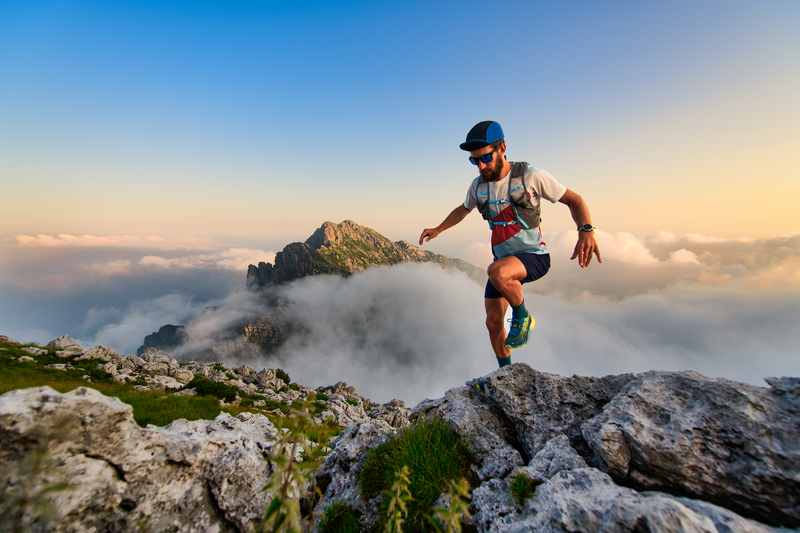
94% of researchers rate our articles as excellent or good
Learn more about the work of our research integrity team to safeguard the quality of each article we publish.
Find out more
ORIGINAL RESEARCH article
Front. Pharmacol. , 13 November 2019
Sec. Experimental Pharmacology and Drug Discovery
Volume 10 - 2019 | https://doi.org/10.3389/fphar.2019.01305
Background: Pulmonary fibrosis (PF) is a devastating interstitial lung disease and characterized by an abnormal accumulation of extracellular matrix (ECM). Nintedanib (NDN) and pirfenidone are two approved therapies for PF, but their potential side-effects have been reported. Recently, the use of natural supplements for PF is attracting attention. Alpha-mangostin (α-MG) is an active xanthone-type compound isolated from the nutritious fruit mangosteen.
Purpose: In the present study, the potential effect and underlying mechanism of α-MG were evaluated in bleomycin (BLM)-induced PF and activated primary lung fibroblasts (PLFs).
Methods: Histopathological changes and collagen deposition were analyzed via hematoxylin-eosin staining and Masson staining, the expression of nicotinamide adenine dinucleotide phosphate oxidase-4 (NOX4) involved in oxidative stress in lung tissues was analyzed by immunochemistry staining. The expressions of α-smooth muscle actin (α-SMA), collagen I (Col I), p-adenosine 5′-monophosphate-activated protein kinase (AMPK)/AMPK, and NOX4 were detected by Western blot, immunofluorescence or RT-PCR, and effects of α-MG on cell viability were detected using the 3-(4,5-dimethylthiazol-2-yl)-2,5-diphenyl tetrazolium bromide.
Results:In vivo results demonstrated that α-MG treatment (10 mg/kg/day) significantly ameliorated BLM-induced deposition of ECM in lung tissues. Moreover, α-MG could inhibit protein expressions of α-SMA and Col I as well as its mRNA levels. In addition, α-MG also significantly inhibited transforming growth factor-β1/Smad2/3 pathway and regulated the protein expression of matrix metalloproteinase-9 and tissue inhibitor of metalloproteinase-1 in lung tissues. In vitro results demonstrated that α-MG significantly increased p-AMPK/AMPK but reduced the protein expression level of α-SMA and Col I as well as NOX4 in activated PLFs. Further study demonstrated that these improvement effects were significantly blocked by compound C.
Conclusion: α-MG treatment significantly decreased oxidative stress in lungs partly by activating AMPK mediated signaling pathway in BLM-induced PF and activated PLFs and decreased the deposition of ECM. The present study provides pharmacological evidence to support therapeutic application of α-MG in the treatment of PF.
Pulmonary fibrosis (PF) is a chronic, progressive, and currently untreatable complication of several interstitial pulmonary diseases (Todd et al., 2012; Rafii et al., 2013). Clinical research demonstrated that prednisone, azathioprine, and N-acetylcysteine increased mortality in PF patients compared with the placebo (Raghu et al., 2012). Nintedanib (NDN) and pirfenidone are two approved therapies for PF, but their potential side-effects have been reported (Raghu et al., 2015). Recently, the use of natural supplements for PF is attracting attention (Lili et al., 2015; He et al., 2015; Xia et al., 2016).
Alpha-mangostin (α-MG) is a major xanthone-type compound in the peels of mangosteen (Nguemfo et al., 2009), which exhibits anti-inflammatory, anti-tumor (Hsieh et al., 2013; Franceschelli et al., 2016), anti-steatosis (Choi et al., 2015), and other biological activities (Chao et al., 2011; Chae et al., 2016). Although it has been reported that it can attenuate liver fibrosis (Khunvirojpanich et al., 2015), the therapeutic effect of α-MG on PF is not sure.
The fibrogenic process is a complex three-step pathophysiological process, including inflammatory responses, effector cell activation and migration, and excessive extracellular matrix (ECM) deposition. PF presents as excessive accumulation of ECM. Activated alveolar epithelial cells and myofibroblasts are the primary source of matrix components, which plays a critical role in the development of PF (Raghu, 2011). Elevated transforming growth factor-β1 (TGF-β1) levels are major inducers of PF (Leask and Abraham, 2004), direct blockade of TGF-β1 may be a strategy to suppress matrix protein synthesis, but this approach would produce many undesired systemic side effects because of the multiple biological activities of TGF-β1 (Pardali et al., 2010; Deelman and Sharma, 2015). Therefore, further characterization of the downstream signaling pathway of TGF-β1 in the generation of matrix proteins may provide an alternative anti-fibrotic strategy. Recent evidence suggests NAPDH oxidases-4 (NOX4) and AMP-activated protein kinase (AMPK) as potential therapeutic targets in PF (Amara et al., 2010; Li et al., 2015). NOX4 is expressed in pulmonary fibroblasts and targeting of NOX4 inhibited the fibrotic process in experimental PF (Chan et al., 2009; Hecker et al., 2009). AMPK is a metabolic regulator, the activation of it exhibits improvement in metabolic disorders and prevention of organ dysfunction during pulmonary diseases (Myerburg et al., 2010; Muanprasat et al., 2013; Zhao et al., 2014; Malik et al., 2015). What’s more, several studies have demonstrated that activation of AMPK effectively inhibited the over-expression of NOX4 in activated lung fibroblasts (Park et al., 2012; Jian et al., 2013; Li et al., 2015; Lu et al., 2015). However, whether the treatment of α-MG can target AMPK and NOX4 remains unknown. And after treatment of α-MG, the role of AMPK and related signaling pathway in the progression of PF remains to be further studied. Bleomycin (BLM) is a chemotherapeutic cancer agent, and PF is a primary side effect of clinic treatment. In present study, we evaluated the potential protective effect and underlying mechanism of α-MG in both BLM-induced PF and activated lung fibroblasts, which involved AMPK mediated signaling pathway. We demonstrated a beneficial function of α-MG in PF, and activating of AMPK by α-MG may be a therapeutic approach for the PF treatment.
