- 1Department of Otorhinolaryngology–Head and Neck Surgery, Korea University Guro Hospital, Seoul, South Korea
- 2Institute for Medical Device Clinical Trials, Korea University College of Medicine, Seoul, South Korea
- 3College of Pharmacy and Integrated Research Institute for Drug Development, Dongguk University, Seoul, South Korea
Polyphenol ε-viniferin (2) is a protective phytochemical found in several plant families. Here, we report a simple and effective method for the synthesis of (±)-ε-viniferin (2) as major product and (±)-(E)-ω-viniferin (3) as a minor product. Synthesized viniferin compounds and standard viniferin were analyzed for antibacterial and antibiofilm activity against Gram-positive bacteria Streptococcus pneumoniae. The minimum inhibitory concentrations (MICs) of (±)-ε-viniferin (2) and standard viniferin were 20 µm. However, the MICs of (±)-(E)-ω-viniferin (3) and compound 8 were 40 µm. Although viniferin significantly (p < 0.05) reduced pre-established in vitro biofilms and killed bacteria within the biofilm, it was unable to prevent biofilm formation at sub-MIC concentrations. The time kill experiment revealed that viniferin killed bacteria and reduced 2.8 log10 bacteria at 2 × MIC concentration after 24 h. Scanning electron microscope (SEM) analysis and live/dead biofilm staining of pre-established biofilms revealed that viniferin treatment disrupts membrane integrity of biofilm bacteria. Crystal violet absorption, total protein, and DNA and RNA release revealed that viniferin alters bacterial cell permeability, eventually killing bacteria.
Introduction
Streptococcus pneumoniae (S. pneumoniae) asymptotically colonizes the nosocomial cavity, causing infections under immune-compressed conditions. Pneumococci initially colonize nasopharyngeal mucosa in the form of biofilms (Bogaert et al., 2004; Simell et al., 2012). Biofilms in the nasopharyngeal cavity serve as reservoirs of bacteria that transit to sterile sites, causing infections such as otitis media (OM) pneumonia, bacteremia, meningitis, and sepsis (Wardlaw et al., 2006; Bergenfelz and Hakansson, 2017). Pneumococcal biofilms have been detected in infected sites such as tympanostomy tubes, human mucosa biopsies, and resected adenoids (Hall-Stoodley et al., 2006; Hoa et al., 2009). S. pneumoniae biofilms were also detected in the middle ear and nasopharynx of experimentally infected cinchona and mice (Reid et al., 2009; Sanchez et al., 2010). Bacteria in the biofilms were found in a self-produced matrix consisting of an extracellular polymeric substance (EPS) that confers resistance against conventional antibiotics and host immune defense (Donlan and Costerton, 2002). In mature biofilms, the EPS matrix resists antibiotic penetration via: i) charged polymers that prevent drug diffusion or ii) restriction of antimicrobial agents to the surface, leaving deeper cells unaffected, causing bacteria persistence (Marsh, 2004). Also, compared to planktonic bacteria, biofilm bacteria exhibit differential gene expression by adopting metabolic pathways less sensitive to the quinolone class of antibiotics and β-lactams, which target macromolecules or metabolic pathways (Xie et al., 2005; Mascio et al., 2007; Yadav et al., 2012). Antimicrobials inhibiting normal cells are made ineffective due to bacteria in biofilms growing slowly under nutrient- and aeration-depleted conditions (Davies, 2003). Resistance against commonly used antibiotics hampers treatment options (Korona-Glowniak et al., 2018). Consequently, new safe antimicrobials or antibiofilm agents that limit emergence of antibiotic-resistant bacteria are needed (Hurdle et al., 2011). Reportedly, novel phyto-compounds display potential to mitigate bacterial growth, including both pneumococcal planktonic and biofilm growth (Kwieciński et al., 2009; Ma et al., 2012; Quave et al., 2012; Burt et al., 2014; Yadav et al., 2015; Ma et al., 2018).
Natural polyphenols exhibit a range of biological activities (Lee et al., 2014). Stilbenes, known for antioxidant, anti-fungal and antimicrobial activities, are natural products used in chemotherapy (Shimizu et al., 2000). The antimicrobial activity and mechanism of stilbene monomer, trans-resveratrol (1), was investigated (Hwang and Lim, 2015). Oligostilbenes (Figure 1), produced via oxidative oligomerization of phenols, display various biological activities (Sotheeswaran and Pasupathy, 1993; Cichewicz and Kouzi, 2002). A dimeric product of trans-resveratrol (1), ε-viniferin (2), found in many plant families including Vitaceae, grapvines (Vitis), and Carex, exhibits P450 inhibitory antioxidants, as well as hepato-protective and antimicrobial activities (Fiorentino et al., 2008; Santamaria et al., 2012). Naturally occurring ε-viniferin, in Carex pumila and C. lactiflora extracts, displays antibiofilm activities against Gram-negative bacteria such as Escherichia (E. coli) (Lee et al., 2014). However, antimicrobial and antibiofilm activities of synthetic ε-viniferin and its derivatives against Gram-positive bacteria remain unknown.
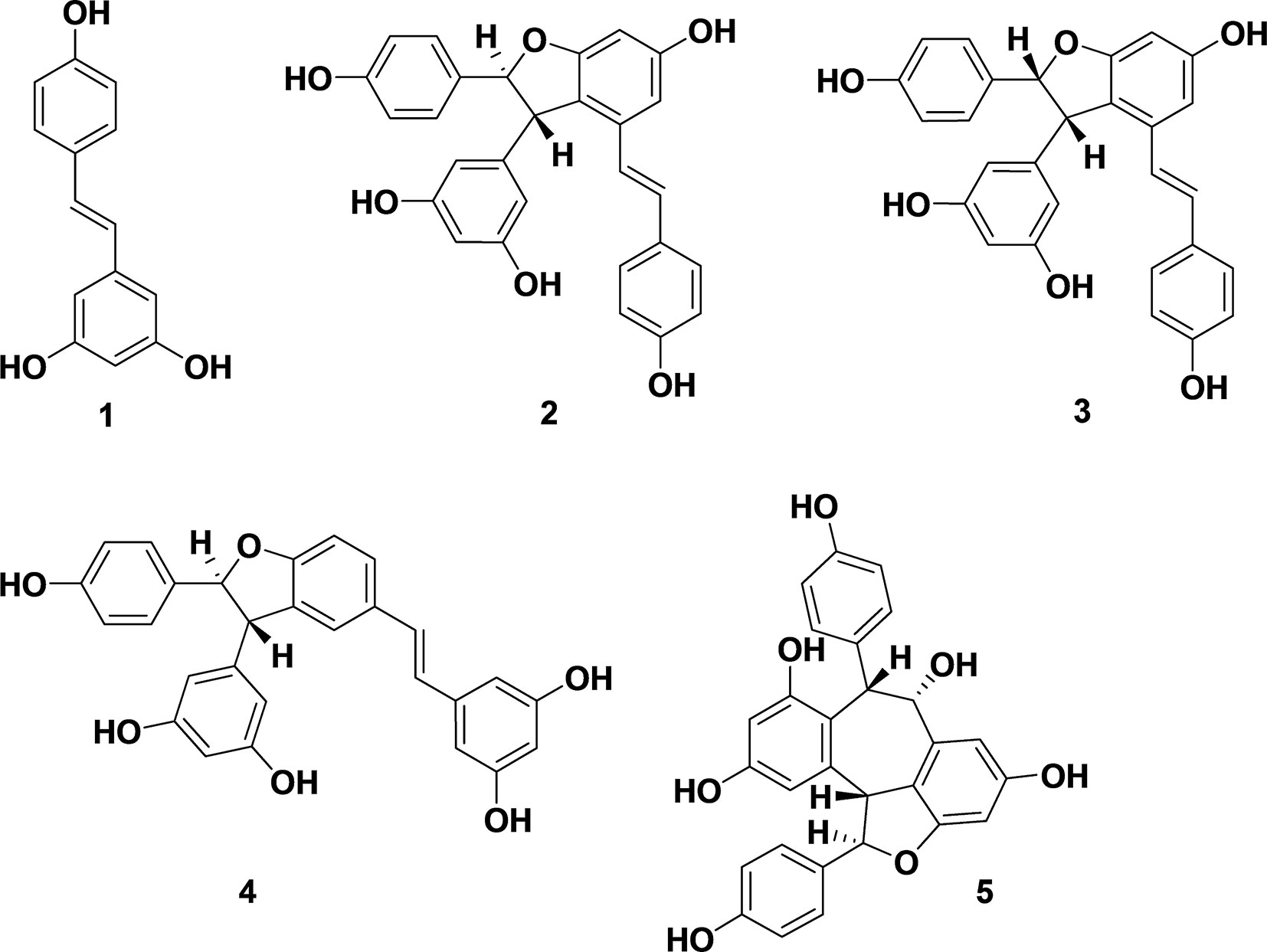
Figure 1 Biologically active stilbenes: resveratrol (1), ε-viniferin (2), (E)-ω-viniferin (3), δ-viniferin (4), and balanocarpol (5).
Few reports are available on the synthesis of ε-viniferin. However, related natural products have been synthesized. Oxidative cyclization of resveratrol using FeCl3·6H2O and Ti (NO3)3 in methanol has been reported (Yao et al., 2004; Takaya et al., 2005). Although a method for total synthesis of ε-viniferin has been reported, it only yields low amounts of viniferin and requires more steps (Lindgen et al., 2016). To overcome these limitations, we synthesized ε-viniferin (2) and (E)-ω-viniferin (3) as racemates using ruthenium chloride–induced oxidative cyclization of trans-resveratrol. Compounds 8 and 9 were also synthesized from ε-viniferin (2). This novel method is economical and improves yields for large-scale synthesis. Synthetic viniferins were evaluated for antimicrobial and antibiofilm activity against S. pneumoniae planktonic and biofilm states.
Materials and Methods
Synthesis of Viniferins
All chemicals were purchased commercially and used without further purification. Flash column chromatography enabled product purification, and thin layer chromatography (TLC) analysis was conducted on commercial plates coated with silica gel 60 F254. Visualization of spots on TLC plates was achieved via ultraviolet (UV) radiation. Mass spectra were recorded using high-resolution mass spectrometry (HRMS, electrospray ionization MS) obtained on a G2 quadrupole time-of-flight (QTOF) mass spectrometer. Proton nuclear magnetic resonance (1H NMR) spectra were determined on a Varian (400 MHz) spectrometer (Varian Medical Systems, Inc., Palo Alto, CA, USA). 13C NMR spectra were recorded on a Varian (100 MHz) spectrometer using tetramethylsilane (TMS) as an internal standard. Chemical shifts are provided in parts per million (ppm) downfield with coupling constants in hertz (Hz). Standard abbreviations s, d, t, and m refer to singlet, doublet, triplet, and multiplet, respectively. Infrared (IR) spectra were recorded on FT-IR (NICOLET-iS5). Final product purity was checked by reversed-phase high-pressure liquid chromatography (RP-HPLC) performed on the YL9100 YoungLin HPLC system equipped with an UV detector set at 280 and 254 nm. Mobile phases used were: (A) H2O containing 0.1% trifluoroacetic acid and (B) acetonitrile containing 0.1% trifluoroacetic acid. HPLC chromatogram for purity check was: 5–55% B for 0–12 min, 55–100% B for 12–15 min, 100% B for 15–19 min, 100–5% B for 19–22 min, and 5% B for 22–23 min. HPLC employed an Agilent Eclipse Plus C18 reverse-phase column (3.5 μm particle size) that was 4.6 in diameter × 100 mm in size with a flow rate of 1.0 ml/min. Starter chemicals such as resveratrol and RuCl3.H2O were purchased from Sigma Aldrich, TCI, Alfa-Aesar, and Combi Blocks. The purity of all biologically evaluated compounds was >95% (per HPLC).
Experimental Procedure
1. (±)-ε-Viniferin Penta-Acetate (6) and (±)-(E)-ω-Viniferin Penta-Acetate (7)
Ruthenium (III) chloride (1.09 g, 5.26 mmol) was added to a stirred solution of resveratrol (1.0 g, 4.38 mmol) in methanol/water (10:1, 11 ml) at 0°C. The reaction mass was stirred at 35°C for 3 h. After removing methanol in vacuum, the crude residue was dissolved in ethyl acetate (200 ml) and washed with sat. brine solution. The organic layer was dried over anhydrous MgSO4, filtered, and evaporated. The crude residue was purified via silica gel column chromatography (0–20% acetone in methylene chloride) to obtain an ε- and ω-viniferin–enriched product (340 mg) and unreacted resveratrol (350 mg, 1.53 mmol). The ε- and ω-viniferin–enriched products were dissolved in dichloromethane (20 ml) and dimethyl sulfoxide (DMSO) (1 ml). Triethylamine (TMS) (2.8 ml, 17.8 mmol) and Ac2O (1.36 ml, 14.4 mmol) were added at 0 °C and stirred at room temperature (RT) for 5 h. The reaction mixture was diluted with methylene chloride (100 ml) and washed using sat. NaHCO3. The organic layer was washed with sat. brine, dried over MgSO4, filtered, and evaporated. The crude product was purified via silica gel column chromatography (15–25% ethyl acetate in hexane) to afford ε-viniferin penta-acetate (6) (0.18 g, 18%) as a white solid and ω-viniferin penta-acetate (7) (75 mg, 7.5%) as a white solid following 2 steps.
