- 1Department of Life Sciences, University of Siena, Siena, Italy
- 2Department of Biotechnology, Chemistry and Pharmacy, University of Siena, Siena, Italy
- 3Landcare Research, Lincoln, New Zealand
- 4School of Chemical Sciences, University of Auckland, Auckland, New Zealand
- 5Department of Pharmaceutical and Pharmacological Sciences, University of Padua, Padua, Italy
Norbormide is a toxicant selective for rats to which it induces a widespread vasoconstriction. In a recent paper, we hypothesized a role of ATP-sensitive potassium (KATP) channels in norbormide-induced vasoconstriction. The current study was undertaken to verify this hypothesis by comparing the effects of norbormide with those of glibenclamide, a known KATP channel blocker. The whole-cell patch-clamp method was used to record KATP currents in myocytes freshly isolated from the rat and mouse caudal artery and from the rat gastric fundus, as well as in insulin-secreting pancreatic beta cells (INS-1 cells). Smooth muscle contractile function was assessed on either rat caudal artery rings or gastric fundus strips. Molecular modeling and docking simulation to KATP channel proteins were investigated in silico. Both norbormide (a racemic mixture of endo and exo isomers) and glibenclamide inhibited KATP currents in rat and mouse caudal artery myocytes, as well as in gastric fundus smooth muscle cells. In rat INS-1 cells, only glibenclamide blocked KATP channels, whereas norbormide was ineffective. The inhibitory effect of norbormide in rat caudal artery myocytes was not stereo-specific as both the endo isomers (active as vasoconstrictor) and the exo isomers (inactive as vasoconstrictor) had similar inhibitory activity. In rat caudal artery rings, norbormide-induced contraction was partially reverted by the KATP channel opener pinacidil. Computational approaches indicated the SUR subunit of KATP channels as the binding site for norbormide. KATP channel inhibition may play a role in norbormide-induced vasoconstriction, but does not explain the species selectivity, tissue selectivity, and stereoselectivity of its constricting activity. The lack of effect in INS-1 cells suggests a potential selectivity of norbormide for smooth muscle KATP channels.
Introduction
Norbormide, a selective rat toxicant, induces a lethal effect in rats but has little or no effect in non-rat species, including humans, and for this reason, it has been marketed for many years as an eco-sustainable pesticide (Roszkowski, 1965). The mechanism/s underlying the toxic effect of norbormide in rats is/are not understood, although existing evidence supports the idea that this toxicant induces a marked and irreversible vasoconstriction of rat peripheral vessels by targeting a receptor abundantly and/or selectively expressed in the rat peripheral artery myocytes (Bova et al., 2001). Norbormide contractile effect is endothelium-independent (Bova et al., 1996) and is restricted to rat vascular smooth muscle (Bova et al., 2003). Intriguingly, in rat conduit arteries (i.e., aorta), in rat non-vascular muscles, and in all muscles from non-rat species, norbormide, at concentrations partially overlapping those that induce vasoconstriction in rats, shows vasorelaxant properties, attributed to an inhibitory effect on CaV1.2 channels (Bova et al., 2003).
From a chemical perspective, norbormide exists as a racemic mixture of up to eight stereoisomers, namely, four endo and four exo. Among them, only the endo isomers show rat-specific vasoconstrictor activity, being lethal to rats (Poos et al., 1966). Collectively, these observations indicate that the contractile activity of norbormide is not only species- and tissue-selective but also stereo-specific, thus suggesting the existence of a highly specific target for this compound in rat vessels.
KATP channels are a heterogeneous family of ion channels physiologically regulated by the intracellular concentration of ATP. They are composed of two subunits: the Kir subunit, forming the channel pore, and the SUR subunit, which binds ATP and exerts a regulatory role over the Kir subunit (Yokoshiki et al., 1998). Various isoforms of Kir and SUR exist and assemble in differing partnerships to form tissue-specific KATP channels (Shi et al., 2005). In vascular smooth muscle, sarcolemmal KATP channels play a pivotal role in the regulation of vessel tone and blood pressure. In particular, activation of these channels allows K+ efflux from the cytoplasm to the extracellular space with a net loss of positive charges. This leads to membrane hyperpolarization, CaV1.2 channel closure, cytoplasmic Ca2+ concentration decrease, and vasodilation (Ko et al., 2008; Foster and Coetzee, 2016). Conversely, KATP channel inhibition causes vasoconstriction. Compounds capable of activating KATP channels, e.g., pinacidil and cromakalim, are effective relaxant agents in vitro and show powerful hypotensive effects in vivo (Ashwood et al., 1986; Gollasch et al., 1995). In contrast, inhibition of vascular KATP channels contributes to the contractile response induced by receptor-coupled vasoconstrictor agents (Bonev and Nelson, 1996) regulating vascular tone, especially in resistance and coronary arteries (Tinker et al., 2014).
Glibenclamide is a sulfonylurea widely used as an antidiabetic agent (Rydén et al., 2014). In pancreatic beta cells, in fact, it blocks KATP channels, thus allowing Ca2+ to enter the cytoplasm and stimulate insulin secretion that, in turn, reduces blood glucose concentration (Ashcroft and Rorsman, 1989). Although glibenclamide displays high affinity for the pancreatic KATP channel isoform, it also affects KATP channels in other tissues and is therefore widely used in vitro as a pharmacological tool.