α-MG (purity over 99%) was prepared by Chengdu Biopurify Phytochemical Ltd. (Chengdu, China). BLM was obtained from Nippon Kayaku (Tokyo, Japan). NDN (40 mg/kg) was obtained from Shanghai Yuke Chemical Co., Ltd. (Shanghai, China). Recombinant TGF-β1 was purchased from PeproTech Inc. (Rocky Hill, NJ, USA). Compound C (CC) was provided by Selleck (Shanghai, China). 3-(4,5-Dimethylthiazol-2-yl)-2,5-diphenyl tetrazolium bromide (MTT) was obtained from Biosharp Technology Inc. (Hefei, China).
A Hydroxyproline (HYP) Assay Kit was purchased from Nanjing Jiancheng Bio-Engineering Institute (Nanjing, China). Antibodies against AMPK, phospho-AMPK (p-AMPK), TGF-β1, Smad2/3, and phospho-Smad2/3 (p-Smad2/3) were purchased from Cell Signal Technology Inc. (Massachusetts, USA). Antibodies against β-actin, alpha-smooth muscle actin (α-SMA), and horseradish peroxidase (HRP)-conjugated secondary antibody were purchased from Bioworld Technology Inc. (Dublin, OH, USA). An antibody against NOX4 was purchased from Abcam Technology Inc. (Cambridge, UK). An antibody against collagen-1 (Col I) was purchased from Wanleibio Technology Inc.
Male C57/BL6 mice (6–8 weeks old, weighing 18–20 g) were supplied from the Comparative Medicine Centre of Yangzhou University (Yangzhou, China). Animals were cared for according to the General Recommendation and Provisions of the Chinese Experimental Animals Administration Legislation. The Institutional Ethical Committee of China Pharmaceutical University approved the animal protocol (SCXK, su, 2012–0004). Mice were housed in a climate-controlled room at 22 ± 2°C and 50 ± 10% humidity (12 h light/dark cycle), with free access to food and drinking water.
BLM-induced PF was induced as described previously (You et al., 2015). Briefly, mice were acclimated for 1 week and divided randomly into four groups. Mice were anaesthetized via intraperitoneal injection of a chloral hydrate solution (4%, 10 ml/kg) followed by intratracheal instillation of BLM (5 mg/kg) or saline as a control. One week after BLM administration, α-MG (10 mg/kg) was intragastrically administered daily for 14 consecutive days. NDN (40 mg/kg) was used as a positive control drug. The control groups and BLM groups received the same volume of 0.9% sterilized saline. Mice were euthanized on day 21 via an excessive intraperitoneal injection of chloral hydrate. Lung tissue was excised for lung coefficient measurement (lung weight/body weight; mg/g). The left lower lobes were fixed in 10% formalin for histopathological examination, and the remaining lung tissue was stored at −80°C.
Lung tissues were fixed with 10% formalin, embedded in paraffin, sectioned, and stained with hematoxylin-eosin (H&E) or Masson’s trichrome. Experienced pathologists performed pathological evaluations in a single-blind manner. The specific scoring rules are as follows (Szapiel et al., 1979): 1) whether the alveolar wall is thickened, congested, and there is no hemorrhage or edema in the alveolar cavity; 2) presence or absence of fibrous tissue hyperplasia, emphysema, macrophage hyperplasia, or other cell proliferation; 3) bronchial epithelial cells with or without degeneration, necrosis and other diseases. According to the degree of light to heavy lesions: basic normal score is 0, slight lesions are recorded as 0.5, mild lesions are scored as 1 point, moderate lesions are scored as 2 points, and severe lesions are scored as 3 points. Sections were viewed using an Olympus BX53 microscope at 200× magnification.
Approximately 40 mg of lung tissue homogenate was hydrolyzed in 1 ml of hydrolysate at 95°C for 20 min. HYP content in lung tissue was determined using commercial test kits according to the manufacturers’ instructions. HYP content was expressed as µg/mg.
Paraffin-embedded lung sections (5 µm) were incubated with 3% H2O2 to eliminate endogenous peroxidase. Antigen retrieval was performed via heating, and non-specific binding sites were blocked using 5% skim milk in phosphate buffer saline (PBS) for 1 h. Sections were incubated with primary antibodies and second antibodies conjugated with HRP. Color was visualized via incubation of the sections with diaminobenzidine (DAB). Sections were viewed at 200× magnification.
Before immunofluorescence staining, cells were pretreated with or without the AMPK inhibitor CC (50 nM) for 1.5 h and subsequently in the absence or presence of TGF-β1 (10 ng/ml), α-MG (50 nM), or control [dimethylsulfoxide (DMSO)] for 48 h. Then, cells were fixed with 4% paraformaldehyde/PBS for 15 min, followed by incubation with 0.3% Triton X-100/PBS for 10 min, 2% bovine serum albumin (BSA)/PBS block solution for 2 h, and probed with primary antibody α-SMA (Bioworld Technology, Dublin, OH, USA; 1:200) overnight at 4°C. Cy3-conjugated secondary antibodies were used to amplify the signal. 4’,6-Diamidino-2-phenylindole (DAPI) was used to stain nuclei. Images were obtained by fluorescent microscopy (Olympus IX53).
Mouse primary lung fibroblasts (PLFs) were isolated as previously described (Tao et al., 2017). Briefly, PLFs outgrown from lung fragments were cultured in fibroblast growth media [Dulbecco’s modification of eagle’s medium (DMEM) with 10% fetal bovine serum (FBS) and penicillin-streptomycin] at 37°C in a 5% CO2 atmosphere. The percentage of fibroblasts was identified as 95% using morphology under microscope. Cells were detached with 0.25% trypsinization and seeded in 96-well plates at 4 × 104 cells per well or six-well plates at 2 × 105 cells per well. Cells were pretreated with or without the AMPK inhibitor CC (50 nM) for 1.5 h and subsequently in the absence or presence of TGF-β1 (10 ng/ml), α-MG (1–50 nM) or control (DMSO) for 48 h.