Analytical data of ε-viniferin penta-acetate (6): 1H NMR (400 MHz, CDCl3) δ (ppm) 7.3 (d, J = 8.8 Hz, 2 H), 7.18 (d, J = 8.4 Hz, 2 H), 7.1 (d, J = 8.4 Hz, 2 H), 6.99 (d, J = 8.4 Hz, 2 H), 6.94 (s, 1 H), 6.9 (s, 1 H), 6.89 (d, J = 16.8 Hz, 1 H), 6.85 (s, 2 H), 6.64 (s, 1 H), 6.55 (d, J = 16 Hz, 1 H), 5.6 (d, J = 6.8 Hz, 1 H), 4.6 (d, J = 6.4 Hz, 1 H), 2.33 (s, 3 H), 2.29 (s, 3 H), 2.26 (bs, 9 H).
Analytical data of ω-viniferin penta-acetate (7): 1H NMR (400 MHz, CDCl3) δ (ppm) 7.27 (d, J = 8.4 Hz, 2 H), 7.12 (d, J = 8.4 Hz, 2 H), 7.0 (d, J = 8.4 Hz, 2 H), 6.96 (d, J = 2.0 Hz, 1 H), 6.94 (d, J = 15.6 Hz, 1 H), 6.92 (d, J = 9.2 Hz, 2 H), 6.7 (d, J = 2.0 Hz, 1 H), 6.68 (d, J = 16.4 Hz, 1 H), 6.63 (t, J = 1.8 Hz, 1 H), 6.37 (d, J = 1.6 Hz, 2 H), 6.0 (d, J = 8.8 Hz, 1 H), 4.82 (d, J = 8.8 Hz, 1 H), 2.35 (s, 3 H), 2.27 (s, 3 H), 2.23 (s, 3 H), 2.18 (s, 6 H).
2. (±)-ε-Viniferin (2)
KOH (25 mg, 0.44 mmol) was added to a stirred solution of 6 (50 mg, 0.075 mmol) in methanol (6 ml), at RT. Reaction mass was stirred for 30 min. After removing methanol in a vacuum, crude residue was dissolved in ethyl acetate (25 ml) and 1N HCl (10 ml). The organic layer was dried over anhydrous MgSO4, filtered, and evaporated. Crude residue was purified via recrystallization (acetone: methylene chloride), producing (±)-ε-viniferin (2) (30 mg, 88%) as a pale yellow-colored solid. 1H NMR (400 MHz, CD3OD) δ (ppm) 7.14 (d, J = 8.8 Hz, 2 H), 7.04 (d, J = 8 Hz, 2 H), 6.83 (d, J = 16.4 Hz, 1 H), 6.75 (d, J = 8.4 Hz, 2 H), 6.65 (d, J = 8.4 Hz, 2 H), 6.62 (d, J = 1.6 Hz, 1 H), 6.58 (d, J = 16.4 Hz, 1 H), 6.24 (d, J = 1.6 Hz, 1 H), 6.17 (d, J = 2 Hz, 1 H), 6.16 (bs, 2 H), 5.37 (d, J = 6.8 Hz, 1 H), 4.35 (d, J = 6.8 Hz, 1 H); 13C NMR (100 MHz, CD3OD) δ (ppm) 161.3, 158.3–156.9, 145.9, 135.5, 132.4, 128.9, 127.3, 126.7, 122.2, 118.6, 114.9, 106, 102.9, 100.7, 95.4, 93.4, 56.8; IR (cm-1): 3311, 1590, 1440, 1237, 1148, 998, 824 HRMS (ESI) m/z calcd for C28H23O6 [M+H]+: 455.1495, found: 455.1487; purity 95.4% (as determined by RP-HPLC, tR = 11.05 min).
3. (±)-E-ω-Viniferin (3)
Compound 3 was obtained as a brown-colored solid (18 mg, 75%) from compound 7 (30 mg), according to the procedure for compound 2. 1H NMR (400 MHz, CD3OD) δ (ppm) 7.13 (d, J = 8.4 Hz, 2 H), 6.97 (d, J = 8.8 Hz, 2 H), 6.88 (d, J = 16.4 Hz, 1 H), 6.67 (d, J = 8.4 Hz, 2 H), 6.67 (d, J = 16.4 Hz, 1 H), 6.63 (d, J = 2.0 Hz, 1 H), 6.56 (d, J = 8.8 Hz, 2 H), 6.3 (d, J = 1.2 Hz, 1 H), 5.92 (t, J = 2.4 Hz, 1 H), 5.84 (d, J = 8.4 Hz, 1 H), 5.75 (bs, 2 H), 4.6 (d, J = 8.4 Hz, 1 H); 13C NMR (100 MHz, CD3OD) δ (ppm) 161.2, 157.9, 157.3, 156.9, 156, 142.3, 135.4, 129.4, 128.9, 128.2, 127.8, 127.3, 122.5, 120.9, 115, 113.8, 107.7, 103.7, 100.2, 95.7, 89.4, 52.1; IR (cm-1): 3311, 1621, 1512, 1442, 1226, 1120, 992; HRMS (ESI) m/z calcd for C28H23O6 [M+H]+: 455.1495, found: 455.1495; purity 96.2% (as determined by RP-HPLC, tR = 11.4 min).
4. Compound (8)
Pd/C (10%, 4.4 mg) was added to a stirred solution of 2 (22 mg, 0.048 mmol) in ethanol (1 ml), in a flask sealed with a hydrogen balloon. The suspension was stirred for 3 h at RT. The resulting suspension was filtered through a pad of celite, and the filtrate was evaporated. The crude residue was purified via silica gel column chromatogra (ppm) phy (0–5% MeOH in methylene chloride) to afford 8 (8.4 mg, 38%) as a brown-colored solid. 1H NMR (400 MHz, CD3OD) δ (ppm) 7.03 (d, J = 8.4 Hz, 2 H), 6.74 (d, J = 8.4 Hz, 2 H), 6.69 (d, J = 8.4 Hz, 2 H), 6.57 (d, J = 8 Hz, 2 H), 6.17 (s, 1 H), 6.15 (bs, 2 H), 6.06 (bs, 2 H), 5.23 (d, J = 6.4 Hz, 1 H), 3.95 (d, J = 6.4 Hz, 1 H), 2.47–2.38 (bm, 4 H); 13C NMR (100 MHz, CD3OD) δ (ppm) 160.8, 158.5, 158.1, 157, 154.9, 146.1, 139.9, 132.4, 129, 126.9, 119.2, 114.8, 114.4, 108.1, 106, 100.6, 94.1, 93.4, 56.5, 35.6, 35; IR (cm-1): 3261, 1616, 1440, 1224, 1117, 1000; HRMS (ESI) m/z calcd for C28H25O6 [M+H]+: 457.1651, found: 457.1758; purity 100% (as determined by RP-HPLC, tR = 10.78 min).
5. Compound (9)
K2CO3 (92 mg, 0.06 mmol) and MeI (0.31 g, 2.2 mmol) were added to a stirred solution of compound 2 (100 mg, 0.22 mmol) in acetone (5 ml). The reaction suspension was slowly heated to 65°C and maintained overnight. The resulting suspension was filtered through a celite pad and the filtrate evaporated. The residue was dissolved in ethyl acetate (50 ml), washed with water, sat. brine, dried over MgSO4, filtered, and evaporated. The crude product was purified by silica gel column chromatography (hexane/ethyl acetate = 8:2, v/v) to afford 9 (20 mg, 17%) as an off-white solid. 1H NMR (400 MHz, CDCl3) δ (ppm) 7.27 (d, J = 8.4 Hz, 2 H), 7.13 (d, J = 8.8 Hz, 2 H), 6.88 (d, J = 16.8 Hz, 1 H), 6.86 (d, J = 8.8 Hz, 2 H), 6.79 (d, J = 8.8 Hz, 2 H), 6.72 (d, J = 2 Hz, 1 H), 6.61 (d, J = 16.8 Hz, 1 H), 6.46 (d, J = 2.4 Hz, 1 H), 6.36 (bs, 3 H), 5.52 (d, J = 5.6 Hz, 1 H), 4.51 (d, J = 5.6 Hz, 1 H), 3.86 (s, 3 H), 3.79 (s, 3 H), 3.77 (s, 3 H), 3.73 (bs, 6 H); 13C NMR (100 MHz, CDCl3) δ (ppm) 161.3–161.1, 159.4, 159.2, 145.6, 135.3, 133.6, 129.8, 129.3, 127.6, 126.9, 123.1, 119.5, 114, 105.8, 102.3, 98.8, 95, 93, 56.9, 55.5–55.2; HRMS (ESI) m/z calcd. for C33H33O6 [M+H]+: 525.2277, found: 525.2383; purity 100% (as determined by RP-HPLC, tR = 17.7 min).
Bacteria Strain and Culture Medium
S. pneumonia [National Collection of Type Cultures (NCTC) 7466] was purchased from the Health Protection Agency Culture Collection (Salisbury, UK). S. pneumoniae National Collection of Type Cultures (NCTC) 7466 is Avery’s virulent strain D39, serotype 2, encapsulated strain (Avery et al., 1944). S. pneumoniae CCARM 4003, an antibiotic resistance strain (clindamycin >128, erythromycin >512, tetracycline 16 µg/mL), was purchased from the Culture Collection of Antimicrobial Resistance Microorganisms (CCARM 4003, Seoul, South Korea). S. pneumoniae strain 11 (strain 7101975) was obtained from the infectious disease center, Korea University College of Medicine, Seoul; serotype 3 (ATCC 6303), 19F (ATCC 49619) and 19A, and R6 were purchased from the American Type Culture Collection (Manassas, VA, USA) (Yadav et al., 2017). Bacteria were grown on an agar plate supplemented with 5% defibrinated sheep blood agar plates (BAPs) purchased from Shin Yang Chemicals Co., Ltd., Seoul, Korea and in brain heart infusion (BHI) broth.
Minimum Inhibitory Concentration (MIC) Detection
MICs were determined using the broth microdilution method recommended by the Clinical and Laboratory Standards Institute (CLSI, 2005). S. pneumoniae D39 colonies grown on BAP were scraped and grown in MH broth until log phase. Log-phase cells were diluted to prepare bacterial suspensions containing 1–5 × 105 colony-forming units (cfu)/ml. Viniferin solutions were added to Mueller Hinton Broth (MHB) cell suspension from 10 to 50 µm concentrations. Next, 200 µl of cell suspension along with viniferin solutions were inoculated in each well of a 96-well plate. Negative control samples were supplied with DMSO. Plates were incubated for 18 h at 37°C. Bacterial growth was detected by measuring optical density at 600 nm using a microplate reader. MIC was defined as the lowest concentration of viniferin solution at which no visible bacterial growth was observed. Alternately, MICs were confirmed by determining bacterial cfu counts. Cell suspensions were diluted two-fold, plated on BAP agar plates, and incubated overnight at 37°C, and cfu were counted. The experiment was repeated twice in triplicate. The inhibitory effect of 20 µm viniferin on strains resistant to erythromycin (> 512 µg/ml) and different serotypes (2, 3, 19A, 19F) of S. pneumoniae was evaluated.
Effect of Viniferin on Biofilm Inhibition
The effect of ε-viniferin (2) on S. pneumoniae biofilm growth was evaluated in vitro using static biofilm models, and biofilm biomass was quantified using crystal violet (CV) microtiter plate assays (Christensen et al., 1985; Yadav et al., 2017). Bacteria grown on blood agar plates were scraped and grown to log phase in BHI broth. Freshly prepared bacterial cell suspensions were diluted 1:200 and inoculated at 200 µl in 96-well plates or at 1 ml in 24-well plates. Viniferin solution was added to each well at concentrations from 10 to 50 µm. The control samples (vehicle) were treated with similar volumes of DMSO (0.1 to 0.5%). Plates were incubated at 37°C for 18 h. Following incubation, the medium and planktonic cells were decanted and the plate washed twice with phosphate-buffered saline (PBS). Biofilms at the bottom of the plates were stained using 50 µl of 0.1% CV for 15 min. The biofilms were washed twice with PBS and air-dried, and CV was dissolved in ethanol (200 µl ethanol for 96-well or 1 ml for 24-well plate). Absorbance of the dissolved CV was measured at 570 nm using microplate readers. To evaluate the effects of viniferin on planktonic growth, S. pneumoniae D39 were grown in different concentrations of synthetic ε-viniferin (2) (MIC, 0.5 × MIC, and 0.25 × MIC). Growth was detected by measuring absorbance at 600 nm at different time intervals.
Effect of Viniferin on Eradication of Pre-Established Biofilms
Microbial biofilms are difficult to eradicate, and several-fold-high concentrations of antibiotics are needed to eradicate pre-established biofilms (Høiby et al., 2010; Co et al., 2018). We evaluated in vitro biofilm eradication potential of viniferin. In vitro biofilms of S. pneumoniae were grown in 24-well plates for 18 h via procedures described above. These biofilms were treated with MIC and 2 × MIC concentrations of viniferin for 6 h. Biofilm biomasses were quantified using CV microtiter plate assays, and viable bacteria were detected by cfu counts as described above. Daptomycin, a lipopeptide antibiotic known to eradicate biofilm, was used as a positive control.
