularly LUVfuso VAN which was the most effective. Therefore, encapsulated VAN showed greater bactericidal effects on mature biofilms probably due to its increased ability to penetrate the peptidoglycan layers, whereas free VAN remained trapped in the cell wall.
Fusogenic liposomes have an increased potential to interact with extracellular matrix and cell wall due to their ability to merge with lipid membranes (Forier et al., 2014; Nicolosi et al., 2015). These vesicles are composed of lipids that promote destabilization of the lipid bilayers (Forier et al., 2014) and their fusion with the bacterial cell wall was previously proved through flow cytometry, lipid-mixing assay, electronic transmission microscopy and immunochemistry (Beaulac et al., 1998; Sachetelli et al., 2000; Forier et al., 2014; Wang et al., 2016). These vesicles can pass through the cell wall and deliver VAN into the periplasmic space, thereby making it easier for the drug to reach the division septum and block peptidoglycan biosynthesis (Howden et al., 2010; Sande et al., 2012; Nicolosi et al., 2015). Besides, cationic liposomes may have a higher affinity for negatively charged biofilms, which can decrease VAN delivery time into the infectious focus (Kim et al., 1999; Kadry et al., 2004). Accordingly, these liposomes probably release VAN in the vicinities of the bacterial cell wall due to the affinity with its negative charge, resulting in inhibition of cell wall biosynthesis.
There are other studies with VAN-loaded liposomal formulations (Ma et al., 2011; Barakat et al., 2014), but very few tested the ability in inhibit or eradicate S. aureus biofilm, which is a more resistant form of growth and much less sensitive to antibiotics. In the present study, we compared two formulations that are claimed to be effective against bacterial growth: fusogenic and cationic vesicles. Both formulations were effective in reducing mature biofilm, but with superiority to fusogenic vesicles.
To the best of the author´s knowledge, there are only two studies that encapsulate vancomycin into fusogenic liposomes (Nicolosi et al., 2010; Garcia et al., 2017), but none of them tested the activity against S. aureus. In addition, there are other non-fusogenic VAN-loaded liposomes that were tested against S. aureus, but very few aimed to test against biofilm (Ma et al., 2011; Barakat et al., 2014). Other drug delivery systems have also been proposed to improve drug delivery at sites of infection and to overcome antimicrobial resistance, such as injectable and biodegradable hydrogels (Zhao et al., 2017; Qu et al., 2018; Liang et al., 2019; Qu et al., 2019), polymeric nanoparticles (Lakshminarayanan et al., 2018), metal-based nanoparticles (Brown et al., 2012; Noronha et al., 2017), carbon-based nanoparticles (Zhao et al., 2017; Jiang et al., 2018), etc. Contributing to the development and comparison of antibiotics delivery systems, the present study showed that the liposomes here tested can reduce the formation and viability of mature biofilm, in a way superior to free vancomycin.
Conclusion
We demonstrated the successful development, characterization and stability of LUV, LUVfuso and LUVcat encapsulated VAN formulations. Liposomes improved the antimicrobial activity of vancomycin against S. aureus biofilm, with better efficacy for fusogenic vesicles. Future studies are needed to validate this formulation as a candidate for S. aureus infection control.
Data Availability Statement
All datasets generated for this study are included in the article/supplementary material.
Author Contributions
AS: Conception and design of the project. Acquisition of data. Analysis and interpretation of data. Writing and revision of the manuscript. Approval of the final version of the manuscript. VC: Acquisition of data. Analysis and interpretation of data. Final approval of the version to be published. LR: Acquisition of data. Analysis and interpretation of data. Writing and revision of the manuscript. Approval of the final version of the manuscript. IF: Writing and revision of the manuscript. Approval of the final version of the manuscript. FG: Conception and design of the project. Analysis and interpretation of data. Approval of the final version. EP: Conception and design of the project. Analysis and interpretation of data. Writing and revision of the manuscript. Approval of the final version. MF-M: Conception and design of the project. Analysis and interpretation of data. Writing and revision of the manuscript. Approval of the final version. KC-M: Conception and design of the project. Coordination and execution of the experiments. Analysis and interpretation of data. Writing and revision of the manuscript. Approval of the final version of the manuscript.
Conflict of Interest
The authors declare that the research was conducted in the absence of any commercial or financial relationships that could be construed as a potential conflict of interest.
Acknowledgments
The authors thank the São Paulo Research Foundation (FAPESP, grant no. 2014/14457-5) for financial support and Teuto/Pfizer for kindly donating the vancomycin.