Cell viability was assessed using the MTT assay. Briefly, cells were seeded in 96-well plates at 4×104 cells per well and incubated in DMEM containing 10% FBS for 24 h. After pretreatment with or without drugs for 48 h, MTT (5 mg/ml) was added to the wells and incubated for an additional 4 h. The optical density was measured at 490 nm.
Total proteins extracted from lung homogenates or cell lysates were lysed in ice-cold RIPA lysis buffer containing a 1:100 dilution of phenylmethanesulfonyl fluoride (PMSF, Beyotime, China). Protein concentrations were determined using the bicinchoninic acid (BCA) Protein Assay Kit (Beyotime, China). Samples were boiled for 10 min, equal amounts of protein (50 µg/lane) were separated using SDS-PAGE and transferred to polyvinylidene fluoride membranes (Merck Millipore, Billerica, USA). Membranes were probed with primary antibodies overnight at 4°C and incubated with HRP-conjugated secondary antibodies at 25°C for 2 h. Bands were visualized using high-sig electrochemiluminescence (ECL) detection reagent (Tanon, China).
Total RNA was extracted from lung tissues using TRIzol reagent (Invitrogen Life Technologies, USA), reverse-transcribed to complementary DNA (cDNA) using the TransScript First-Strand cDNA Synthesis Kit (Toyobo, Japan), and stored at −80°C. Relative gene expression was quantified using Q-PCR using SYBR® Premix Ex TaqTM (Takara, China) in StepOneTM Real-Time PCR (Life Technologies, USA). Total RNA (0.5 µg) was reverse transcribed before the following PCR conditions were performed: 94°C for 2 min followed by 40 cycles at 94°C for 15 s, 58°C for 30 s, 72°C for 30 s, and final extension at 72°C for 10 min. Table 1 shows the primer sequences (5′ to 3′), and data were quantified using the comparative Ct (ΔCt) method. The results are presented as the mean ratio to β-actin.
All data were presented as mean ± SD. Comparisons between two groups were analyzed using Student’s t-test, and one-way ANOVA with Dunnett’s multiple comparison test was used for comparisons of multiple groups. Significance of survival curve was analyzed using the Kaplan-Meier method and log-rank test. Statistical analyses were performed using GraphPad Prism (San Diego, CA). P-values< 0.05 were considered to be statistically significant.
BLM-induced murine PF is a useful experimental model of interstitial PF. C57/BL6 mice are highly susceptible to BLM-induced fibrosis (Lattaa et al., 2015). Therefore, C57/BL6 mice were selected to prove α-MG’s effectiveness on BLM-induced PF model. Day 7 after BLM administration is considered the beginning of the fibrotic phase with concomitant resolution of the acute inflammatory reaction. α-MG (10 mg/kg) was intragastrically administered at day 7 for 14 days after BLM (5 mg/kg) administration. BLM (16.2 g in average in day 21) significantly decreased mouse body weight compared with CON (22.9 g in average in day 21), but treatment with α-MG (10 mg/kg/day) (19.4 g in average in day 21) or NDN (positive drug, 40 mg/kg/day) (16.7 g in average in day 21) tended to not only prevent the body weight loss and survival rate reduction (Figures 1A, B), but also exhibited a significant decrease in the lung coefficient and HYP levels (Figures 1C, G). Qualitative examination of lung sections using H&E and Masson trichrome staining suggested that α-MG markedly ameliorated pulmonary histopathological changes and reduced the progression of fibrosis (Figures 1D–F).
Figure 1 Alpha-mangostin (α-MG) treatment diminished bleomycin (BLM)-induced PF damage in mice. One week after BLM treatment (5 mg/kg), mice were intragastrically administered with α-MG (10 mg/kg) or nintedanib (40 mg/kg) once a day for 14 days. Mouse lungs were collected on day 21 after BLM treatment. The body weight (A), survival rate (B), pulmonary coefficient (C), and hydroxyproline content (G) in lungs were determined, and the representative images (HE or Masson’s trichrome staining) of pulmonary histopathological changes in mice (D) and comparisons of the inflammation score (E) and fibrosis score (F) between the experimental groups are shown. Representative H&E and Masson’s trichrome-stained tissue sections of lungs under 200 × magnification. Data were expressed as the means ± SD (n = 9). *p < 0.05, **p < 0.01, ***p < 0.001, NS, non-significant.
The massive secretion of collagen by myofibroblasts is the main source of ECM, while α-SMA is a marker protein of myofibroblasts. As shown in Figures 2A–D, α-MG treatment dramatically reduced the expression of α-SMA and Col I both at mRNA and protein levels.
Figure 2 Alpha-mangostin (α-MG) reduced the expression of α-smooth muscle actin (α-SMA) and collagen I (Col I) in bleomycin (BLM)-induced PF. One week after BLM treatment (5 mg/kg), mice were intragastrically administered with α-MG (10 mg/kg) or nintedanib (40 mg/kg) once a day for 14 days. Mouse lungs were collected on day 21 after BLM treatment. Protein expression of α-SMA (A) and Col I (B) were detected using Western blot analysis. Relative mRNA expression of α-SMA (C), Col I (D) were detected using real time-PCR analysis. Data were expressed as mean ± SD (n ≥ 3). *p < 0.05, **p < 0.01, ***p < 0.001, NS, non-significant.
Increased deposition of ECM and reduced matrix degradation are the major characters of PF. Matrix metalloproteinase (MMPs) accelerate the degradation of ECM in PF lungs while tissue inhibitor of metalloproteinase (TIMPs) are endogenous inhibitor of MMPs and the balance of MMPs and TIMPs is critical to the steady state of ECM (Pardo et al., 2016). After the last drug administration, MMP-9 and TIMP-1 protein expressions were examined. We found an increase in both MMP-9 and TIMP-1 expression in BLM-damaged lung tissues, which were significantly alleviated by treatment with α-MG (Figures 3A, B). Additionally, TGF-β1/Smad2/3 is the most studied pro-fibrotic canonical pathway in the process of PF (Akhurst and Hata, 2012). Compared with the model group, α-MG treatments markedly reduced abnormal expression of TGF-βl expression and Smad 2/3 phosphorylation in lungs (Figures 3C, D).
Figure 3 Alpha-mangostin (α-MG) blocked transforming growth factor-β1 (TGF-β1)/Smad2/3 signaling pathway and regulate MMPs/tissue inhibitor of metalloproteinase (TIMP) system balance in bleomycin (BLM)-induced pulmonary fibrosis. One week after BLM treatment (5 mg/kg), mice were intragastrically administered with α-MG (10 mg/kg) or nintedanib (40 mg/kg) once a day for 14 days. Mouse lungs were collected on day 21 after BLM treatment. Protein expression of MMP-9 (A), TIMP-1 (B), TGF-β1 (C), and p-Smad2/3 and Smad2/3 (D) were detected using Western blot analysis. Data were expressed as mean ± SD (n ≥ 3). *p < 0.05, **p < 0.01, ***p < 0.001, NS, non-significant.