Metabolically active bacteria within pre-established biofilms were detected using resazurin staining. Resazurin is a blue-colored, non-fluorescent dye, and in the presence of the metabolically active cells, it gets reduced to a pink and highly fluorescent compound called resorufin. The fluorescent detection allows the quantitative measurement of cell viability. Resazurin staining was performed as per previously reported procedure with minor modification (Paytubi et al., 2017). 0.02% (w/v) resazurin sodium salt (Sigma, USA) solution was prepared in sterile distilled water and filter-sterilized. Pre-established biofilms grown in 24-well plates and treated with viniferin as mentioned above were dissolved in sterile water, and 100 μl was transferred to a 96-well plate. The biofilm suspensions were incubated with 25 μl (0.02%) resazurin dye and 100 μl BHI broth. The plate was incubated at 37°C for 2 h, and fluorescence was measured (excitation 530 nm, emission 590 nm) using a microplate reader (Thermo Scientific, Waltham, MA, USA).
SEM Analysis of Pre-Established Biofilms
S. pneumoniae form robust biofilms of significant depth in vitro (Moscoso et al., 2006). We evaluated changes in S. pneumoniae in vitro biofilm morphology upon viniferin treatment using SEM. Synthetic ε-viniferin (2) was the most active; therefore, experiments were performed with ε-viniferin (2). In vitro biofilms were grown in 24-well plates for 18 h via procedures described above and treated with 2 × MIC concentration of ε-viniferin (2) for 6 h. Control biofilms were treated with DMSO. Biofilms were washed with PBS pre-fixed by 2% glutaraldehyde + formaldehyde solution and post fixed with osmic acid (2%) for 2 h. The biofilms were dehydrated via increasing ethanol concentration gradients (60–95%) followed by t-butyl alcohol treatment. The samples were freeze-dried and coated with platinum. Images were captured using field emission SEM (FE-SEM; Hitachi, S-4700, Tokyo, Japan).
Confocal Microscopy Analysis of Pre-Established Biofilms
Alteration of pre-established biofilm structure and viability upon viniferin treatment was analyzed using live/dead biofilm staining and confocal microscopy. S. pneumoniae D39 biofilms were grown on micro-discs for 18 h via procedures described above, and treated with ε-viniferin (2) (2 × MIC) for 6 h. The biofilms were stained with a LIVE/DEAD biofilm viability kit (Invitrogen, California, USA) according to the manufacturer’s instructions. Control biofilms were treated with similar volumes of DMSO. After washing, stained biofilms were viewed under a Nikon A1 confocal microscope (Nikon Instruments Inc., NY, USA) using fluorescein (green) and Texas red (red) band-pass filter sets.
Effect of Viniferin on Bacteria Killing
To evaluate the bactericidal or bacteriostatic effect of viniferin on S. pneumoniae, a bacteria-killing experiment was performed. An S. pneumoniae D39 cell suspension was grown in BHI broth up to early log phase, and aliquots were treated with 2 × MIC concentrations of viniferin (compounds 2, 3, and 8, and ε-viniferin standard). Cells were further grown at 37°C, and viable bacteria were detected at different time points (0, 6, 12, and 24 h) via cfu counts as described above. Control samples were treated with DMSO.
Crystal Violet Absorption Assay
Alteration of bacterial membrane permeability upon viniferin treatment was evaluated using CV absorption assays (Devi et al., 2010). S. pneumoniae D39 colonies were grown in BHI broth up to early log phase. The cells were pelleted via centrifugation at 4,500g for 5 min at 4°C. Bacterial cells were washed with PBS and treated with ε-viniferin (2) and erythromycin (MIC and 2 × MIC) for 3 h at 37°C. Erythromycin was used as the control, as it had no effect on bacterial membrane permeability (non-dividing cells). Negative control samples were treated with DMSO. Following incubation, cells were harvested via centrifugation at 9300g for 5 min and suspended in 10 µl/ml CV solution (0.01%). The cell suspension was incubated for 10 min and centrifuged at 13,400g for 15 min. The OD of the supernatant was detected at 590 nm using a microplate reader. The OD value of the original solution was considered 100%, and the percentage of CV absorption was detected as follows:
Leakage of Total Proteins Through the Bacterial Membrane
Integrity of S. pneumoniae cell membrane upon viniferin treatment was examined by determining total protein release in supernatants. Protein concentrations in the supernatants were evaluated using the Pierce bicinchoninic acid (BCA) protein assay kit (Thermo Scientific, MA, USA). S. pneumoniae were grown to log phase, pelleted via centrifugation, and suspended in PBS. Cell suspensions (approx. 107/ml) were treated with viniferin (MIC and 2 × MIC) and incubated at 37°C for 3 h. Next, cells were pelleted, and supernatant was filtered through a 0.2 µm syringe filter. The cell-free supernatants were treated with 200 µl each of solutions A and B (provided in kit) for 30 min, and absorbance at 562 nm was measured.
Leakage of DNA and RNA Through the Bacterial Membrane
The integrity of the pneumococci’s cell membrane upon viniferin treatment was evaluated by monitoring release of cytoplasmic constituents such as DNA and RNA as per a previous report (Bouyahya et al., 2019). S. pneumoniae were grown up to mid-log phase in BHI medium. The cell suspensions were pelleted by centrifugation and dissolved in PBS. The cells were treated with viniferin (MIC and 2 × MIC) for 3 h at 37°C. After treatment, cells were pelleted by centrifugation (10,000g for 5 min), and supernatants were filter-sterilized using a 0.2 µm syringe filter. The released DNA and RNA in supernatants were determined by measuring optical density at 260 nm.
Statistical Analysis
Experiments were performed in replicate, and means were calculated and compared with those of the control group. Statistical significance was assessed using Student’s t-tests, and a P < 0.05 was considered significant.
Results
Synthesis of (±)-ε-Viniferin (2), (±)-(E)-ω-Viniferin (3), 8, and 9
In the current study, resveratrol (1) was used as a precursor for the transformation with oxidizing agents. Initially, synthesis of ε-viniferin by oxidative coupling of resveratrol with known procedures was unsuccessful. The usefulness of ruthenium (III) chloride hydrate in the formation of the 2,3-dihydrobenzofuran unit is unknown, but the reagent is widely used to catalyze oxidation (like sulfite to sulfate) (Gao and Sharpless, 1988). Resveratrol (1) was treated with RuCl3·H2O in MeOH/water (10:1) at 0°C to 35°C to yield crude (±)-ε-viniferin (2) as a major product and (±)-(E)-ω-viniferin (3) as a minor product along with unconverted 1. Simple column chromatography provided viniferin-enriched fractions. For purification purposes, crude column fractions were acetylated in methylene chloride (MC) to provide pure penta-acetate viniferins (6 and 7), and subsequent hydrolysis yielded 2 and 3, respectively. Compounds 8 and 9 were also synthesized via compound 2. Hydrogenation of (±)-ε-viniferin (2) with of Pd/C (10%) in EtOH produced 8, while penta-methylation of 2 with MeI/K2CO3 yielded compound 9. Analytical data of (±)-ε-viniferin (2) and (±)-(E)-ω-viniferin (3) (1H NMR and 13C NMR, HRMS) were in agreement with standard products (Ito et al., 1999; Mattivi et al., 2011).
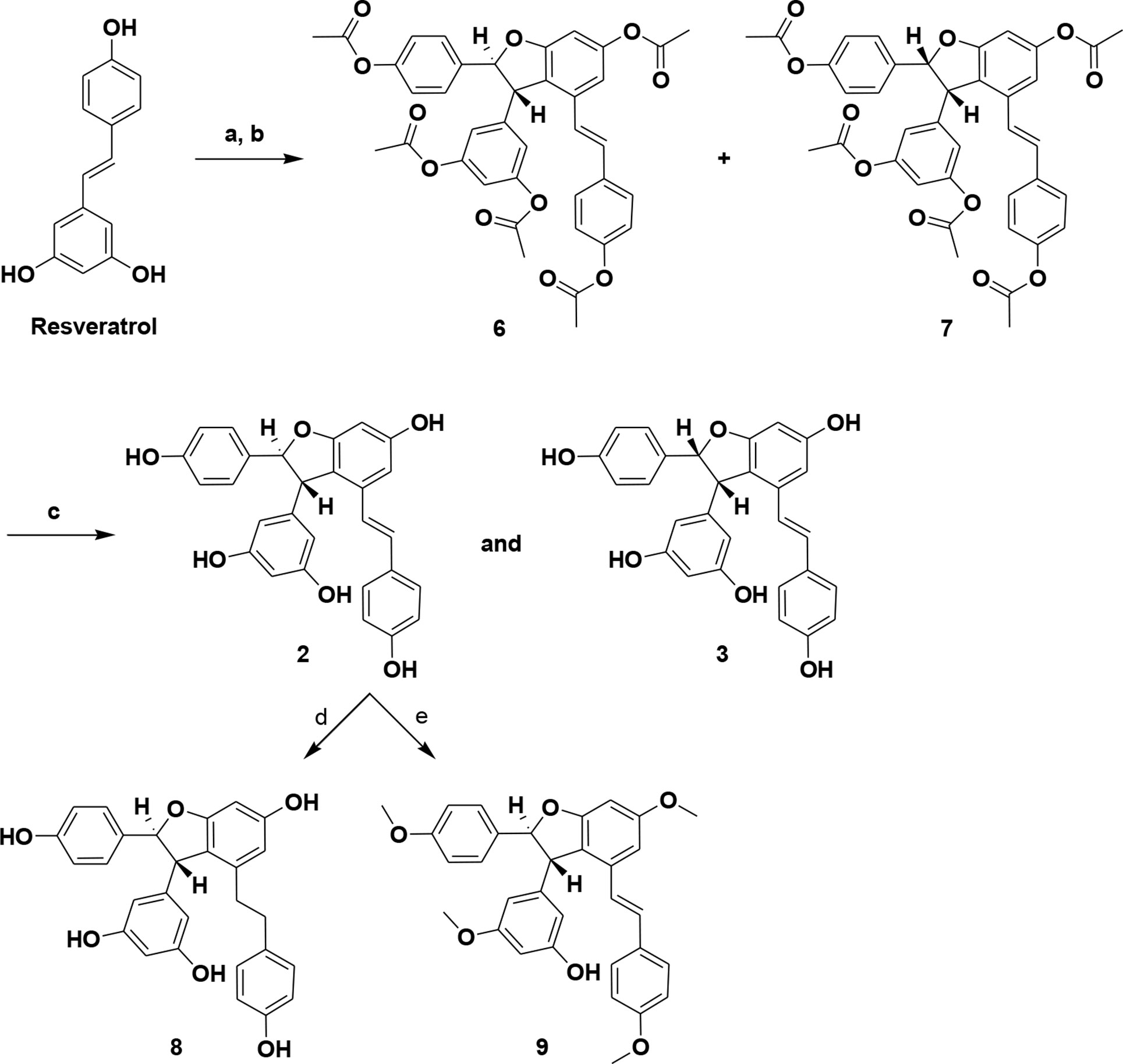
Scheme 1 Synthesis of (±)-ε-viniferin (2), (±)-(E)-ω-viniferin (3), 8, and 9. (a) RuCl3·H2O, MeOH, water, 0°C to 35°C, 3 h; (b) (Ac)2O, TEA, MC, DMSO, 0°C to RT, 5 h; (c) KOH, MeOH, RT; (d) 10% Pd/C, H2, EtOH, RT, 3 h; (e) MeI, K2CO3, acetone, 65°C, 6 h.
Minimum Inhibitory Concentration of Viniferin
The MIC of standard viniferin and synthetic (±)-ε-viniferin (2) for S. pneumoniae was 20 µm, while the MIC of (±)-E-ω-viniferin (3) and 8 was 40 µm. However, concentrations of penta-methylated viniferin (9) up to 50 µm were unable to inhibit S. pneumoniae growth (Figure 2A). The most active compound, (±)-ε-viniferin, was tested against different S. pneumoniae serotypes. Results demonstrated that (±)-ε-viniferin inhibited the growth of S. pneumonia serotypes 2, 3, 19A, and 19F and clinical strains at 20 µm (Figure 2B). Similarly, the erythromycin-resistant strain of S. pneumoniae and R6 (un-encapsulated) were also inhibited by 20 µm synthetic (±)-ε-viniferin (Figure 2B).