We have recently demonstrated that a fluorescent derivative of endo-norbormide (NRB-AF12) features a subcellular fluorescence profile similar to that of ER-Tracker®, a commercially available fluorescent derivative of glibenclamide (D’Amore et al., 2016; D’Amore et al., 2018; Forgiarini et al., 2019). This phenomenon occurred in several cell types, including freshly isolated rat caudal artery myocytes, where norbormide behaves as a contracting agent. Based on these results, we hypothesized that norbormide and glibenclamide can potentially bind to the same cellular target, namely, KATP channels, and that these channels can play a role in norbormide-induced vasoconstriction. This hypothesis was assessed in the present study by: i) studying norbormide effects on KATP currents recorded in vascular and non-vascular myocytes, as well as in INS-1 beta cells; ii) evaluating its functional activity on smooth muscle preparations; and iii) performing an in silico analysis to identify its potential binding site in KATP channels. In all these experiments, glibenclamide was used as a reference KATP inhibitor.
Materials and Methods
All animal care and experimental protocols conformed to the European Union Guidelines for the Care and Use of Laboratory Animals (European Union Directive 2010/63/EU) and had been approved by the Italian Department of Health (666/2015-PR, 650/2015, 41451.N.ZRS/2018).
Vasoconstriction Assay
Two-millimeter-long caudal artery rings were obtained from the main (ventral) caudal artery of male Wistar rats (160–230 g, Charles River Italia, Calco, Italy) anesthetized by CO2 and killed by decapitation.
The rings, cleaned of the adventitia, were mounted by inserting two 100-µm-thick tungsten wires into their lumen in home-made myographs, as previously described (Bova et al., 2003). The devices holding the rings were then immersed into 20-ml double-jacketed organ baths containing a salt solution of the following composition (in mM): NaCl 125, KCl 5, MgSO4 1, KH2PO4 1.2, CaCl2 2.7, NaHCO3 25, and glucose 11, pH 7.35, bubbled with a mixture of O2 (95%) and CO2 (5%), and maintained at 37°C. At the beginning of the experiment, a preload of 2 g (1 g/mm) was applied to each ring. The preloaded rings were left to equilibrate for at least 60 min and then repeatedly stimulated with 90 mM KCl and 10 µM phenylephrine until two consecutive reproducible contractile responses to each stimulus were obtained. The contractile force was measured through an isometric force transducer (2B Instruments, Milan, Italy) coupled to an analog-to-digital converter (PowerLab) and was displayed on the monitor of a PC. The experiments were conducted in rings mechanically deprived of the endothelium; the absence of a functional endothelium was confirmed by the lack of carbachol-induced relaxation of rings precontracted with phenylephrine.
Rat Fundus Assay
Gastric fundus strips were obtained from the stomach of male Wistar rats (200–250 g, Charles River Italia, Calco, Italy) anesthetized (i.p). with a mixture of Zoletil 100® (7.5 mg/kg tiletamine and 7.5 mg/kg zolazepam; Virbac Srl, Milano, Italy) and Xilor® (4 mg/kg xylazine; Bayer, Milan, Italy) containing heparin (5,000 U/kg), decapitated, and bled. The stomach was removed, opened along the longitudinal axis of the greater curvature and washed in cold Ca2+-free physiological salt solution (Ca2+-free PSS) containing the following (in mM): NaCl 118, KCl 4.7, KH2PO4 1.2, MgCl2 1.2, NaHCO3 25, and glucose 11.5. Smooth muscle strips (1–2 mm in width; 1.5–2 cm in length), dissected from the circular layer of the anterior fundus wall, were transferred into 25-ml organ bath chambers filled with Ca2+-free PSS, pH 7.4, bubbled with O2 (95%) and CO2 (5%) gas mixture, and maintained at 37°C. Strips, connected to isometric transducers (BLPR, WPI, Berlin, Germany), were stretched to a tension of 1 g. After equilibration for 15 min, 2.5 mM Ca2+ was added and the strips were allowed to develop stable, spontaneous tone over a 30-min period. Afterward, they were challenged with two consecutive stimulations with 60 mM K+ (K60), until a stable response was obtained. After 45 min of washing in PSS, either norbormide or glibenclamide was added and left in contact with tissues for 15 min, followed by a K60 stimulation to test for muscle functional integrity. Tension was expressed as a percentage of the initial response to K60, which was regarded as 100% (Fusi et al., 1998).
Electrophysiological Experiments
Cell Isolation Procedure From Rat and Mouse Tail Main Artery
Rat tail artery myocytes were obtained from the same rats used for the fundus assay (see above); mice tail artery myocytes were obtained from C57BL6 mice (20–25 g, Internal breeding of the Department of Pharmaceutical and Pharmacological Sciences of the University of Padua) killed by cervical dislocation. The tail artery was cut, cleaned of skin, and placed in external solution (containing in mM: 130 NaCl, 5.6 KCl, 10 4-(2-hydroxyethyl)-1-piperazineethanesulfonic acid (HEPES), 20 glucose, 1.2 MgCl2, and 5 Na-pyruvate; pH was adjusted to 7.4 with NaOH). Smooth muscle cells were freshly isolated from two mice arteries and a 5-mm-long piece of rat artery by incubation at 37°C for 40–45 min in 2 ml of 0.1 mM Ca2+ external solution containing 20 mM taurine (prepared by replacing NaCl with equimolar taurine), 1.25 mg/ml collagenase (type XI), 1 mg/ml soybean trypsin inhibitor, and 1 mg/ml bovine serum albumin. This solution was gently bubbled with an O2 (95%) and CO2 (5%) gas mixture to stir the enzyme solution, as previously described (Fusi et al., 2016). Cells were stored in 0.05 mM Ca2+ external solution containing 20 mM taurine and 0.5 mg/ml bovine serum albumin at 4°C under normal atmosphere, and were used within 2 days of isolation (Mugnai et al., 2014).