AMPK is a pivotal energy sensor that alleviates the process of fibrogenesis. Current studies identified that AMPK activation blocked increased NOX4 expression in a PF model (Park et al., 2012; Sato et al., 2016). In the present study, we also confirmed that α-MG treatments could significantly promote AMPK activation in lungs compared with the BLM group (Figure 4A)
Figure 4 Alpha-mangostin (α-MG) promoted adenosine 5′-monophosphate-activated protein kinase (AMPK) activation and ameliorated oxidative stress in vivo. One week after bleomycin (BLM) treatment (5 mg/kg), mice were intragastrically administered with α-MG (10 mg/kg) or nintedanib (40 mg/kg) once a day for 14 days. Mouse lungs were collected on day 21 after BLM treatment. Expression of p-AMPK, AMPK (A), and nicotinamide adenine dinucleotide phosphate oxidase-4 (NOX4) (B) in lung tissues were detected using Western blot analysis. Representative images showing NOX4 expression using immunochemistry under 200 × magnification (C and D). NOX4 mRNA level was detected using real time-PCR analysis (E). Data were expressed as the means ± SD (n ≥ 3). *p < 0.05, **p < 0.01, ***p < 0.001, NS, non-significant.
Previous study has demonstrated a pivotal role for NOX4 in TGF-β1 signaling and myofibroblast differentiation (Sato et al., 2016). NOX4 is expressed in thickened pulmonary arteries in idiopathic PF (Pache et al., 2011; Cheresh et al., 2013; Ashish and Thannickal, 2016). As shown in Figure 4B–E, α-MG treatment markedly inhibited BLM-induced high expression and transcriptional level of NOX4 protein in lungs using Western blot analysis, immunochemistry staining, and real-time quantitative PCR analysis, suggesting α-MG can effectively ameliorate BLM-induced oxidative stress in lungs. Therefore, further research between AMPK activation and NOX4 inhibition following α-MG treatment is needed to elucidate this mechanism.
BLM-induced PF model in mice is characterized by activated myofibroblasts (Bhattacharyya et al., 2013). Trans-differentiation of lung fibroblasts into myofibroblasts facilitated lung wound healing via synthesis of ECM during the repair process (Hinz et al., 2007; Wynn, 2011), and myofibroblasts are characterized by α-SMA expression and collagen synthesis (Klingberg et al., 2013). In the present study, mouse PLFs were treated with TGF-β1 (10 ng/ml), and fibrotic-related protein expression was determined through Western blot analysis. Cell viability of PLFs was investigated using the MTT assay. As shown in Figure 5A, α-MG could significantly inhibit the proliferation of activated PLFs at 100 nM, but no effect on the viability of normal or activated cells was observed at 1–50 nM. Then, α-MG (10 or 50 nM) significantly inhibited α-SMA and Col I expression (Figures 5B, C), suggesting that α-MG can significantly inhibit TGF-β1-induced trans-differentiation of lung fibroblast. In addition, α-MG also significantly activated AMPK but suppressed NOX4 overexpression in activated PLFs (Figures 5D, E)
Figure 5 Alpha-mangostin (α-MG) inhibited transforming growth factor-β1 (TGF-β1)-induced lung fibroblasts differentiation in vitro. Mouse primary lung fibroblasts (PLFs) were treated with TGF-β1 (10 ng/ml) in the absence or presence of α-MG for 48 h. Inhibitory effects of α-MG (1–100 nM) on cell viability were detected using the MTT assay (A). Protein expressions of α-smooth muscle actin (B), collagen I (C), p-adenosine 5′-monophosphate-activated protein kinase (AMPK), AMPK (D), and nicotinamide adenine dinucleotide phosphate oxidase-4 (E) in PLFs after α-MG treatment (1–50 nM) were detected using Western blot analysis. Data were expressed as mean ± SD (n ≥ 3). *p < 0.05, **p < 0.01, ***p < 0.001, NS, non-significant.
As shown in Figure 6A, α-MG (50 nM) could significantly promote AMPK activation in TGF-β1-activated PLFs which were significantly reversed by the co-treatment with an AMPK inhibitor (Compound C, CC). CC is an effective, reversible, selective AMPK inhibitor. It inhibits acetyl-CoA carboxylase (ACC) or metformin-induced ACC inactivation (Zhou et al., 2001), and inhibition of AMPK activity almost completely inhibits proteolysis of autophagy in HT29 cells (Meley et al., 2006). The activation of AMPK effectively alleviated inflammation-related fibrosis in lungs (Sunad et al., 2018). The suppression of α-MG on elevated protein expression of α-SMA and Col I were significantly blocked by CC in activated PLFs (Figures 6B, C and Figure 7B. Similarly, CC also significantly blocked the protection of α-MG on TGF-β1-stimulated NOX4 expression and Smad 2/3 phosphorylation (Figures 6D, E). Collectively, α-MG effectively inhibited TGF-β1-induced lung myofibroblast differentiation partly through activating AMPK.
Figure 6 Alpha-mangostin (α-MG) exerted protective effects against lung myofibroblast differentiation via the targeting of adenosine 5′-monophosphate-activated protein kinase (AMPK) activation. Mouse primary lung fibroblasts were pre-treated with/without compound C (50 nM) for 1.5 h in the absence or presence of α-MG (50 nM) and transforming growth factor-β1 for 48 h. Expression of p-AMPK, AMPK (A), collagen I (B), α-smooth muscle actin (C), nicotinamide adenine dinucleotide phosphate oxidase-4 (D), p-Smad2/3 and Smad2/3 (E) were detected using Western blotting analysis. Data were expressed as mean ± SD (n ≥ 3). *p < 0.05, **p < 0.01, ***p < 0.001, NS, non-significant.
α-MG is a xanthone-type active ingredient originally isolated from mangosteen with anti-inflammatory, anti-fungal, anti-tumor, anti-oxidant, cardio-protective, and anti-bacterial properties (Peres et al., 2000; Jindarat, 2014; Zhang et al., 2017). Recently study reported that α-MG showed potential anti-fibrotic effects in experimental liver fibrosis (Khunvirojpanich et al., 2015). In the present study, the effect of α-MG against BLM-induced PF in mice and the related molecular mechanism were investigated.