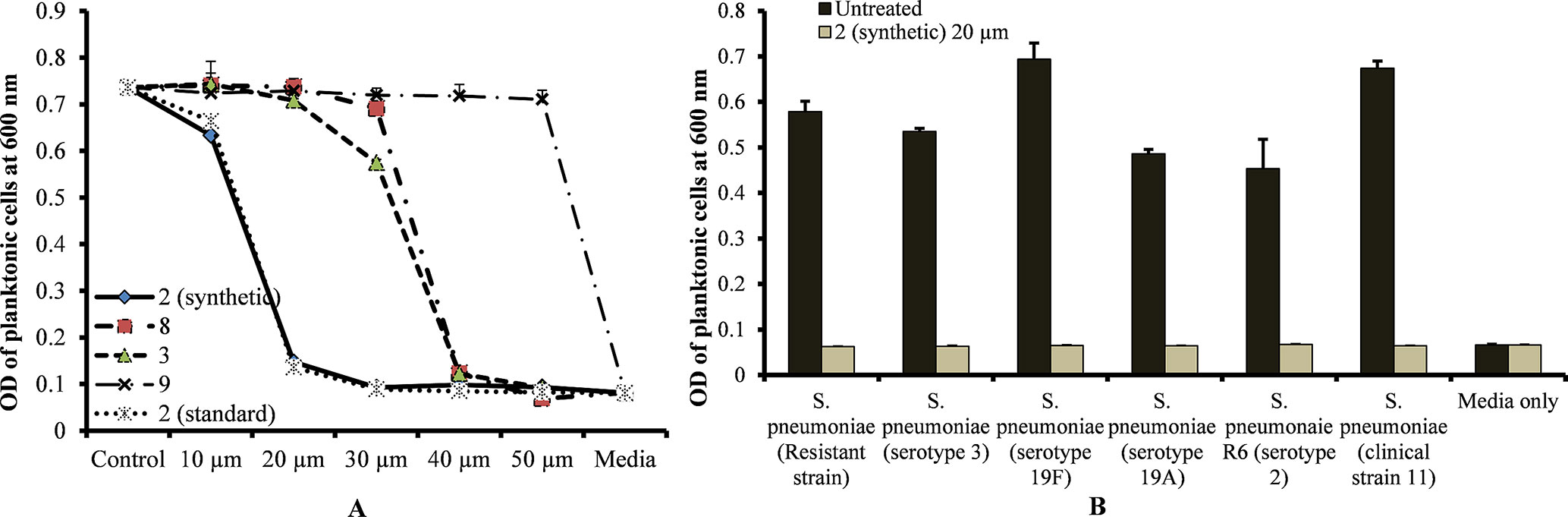
Figure 2 Detection of minimum inhibitory concentrations (MICs) of viniferin compounds against S. pneumoniae. (A) MIC detection of standard and synthetic viniferin compounds (2, 3, 8, and 9) tested from 10 to 50 µm against S. pneumoniae D39 (serotype 2). (B) Inhibitory effect of synthetic ε-viniferin (2) (20 µm) on S. pneumoniae antibiotic resistance strain and different serotypes. Bacterial growth in the presence of viniferin was measured via optical density at 600 nm after 18 h. The means were calculated. Error bars represents standard deviations.
Viniferin Is Not Effective on S. pneumoniae in Vitro Biofilms at Sub-MIC
Planktonic growth results showed no bacterial growth at MIC and slow bacterial growth at 0.25 × MIC (sub-sub-MIC) or 0.5 × MIC (sub-MIC) of ε-viniferin (2) (Figure 3A). No significant difference was detected in the planktonic growth of bacteria between ε-viniferin (2) treated with 0.25 × MIC or 0.5 × MIC and the control following 15 h incubation (Figure 3A). Growing S. pneumoniae in vitro biofilms in presence of 0.25 × MIC or 0.5 × MIC of viniferin did not induce significant inhibition of biofilm growth (Figure 3B). However, at the MIC of viniferins, no biofilm growth was detected, which may have been due to complete inhibition of planktonic cell growth, which prevented the cells from reaching the number required for biofilm buildup. These results suggest that viniferin was unable to impede in vitro biofilms of S. pneumoniae at sub-MIC but hindered bacterial growth in planktonic form and biofilms at MIC.
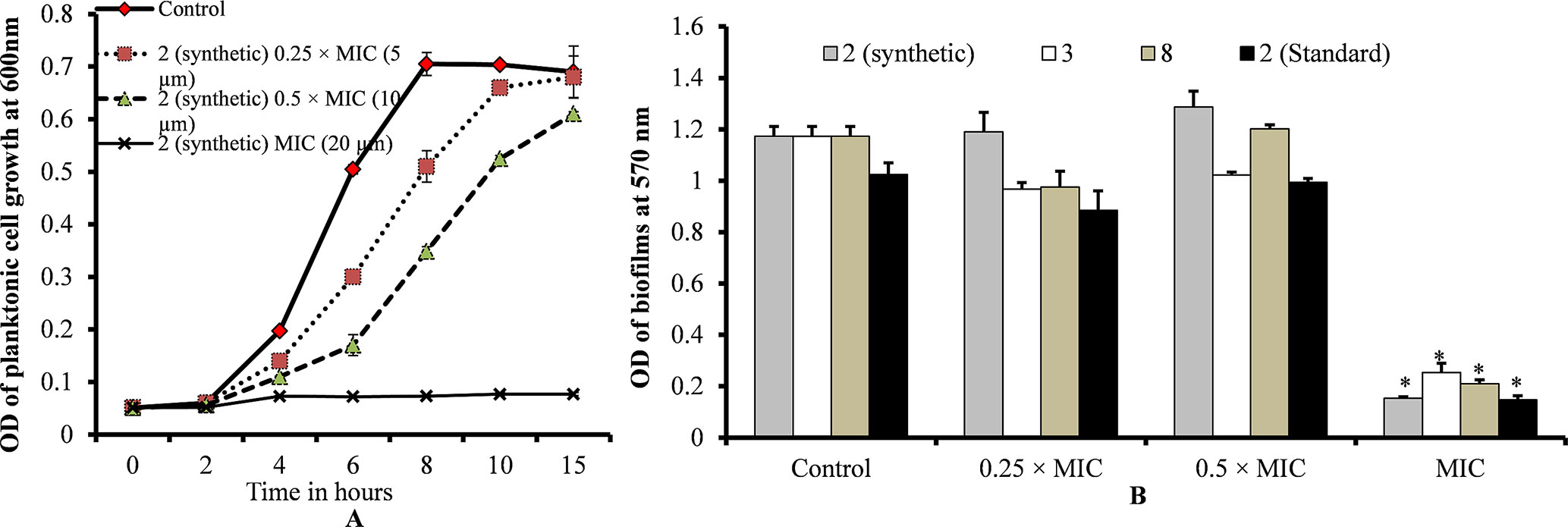
Figure 3 S. pneumoniae planktonic and in vitro biofilm growth in the presence of 0.25 × MIC or 0.5 × MIC or MIC of viniferins. (A) Planktonic growth was detected by measuring observance at 600 nm. (B) Biofilm biomass was detected via CV microplate assay. The error bars represent standard deviations. Statistical significance was calculated via Student’s t-test, and p < 0.05 was considered significant.
Viniferin Eradicated Pre-Established Biofilms
Treatment of pre-established biofilms with viniferin compounds resulted in a significantly (p < 0.05) reduced biofilm biomass. At MIC and 2 × MIC, synthetic compounds 2, 3, and 8 and standard viniferin actively eradicated pre-established biofilms (Figure 4A). Treating biofilms with daptomycin (positive control) also eradicated >50% biofilms. Treatment of pre-established biofilms with MIC and 2 × MIC of ε-viniferin (2) significantly (p < 0.05) reduced viable bacteria in biofilm (Figure 4B). The resazurin staining results showed that the ε-viniferin (2) treatment (MIC and 2 × MIC) significantly (p < 0.05) reduced the metabolically active bacteria within pre-established biofilms (Figure 4C). These results indicated that viniferins effectively decrease pre-established biofilms of pneumococci and that ε-viniferin (2) was able to kill pneumococci in biofilms.
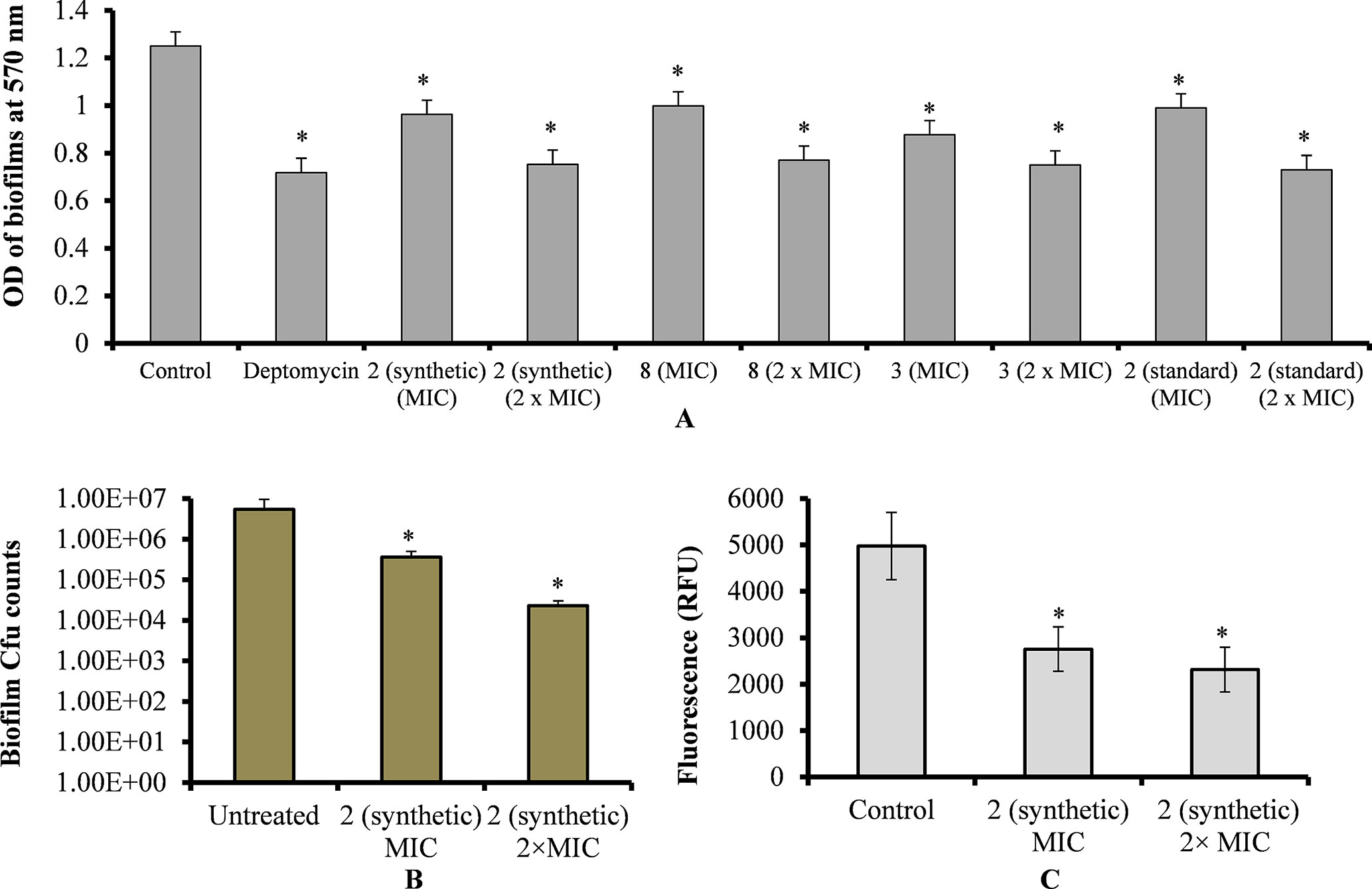
Figure 4 Effect of viniferins on pre-established biofilms of S. pneumoniae. (A) Treatment of pre-established biofilm with MIC and 2 × MIC of viniferins and biofilm biomass was quantified via CV microplate assay. (B) Treatment of pre-established biofilm with MIC and 2 × MIC of ε-viniferin (2) and viable bacteria detected by cfu counts. (C) Metabolically active bacteria within biofilms treated with MIC and 2 × MIC of ε-viniferin (2) via resazurin staining. The error bars represent standard deviations. Statistical significance was calculated by Student’s t-test, and * = p < 0.05 was considered significant.
Viniferin Treatment Distorts S. pneumoniae Within Biofilms
Changes that occur in biofilm structures following viniferin treatment were evaluated using SEM. SEM revealed that intact three-dimensional biofilms with typical EPS matrices were deposited on the surface of bacteria in control samples (Figures 5A, B). However, the analysis also revealed that the structures of biofilms treated with ε-viniferin (2) were altered (Figures 5C, D). Cells in viniferin-treated biofilms were distorted, and the cell membrane appeared shrunken. The lysed cells (Figure 5D, arrow) indicated that viniferin effectively killed bacteria within the biofilm but was unable to completely disassemble the biofilm matrix. Also, the EPS present on bacterial surfaces in biofilms of control samples (Figure 5B, arrow) was absent on viniferin-treated biofilm bacterial surfaces.