References
Aoki, A., Akaboshi, H., Ogura, T., Aikawa, T., Kondo, T., Tobori, N. (2015). Preparation of pH-sensitive anionic liposomes designed for drug delivery system (DDS) application. J. Oleo. Sci. 64, 233–242. doi: 10.5650/jos.ess14157
Balazs, D. A., Godbey, W. (2011). Liposomes for use in gene delivery. J. Drug Delivery 2011, 326497. doi: 10.1155/2011/326497
Barakat, H. S., Kassem, M. A., El-Khordagui, L. K., Khalafallah, N. M. (2014). Vancomycin-eluting niosomes: a new approach to the inhibition of staphylococcal biofilm on abiotic surfaces. AAPS PharmSciTech. 15 (5), 1263–1274. doi: 10.1208/s12249-014-0141-8
Beaulac, C., Sachetelli, S., Lagace, J. (1998). In-vitro bactericidal efficacy of sub-MIC concentrations of liposome-encapsulated antibiotic against Gram-negative and Gram-positive bacteria. J. Antimicrob. Chemother. 41 (1), 35–41 doi: 10.1093/jac/41.1.35
Bozzuto, G., Molinari, A. (2015). Liposomes as nanomedical devices. Int. J. Nanomed. 10, 975–999. doi: 10.2147/IJN.S68861
Brown, A. N., Smith, K., Samuels, T. A., Lu, J., Obare, S. O., Scott, M. E. (2012). Nanoparticles functionalized with ampicillin destroy multiple-antibiotic-resistant isolates of Pseudomonas aeruginosa and Enterobacter aerogenes and methicillin-resistant Staphylococcus aureus. Appl. Environ. Microbiol. 78 (8), 2768–2774. doi: 10.1128/AEM.06513-11
Butler, M. S., Hansford, K. A., Blaskovich, M. A., Halai, R., Cooper, M. A. (2014). Glycopeptide antibiotics: back to the future. J. Antibiot. 67, 631–644. doi: 10.1038/ja.2014.111
Cereda, C. M., Brunetto, G. B., de Arau´jo, D. R., de Paula, E. (2006). Liposomal formulations of prilocaine, lidocaine and mepivacaine prolong analgesic duration. Can. J. Anaesth. 53, 1092–1097. Available at: https://www.ncbi.nlm.nih.gov/pubmed/17079635. doi: 10.1007/BF03022876
Chakraborty, S. P., Sahu, S. K., Pramanik, P., Roy, S. (2012). In vitro antimicrobial activity of nanoconjugated vancomycin against drug resistant Staphylococcus aureus. Int. J. Pharm. 436, 659–676. doi: 10.1016/j.ijpharm.2012.07.033
CLSI (2012). “Performance Standards for Antimicrobial Susceptibility Testing - Twenty-Second Informational Supplement”: In M100-S25, vol. 32, 3.
Da Silva, C. M. G., Fraceto, L. F., Franz-Montan, M., Couto, V. M., Casadei, B. R., Cereda, C. M. S., et al. (2016). Development of egg PC/cholesterol/α-tocopherol liposomes with ionic gradients to deliver ropivacaine. J. Liposome Res. 26 (1), 1–10. doi: 10.3109/08982104.2015.1022555
de Araujo, D. R., Cereda, C. M. S., Brunetto, G. B., Vomero, V. U., Pierucci, A., Neto, H. S., et al. (2008). Pharmacological and local toxicity studies of a liposomal formulation for the novel local anaesthetic ropivacaine. J. Pharm. Pharmacol. 60 (11), 1449–1457. doi: 10.1211/jpp/60.11.0005
de Paula, E., Cereda, C. M., Fraceto, L. F., de Arau´jo, D. R., Franz-Montan, M., Tofoli, G. R., et al. (2012). Micro and nanosystems for delivering local anesthetics. Expert Opin. Drug Deliv. 9, 1505–1524. doi: 10.1517/17425247.2012.738664
Dong, D., Thomas, N., Thierry, B., Vreugde, S., Prestidge, C. A., Wormald, P. J. (2015). Distribution and Inhibition of Liposomes on Staphylococcus aureus and Pseudomonas aeruginosa Biofilm. PloS One 10, e0131806. doi: 10.1371/journal.pone.0131806
Drulis-Kawa, Z., Dorotkiewicz-Jach, A., Gubernator, J., Gula, G., Bocer, T., Doroszkiewicz, W. (2009). The interaction between Pseudomonas aeruginosa cells and cationic PC : Chol:DOTAP liposomal vesicles versus outer-membrane structure and envelope properties of bacterial cell. Int. J. Pharm. 367, 211–219. doi: 10.1016/j.ijpharm.2008.09.043