Cell Isolation Procedure From Rat Gastric Fundus
Gastric smooth muscle cells were freshly isolated from rat gastric fundus strips obtained from the same rats used for the fundus assay (see above). The strips were digested for 40–45 min at 37°C in 2 ml of nitrate-rich digestion solution (in mM: NaCl 55, NaNO3 65, KCl 5, Na-pyruvate 5, glucose 10, taurine 10, HEPES 10, and MgCl2 1.2; pH 7.4) containing 2 mg collagenase (type I), 2.5 mg bovine serum albumin, and 3 mg soybean trypsin inhibitor, bubbled with an O2 (95%) and CO2 (5%) gas mixture (Fusi et al., 2001).
Thereafter, cells were mechanically dispersed with a plastic pipette in a modified Kraft-bruhe (KB) solution [containing 1 mg bovine serum albumine and (in mM) NaCl 105, KH2PO4 7, KCl 5, glucose 5, taurine 10, HEPES 10, MgCl2 1.6, Na-pyruvate 2.5, creatine 1.7, oxalacetate 2, Na2ATP 1.5, and ethylene glycol-bis(2-aminoethylether)-N,N,N´,N´-tetraacetic acid (EGTA) 0.1; pH adjusted to 7.25 with NaOH] and used for experiments within 10 h of isolation. During this time, they were stored at 4°C in KB solution containing bovine serum albumin.
INS-1 Cells
INS-1 beta cells (Asfari et al., 1992), kindly provided by Dr. Andrea Venerando (Department of Comparative Biomedicine and Food Science, University of Padua, Italy), were grown in RPMI 1640 medium containing 11.1 mM D-glucose, 1 mM sodium pyruvate, 50 μM β-mercaptoethanol, 2 mM glutamine, 10 mM HEPES, 10% fetal calf serum (FCS), 100 U/ml penicillin, 100 μg/ml streptomycin, and 250 ng/ml amphotericin B at 37°C in humidified 5% CO2 and 95% air (Kittl et al., 2016). Cell cultures were passaged once a week using the standard trypsin/EDTA treatment.
Whole-Cell Patch-Clamp Recordings
Cells were continuously superfused with recording solution using a peristaltic pump (LKB 2132, Bromma, Sweden) at a flow rate of 400 μl/min.
The conventional whole-cell patch-clamp method was applied to voltage-clamp smooth muscle cells (Saponara et al., 2011). Recording electrodes were pulled from borosilicate glass capillaries (WPI, Berlin, Germany) and fire-polished to obtain a pipette resistance of 2–5 MΩ when filled with internal solution.
An Axopatch 200B patch-clamp amplifier (Molecular Devices Corporation, Sunnyvale, CA, USA) was used to generate and apply voltage pulses to the clamped cells and to record the corresponding membrane currents. At the beginning of each experiment, the junction potential between the pipette and bath solution was electronically adjusted to zero. Current signals, after compensation for whole-cell capacitance and series resistance (between 70% and 75%), were low-pass filtered at 2 kHz and digitized at 3 kHz prior to being stored on the computer hard disk. Electrophysiological responses were tested at room temperature (20–22°C).
The osmolarity of the external solution and that of the internal solution were measured with an osmometer (Osmostat OM 6020, Menarini Diagnostics, Florence, Italy) (Fusi et al., 2005).
Analysis of data was accomplished by using pClamp 9.2.1.8 software (Molecular Devices Corporation).
KATP Current Recording in Tail Artery and Gastric Myocytes
Recording solution contained the following (in mM): NaCl 25, KCl 140, HEPES 10, glucose 10, MgCl2 1, CaCl2 0.1, and tetraethylammonium (TEA) 1; pH was adjusted to 7.4 with NaOH (320 mosmol). Pipette solution consisted of the following (in mM): KCl 140, HEPES 10, EGTA 10, MgCl2 1, glucose 5, Na2ATP 0.1, KADP 1, and Na2GTP 0.1; pH was adjusted to 7.3 with KOH (290 mosmol). To minimize voltage-dependent K+ currents, KATP currents were recorded at a steady membrane potential (Vh) of −50 mV using a continuous gap-free acquisition protocol. Currents, activated by the KATP channel opener pinacidil (10 µM), did not run down during the following 10 min under these conditions (data not shown). Care was taken to complete each experiment within this period. Current values were corrected for leakage using 10 µM glibenclamide, which completely blocked KATP currents (Trezza et al., 2018).
Ca2+ is known to stabilize the cell membrane. Due to its low concentration in the recording solution, the seal between the pipette tip and the cell membrane was frequently lost a few minutes after breaking the patch to get the whole-cell configuration. Thus, only single concentrations of norbormide were assessed in each myocyte. Furthermore, as few cells did not respond to pinacidil stimulation, norbormide was always added after pinacidil had induced a stable current amplitude.
KATP Current Recording in INS-1 Cells
Recording solution contained the following (in mM): NaCl 140, KCl 5.6, CaCl2 2.5, MgCl2 1.5, HEPES 10, D-glucose 4.5, and mannitol 5; pH was adjusted to 7.4 with NaOH (300 mosmol). Pipette solution contained the following (in mM): potassium D-gluconate 120, NaCl 5, KCl 10, CaCl2 2, MgCl2 4, Mg2ATP 2, HEPES 5, EGTA 10, and raffinose 5; pH was adjusted to 7.2 with KOH.