A previous study reported that α-MG exacerbates experimental colitis and promotes dysbiosis in mice (Gutierrez-Orozco et al., 2014), but another study showed α-MG ameliorates dextran sulfate sodium (DSS)-induced colitis through inhibition of nuclear factor kappa-B (NF-κB) and mitogen-activated protein kinase (MAPK) pathways (You et al., 2017). We speculated that these two opposite conclusions may be due to the different amounts of α-MG. So we examined the effect of α-MG on the colon of mice with BLM-induced PF, the result suggested that the treatment of α-MG at the dose of 10 mg/kg didn’t cause colonic lesions (Supplementary Figure 1). Besides, α-MG had no toxicity on normal mice at the dose of 10 mg/kg (Supplementary Figure 2). In conclusion, 10 mg/kg of α-MG for mice is a safe and effective dose.
Severe and progressive scar formation are important characteristics of PF (Barkauskas and Noble, 2014). Excessive lung scarring ultimately severely impairs gas exchange (Berend, 2014). In the BLM-induced PF model, the BLM alone group exhibited significantly elevated HYP levels and obvious collagen deposition in lungs when compared with the control group (Figures 1 and 2), but 1 week after BLM administration, α-MG treatments for 2 weeks significantly decreased lung inflammatory and fibrotic lesions in C57/BL6 mice (Figure 1). HYP is a key amino acid of collagen synthesis in fibrotic lung tissues (Yang et al., 2017). α-MG treatment not only significantly ameliorated the elevated HYP contents in lungs (Figure 1), but also inhibited BLM-induced high levels of TGF-β1, α-SMA and Col I as well as Smad2/3 phosphorylation in lungs (Figures 2 and 3). An imbalance in MMPs and TIMPs may result in fibrosis progression, and MMP-9 activates TGF-β1 and stimulates fibroblast contraction of collagen gels. α-MG treatments obviously reduced BLM-induced abnormal protein expression of MMP-9 and TIMP-1 in lung tissues (Figure 3), suggesting that α-MG could inhibit excessive accumulation of ECM during the process of BLM-induced PF in mice.
Several studies have confirmed there is a close relationship between AMPK and fibrogenesis, AMPK activation may be a possible treatment for PF (Myerburg et al., 2010; Lu et al., 2015; Jiang et al., 2017). The AMPK agonist metformin can down-regulate TGF-β1-induced NOX4 expression and attenuate BLM-induced experimental PF (Sato et al., 2016). Oxidative stress contributes to the development of PF, NOX4 activation prolongs Smad 2/3 phosphorylation in cardiac fibroblasts (Cucoranu et al., 2005). The targeting NOX4 with small interfering RNA (siRNA) also suppresses mouse PF (Hecker et al., 2009) and NOX4 expression is upregulated in lung fibroblasts from idiopathic PF patients (Amara et al., 2007). In vitro studies have demonstrated that xanthones exhibit anti-oxidant activity (Williams et al., 1995; Jung et al., 2006). Mangosteen extract can attenuate the metabolic disorders of high-fat-fed mice via AMPK activation (Chae et al., 2016), and α-MG induces autophagy of glioblastoma cell via AMPK activation (Chao et al., 2011). However, the precise role of AMPK activation by α -MG treatment in its anti-PF effects is not clearly tested. In the present study (Figures 2– 4), we have confirmed that α -MG treatment can dramatically activate AMPK and inhibited NOX4 expression as well as TGF-β1/Smad2/3 signaling pathway in lung tissues compared with the BLM alone group.
The aetiology of PF is not known, but the activation of fibroblasts is a seminal step in the process of PF with the subsequent release of pro-fibrotic factors and ECM proteins, activated fibroblasts are responsible for matrix protein production, which is modulated by TGF-β1 (Wynn, 2007). Lung fibroblast is believed to play an important role in lung tissues repair and regenerative process during the process of BLM-induced PF in mice. In the present study, the mouse PLFs were used to investigate the underlying anti-fibrotic mechanism of α-MG. α-MG treatment (50 nM) significantly inhibited the TGF-β1-induced abnormal expression levels of α-SMA, Col I, and NOX4 (Figure 5). Myofibroblast differentiation is a key element in PF diseases. AMPK inhibition by CC abolished effects of α-MG on TGF-β1-mediated collagen synthesis (α-SMA and Col I) and NOX4 expression (Figures 6 and 7), suggesting that α-MG effectively ameliorated trans-differentiation of lung fibroblasts into myofibroblasts through activating AMPK.
Figure 7 The morphology image of cells and immunofluorescence image of α-smooth muscle actin. The morphology of primary lung fibroblast and the transforming growth factor-β1 (TGF-β1) transdifferentiated cells were presented (A). Immunofluorescence of α-smooth muscle actin (α-SMA) in TGF-β1 stimulated primary lung fibroblasts treated with alpha-mangostin (50 nM) and/or compound C (under 200 × magnification). Red, α-SMA, blue, 4’,6-diamidino-2-phenylindole (B).
Collectively, α-MG treatment exerted anti-fibrotic effects via the targeting of AMPK signaling, which reduced collagen accumulation, modulated the redox status in lung fibroblasts, and ameliorated BLM-induced PF in mice.
All datasets generated for this study are included in the article/Supplementary Material.
The animal study was reviewed and approved by The Institutional Ethical Committee of China Pharmaceutical University.
In this paper, R-SL and G-HX designed the study and carried out experiments. JC and BL contributed to pharmacology experiments. YI provided the experimental background guidance. H-FX contributed to compound preparation. C-FZ as the corresponding author undertook the design of this project and logic train of thought.
Author H-FX was employed by Chengdu Biopurity Phytochemicals Ltd.
The remaining authors declare that the research was conducted in the absence of any commercial or financial relationships that could be construed as a potential conflict of interest.
We would like to thank the National Natural Science Foundation (81573553, 81773982 for Chaofeng Zhang), Youth Academic leaders of the Qinglan Project in Jiangsu province (to Chaofeng Zhang), “Double First-Class” University project (CPU2018GF06) and Postgraduate Research & Practice Innovation Program of Jiangsu Province (KYCX18_0810) for financial support.
α-MG, alpha-mangostin; AMPK, adenosine 5′-monophosphate (AMP)-activated protein kinase; α-SMA, α-smooth muscle actin; ACC, acetyl-CoA carboxylase; BCA, bicinchoninic acid; BLM, bleomycin; BSA, bovine serum albumin; CC, compound C; Col I, collagen I; DAB, diaminobenzidine; DAPI, 4’,6-diamidino-2-phenylindole; DMEM, Dulbecco’s modification of Eagle’s medium; ECL, electrochemiluminescence; ECM, extracellular matrix; FBS, fetal bovine serum; H&E, hematoxylin-eosin; HRP, horseradish peroxidase; HYP, hydroxyproline; MAPK, mitogen-activated protein kinase; MMP-9, matrix metalloproteinase-9; MTT, 3-(4,5-dimethylthiazol-2-yl)-2,5-diphenyl tetrazolium bromide; NDN, nintedanib; NF-κB, nuclear factor kappa-B; NOX4, NAPDH oxidases-4; PBS, phosphate buffer saline; PF, pulmonary fibrosis; PLFs, primary lung fibroblasts; siRNA, small interfering RNA; TGF-β1, transforming growth factor-β1; TIMP-1, tissue inhibitor of metalloproteinase-1.