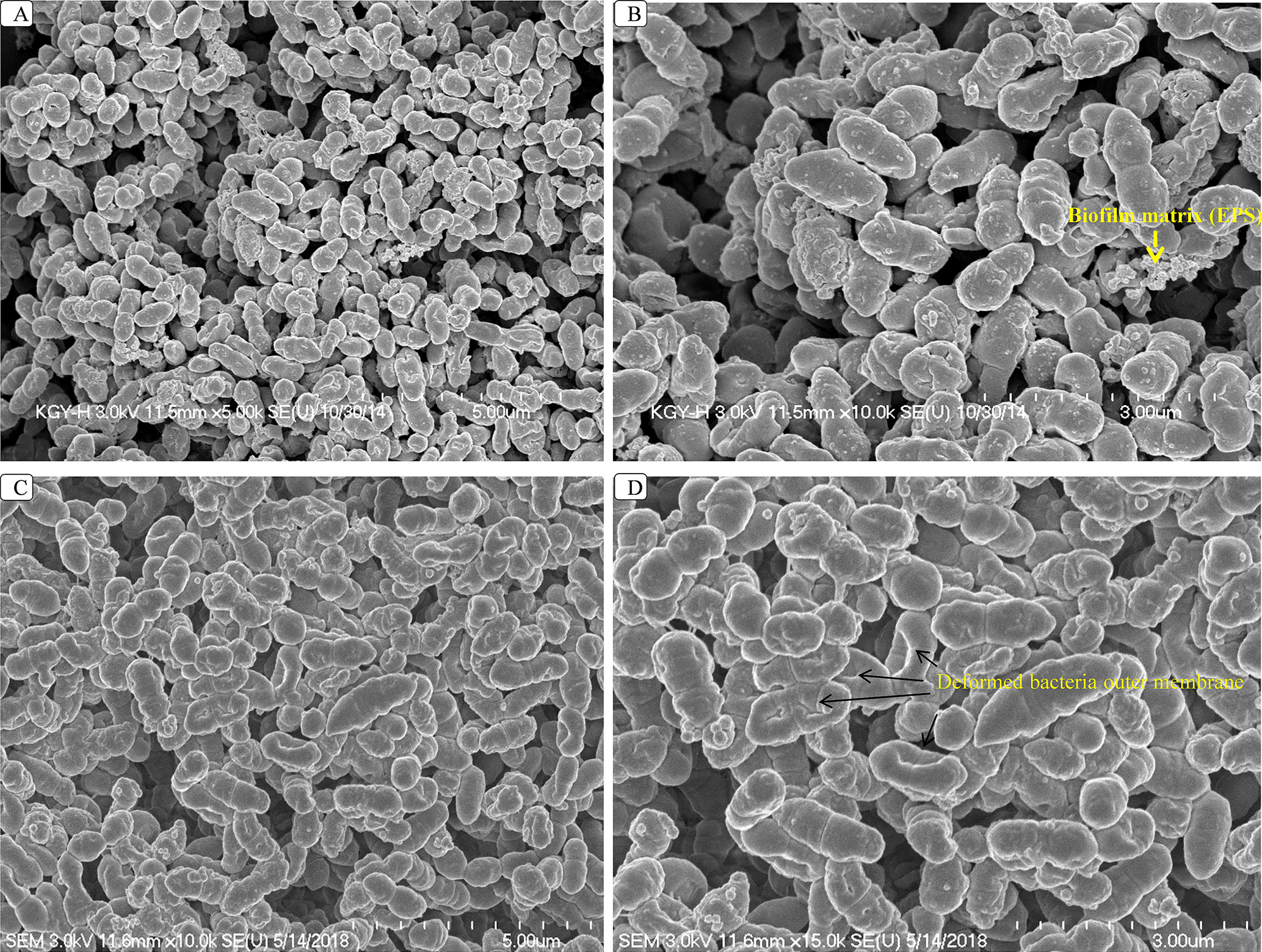
Figure 5 SEM analysis of S. pneumoniae biofilms treated with 2 × MIC of ε-viniferin (2). Images A and B are SEM images of control biofilms. Images C and D are SEM images of viniferin-treated biofilms. In viniferin-treated biofilms, cells appear shrunken, and cell membranes were damaged (indicated by arrow). SEM images were 5 and 3 µm.
Confocal Microscopy Analysis of Pre-Established Biofilms
The LIVE/DEAD biofilm viability kit utilizes a mixture of the SYTO® 9 green-fluorescent nucleic acid stain and the red-fluorescent nucleic acid stain, propidium iodide. Bacteria with intact cell membranes (live cells) are stained green, and those with damaged membranes are stained red. Live/dead biofilm staining revealed that control (DMSO) biofilms were compact with no visible dead cells (Figures 6A, B). However, biofilms treated with ε-viniferin (2) were thin, and dead cells (red) or cells with compromised membranes (yellow) were visible (Figures 6C, D), indicating that viniferin treatment kills pneumococci in biofilm.
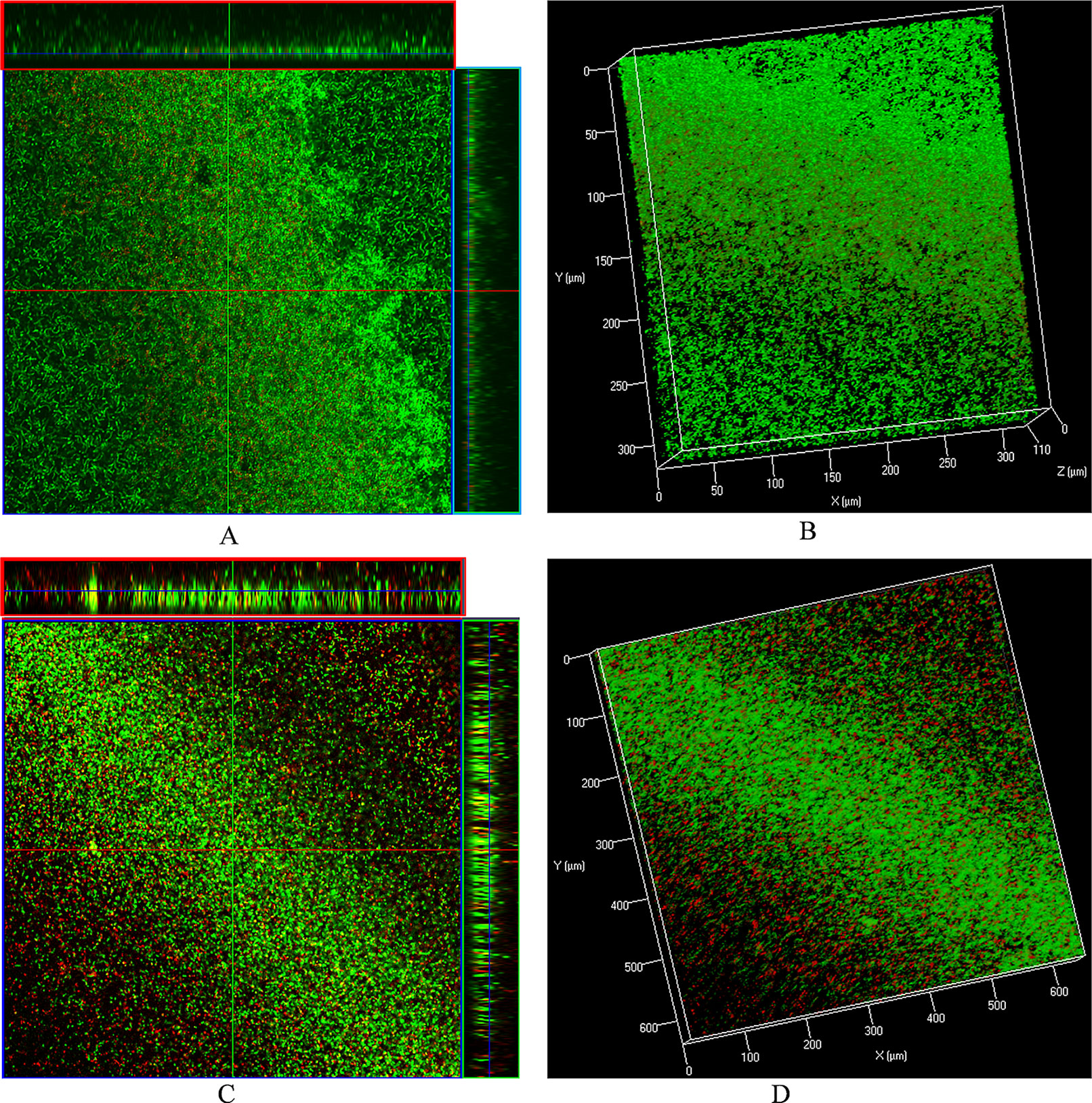
Figure 6 Live/dead biofilm viability staining and confocal microscopy of pre-established biofilms treated with ε-viniferin (2). (A) 2-dimensional (with ortho-section) confocal microscopy image of control biofilms and (B) 3-dimensional image of control biofilms. (C) 2-D (with ortho-section) confocal microscopy image of pre-established biofilm treated with 2 × MIC viniferin and (D) 3-D confocal microscopy image of viniferin-treated biofilms.
Viniferin Kills 99% of Streptococcus pneumoniae Bacteria
A bacteria killing time experiment was performed to determine whether the inhibitory activity of viniferin is bacteriostatic or bactericidal. Untreated (control) bacterial growth was increased at 6 h but declined at 12 and 24 h (Figure 7). However, viable bacteria in viniferin-treated samples declined after 6 h incubation. Viability of S. pneumoniae treated with viniferins 2, 3, and 8 for 6, 12, and 24 h are shown (Figure 7). At 0 h treatment, the cfu counts of the untreated (control) sample and those treated with viniferin 2, 3, and 8 were approximately similar (4×107 cfu/ml). After 6 h of treatment, the cfu of the untreated sample was elevated and reached 4.30×108 cfu/ml. Following 12 and 24 h of treatment, cfu declined to 4.20×107 and 3.0×105 cfu/ml, respectively. In contrast, the cfu of S. pneumoniae treated with viniferins 2, 3, and 8 declined in a time-dependent manner. After 6 h treatment with 2 (synthetic), 3, 8, and 2 (standard), the cfu counts of S. pneumoniae declined to 8.3×106, 1.6×107, 9.0×106, and 8.0×106, respectively. With increasing viniferin 2, 3, and 8 treatment time, the viability of S. pneumoniae further declined at 12 h (1.6×106, 5.4×106, 5.0×106, and 5.4×106) and 24 h (5.0×104, 4.1×105, 3.0×105, and 5.5×104) (Figure 7). Viable bacteria in samples treated with viniferins 2 (synthetic), 3, 8, and 2 (standard) decreased by 87–96% at 12 h and 98–99% at 24 h. Among all compounds tested, synthetic and standard ε-viniferin 2 were most effective in killing bacteria (approximately decreased 2.8 log10). By definition, a compound that kills >3 log10 bacteria following 24 h incubation is considered bactericidal (CLSI, 1999). In this study, ε-viniferin 2 killed a maximum of 2.8 log10 bacteria following 24 h incubation and therefore did not qualify for bactericidal activity as per definition.
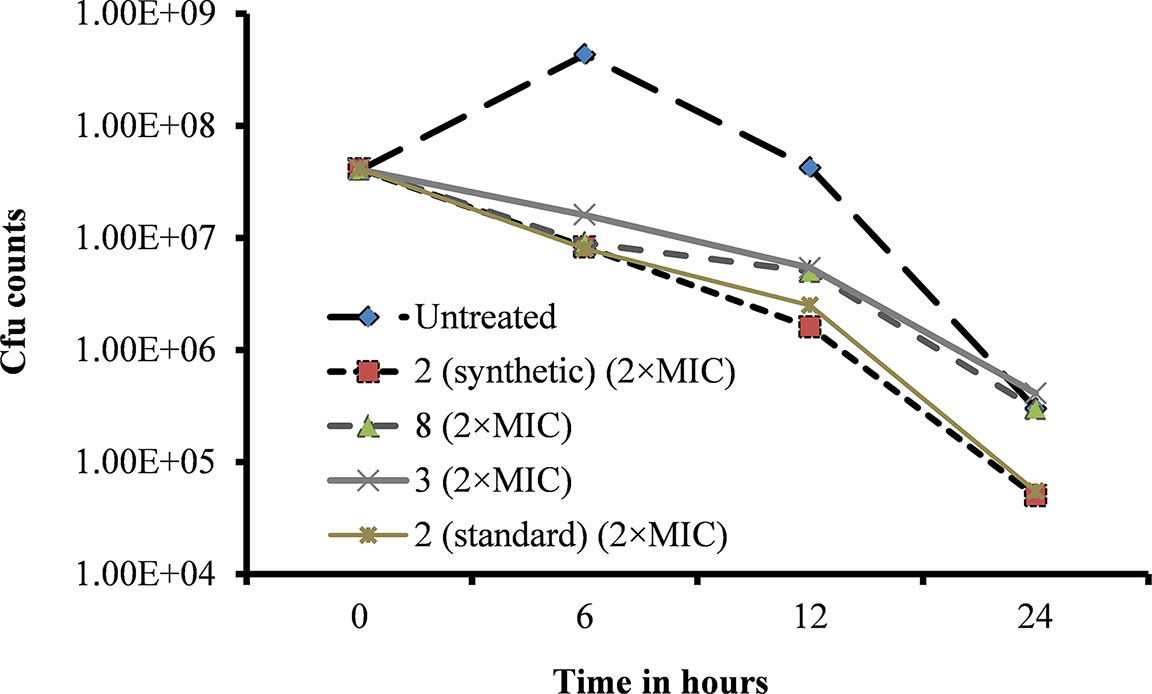
Figure 7 Effect of viniferins on viability of S. pneumoniae D39. Early-log-phase bacteria were treated with 2 × MIC concentration of viniferins, and bacterial viability was detected via cfu counts at 0, 6, 12, and 24 h.
Viniferin Increases CV Absorption
A CV absorption assay was performed to determine alterations in bacteria cell membrane permeability due to viniferin treatment. The assay demonstrated increased CV absorption by viniferin-treated bacteria, compared with untreated cells (control). CV uptake by untreated bacteria (control) was approximately 40% (Figure 8). However, the bacteria treated with ε-viniferin 2 at MIC and 2 × MIC demonstrated 61% and 74% CV uptake, respectively (Figure 8). Increased CV absorption by viniferin-treated bacteria indicates that bacterial cell membrane permeability was altered (Figure 8). Erythromycin, known for its undetectable effect on cell permeability in non-growing bacteria, was used as a negative control.