KATP currents were recorded by means of an “activator-free” protocol, consisting in 500-ms pulses to −80 and −60 mV, delivered at 10-s intervals, from a Vh of −70 mV, as previously described (Kittl et al., 2016). Under these conditions, currents measured were almost entirely KATP currents, as proved by the 10 µM glibenclamide blockade. The latter was used to correct current values for leakage.
Chemicals
Endo- and exo-norbormide isomers were prepared at the University of Auckland, New Zealand. Endo-norbormide was synthesized as previously described (Brimble et al., 2004). The exo isomer was obtained from a solution of endo-norbormide (1.00 g, 1.95 mmol) in mixed xylenes (20 ml) heated at 140°C for 30 h, and the solvent was then removed in vacuo. Purification by flash chromatography (petroleum ether/ethyl acetate, 1:1) afforded exo-norbormide as a mixture of stereoisomers (R/S/T/X) (beige solid; 0.80 g, 1.56 mmol, 80%). 1H NMR (400 MHz, CDCl3) δ 2.89 (0.15H, dd, J = 7.3, 0.9 Hz, S/H-2), 2.92–2.95 (0.6H, m, T/H-2, X/H-2), 2.97 (0.25H, dd, J = 7.3, 0.9 Hz, R/H-2), 3.03 (0.45H, dd, J = 7.3, 1.1 Hz, X/H-3), 3.06 (0.15H, dd, J = 7.3, 1.1 Hz, S/H-3), 3.25 (0.15H, dd, J = 7.3, 1.1 Hz, T/H-3), 3.41 (0.25H, dd, J = 7.3, 1.1 Hz, R/H-3), 3.49–3.50 (0.15H, m, S/H-4), 3.73–3.74 (0.25H, m, R/H-1), 3.76–3.77 (0.6H, m, X/H-1, T/H-4), 3.95–3.96 (0.45H, m, X/H-4), 4.09–4.10 (0.25H, m, R/H-4), 4.33–4.35 (0.3H, m, S/H-1, T/H-1), 5.74–5.76 (0.4H, m, T/H-6, R/H-6), 5.80 (0.15H, s, S/OH), 5.86 (0.45H, s, X/OH), 6.15 (0.45H, dd, J = 3.4, 1.1 Hz, X/H-6), 6.18 (0.15H, s, T/OH), 6.19 (0.15H, dd, J = 3.4, 1.1 Hz, S/H-6), 6.51 (0.25H, s, R/OH), 6.85–7.65 (16H, m, Ar), 8.42–8.59 (2H, m, αPyr). RP-HPLC: tR = 45.7 (S), 51.7 (T), 58.7 (X), 61.1 (R) min (purity λ254nm = >99%). A 1H NMR spectrum and RP-HPLC chromatogram are reported in Supplementary Figure 1.
Collagenase (types I and XI), trypsin inhibitor, bovine serum albumin, TEA, EGTA, HEPES, taurine, pinacidil, glibenclamide, RPMI 1640, β-mercaptoethanol, glutamine, FCS, penicillin, streptomycin, and amphotericin B were resourced from Sigma Chimica (Milan, Italy). Norbormide (mixed endo and exo isomers) was a kind gift from I.N.D.I.A. Industrie Chimiche Srl, Padua, Italy. Endo- and exo-norbormide were synthesized at the School of Chemical Sciences, University of Auckland, New Zealand. Norbormide, pinacidil, and glibenclamide, dissolved directly in dimethyl sulfoxide (DMSO), were diluted at least 1,000 times prior to use. All solutions were stored at −20°C and protected from light by wrapping containers with aluminum foil. The resulting concentrations of DMSO (below 0.1%, v/v) had no influence on tissue or cell responses.
Statistical Analysis
Individual values, contributing to calculation of the groups’ mean ± SEM values, derived from independent cells, rings, or strips that, sometimes, were isolated from the same animal. To ensure that mean values are representative of the population, however, cells or rings included in the same group were isolated from at least three different animals.
Statistical analysis and significance, as measured by either repeated measures ANOVA (followed by Dunnett post hoc test) or Student’s t test for paired samples (two-tailed), were obtained using GraphPad Prism version 5.04 (GraphPad Software Inc., San Diego, CA, USA). Post hoc tests were performed only when ANOVA found a significant value of F and no variance in homogeneity. In all comparisons, P < 0.05 was considered significant.
Homology Model Building and Validation
The cryo-electron microscopy (cryo-EM) structure of the pancreatic β-cell KATP channel bound to ATP and glibenclamide, with Protein Data Bank identity (PDB) ID 6BAA (Martin et al., 2017), was used as the main template for the homology model (Protein Model Portal; Haas et al., 2013). The UniProt database (www.uniprot.org) sequences of Rattus norvegicus KATP channel characterized by Kir6.1 Q63664 and Kir6.2 P70673 subunits, together with the SUR1 Q09429 and SUR2 Q63563 subunits, obtained with ClustalX tool (Jeanmougin et al., 1998), were used for alignment. PyMOL plugin PyMod 2.0 (Janson et al., 2017) was used to achieve a protein structure prediction by means of ClustalO (Sievers et al., 2011). Sequence alignment between R. norvegicus Kir6.1 and Kir6.2 exhibited 82% query coverage and 74% identity with 0.0 E value, while that between R. norvegicus SUR1 and SUR2 showed a query coverage of 98% with an identity of 72.8% with 0.0 E value.