The Supplementary Material for this article can be found online at: https://www.frontiersin.org/articles/10.3389/fphar.2019.01305/full#supplementary-material
Akhurst, R. J., Hata, A. (2012). Targeting the TGFβ signalling pathway in disease. Nat. Rev. Drug Discovery 11, 790–811. doi: 10.1038/nrd3810
Amara, N., Bachoual, R., Desmard, M., Golda, S., Guichard, C., Lanone, S., et al. (2007). Diesel exhaust particles induce matrix metalloprotease-1 in human lung epithelial cells via a NADP(H) oxidase/NOX4 redox-dependent mechanism. Am. J. Physiol. Lung Cell Mol. Physiol. 293, 170–181. doi: 10.1152/ajplung.00445.2006
Amara, N., Goven, D., Prost, F., Muloway, R., Crestani, B., Boczkowski, J. (2010). NOX4/NADPH oxidase expression is increased in pulmonary fibroblasts from patients with idiopathic pulmonary fibrosis and mediates TGFβ1-induced fibroblast differentiation into myofibroblasts. Thorax 65, 733–738. doi: 10.1136/thx.2009.113456
Ashish, K., Thannickal, V. J. (2016). Redox mechanisms in age-related lung fibrosis. Redox Biol. 9, 67–76. doi: 10.1016/j.redox.2016.06.005
Barkauskas, C. E., Noble, P. W. (2014). Cellular mechanisms of tissue fibrosis. 7. New insights into the cellular mechanisms of pulmonary fibrosis. Am. J. Physiol. Cell Physiol. 306, C987–C996. doi: 10.1152/ajpcell.00321.2013
Berend, N. (2014). Respiratory disease and respiratory physiology: putting lung function into perspective interstitial lung disease. Respirology 19, 952–959. doi: 10.1111/resp.12348
Bhattacharyya, S., Kelley, K., Melichian, D. S., Tamaki, Z., Fang, F., Su, Y., et al. (2013). Toll-Like receptor 4 signaling augments transforming growth factor-b responses: a novel mechanism for maintaining and amplifying fibrosis in scleroderma. Am. J. Pathol. 182: 192–205. doi: 10.1016/j.ajpath.2012.09.007
Chae, H. S., Kim, Y. M., Bae, J. K., Sorchhann, S., Yim, S., Han, L., et al. (2016). Mangosteen extract attenuates the metabolic disorders of high-fat-fed mice by activating AMPK. J. Med. Food 19, 148–154. doi: 10.1089/jmf.2015.3496
Chan, E. C., Jiang, F., Peshavariya, H. M., Dusting, G. J. (2009). Regulation of cell proliferation by NADPH oxidase-mediated signaling: potential roles in tissue repair, regenerative medicine and tissue engineering. Pharmacol. Ther. 122, 97–108. doi: 10.1016/j.pharmthera.2009.02.005
Chao, A. C., Hsu, Y. L., Liu, C. K., Kuo, P. L. (2011). α-Mangostin, a dietary xanthone, induces autophagic cell death by activating the AMP-activated protein kinase pathway in glioblastoma cells. J. Agric. Food Chem., 59, 2086–2096. doi: 10.1021/jf1042757
Cheresh, P., Kim, S. J., Tulasiram, S., Kamp, D. W. (2013). Oxidative stress and pulmonary fibrosis. Biochim. Biophys. Acta 1832, 1028–1040. doi: 10.1016/j.bbadis.2012.11.021
Choi, Y. H., Bae, J. K., Chae, H. S., Kim, Y. M., Sreymom, Y., Han, L., et al. (2015). αMangostin regulates hepatic steatosis and obesity through SirT1-AMPK and PPARγPathways in high-fat diet-induced obese mice. J. Agric. Food Chem. 63, 8399–8406. doi: 10.1021/acs.jafc.5b01637
Cucoranu, I., Clempus, R., Dikalova, A., Phelan, P. J., Ariyan, S., Dikalov, S., et al. (2005). NAD(P)H oxidase 4 mediates transforming growth factor-beta1- induced differentiation of cardiac fibroblasts into myofibroblasts. Circ. Res. 97, 900–907. doi: 10.1161/01.res.0000187457.24338.3d
Deelman, L., Sharma, K. (2015). Mechanisms of kidney fibrosis and the role of anti-fibrotic therapies. Curr. Opin. Nephrol. Hypertens. 18, 85–90. doi: 10.1097/MNH.0b013e32831c50a1
Franceschelli, S., Pesce, M., Ferrone, A., Patruno, A., Livia, P., Carlucci, G. A., et al. (2016). A novel biological role of α-Mangostin in modulating inflammatory response through the activation of SIRT-1 signaling pathway. J. Cell. Physiol. 231, 2439–2451. doi: 10.1002/jcp.25348
Gutierrez-Orozco, F., Thomas-Ahner, J. M., Berman-Booty, L. D., Galley, J. D., Chitchumroonchokchai, C., Mace, T., et al. (2014). Dietary α-mangostin, a xanthone from mangosteen fruit, exacerbates experimental colitis and promotes dysbiosis in mice. Mol. Nutr. Food Res. 58, 1226–1238. doi:10.3892/mmr.2015.3333
He, H., Tang, H., Gao, L., Wu, Y., Feng, Z., Lin, H., et al. (2015). Tanshinone IIa attenuates bleomycin-induced pulmonary fibrosis in rats. Molecular Medicine Reports. 11, 4190–4196. doi: 10.3892/mmr.2015.3333
Hecker, L., Vittal, R., Jones, T., Jagirdar, R., Luckhardt, T. R., Horowitz, J. C., et al. (2009). NADPH oxidase-4 mediates myofibroblast activation and fibrogenic responses to lung injury. Nat. Med. 15, 1077–1081. doi: 10.1038/nm.2005
Hinz, B., Phan, S. H., Thannickal, V. J., Galli, A., Bochaton-Piallat, M. L., Gabbiani, G. (2007). The myofibroblast: one function, multiple origins. Am. J. Pathol. 170, 1807–1816. doi: 10.2353/ajpath.2007.070112
Hsieh, S. C., Huang, M. H., Cheng, C. W., Hung, J. H., Yang, S. F., Hsieh, Y. H. (2013). α-Mangostin induces mitochondrial dependent apoptosis in human hepatoma SK-Hep-1 cells through inhibition of p38 MAPK pathway. Apoptosis 18, 1548–1560. doi: 10.1007/s10495-013-0888-5
Jian, M. Y., Alexeyev, M. F., Wolkowicz, P. E., Zmijewski, J. W., Creighton, J. R. (2013). Metformin-stimulated AMPK-α1 promotes microvascular repair in acute lung injury. Am. J. Physiol. Lung Cell Mol. Physiol. 305, 844–855. doi: 10.1152/ajplung.00173.2013
Jiang, S., Li, T., Yang, Z., Yi, W., Di, S., Sun, Y., et al. (2017). AMPK orchestrates an elaborate cascade protecting tissue from fibrosis and aging. Ageing Res. Rev. 38, 18–27. doi: 10.1016/j.arr.2017.07.001
Jindarat, S. (2014). Xanthones from mangosteen (Garcinia mangostana): multitargeting pharmacological properties. J. Med. Assoc. Thai. 97 Suppl 2, S196– S201.