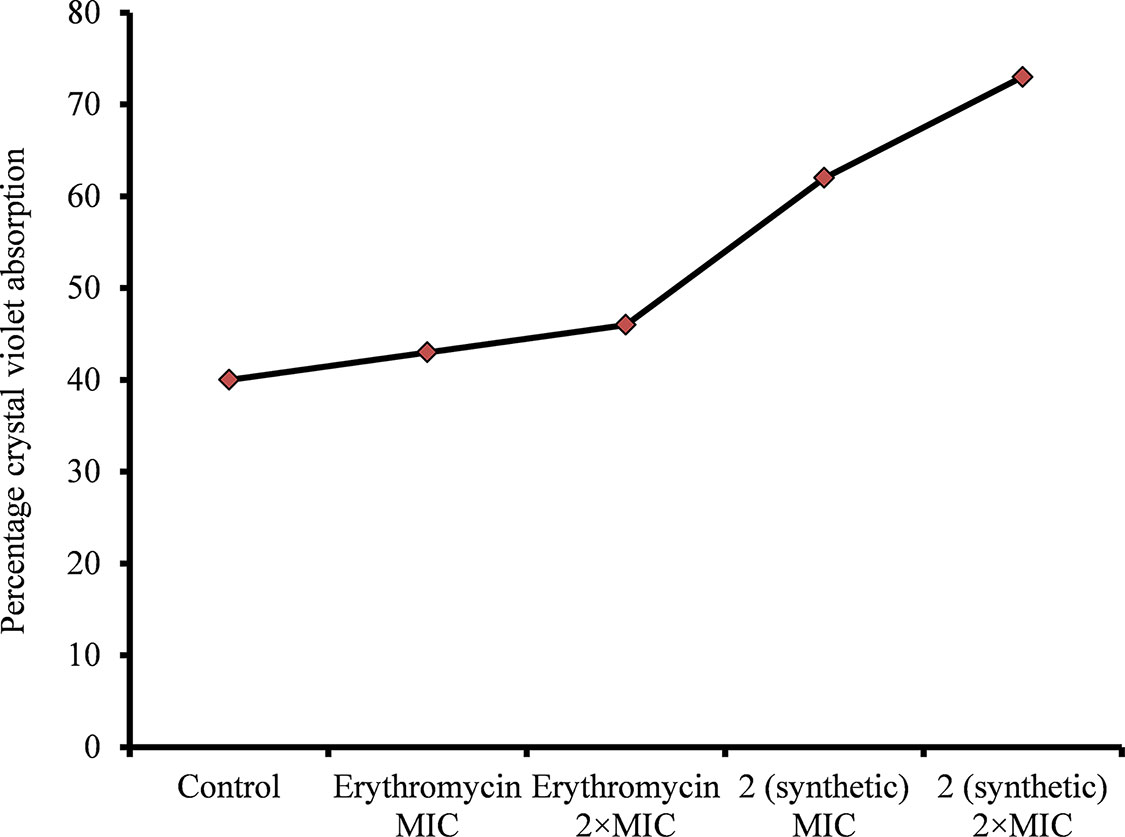
Figure 8 Crystal violet absorption by S. pneumoniae D39 treated with ε-viniferin (2) or erythromycin. The log-phase S. pneumoniae cells were treated with MIC or 2 × MIC of ε-viniferin or erythromycin, and percentage crystal violet absorption was measured as shown in the “Materials and Methods” section.
Total Protein, DNA, and RNA Release by Viniferin-Treated Bacteria
The leakage of cytoplasmic proteins through the cell membrane was examined to evaluate the integrity of pneumococci membrane on viniferin treatment. Protein quantification results showed that total protein release by viniferin-treated bacteria was significantly elevated (P < 0.05) compared to that by control bacteria. Total protein released by bacteria treated with MIC and 2 × MIC viniferin was significantly increased by 43% and 59%, respectively (p < 0.05) (Figure 9A).
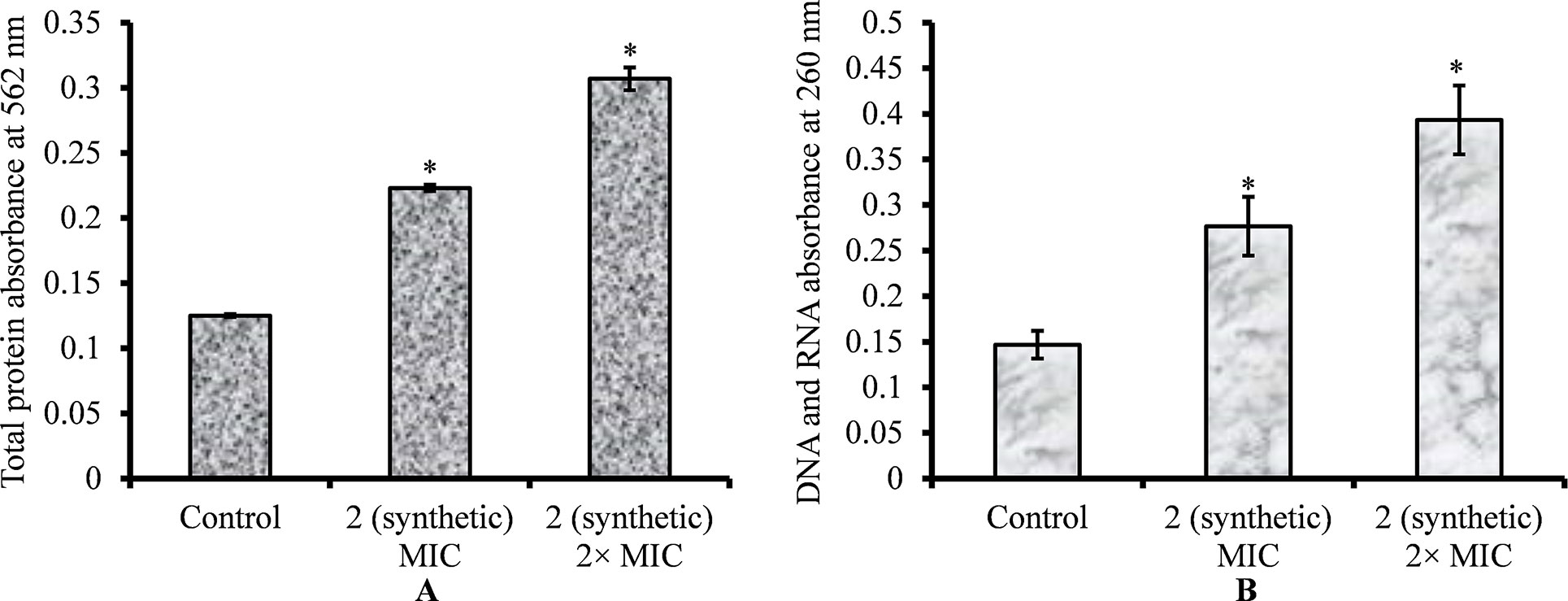
Figure 9 Quantification of total protein and genetic material (DNA and RNA) released by S. pneumoniae D39 on viniferin treatment. (A) Quantification of total protein released by pneumococci on viniferin treatment (MIC or 2 × MIC), detected by Pierce BCA protein assay. The results are expressed as the absorbance of total proteins at 562 nm. (B) Measurement of total DNA and DNA in cell-free supernatant, assessed by detecting absorbance at 260 nm. Error bars represent standard deviations. Statistical significance was calculated using Student’s t-test, and p < 0.05 was considered significant.
The leakage of genetic material (DNA and RNA) through the pneumococcal membrane on viniferin treatment was increased. Quantification of DNA and RNA results showed significantly increased (p < 0.05) DNA and RNA in viniferin-treated bacteria (Figure 9B). At MIC and 2 × MIC viniferin, DNA and DNA release increased by 46% and 64%, respectively, in comparison to the control (Figure 9B).
Discussion
S. pneumoniae causes various infectious diseases and is a leading cause of child morbidity. Approximately 25–60% of healthy children are pneumococci carriers (Korona-Glowniak et al., 2018). Reportedly, biofilms of S. pneumoniae are established in the nasopharynx, and planktonic bacteria from biofilms may transit to sterile mucosal tissue, causing Acute Otitis Media (AOM), pneumonia, bacteremia, or local infections (Bogaert et al., 2004). The biofilm resistance paradigm obstructs treatment, resulting in recurrent and recalcitrant infections, which increase recovery time and treatment costs (Costerton et al., 1999; Thomas et al., 2006). Classical antibiotics targeting actively growing bacteria are ineffective due to the dynamic structure of biofilms. Therefore, it is necessary to find new antimicrobials that can effectively control biofilms and prevent the development of antimicrobial resistance (Chopra et al., 2002). One strategy to prevent biofilm-related infections is to inhibit or eradicate initial colonization by bacteria using natural compounds. Reportedly, various phyto-compounds such as 220D-F2 derived from Rubus ulmifolius, carvacrol (a constituent of oregano), Melaleuca alternifolia (tea tree oil), magnolol, and eugenol are effective against biofilms (Kwieciński et al., 2009; Ma et al., 2012; Quave et al., 2012; van Alphen et al., 2012; Burt et al., 2014; Yadav et al., 2015). We developed a novel method for synthesizing viniferin via oxidative coupling with ruthenium chloride and tested its antimicrobial and antibiofilm potential against S. pneumoniae.
Different viniferin compounds, ε-viniferin (2), (E)-ω-viniferin (3), 8, and 9, exhibited different MICs for S. pneumoniae inhibition. At 20 µm, ε-viniferin 2 completely inhibited bacterial growth, whereas compounds 3, 8, and 9 were ineffective. At 40 µm, compounds 3 and 8, inhibited bacterial growth, but compound 9 did not. These results indicated that ε-viniferin (2) is more active compared to compounds 3 and 8, while 9 was least active. Structural or functional group differences probably contribute to differences in the antimicrobial activity of compounds 2, 3, 8, and 9. Similarly, differences in antimicrobial activity associated with alterations in structural or functional groups have been reported for eugenol and carvacrol (Cacciatore et al., 2015; da Silva et al., 2018).
S. pneumoniae consists of various serotypes, and our results showed that ε-viniferin (2) was able to inhibit S. pneumoniae serotypes 2, 3, 19A, 19F, and un-encapsulated R6. Serotype 19 is the most prevalent pneumococcus following introduction of the 7-valent pneumococci vaccine (Isturiz et al., 2017; Koutouzis et al., 2017). Treatment of pneumococcal infections caused by antibiotic resistance strains remains a considerable challenge in clinical settings (Korona-Glowniak et al., 2018; Setchanova et al., 2018). Our results showed that ε-viniferin (2) effectively inhibited pneumococci resistant to antibiotics (clindamycin, erythromycin, and tetracycline). Altogether, our results indicated that viniferin is equally effective on pneumococci resistant to antibiotics (clindamycin >128, erythromycin >512, and tetracycline 16 µg/ml), as well as serotypes 2, 3, 19A, 19F, and un-encapsulated R6.
Pneumococcal biofilms cause various infections including AOM, pneumonia, bacteremia, and meningitis (Hall-Stoodley et al., 2006; Pichichero et al., 2013; Shak et al., 2013). These biofilms act as bacterial reservoirs from where bacteria can transit in the form of planktonic bacteria to other sterile anatomical sites, causing infections (Hall-Stoodley et al., 2006; Sanchez et al., 2010; Weimer et al., 2010). Therefore, controlling pneumococcal biofilms is important for infection management. Viniferin at MIC prevented biofilm formation in all tested serotypes, including strains resistant to clindamycin >128, erythromycin >512, and tetracycline 16 µg/ml. In contrast, classical aminoglycoside antibiotics accelerate stress in bacteria, resulting in increased biofilm formation at low MICs or sub-MICs (Hoffman et al., 2005). Therefore, an ideal antimicrobial agent should not induce biofilm growth at low concentrations but be effective in preventing biofilm aggregation and eradicating pre-established biofilms. Viniferin shows potential as an ideal antimicrobial agent for pneumococcal biofilm control. Our findings demonstrate that viniferin does not induce biofilm formation at sub-MIC, although it was unable to inhibit pneumococci biofilm formation at sub-MIC. However, it completely inhibited pneumococcal growth under biofilm and planktonic states at MIC. Moreover, viniferin is toxic to bacteria, and the treatment by 2 × MIC viniferin significantly reduced bacterial load by 2.8 log10 after 24 h. However, definitions of bactericidal and bacteriostatic indicate that viniferin was not bactericidal (a drug is bacteriostatic if it kills <3 log10 bacteria and bactericidal if it kills >3 log10 bacteria after 24 h of incubation) (Basri et al., 2014). Similarly, bacteriostatic effects were previously reported for methicillin-resistant Staphylococcus aureus (Basri et al., 2014). Reportedly, plant extracts containing viniferin inhibited in vitro biofilm growth of Gram-negative bacteria such as Pseudomonas aeruginosa and E. coli. However, the target of viniferin or underlying mechanisms was not reported (Cho et al., 2013; Lee et al., 2014). In this study, no effects of viniferin on in vitro biofilm growth of pneumococci were detected. This implies that viniferin activity is different for Gram-positive and Gram-negative bacteria. The difference between biofilm-inhibiting activities of viniferin in P. aeruginosa (Gram-negative bacteria) and S. pneumoniae (Gram-positive) may be due to a difference in mechanisms underlying biofilm regulation and biofilm matrix composition (Hall and Mah, 2017). Another reason may be cell membrane differences; the cytoplasmic membrane of Gram-positive bacteria is surrounded by a relatively simple porous cell wall, whereas the cell envelope of Gram-negative bacteria is more complex and relatively impermeable, consisting of an inner cytoplasmic membrane and an outer membrane separated by a peptidoglycan layer (Minnock et al., 2000).