Based on those alignments, 3D models for target protein were generated by using Modeller integrated in Pymod 2.0. The quality of the modeled subunits (Kir6.1 and SUR2) was assessed using local factors such as packing quality, backbone conformation, bond length, and side-chain planarity. Structural superimposition between the Kir6.1 and SUR2 model with the cryo-EM structure of Kir6.2 and SUR1 (PDB ID 6BAA), as reported in Figure 1, showed a high structural similarity with backbone root-mean-square distance (RMSD) values of 0.57 and 1 Å, respectively. Structure validation indicated that the protein homology models of subunits Kir6.1 and SUR2 possessed reasonable 3D structure with a good stereochemical quality of 98.8% and 97% of the amino acid residues in the most favored regions, respectively, as assessed by Ramachandran plot. Energy minimization protocol in the simple point charge water model and the RMSD were computed for the KATP channel Kir6.1/SUR2 three-dimensional model by using GROMACS 2016.4 software package (Abraham et al., 2015).
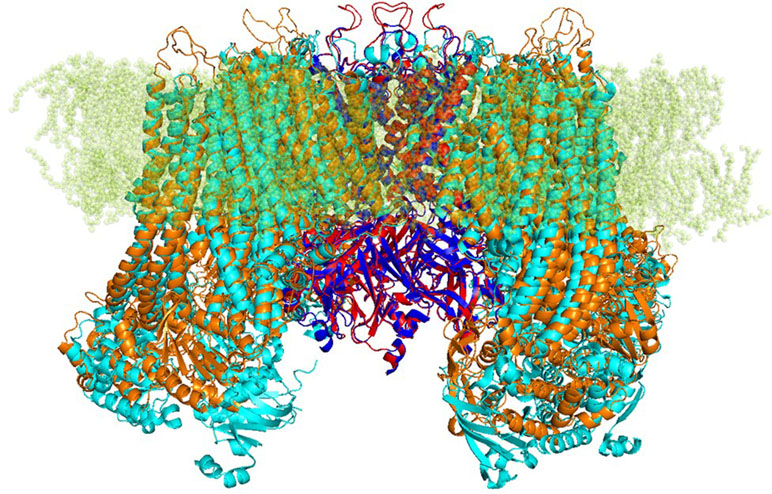
Figure 1 Strucutre of transmembrane KATP channels. Ribbon representation of superimposed Kir6.1 homology model (red) and Kir6.2 cryo-EM structure 6BAA (blue) complexed with SUR subunits, SUR2 homology model (orange), and SUR1 cryo-EM structure 6BAA (cyan).
Molecular Docking
The molecular structure of norbormide (10468605 ChemSpider ID) was acquired through ChemSpider in Mol format (www.chemspider.com). The norbormide and glibenclamide pdbqt format was generated by using Open Babel tools, adding Gasteiger charge (O’Boyle et al., 2011), whereas the pdbqt format of the proteins was generated using utility scripts included in the AutoDock tools graphical user interface. Norbormide was subjected to the steepest descent minimization (500 steps at 0.02-Å step size) to remove unfavorable clashes, followed by 100 steps of conjugate gradient minimization (0.02-Å step size; Pettersen et al., 2004). Docking simulation studies of ligands against the Kir6.1/SUR2 model and the Kir6.2/SUR1 cryo-EM structure (Martin et al., 2017) were performed using the flexible side-chain protocol based on Iterated Local Search Global Optimizer Algorithm of AutoDock/VinaXB (Koebel et al., 2016). Protein–ligand network interaction was evaluated with protein–ligand interaction profiler (PLIP) (Salentin et al., 2015). PyMOL 1.7.6.0 was used as the molecular graphics system (The PyMOL Molecular Graphics System, Version 1.8; Schrödinger, LLC, New York, NY, USA).
Results
Effects of Norbormide on KATP Currents in Vascular and Non-Vascular Single Cells
The effect of norbormide on KATP channels was assessed in vascular (myocytes freshly isolated from rat and mouse tail main artery) and non-vascular (myocytes freshly isolated from rat gastric fundus) single cells and single INS-1 cells. To limit activation of KV and KCa1.1 channels in the vascular and gastric myocytes, KATP currents were induced at a Vh of −50 mV in the presence of 0.1 mM ATP and 1 mM ADP in the pipette solution, and 1 mM TEA in the recording solution. In rat vascular myocytes, the KATP channel opener pinacidil (10 µM) activated an inward current that was significantly antagonized by the KATP channel blocker glibenclamide (10 µM; Figure 2A and B). Racemic norbormide (5 and 50 µM) inhibited, in a concentration-dependent manner, glibenclamide-sensitive currents recorded in the presence of pinacidil (Figure 2C and D). Of interest, we found that exo-norbormide (50 µM) and endo-norbormide (50 µM) had an inhibitory efficacy that was comparable to that of norbormide (50 µM) (Figure 3A and B). Norbormide (50 µM) also caused a significant reduction of KATP current in mice vascular (Figure 3C) and in rat gastric fundus myocytes (Figure 3D), similarly to that observed in rat vascular myocytes.