Elkhodairy, K. A., Afifi, S. A., Zakaria, A. S. (2014). A promising approach to provide appropriate colon target drug delivery systems of vancomycin HCL: pharmaceutical and microbiological studies. Biomed. Res. Int. 2014, 182197. doi: 10.1155/2014/182197
Forier, K., Raemdonck, K., De Smedt, S. C., Demeester, J., Coenye, T., Braeckmans, K. (2014). Lipid and polymer nanoparticles for drug delivery to bacterial biofilms. J. Control Release 190, 607–623. doi: 10.1016/j.jconrel.2014.03.055
Franz, T. J. (1975). Percutaneous absorption on the relevance of in vitro data. J. Invest. Dermatol. 64, 190–195. Available at: https://www.ncbi.nlm.nih.gov/pubmed/123263. doi: 10.1111/1523-1747.ep12533356
Garcia, C. B., Shi, D., Webster, T. J. (2017). Tat-functionalized liposomes for the treatment of meningitis: An in vitro study. Int. J. Nanomed. 12, 3009–3021. doi: 10.2147/IJN.S130125
Graziano, T. S., Cuzzullin, M. C., Franco, G. C., Schwartz-Filho, H. O., de Andrade, E. D., Groppo, F. C., et al. (2015). Statins and antimicrobial effects: simvastatin as a potential drug against Staphylococcus aureus biofilm. PloS One 10, e0128098. doi: 10.1371/journal.pone.0128098
Gudiol, C., Cuervo, G., Shaw, E., Pujol, M., Carratalà, J. (2017). Pharmacotherapeutic options for treating Staphylococcus aureus bacteremia. Expert Opin. Pharmacother. 18, 1947–1963. doi: 10.1080/14656566.2017.1403585
Holland, T. L., Arnold, C., Fowler, V. G. (2014). Clinical management of Staphylococcus aureus bacteremia: a review. JAMA 312, 1330–1341. doi: 10.1001/jama.2014.9743
Honary, S., Ebrahimi, P., Hadianamrei, R. (2013). Optimization of size and encapsulation efficiency of 5-FU loaded chitosan nanoparticles by response surface methodology. Curr. Drug Deliv. 10, 742–752. Available at: https://www.ncbi.nlm.nih.gov/pubmed/24274636. doi: 10.1001/jama.2014.9743
Howden, B. P., Davies, J. K., Johnson, P. D., Stinear, T. P., Grayson, M. L. (2010). Reduced vancomycin susceptibility in Staphylococcus aureus, including vancomycin-intermediate and heterogeneous vancomycin-intermediate strains: resistance mechanisms, laboratory detection, and clinical implications. Clin. Microbiol. Rev. 23, 99–139. doi: 10.1128/CMR.00042-09
Jiang, L., Su, C., Ye, S., Wu, J., Zhu, Z., Wen, Y., et al. (2018). Synergistic antibacterial 2nanostructures. Nanotechnology 29 (50), 505102. doi: 10.1088/1361-6528/aae424
Kadry, A. A., Al-Suwayeh, S. A., Abd-Allah, A. R., Bayomi, M. A. (2004). Treatment of experimental osteomyelitis by liposomal antibiotics. J. Antimicrob. Chemother. 54, 1103–1108. doi: 10.1093/jac/dkh465
Khameneh, B., Zarei, H., Bazzaz, B. S. F. (2014). The effect of silver nanoparticles on Staphylococcus epidermidis biofilm biomass and cell viability. Nanomed. J. 1, 302–307. doi: 10.7508/NMJ.2015.05.003
Kim, H. J., Jones, M. N. (2004). The delivery of benzyl penicillin to Staphylococcus aureus biofilms by use of liposomes. J. Liposome Res. 14, 123–139. doi: 10.1081/LPR-200029887
Kim, H. J., Michael Gias, E. L., Jones, M. N. (1999). “The adsorption of cationic liposomes to Staphylococcus aureus biofilms”: In Colloids and Surfaces A: Physicochemical and Engineering Aspects. doi: 10.1016/S0927-7757(98)00765-1
Lakshminarayanan, R., Ye, E., Young, D. J., Li, Z., Loh, X. J. (2018). Recent advances in the development of antimicrobial nanoparticles for combating resistant pathogens. Adv. Healthc. Mater. 7 (13), e1701400 doi: 10.1002/adhm.201701400
Lankalapalli, S., Vinai Kumar Tenneti, V. S., Nimmali, S. K. (2015). Design and development of vancomycin liposomes. Ind. J. Pharm. Educ. Res. 49, 208–215. doi: 10.5530/ijper.49.3.6