Jung, H. A., Su, B. N., Keller, W. J., Mehta, R. G., Kinghorn, A. D. (2006). Antioxidant xanthones from pericarp of Garcinia mangostana (Mangosteen). J. Agric. Food Chem. 54, 2077–2082. doi: 10.1021/jf052649z
Khunvirojpanich, M., Showpittaporchai, U., Moongkamdi, P., Pradidarcheep, W. (2015). Alpha-mangostin partially preserves expression of ammonia-metabolizing enzymes in thioacetamide-induced fibrotic and cirrhotic rats. J. Med. Assoc. Thai. 98 Suppl 9, S53– S60.
Klingberg, F., Hinz, B., White, E. S. (2013). The myofibroblast matrix: implications for tissue repair and fibrosis. J. Pathol. 229, 298–309. doi: 10.1002/path.4104
Lattaa, V. D., Cecchettini, A., Rya, S. D., Morales, M. A. (2015). Bleomycin in the setting of lung fibrosis induction: From biological mechanisms to counteractions. Pharmacol. Res. 97, 122–130. doi: 10.1016/j.phrs.2015.04.012
Leask, A., Abraham, D. J. (2004). TGF-β signaling and the fibrotic response. FASEB J. 18, 816–827. doi: 10.1096/fj.03-1273rev
Li, L., Huang, W., Li, K., Zhang, K., Lin, C., Han, R., et al. (2015). Metformin attenuates gefitinib-induced exacerbation of pulmonary fibrosis by inhibition of TGF-β signaling pathway. Oncotarget 6, 43605–43619. doi: 10.18632/oncotarget.6186
Lili, G., Haiying, T., Huanyu, H., Jia, L., Jingwei, M., Hong, J., et al. (2015). Glycyrrhizic acid alleviates bleomycin-induced pulmonary fibrosis in rats. Front. Pharmacol. 6, 215. doi: 10.3389/fphar.2015.00215
Lu, J., Shi, J., Li, M., Gui, B., Fu, R., Yao, G., et al. (2015). Activation of AMPK by metformin inhibits TGF-β-induced collagen production in mouse renal fibroblasts. Life Sci. 127, 59–65. doi: 10.1016/j.lfs.2015.01.042
Malik, F. A., Meissner, A., Semenkov, I., Molinski, S., Pasyk, S., Ahmadi, S., et al. (2015). Sphingosine-1-Phosphate is a novelregulator of cystic fibrosis transmembrane conductance regulator (CFTR) activity. PloS One 10, e0130313. doi: 10.1371/journal.pone.0130313
Meley, D., Bauvy, C., Houben-Weerts, J. H. P. M., Dubbelhuis, P. F., Helmond, M. T., Codogno, P., et al. (2006). Amp-activated protein kinase and the regulation of autophagic proteolysis. J. Biol. Chem. 281 (46), 34870–34879. doi: 10.1074/jbc.M605488200
Muanprasat, C., Sirianant, L., Sawasvirojwong, S., Homvisasevongsa, S., Suksamrarn, A., Chatsudthipong, V. (2013). Activation of AMP-activated protein kinase by a plant-derived dihydroisosteviol in human intestinal epithelial cell. Biol. Pharm. Bull. 36, 522–528. doi: 10.1248/bpb.b12-00711
Myerburg, M. M., King, J. D., Oyster, N. M., Fitch, A. C., Magill, A., Baty, C. J., et al. (2010). AMPK agonists ameliorate sodium and fluid transport and inflammation in cystic fibrosis airway epithelial cells. Am. J. Respir. Cell Mol. Biol. 42, 676–684. doi: 10.1165/2009-0147OC
Nguemfo, E. L., Dimo, T., Dongmo, A. B., Azebaze, A. G. B., Alaoui, K., Asongalem, A. E., et al. (2009). Anti-oxidative and anti-inflammatory activities of some isolated constituents from the stem bark of AllanblackiamonticolaStaner L.C (Guttiferae). Inflammopharmacology 17, 37–41. doi: 10.1007/s10787-008-8039-2
Pache, J. C., Carnesecchi, S., Deffert, C., Donati, Y., Herrmann, F. R., Barazzone-Argiroffo, C., et al. (2011). NOX-4 is expressed in thickened pulmonary arteries in idiopathic pulmonary fibrosis. Nat. Med. 17, 31–32. doi: 10.1038/nm0111-31
Pardali, E., Goumans, M. J., Dijke, P. T. (2010). Signaling by members of the TGF-β family in vascular morphogenesis and disease. Trends Cell Biol. 20, 556–567. doi: 10.1016/j.tcb.2010.06.006
Pardo, A., Cabrera, S., Maldonado, M., Selman, M. (2016). Role of matrix metalloproteinase in the pathogenesis of idiopathic pulmonary fibrosis. Respir. Res. 17, 1–10. doi: 10.1186/s12931-016-0343-6
Park, C. S., Bang, B. R., Kwon, H. S., Moon, K. A., Kim, T. B., Lee, K. Y., et al. (2012). Metformin reduces airway inflammation and remodeling via activation of AMP-activated protein kinase. Biochem. Pharmacol. 84, 1660–1670. doi: 10.1016/j.bcp.2012.09.025
Peres, V., Nagem, T. J., Oliveira, F. F. D. (2000). Tetraoxygenated naturally occurring xanthones. Phytochem. 55, 683–710. doi: 10.1016/S0031-9422(00)00303-4
Rafii, R., Juarez, M. M., Albertson, T. E., Chan, A. L. (2013). A review of current and novel therapies for idiopathic pulmonary fibrosis. J. Thorac. Dis. 5, 48–73. doi: 10.3978/j.issn.2072-1439.2012.12.07
Raghu, G. (2011). Idiopathic pulmonary fibrosis: guidelines for diagnosis and clinical management have advanced from consensus-based in 2000 to evidence-based in 2011. Eur. Respir. J. 37, 743–746. doi: 10.1183/09031936.00017711
Raghu, G., Anstrom, K. J., King, T. E., Lasky, J. A., Martinez, F. J. (2012). Prednisone, azathioprine, and N-acetylcysteine for pulmonary fibrosis. N. Engl. J. Med. 366, 1968–1977. doi:10.1056/NEJMoa1113354