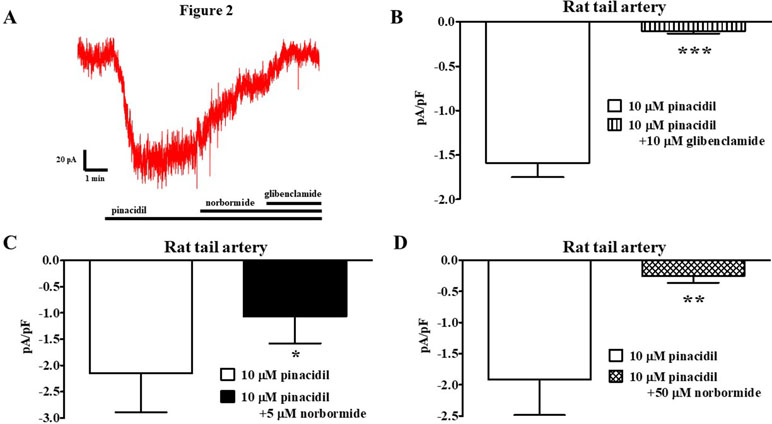
Figure 2 Effect of norbormide on KATP currents of isolated rat tail artery myocytes. (A) Representative whole-cell recordings of inward currents elicited by 10 µM pinacidil at a Vh of −50 mV. The effect of 5 µM norbormide as well as of 10 µM glibenclamide is shown. (B) Pinacidil (10 µM) activated glibenclamide-sensitive KATP currents, which were inhibited by either (C) 5 µM or (D) 50 µM norbormide. Columns are mean ± SEM (n = 5–7). *P < 0.05, **P < 0.01, ***P < 0.001, Student’s t test for paired samples.
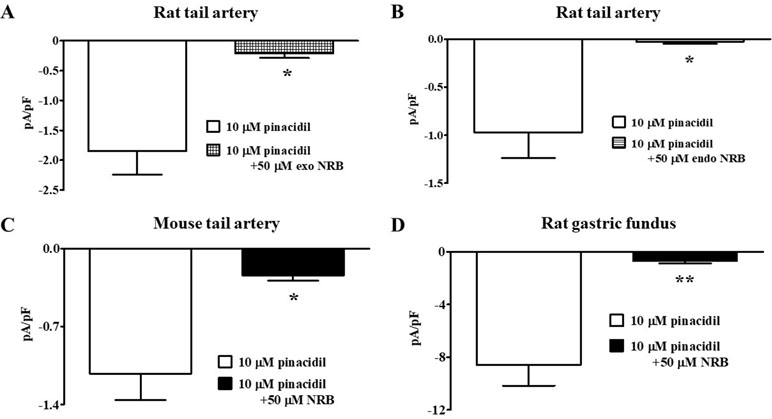
Figure 3 Effect of norbormide on KATP currents of isolated rat and mouse tail artery or rat fundus myocytes. (A and B) Pinacidil (10 µM) activated glibenclamide-sensitive rat tail artery myocytes KATP currents, which were inhibited by either (A) 50 µM exo-norbormide (NRB) or (B) 50 µM endo NRB. (C and D) Pinacidil (10 µM) activated glibenclamide-sensitive (C) mouse tail artery and (D) rat gastric fundus myocytes KATP currents, which were inhibited by 50 µM NRB. Columns are mean ± SEM (n = 4–5). *P < 0.05, **P < 0.01, Student’s t test for paired samples.
In direct contrast, norbormide (50 µM) did not affect KATP current recorded in INS-1 cells, which was significantly inhibited by glibenclamide (10 µM; Figure 4).
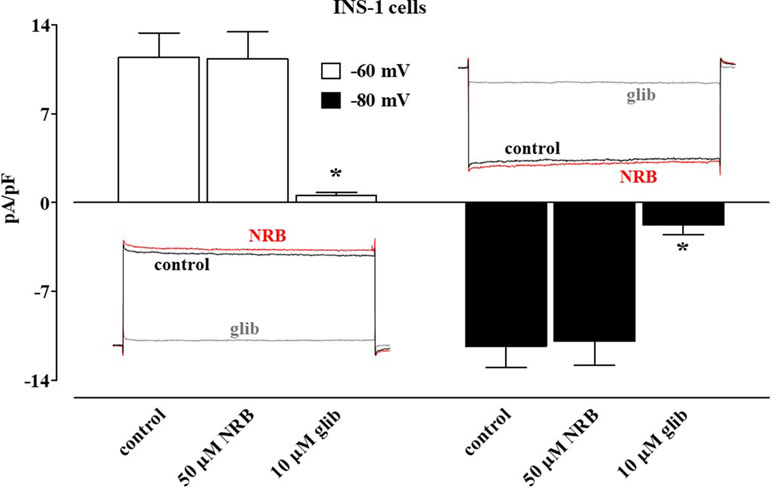
Figure 4 Effect of norbormide on KATP currents of INS-1 cell. KATP currents were recorded at a Vh of −70 mV during 500-ms pulses to −60 mV (white columns) and −80 mV (black columns) in the absence (control) or presence of 50 µM norbormide (NRB) as well as NRB plus 10 µM glibenclamide (glib). Columns are mean ± SEM (n = 7). Insets: average traces of original KATP currents, recorded from seven cells, during 500-ms pulses to −60 mV (left) or −80 mV (glib) in the absence (control) or presence of NRB as well as NRB plus glibenclamide. P < 0.001, ANOVA and Dunnett post test.
Effect of Norbormide and Glibenclamide on the Mechanical Activity of Rat Caudal Artery Rings and Fundus Strips
These experiments were undertaken to assess and compare the mechanical effects of norbormide and glibenclamide on rat caudal artery and fundus preparations.