Liang, Y., Zhao, X., Ma, P. X., Guo, B., Du, Y., Han, X. (2019). pH-responsive injectable hydrogels with mucosal adhesiveness based on chitosan-grafted-dihydrocaffeic acid and oxidized pullulan for localized drug delivery. J. Colloid Interface Sci. 536 (15), 224–234. doi: 10.1016/j.jcis.2018.10.056
Liu, J., Wang, Z., Li, F., Gao, J., Wang, L., Huang, G. (2015). Liposomes for systematic delivery of vancomycin hydrochloride to decrease nephrotoxicity: characterization and evaluation. Asian J. Pharm. Sci. 10 (3), 212–222. doi: 10.1016/j.ajps.2014.12.004
Ma, T., Shang, B. C., Tang, H., Zhou, T. H., Xu, G. L., Li, H. L., et al. (2011). Nano-hydroxyapatite/chitosan/konjac glucomannan scaffolds loaded with cationic liposomal vancomycin: preparation, in vitro release and activity against Staphylococcus aureus biofilms. J. Biomater Sci. Polym. Ed. 22, 1669–1681. doi: 10.1163/092050611X570644
McAuley, M. A. (2012). Allergic reaction or adverse drug effect: correctly classifying vancomycin-induced hypersensitivity reactions. J. Emerg. Nurs. 38, 60–62. doi: 10.1016/j.jen.2011.09.010
McCarthy, H., Rudkin, J. K., Black, N. S., Gallagher, L., O’Neill, E., O’Gara, J. P. (2015). Methicillin resistance and the biofilm phenotype in Staphylococcus aureus. Front. Cell Infect. Microbiol. 5, 1. doi: 10.3389/fcimb.2015.00001
Men, P., Li, H. B., Zhai, S. D., Zhao, R. S. (2016). Association between the AUC0-24/MIC ratio of vancomycin and its clinical effectiveness: a systematic review and meta-analysis. PloS One 11, e0146224. doi: 10.1371/journal.pone.0146224
Moghadas-Sharif, N., Fazly Bazzaz, B. S., Khameneh, B., Malaekeh-Nikouei, B. (2015). The effect of nanoliposomal formulations on Staphylococcus epidermidis biofilm. Drug Dev. Ind. Pharm. 41, 445–450. doi: 10.3109/03639045.2013.877483
Muppidi, K., Pumerantz, A. S., Wang, J., Betageri, G. (2012). Development and stability studies of novel liposomal vancomycin formulations. ISRN Pharm. 2012, 636743. doi: 10.5402/2012/636743
Nicolosi, D., Scalia, M., Nicolosi, V. M., Pignatello, R. (2010). Encapsulation in fusogenic liposomes broadens the spectrum of action of vancomycin against Gram-negative bacteria. Int. J. Antimicrob. Agents 35, 553–558. doi: 10.1016/j.ijantimicag.2010.01.015
Nicolosi, D., Cupri, S., Genovese, C., Tempera, G., Mattina, R., Pignatello, R. (2015). Nanotechnology approaches for antibacterial drug delivery: preparation and microbiological evaluation of fusogenic liposomes carrying fusidic acid. Int. J. Antimicrob. Agents 45, 622–626. doi: 10.1016/j.ijantimicag.2015.01.016
Noronha, V. T., Paula, A. J., Durán, G., Galembeck, A., Cogo-Müller, K., Franz-Montan, M., et al. (2017). Silver nanoparticles in dentistry. Dent. Mater. 33, 1110–1126. doi: 10.1016/j.dental.2017.07.002
Pumerantz, A., Muppidi, K., Agnihotri, S., Guerra, C., Venketaraman, V., Wang, J., et al. (2011). Preparation of liposomal vancomycin and intracellular killing of meticillin-resistant Staphylococcus aureus (MRSA). Int. J. Antimicrob. Agents 37, 140–144. doi: 10.1016/j.ijantimicag.2010.10.011
Qu, J., Zhao, X., Liang, Y., Zhang, T., Ma, P. X., Guo, B. (2018). Antibacterial adhesive injectable hydrogels with rapid self-healing, extensibility and compressibility as wound dressing for joints skin wound healing. Biomaterials. 183, 185–199. doi: 10.1016/j.biomaterials.2018.08.044
Qu, J., Zhao, X., Liang, Y., Xu, Y., Ma, P. X., Guo, B. (2019). Degradable conductive injectable hydrogels as novel antibacterial, anti-oxidant wound dressings for wound healing. Chem. Eng. J. 362, 548–560. doi: 10.1016/j.cej.2019.01.028