Raghu, G., Rochwerg, B., Zhang, Y., Garcia, C. A. C., Azuma, A., Behr, J., et al. (2015). An official ATS/ERS/JRS/ALAT clinical practice guideline: treatment of idiopathic pulmonary fibrosis an update of the 2011 clinical practice guideline. Am. J. Resp. Crit. Care 192, 3–19. doi: 10.1164/rccm.1925erratum
Sato, N., Takasaka, N., Yoshida, M., Tsubouchi, K., Kuwano, K., et al. (2016). Metformin attenuates lung fibrosis development via NOX4 suppression. Resp. Res. 17, 107. doi: 10.1186/s12931-016-0420-x
Sunad, R., Bone, N. B., Zmijewska, A. A., Shaoning, J., Won, P. D., Karen, B., et al. (2018). Metformin reverses established lung fibrosis in a bleomycin model. Nat. Med. 24, 1121–1127. doi: 10.1038/s41591-018-0087-6
Szapiel, S. V., Elson, N. A., Fulmer, J. D., Hunninghake, G. W., Crystal, R. G. (1979). Bleomycin-induced interstitial pulmonary disease in the nude, athymic mouse. Am. Rev. Respir. Dis. 120, 893–899. doi: 10.1164/arrd.1979.120.4.893
Tao, L., Yang, J., Cao, F., Xie, H., Zhang, M., Gong, Y., et al. (2017). Mogroside IIIE, a novel anti-fibrotic compound, reduces pulmonary fibrosis through Toll like receptor 4 pathways. J. Pharmacol. Exp. Ther. 361, 268–279. doi: 10.1124/jpet.116.239137
Todd, N. W., Luzina, I. G., Atamas, S. P. (2012). Molecular and cellular mechanisms of pulmonary fibrosis. Fibrogenes Tissue Repair 5: 11. doi: 10.1186/1755-1536-5-11
Williams, P., Ongsakul, M., Proudfoot, J., Croft, K., Beilin, L. (1995). Mangostin inhibits the oxidative modification of human low density lipoprotein. Free Rad. Res. 23, 175–184. doi: 10.3109/10715769509064030
Wynn, T. A. (2007). Common and unique mechanisms regulate fibrosis in various fibroproliferative diseases. J. Clin. Invest. 117, 524–529. doi: 10.1172/JCI31487
Wynn, T. A. (2011). Integrating mechanisms of pulmonary fibrosis. J. Exp. Med. 208, 1339–1350. doi: 10.1084/jem.20110551
Xia, Y., Xia, Y. F., Lv, Q., Yue, M. F., Qiao, S. M., Yang, Y., et al. (2016). Madecassoside ameliorates bleomycin-induced pulmonary fibrosis in mice through promoting the generation of hepatocyte growth factor via PPAR-gamma in colon. Brit. J. Pharmacol. 173, 1219–1235. doi: 10.1111/bph.13421
Yang, X., Wu, L., Li, G., Ran, Q., Zhang, L. (2017). Alphacalcidol combined with dexamethasone for reducing pulmonary fibrosis in mice and its mechanism. Chin. J. Cell Mol. Immunol. 33, 488–491.
You, B. H., Chae, H. S., Song, J., Ko, H. W., Chin, Y. W., Choi, Y. H. (2017). α-mangostin ameliorates dextran sulfate sodium-induced colitis through inhibition of nf-κb and mapk pathways. Int. Immunopharmacol. 49, 212–221. doi: 10.1016/j.intimp.2017.05.040
You, X., Xue, Q., Fang, Y., Liu, Q., Zhang, C. F., Zhao, C., et al. (2015). Preventive effects of EcliptaeHerba extract and its component, ecliptasaponin A, on bleomycin-induced pulmonary fibrosis in mice. J. Ethnopharmacol. 175, 172–180. doi: 10.1016/j.jep.2015.08.034
Zhang, C., Yu, G., Shen, Y. (2017). The naturally occurring xanthone α-mangostin induces ROS-mediated cytotoxicity in non-small scale lung cancer cells. Saudi J. Biol. Sci. 6, 1090–1095. doi: 10.1016/j.sjbs.2017.03.005
Zhao, J., Miyamoto, S., You, Y. H., Sharma, K. (2014). AMP-activated protein kinase (AMPK) activation inhibits nuclear translocation of Smad4 in mesangial cells and diabetic kidneys. Am. J. Physiol. Renal Physiol. 308, 1167–1177. doi: 10.1152/ajprenal.00234.2014
Keywords: alpha-mangostin, pulmonary fibrosis, myofibroblast differentiation, AMPK activation, NOX4
Citation: Li R-s, Xu G-h, Cao J, Liu B, Xie H-f, Ishii Y and Zhang C-f (2019) Alpha-Mangostin Ameliorates Bleomycin-Induced Pulmonary Fibrosis in Mice Partly Through Activating Adenosine 5′-Monophosphate-Activated Protein Kinase. Front. Pharmacol. 10:1305. doi: 10.3389/fphar.2019.01305
Received: 22 June 2019; Accepted: 15 October 2019;
Published: 13 November 2019.
Edited by:
Andres Trostchansky, University of the Republic, UruguayReviewed by:
Chiara Falciani, University of Siena, ItalyCopyright © 2019 Li, Xu, Cao, Liu, Xie, Ishii and Zhang. This is an open-access article distributed under the terms of the Creative Commons Attribution License (CC BY). The use, distribution or reproduction in other forums is permitted, provided the original author(s) and the copyright owner(s) are credited and that the original publication in this journal is cited, in accordance with accepted academic practice. No use, distribution or reproduction is permitted which does not comply with these terms.
*Correspondence: Chao-feng Zhang, emhhbmdjaGFvZmVuZ0BjcHUuZWR1LmNu
†These authors share first authorship
Disclaimer: All claims expressed in this article are solely those of the authors and do not necessarily represent those of their affiliated organizations, or those of the publisher, the editors and the reviewers. Any product that may be evaluated in this article or claim that may be made by its manufacturer is not guaranteed or endorsed by the publisher.
Research integrity at Frontiers
Learn more about the work of our research integrity team to safeguard the quality of each article we publish.