In vascular rings, norbormide, at the same concentration (50 µM) that almost completely blocked KATP channels in rat caudal artery myocytes, produced a contractile response that was 140% of that induced by 90 mM KCl (Figure 5A and B). Norbormide-induced contraction was not affected by the same concentration of pinacidil (10 µM) used to activate KATP channels in patch-clamp experiments. However, when norbormide concentration was lowered to 5 µM, the addition of pinacidil indeed caused a 20% relaxation of the active tone (Figure 5C). Glibenclamide, up to 200 µM, did not modify basal tension in rat caudal artery rings (Figure 5D).
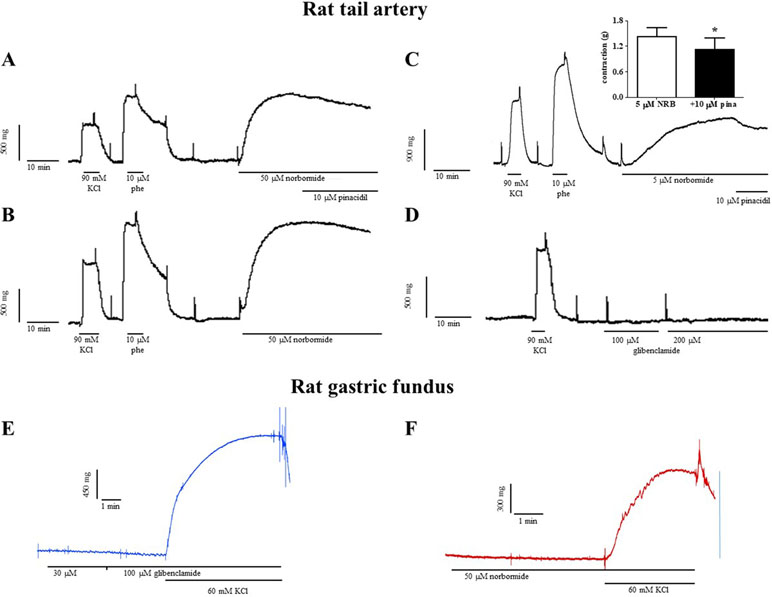
Figure 5 Effects of norbormide and glibenclamide on the resting tone of rat caudal artery rings and gastric fundus strips. (A–D) Rat caudal artery rings. (A) Original recording showing the contractile effect of a maximal concentration of norbormide (50 µM). The addition of 10 µM pinacidil did not affect active muscle tone (compared to panel B). (B) Control experiment, performed in parallel with that represented in (A), showing that norbormide-induced contraction spontaneously relaxes after the plateau phase, thus suggesting that the relaxation observed in (A) is not pinacidil-dependent. (C) Original recording showing the contractile effect of a low concentration of norbormide (5 µM). The addition of 10 µM pinacidil caused a partial relaxation of the active muscle tone. Inset: effect of pinacidil on rat caudal rings precontracted with 5 µM norbormide. On the ordinate scale, contraction is reported in g (n = 6; *P < 0.05, Student’s t test for paired samples). (D) Glibenclamide, up to 200 µM, was unable to affect ring resting tone. (A–D) The contraction induced by 90 mM KCl and/or 10 µM phenylephrine is also shown. Neither (E) glibenclamide nor (F) norbormide contracted rat gastric fundus strips. The effect of 60 mM KCl is also shown. Traces are representative of five (norbormide) or six (glibenclamide) similar experiments.
Fifty micromolar NRB-induced vasoconstriction was reverted upon washout in about 90 min (data not shown). Furthermore, the following addition of 10 µM phenylephrine or 90 mM KCl elicited contractile responses comparable to those recorded before NRB challenging (data not shown).
Both norbormide (50 µM) and glibenclamide (up to 100 µM) were unable to contract rat gastric fundus strips (Figure 5E and F). The subsequent addition of 60 mM KCl, however, elicited a contraction that measured 67.2 ± 10.8% (n = 5) and 90.5 ± 3.5% (n = 6) of that recorded in the absence of the drug, respectively.
Molecular Modeling and Docking Simulation
The predicted KATP 3D model was comparable to the 6BAA template (Martin et al., 2017). Norbormide (both endo and exo conformation) was found to be flexible and free to bind the two KATP channels, namely, Kir6.1/SUR2 in smooth muscle cells and Kir6.2/SUR1 in INS-1 cells. Following docking simulation, the best predicted binding interaction of norbormide (Figure 6A) and glibenclamide (Figure 6B) with the Kir6.1/SUR2 channel showed comparable thermodynamic affinities (−9.2 and −8.7 kcal/mol, respectively), while those towards the Kir6.2/SUR1 channel were different (−6.7 and −8.9 kcal/mol; Figure 6C and D). Protein–ligand interaction profiler (PLIP) tool results showed interaction network profiles with remarkable differences in the number and bond typologies, as reported in Figure 6 and Table 1.
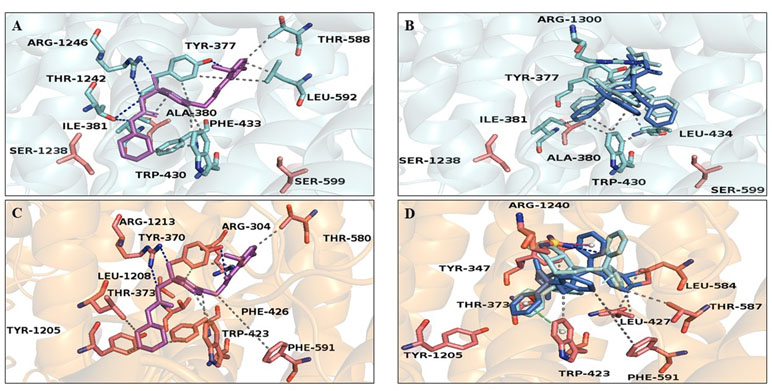
Figure 6 Glibenclamide and norbormide binding site. (A and B) Inhibited SUR2 cartoon structure (PDB accession number 6BAA) showing only the interactions between residues’ binding site (cyan sticks) and (A) glibenclamide (purple sticks) or (B) norbormide (blue sticks). (C and D) SUR1 cartoon model structure, showing only the interactions between residues’ binding site (orange sticks) and (C) glibenclamide (purple sticks) or (D) norbormide (blue sticks).