The microbial biofilms are resistant to antibiotics, and the dynamic structure of mature biofilm confers resistance against antimicrobials. Typically, biofilms are surrounded by a self-generated extracellular polymeric matrix (Costerton et al., 1995; Donlan and Costerton, 2002). Reportedly, the charged polymer of the biofilm obstructs drug diffusion through the matrix, thereby limiting drug effects to the surface and leaving bacteria deep inside the biofilm relatively safe (Marsh, 2004). Our results showed that viniferin-treated pre-established biofilms exhibited decreased cfu counts and biofilm biomasses. These results indicated that viniferin may have killed bacteria within the biofilms. SEM analysis of pre-established biofilms treated with viniferin revealed deformed and shrunken cells in biofilms. The bacteria in viniferin-treated biofilms had lost shape, resulting in a shrunken appearance. Confocal microscopy revealed dead cells in viniferin-treated biofilms. CV absorption assay revealed that the viniferin treatment altered bacterial membrane permeability. Leakage of total protein, DNA, and RNA further confirmed that the viniferin treatment disrupted the integrity of the bacterial membrane (Bouyahya et al., 2019). Altogether, SEM analysis; live/dead biofilm staining; CV absorption; and total protein, DNA, and RNA release revealed that viniferin altered bacterial cell permeability and caused cell lysis, indicating that bacteria cell membrane was the target of viniferin. Similar activity was demonstrated by our group in the polyphonic phyto-compounds, eugenol and carvacrol (Devi et al., 2010; Yadav et al., 2013; Yadav et al., 2015; Khan et al., 2017). Classical antibiotics targeting different metabolic pathways of actively growing bacteria are often ineffective against biofilms. Bacteria in biofilm grow slowly, express different genes than planktonic bacteria, and adapt alternate metabolic pathways (Xie et al., 2005; Mascio et al., 2007; Yadav et al., 2012). Biofilms grow slowly in nutrient-depleted conditions, which makes them insensitive to antibiotics (Davies, 2003). The findings of this study and previous reports indicate that viniferin is an important, therapeutic bioactive compound that possesses anti-obesity, anticancer, antimicrobial and antibiofilm properties (Piver et al., 2003; Ohara et al., 2015; Monteillier et al., 2018).
Conclusion
The current study proposes a simple and short procedure for the synthesis of (±)-ε-viniferin (2) and (±)-E-ω-viniferin (3) in large quantities. Furthermore, (±)-ε-viniferin (2), (±)-E-ω-viniferin (3), and compound 8 effectively inhibited S. pneumoniae growth and killed bacteria in biofilms. The killing effect of viniferin may due to alteration of cell membrane permeability. Viniferin is known for anti-obesity and anticancer activities, and our study demonstrated its antibacterial and antibiofilm activities against S. pneumoniae. Therefore, viniferin and its derivatives show potential as promising candidate molecules for the development of novel antimicrobial agents against pneumococci.
Data Availability
The raw data supporting the conclusions of this manuscript will be made available by the authors, without undue reservation, to any qualified researcher.
Author Contributions
MKY and WJC conceived and designed the experiments. MKY, KM, and JNM performed the experiments. MKY, KB, and JNM were in charge of manuscript writing. J-JS, WJC, and MKY were in charge of manuscript review. J-JS, WJC, and S-WC analyzed the data. J-JS, S-WC, and WJC contributed reagents/materials/analysis tools.
Funding
This research was supported by the Basic Science Research Program of the National Research Foundation (NRF) of Korea funded by Ministry of Education grant (2017R1D1A1B03035306 to MKY). This research was also supported by the Basic Science Research Program through the National Research Foundation of Korea (NRF) funded by the Ministry of Education (NRF-2017R1D1A1B03036116).
Conflict of Interest Statement
The authors declare that the research was conducted in the absence of any commercial or financial relationships that could be construed as a potential conflict of interest.
Acknowledgments
We thank Professor Kee Dong Yoon (Catholic University of Korea) for kindly providing an authentic sample of standard viniferin and analytical data.
References
Avery, O. T., Macleod, C. M., McCarty, M. (1944). Studies on the chemical nature of the substance inducing transformation of pneumococcal types: induction of transformation by a desoxyribonucleic acid fraction isolated from pneumococcus type III. J. Exp. Med. 79, 137–158.
Basri, D. F., Xian, L. W., Abdul Shukor, N. I., Latip, J. (2014). Bacteriostatic antimicrobial combination: antagonistic interaction between epsilon-viniferin and vancomycin against methicillin-resistant Staphylococcus aureus. BioMed. Res. Int. 2014, 8. doi: 10.1155/2014/461756
Bergenfelz, C., Hakansson, A. P. (2017). Streptococcus pneumoniae otitis media pathogenesis and how it informs our understanding of vaccine strategies. Curr. Otorhinolaryngol. Rep. 5 (2), 115–124. doi: 10.1007/s40136-017-0152-6
Bogaert, D., de Groot, R., Hermans, P. W. M. (2004). Streptococcus pneumoniae colonisation: the key to pneumococcal disease. Lancet Infect. Dis. 4 (3), 144–154. doi: 10.1016/S1473-3099(04)00938-7
Bouyahya, A., Abrini, J., Dakka, N., Bakri, Y. (2019). Essential oils of Origanum compactum increase membrane permeability, disturb cell membrane integrity, and suppress quorum-sensing phenotype in bacteria. J. Pharm. Anal. doi: 10.1016/j.jpha.2019.03.001
Burt, S. A., Ojo-Fakunle, V. T. A., Woertman, J., Veldhuizen, E. J. A. (2014). The natural antimicrobial carvacrol inhibits quorum sensing in Chromobacterium violaceum and reduces bacterial biofilm formation at sub-lethal concentrations. PLoS One 9 (4), e93414. doi: 10.1371/journal.pone.0093414
Cacciatore, I., Di Giulio, M., Fornasari, E., Di Stefano, A., Cerasa, L. S., Marinelli, L., et al. (2015). Carvacrol codrugs: a new approach in the antimicrobial plan. PLoS One 10 (4), e0120937. doi: 10.1371/journal.pone.0120937
Cho, H. S., Lee, J.-H., Ryu, S. Y., Joo, S. W., Cho, M. H., Lee, J. (2013). Inhibition of Pseudomonas aeruginosa and Escherichia coli O157:H7 biofilm formation by plant metabolite -viniferin. J. Agric. Food Chem. 61 (29), 7120–7126. doi: 10.1021/jf4009313
Chopra, I., Hesse, L., O’Neill, A. J. (2002). Exploiting current understanding of antibiotic action for discovery of new drugs. J. Appl. Microbiol. 92 (s1), 4S–15S. doi: 10.1046/j.1365-2672.92.5s1.13.x
Christensen, G. D., Simpson, W. A., Younger, J. J., Baddour, L. M., Barrett, F. F., Melton, D. M., et al. (1985). Adherence of coagulase-negative staphylococci to plastic tissue culture plates: a quantitative model for the adherence of staphylococci to medical devices. J. Clin. Microbiol. 22 (6), 996–1006.
Cichewicz, R. H., Kouzi, S. A. (2002). “Resveratrol oligomers: structure, chemistry, and biological activity,” in Studies in Natural Products Chemistry. Ed. Atta ur, R.. (California, USA: Elsevier), 507–579. doi: 10.1016/S1572-5995(02)80014-X
CLSI (1999). Methods for determining bactericidal activity of antimicrobial agents, Clinical and Laboratory Standards Institute 1999. M26-A Vol. 19. Wayne, PA: Clinical and Laboratory Standards Institute.
CLSI. (2005). “Performance standards for antimicrobial susceptibility testing fifteenth informational supplement,” in CLSI/NCCLS Document M100-S15 (CLSI Wayne, PA: Clinical and Laboratory Standards Institute).
Co, J. Y., Cárcamo-Oyarce, G., Billings, N., Wheeler, K. M., Grindy, S. C., Holten-Andersen, N., et al. (2018). Mucins trigger dispersal of Pseudomonas aeruginosa biofilms. NPJ Biofilms Microbiomes 4, 23. doi: 10.1038/s41522-018-0067-0
Costerton, J. W., Lewandowski, Z., Caldwell, D. E., Korber, D. R., Lappin-Scott, H. M. (1995). Microbial biofilms. Annu. Rev. Microbiol. 49 (1), 711–745. doi: 10.1146/annurev.mi.49.100195.003431
Costerton, J. W., Stewart, P. S., Greenberg, E. P. (1999). Bacterial biofilms: a common cause of persistent infections. Science 284 (5418), 1318–1322. doi: 10.1126/science.284.5418.1318
da Silva, F. F. M., Monte, F. J. Q., de Lemos, T. L. G., Nascimento, P. G. G., de Medeiros Costa, A. K., de Paiva, L. M. M. (2018). Eugenol derivatives: synthesis, characterization, and evaluation of antibacterial and antioxidant activities. Chem. Cent. J. 12, 34. doi: 10.1186/s13065-018-0407-4
Davies, D. (2003). Understanding biofilm resistance to antibacterial agents. Nat. Rev. Drug Discov. 2, 114. doi: 10.1038/nrd1008
Devi, K. P., Nisha, S. A., Sakthivel, R., Pandian, S. K. (2010). Eugenol (an essential oil of clove) acts as an antibacterial agent against Salmonella typhi by disrupting the cellular membrane. J. Ethnopharmacol. 130 (1), 107–115. doi: 10.1016/j.jep.2010.04.025
Donlan, R. M., Costerton, J. W. (2002). Biofilms: survival mechanisms of clinically relevant microorganisms. Clin. Microbiol. Rev. 15 (2), 167–193. doi: 10.1128/CMR.15.2.167-193.2002
Fiorentino, A., D’Abrosca, B., Pacifico, S., Izzo, A., Letizia, M., Esposito, A., et al. (2008). Potential allelopatic effects of stilbenoids and flavonoids from leaves of Carex distachya Desf. Biochem. Syst. Ecol. 36 (9), 691–698. doi: 10.1016/j.bse.2008.07.002
Gao, Y., Sharpless, K. B. (1988). Vicinal diol cyclic sulfates. Like epoxides only more reactive. J. Am. Chem. Soc. 110 (22), 7538–7539. doi: 10.1021/ja00230a045
Hall, C. W., Mah, T. F. (2017). Molecular mechanisms of biofilm-based antibiotic resistance and tolerance in pathogenic bacteria. FEMS Microbiol. Rev. 41 (3), 276–301. doi: 10.1093/femsre/fux010
Hall-Stoodley, L., Hu, F. Z., Gieseke, A., Nistico, L., Nguyen, D., Hayes, J., et al.(2006). Direct detection of bacterial biofilms on the middle-ear mucosa of children with chronic otitis media. JAMA 296 (2), 202–211. doi: 10.1001/jama.296.2.202
Hemaiswarya, S., Doble, M. (2009). Synergistic interaction of eugenol with antibiotics against Gram negative bacteria. Phytomedicine 16 (11), 997–1005. doi: 10.1016/j.phymed.2009.04.006
Hoa, M., Tomovic, S., Nistico, L., Hall-Stoodley, L., Stoodley, P., Sachdeva, L., et al. (2009). Identification of adenoid biofilms with middle ear pathogens in otitis-prone children utilizing SEM and FISH. Int. J. Pediatr. Otorhinolaryngol. 73 (9), 1242–1248. doi: 10.1016/j.ijporl.2009.05.016
Hoffman, L. R., D’Argenio, D. A., MacCoss, M. J., Zhang, Z., Jones, R. A., Miller, S. I. (2005). Aminoglycoside antibiotics induce bacterial biofilm formation. Nature 436, 1171. doi: 10.1038/nature03912
Høiby, N., Bjarnsholt, T., Givskov, M., Molin, S., Ciofu, O. (2010). Antibiotic resistance of bacterial biofilms. Int. J. Antimicrob. Agents 35 (4), 322–332. doi: 10.1016/j.ijantimicag.2009.12.011
Hurdle, J. G., O’Neill, A. J., Chopra, I., Lee, R. E. (2011). Targeting bacterial membrane function: an underexploited mechanism for treating persistent infections. Nature reviews. Microbiology 9 (1), 62–75. doi: 10.1038/nrmicro2474
Hwang, D., Lim, Y.-H. (2015). Resveratrol antibacterial activity against Escherichia coli is mediated by Z-ring formation inhibition via suppression of FtsZ expression. Sci. Rep. 5, 10029. doi: 10.1038/srep10029