Rose, W. E., Fallon, M., Moran, J. J., Vanderloo, J. P. (2012). Vancomycin tolerance in methicillin-resistant Staphylococcus aureus: influence of vancomycin, daptomycin, and telavancin on differential resistance gene expression. Antimicrob. Agents Chemother. 56, 4422–4427. doi: 10.1128/AAC.00676-12
Sachetelli, S., Khalil, H., Chen, T., Beaulac, C., Sénéchal, S., Lagacé, J. (2000). Demonstration of a fusion mechanism between a fluid bactericidal liposomal formulation and bacterial cells. Biochim. Biophys. Acta - Biomembr. 1463 (2), 254–266. doi: 10.1016/S0005-2736(99)00217-5
Sande, L., Sanchez, M., Montes, J., Wolf, A. J., Morgan, M. A., Omri, A., et al. (2012). Liposomal encapsulation of vancomycin improves killing of methicillin-resistant Staphylococcus aureus in a murine infection model. J. Antimicrob. Chemother. 67, 2191–2194. doi: 10.1093/jac/dks212
Shi, J., Mao, N. F., Wang, L., Zhang, H. B., Chen, Q., Liu, H., et al. (2014). Efficacy of combined vancomycin and fosfomycin against methicillin-resistant Staphylococcus aureus in biofilms in vivo. PloS One 9, e113133. doi: 10.1371/journal.pone.0113133
Uhl, P., Pantze, S., Storck, P., Parmentier, J., Witzigmann, D., Hofhaus, G., et al. (2017). Oral delivery of vancomycin by tetraether lipid liposomes. Eur. J. Pharm. Sci. 108, 111–118. doi: 10.1016/j.ejps.2017.07.013
Torchilin, V. (2012). “Liposomes in Drug Delivery,” in Fundamentals and Applications of Controlled Release Drug Delivery. Eds. Siepmann, J., Siegel, R. A., Rathbone, M. J. (Springer), 289–328.
Wang, Z., Ma, Y., Khalil, H., Wang, R., Lu, T., Zhao, W., et al. (2016). Fusion between fluid liposomes and intact bacteria: Study of driving parameters and in vitro bactericidal efficacy. Int. J. Nanomed. 11, 4025–4036. doi: 10.2147/IJN.S55807
Wu, W. S., Chen, C. C., Chuang, Y. C., Su, B. A., Chiu, Y. H., Hsu, H. J. (2013). Efficacy of combination oral antimicrobial agents against biofilm-embedded methicillin-resistant Staphylococcus aureus. J. Microbiol. Immunol. Infect. 46, 89–95. doi: 10.1016/j.jmii.2012.03.009
Zhang, L., Yan, J., Yin, Z., Tang, C., Guo, Y., Li, D., et al. (2014). Electrospun vancomycin-loaded coating on titanium implants for the prevention of implant-associated infections. Int. J. Nanomed. 9, 3027–3036. doi: 10.2147/IJN.S63991
Keywords: fusogenic liposomes, cationic liposomes, Staphylococcus aureus, vancomycin, biofilm
Citation: Scriboni AB, Couto VM, Ribeiro LNdM, Freires IA, Groppo FC, de Paula E, Franz-Montan M and Cogo-Müller K (2019) Fusogenic Liposomes Increase the Antimicrobial Activity of Vancomycin Against Staphylococcus aureus Biofilm. Front. Pharmacol. 10:1401. doi: 10.3389/fphar.2019.01401
Received: 22 February 2019; Accepted: 01 November 2019;
Published: 29 November 2019.
Edited by:
Silvio Barberato-Filho, Universidade de Sorocaba, BrazilReviewed by:
Baolin Guo, Xi’an Jiaotong University, ChinaCristiane Duque, São Paulo State University, Brazil
Copyright © 2019 Scriboni, Couto, Ribeiro, Freires, Groppo, de Paula, Franz-Montan and Cogo-Müller. This is an open-access article distributed under the terms of the Creative Commons Attribution License (CC BY). The use, distribution or reproduction in other forums is permitted, provided the original author(s) and the copyright owner(s) are credited and that the original publication in this journal is cited, in accordance with accepted academic practice. No use, distribution or reproduction is permitted which does not comply with these terms.
*Correspondence: Michelle Franz-Montan, bWljaGVsbGVAZm9wLnVuaWNhbXAuYnI=; Karina Cogo-Müller, a2FyaW5hLm11bGxlckBmY2YudW5pY2FtcC5icg==