Isturiz, R., Sings, H. L., Hilton, B., Arguedas, A., Reinert, R.-R., Jodar, L. (2017). Streptococcus pneumoniae serotype 19A: worldwide epidemiology. Expert Rev. Vaccines 16 (10), 1007–1027. doi: 10.1080/14760584.2017.1362339
Ito, J., Takaya, Y., Oshima, Y., Niwa, M. (1999). New oligostilbenes having a benzofuran from Vitis vinifera‘Kyohou’. Tetrahedron 55, 2529–2544. doi: 10.1016/S0040-4020(99)00039-3
Khan, I., Bahuguna, A., Kumar, P., Bajpai, V. K., Kang, S. C. (2017). Antimicrobial potential of carvacrol against uropathogenic Escherichia coli via membrane disruption, depolarization, and reactive oxygen species generation. Front. Microbiol. 8 (2421). doi: 10.3389/fmicb.2017.02421
Korona-Glowniak, I., Zychowski, P., Siwiec, R., Mazur, E., Niedzielska, G., Malm, A. (2018). Resistant Streptococcus pneumoniae strains in children with acute otitis media– high risk of persistent colonization after treatment. BMC Infect. Dis. 18, 478. doi: 10.1186/s12879-018-3398-9
Koutouzis, E. I., Daikos, G. L., Chatzichristou, P., Michos, A., Tsakris, A., Koutouzi, F. I., et al. (2017). Characteristics of Streptococcus pneumoniae serotype 19A isolates from children in the pre- and post-conjugate vaccine era. Open Forum Infect. Dis. 4 (Suppl 1), S127. doi: 10.1093/ofid/ofx163.174
Kwieciński, J., Eick, S., Wójcik, K. (2009). Effects of tea tree (Melaleuca alternifolia) oil on Staphylococcus aureus in biofilms and stationary growth phase. Int. J. Antimicrob. Agents 33 (4), 343–347. doi: 10.1016/j.ijantimicag.2008.08.028
Lee, J.-H., Kim, Y.-G., Ryu, S. Y., Cho, M. H., Lee, J. (2014). Resveratrol oligomers inhibit biofilm formation of Escherichia coli O157:H7 and Pseudomonas aeruginosa. J. Nat. Prod. 77 (1), 168–172. doi: 10.1021/np400756g
Lindgen, A. E. G., Oberg, C. T., Hillgren, J. M., Elofsson, M. (2016). Total synthesis of the resveratrol oligomers (±)-ampelopsin B and (±)-E-viniferin. Eur. J. Org. Chem. 2016, 426–429. doi: 10.1002/ejoc.201501486
Ma, D. S. L., Tan, L. T.-H., Chan, K.-G., Yap, W. H., Pusparajah, P., Chuah, L.-H., et al. (2018). Resveratrol—potential antibacterial agent against foodborne pathogens. Front. Pharmacol. 9, 102. doi: 10.3389/fphar.2018.00102
Ma, Y., Xu, Y., Yestrepsky, B. D., Sorenson, R. J., Chen, M., Larsen, S. D., et al. (2012). Novel Inhibitors of Staphylococcus aureus virulence gene expression and biofilm formation. PLoS One 7 (10), e47255. doi: 10.1371/journal.pone.0047255
Marsh, P. D. (2004). Dental plaque as a microbial biofilm. Caries Res. 38 (3), 204–211. doi: 10.1159/000077756
Mascio, C. T. M., Alder, J. D., Silverman, J. A. (2007). Bactericidal action of daptomycin against stationary-phase and nondividing Staphylococcus aureus cells. Antimicrob. Agents Chemother. 51 (12), 4255–4260. doi: 10.1128/AAC.00824-07
Mattivi, F., Vrhovsek, U., Malacarne, G., Masuero, D., Zulini, L., Stefanini, M., et al. (2011). Profiling of resveratrol oligomers, important stress metabolites, accumulating in the leaves of hybrid Vitis vinifera (Merzling × Teroldego) genotypes infected with Plasmopara viticola. J. Agric. Food Chem. 59 (10), 5364–5375. doi: 10.1021/jf200771y
Minnock, A., Vernon, D. I., Schofield, J., Griffiths, J., Parish, J. H., Brown, S. B. (2000). Mechanism of uptake of a cationic water-soluble pyridinium zinc phthalocyanine across the outer membrane of Escherichia coli. Antimicrob. Agents Chemother. 44 (3), 522–527. doi: 10.1128/AAC.44.3.522-527.2000
Monteillier, A., Voisin, A., Furrer, P., Allémann, E., Cuendet, M. (2018). Intranasal administration of resveratrol successfully prevents lung cancer in A/J mice. Sci Rep 8, 14257. doi: 10.1038/s41598-018-32423-0
Moscoso, M., García, E., López, R. (2006). Biofilm formation by Streptococcus pneumoniae: role of choline, extracellular DNA, and capsular polysaccharide in microbial accretion. J. Bacteriol. 188 (22), 7785–7795. doi: 10.1128/JB.00673-06
Ohara, K., Kusano, K., Kitao, S., Yanai, T., Takata, R., Kanauchi, O. (2015). -Viniferin, a resveratrol dimer, prevents diet-induced obesity in mice. Biochem. Biophys. Res. Commun. 468 (4), 877–882. doi: 10.1016/j.bbrc.2015.11.047
Paytubi, S., de La Cruz, M., Tormo, J. R., Martín, J., González, I., González-Menendez, V., et al. (2017). A high-throughput screening platform of microbial natural products for the discovery of molecules with antibiofilm properties against Salmonella. Front. Microbiol. 8 (326). doi: 10.3389/fmicb.2017.00326
Pichichero, M. E., Casey, J. R., Almudevar, A. (2013). Reducing the frequency of acute otitis media by individualized care. Pediatr. Infect. Dis. J. 32 (5), 473–478. doi: 10.1097/INF.0b013e3182862b57
Piver, B., Berthou, F., Dreano, Y., Lucas, D. (2003). Differential inhibition of human cytochrome P450 enzymes by -viniferin, the dimer of resveratrol: comparison with resveratrol and polyphenols from alcoholized beverages. Life Sci. 73 (9), 1199–1213. doi: 10.1016/S0024-3205(03)00420-X
Quave, C. L., Estévez-Carmona, M., Compadre, C. M., Hobby, G., Hendrickson, H., Beenken, K. E., et al. (2012). Ellagic acid derivatives from Rubus ulmifolius inhibit Staphylococcus aureus biofilm formation and improve response to antibiotics. Plos One 7 (1), e28737. doi: 10.1371/journal.pone.0028737
Reid, S. D., Hong, W., Dew, K. E., Winn, D. R., Pang, B., Watt, J., et al. (2009). Streptococcus pneumoniae forms surface-attached communities in the middle ear of experimentally infected chinchillas. J. Infect. Dis. 199 (6), 786-794. doi: 10.1086/597042
Sanchez, C. J., Shivshankar, P., Stol, K., Trakhtenbroit, S., Sullam, P. M., Sauer, K., et al. (2010). The pneumococcal serine-rich repeat protein is an intra-species bacterial adhesin that promotes bacterial aggregation in vivo and in biofilms. PLoS Pathog. 6 (8), e1001044. doi: 10.1371/journal.ppat.1001044
Santamaria, A. R., Innocenti, M., Mulinacci, N., Melani, F., Valletta, A., Sciandra, I., et al. (2012). Enhancement of viniferin production in Vitis vinifera L. cv. Alphonse Lavallée cell suspensions by low-energy ultrasound alone and in combination with methyl jasmonate. J. Agric. Food Chem. 60 (44), 11135–11142. doi: 10.1021/jf301936u
Setchanova, L., Alexandrova, A., Pencheva, D., Sirakov, I., Mihova, K., Kaneva, R., et al. (2018). Rise of multidrug-resistant Streptococcus pneumoniae clones expressing non-vaccine serotypes among children following introduction of the 10-valent pneumococcal conjugate vaccine in Bulgaria. J. Glob. Antimicrob. Resist. 15, 6–11. doi: 10.1016/j.jgar.2018.05.012
Shak, J. R., Vidal, J. E., Klugman, K. P. (2013). Influence of bacterial interactions on pneumococcal colonization of the nasopharynx. Trends Microbiol. 21 (3), 129–135. doi: 10.1016/j.tim.2012.11.005
Shimizu, K., Kondo, R., Sakni, K. (2000). Inhibition of tyrosinase by flavonoids, stilbenes and related 4-substituted resorcinols: structure–activity investigations. Planta. Med. 66, 11–15. doi: 10.1055/s-2000-11113
Simell, B., Auranen, K., Käyhty, H., Goldblatt, D., Dagan, R., O’Brien, K. L. (2012). The fundamental link between pneumococcal carriage and disease. Expert Rev. Vaccines 11 (7), 841–855. doi: 10.1586/erv.12.53
Sotheeswaran, S., Pasupathy, V. (1993). Distribution of resveratrol oligomers in plants. Phytochemistry 32 (5), 1083–1092. doi: 10.1016/S0031-9422(00)95070-2
Takaya, Y., Terashima, K., Ito, J., He, Y.-H., Tateoka, M., Yamaguchi, N., et al. (2005). Biomimic transformation of resveratrol. Tetrahedron 61 (43), 10285–10290. doi: 10.1016/j.tet.2005.08.023
Thomas, J. G., Litton, I., Rinde, H. (2006). “Economic impact of biofilms on treatment costs,” in Biofilms, Infection and Antimicrobial Therapy. Eds. Pace, J. L., Rupp, M. E., Finch, R. G. (Boca Raton, FL: CRC Press. Taylor and Francis), 21–37.H. doi: 10.1201/9781420028232.ch2
van Alphen, L. B., Burt, S. A., Veenendaal, A. K. J., Bleumink-Pluym, N. M. C., van Putten, J. P. M. (2012). The natural antimicrobial carvacrol inhibits Campylobacter jejuni motility and infection of epithelial cells. Plos One 7 (9), e45343. doi: 10.1371/journal.pone.0045343
Wardlaw, T. M., Johansson, E. W., Hodge, M. J. (2006). Pneumonia: the forgotten killer of children. UNICEF. doi: 10.1016/S0140-6736(06)69334-3
Weimer, K. E. D., Armbruster, C. E., Juneau, R. A., Hong, W., Pang, B., Swords, W. E. (2010). Coinfection with Haemophilus influenzae promotes pneumococcal biofilm formation during experimental otitis media and impedes the progression of pneumococcal disease. J. Infect. Dis. 202 (7), 1068–1075. doi: 10.1086/656046
Xie, Z., Siddiqi, N., Rubin, E. J. (2005). Differential antibiotic susceptibilities of starved Mycobacterium tuberculosis isolates. Antimicrob. Agents Chemother. 49 (11), 4778–4780. doi: 10.1128/AAC.49.11.4778-4780.2005
Yadav, M. K., Chae, S.-W., Im, G. J., Chung, J.-W., Song, J.-J. (2015). Eugenol: a phyto-compound effective against methicillin-resistant and methicillin-sensitive Staphylococcus aureus clinical strain biofilms. Plos One 10 (3), e0119564. doi: 10.1371/journal.pone.0119564
Yadav, M. K., Go, Y. Y., Kim, S. H., Chae, S.-W., Song, J.-J. (2017). Antimicrobial and antibiofilm effects of human amniotic/chorionic membrane extract on Streptococcus pneumoniae. Front. Microbiol. 8 (1948). doi: 10.3389/fmicb.2017.01948
Yadav, M. K., Kwon, S. K., Cho, C. G., Park, S.-W., Chae, S.-W., Song, J.-J. (2012). Gene expression profile of early in vitro biofilms of Streptococcus pneumoniae. Microbiol. Immunol. 56 (9), 621–629. doi: 10.1111/j.1348-0421.2012.00483.x
Yadav, M. K., Park, S.-W., Chae, S.-W., Song, J.-J., Kim, H. C. (2013). Antimicrobial activities of Eugenia caryophyllata extract and its major chemical constituent eugenol against Streptococcus pneumoniae. APMIS 121 (12), 1198–1206. doi: 10.1111/apm.12067
Keywords: ε-viniferin, antimicobacterial, antibiofilm, Streptococcus pneumoniae, cell membrane
Citation: Yadav MK, Mailar K, Nagarajappa Masagalli J, Chae S-W, Song J-J and Choi WJ (2019) Ruthenium Chloride—Induced Oxidative Cyclization of Trans-Resveratrol to (±)-ε-Viniferin and Antimicrobial and Antibiofilm Activity Against Streptococcus pneumoniae. Front. Pharmacol. 10:890. doi: 10.3389/fphar.2019.00890
Received: 19 March 2019; Accepted: 15 July 2019;
Published: 14 August 2019.
Edited by:
Rong-Rong He, Jinan University, ChinaReviewed by:
Santosh Pandit, Chalmers University of Technology, SwedenLaura Renee Marks, Washington University in St. Louis, United States
Copyright © 2019 Yadav, Mailar, Nagarajappa Masagalli, Chae, Song and Choi. This is an open-access article distributed under the terms of the Creative Commons Attribution License (CC BY). The use, distribution or reproduction in other forums is permitted, provided the original author(s) and the copyright owner(s) are credited and that the original publication in this journal is cited, in accordance with accepted academic practice. No use, distribution or reproduction is permitted which does not comply with these terms.
*Correspondence: Jae-Jun Song, ampzb25nMjNAZ21haWwuY29t; Won Jun Choi, bXA4OUBkb25nZ3VrLmVkdQ==
†These authors have contributed equally to this work.