Structural analysis of SUR2–norbormide complex binding pocket residues revealed hydrophobic interactions, π-cationic, π-stacking, and hydrogen bonds, whereas, in the case of the SUR1–norbormide complex, the different amino acid composition prevented analogous interactions, as shown in Figure 7. In contrast, glibenclamide formed bonds, similar per number and typologies, with both SUR subunits, as shown in Figure 6 and Table 1.
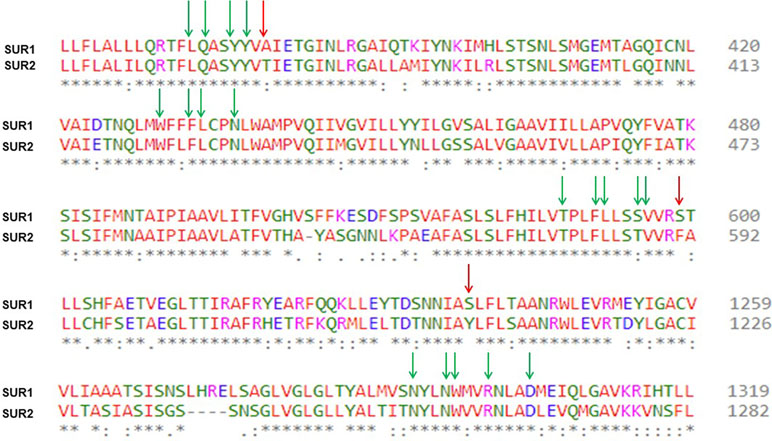
Figure 7 Sequence alignment results between R. norvegicus SUR2 and the template sequence of R. norvegicus SUR1. Identical and positively conserved amino acids (marked with a star and colon, respectively) are highlighted in colors according to the property of the corresponding query residue in the alignment. Green arrows highlight all the amino acids involved in glibenclamide and norbormide binding regions. Red arrows: non-conserved amino acids engaged in interaction network.
Discussion
A key finding of this study is that norbormide inhibits KATP current in myocytes freshly isolated from the rat caudal artery. This effect was concentration-dependent and was observed at norbormide concentrations overlapping those eliciting a slowly reversible, contractile effect in the rat caudal artery rings (see also Bova et al., 1996).
Pinacidil-induced KATP current was only partially antagonized by 5 µM norbormide and almost completely blocked by 10 times higher concentrations of the compound. Accordingly, pinacidil failed to counteract the contraction induced by 50 µM norbormide and partly reverted that elicited by a 10 times lower concentration of the compound. Though these data point to KATP channel blockade as the mechanism underlying norbormide-induced vasoconstriction, other observations presented in this study suggest that this activity is only partially responsible for norbormide vasotonic effect and that there are likely other mechanisms involved. In fact, norbormide-induced KATP current inhibition could be observed not only with endo-specific norbormide (vasoactive), but also with exo-specific norbormide, known to be inactive as a vasoconstrictor and non-toxic to rats (Poos et al., 1966). Furthermore, KATP current inhibition could also be demonstrated in myocytes freshly isolated from mouse caudal artery and from rat stomach, i.e., from smooth muscles that are not contracted, but rather relaxed, by norbormide (Bova et al., 2003). Taken together, these findings indicate that the norbormide-induced block of KATP channels is not sufficient to trigger the contractile process.
Glibenclamide inhibited KATP current in all the smooth muscle cells investigated, though it was unable to induce contraction in the corresponding whole tissues, i.e., rat caudal artery rings and fundus strips, even at concentrations much higher than those required to block KATP current. However, not all the arteries are insensitive to glibenclamide: several reports show that this drug causes constriction of coronary arteries in various animal species, including rat (Bril et al., 1992; Imamura et al., 1992; Jackson et al., 1993). In this regard, it is worth noting that norbormide is also endowed with a strong coronaro-constrictor activity, which is observed only in rats (Roszkowski, 1965).
On the one hand, the results of the present study suggest that glibenclamide and norbormide share several functional and electrophysiological properties in vascular and non-vascular smooth muscles. Previous studies also revealed other similarities between the two drugs, i.e., activation of the mitochondrial permeability transition pore (Ricchelli et al., 2005; Skalska et al., 2005; Zulian et al., 2011) and inhibition of CaV1.2 channels (Bian and Hermsmeyer, 1994; Fusi et al., 2002). The latter is fascinating since it apparently contrasts to the blockade of KATP channels (usually causing membrane potential to depolarize and CaV1.2 channels to open) and the corresponding vasoconstrictor activity. On the other hand, norbormide and glibenclamide differ, as the former did not affect KATP channel currents of INS-1 cells. This observation suggests a possible selectivity of norbormide towards smooth muscle KATP channels and indicates that channel inhibition operated by norbormide and glibenclamide is driven by a different mechanism of action. This hypothesis is further supported by the docking results.
The structural analysis of the protein–ligand complex was initially focused on a sequence-conserved, cytoplasmic, physical gate site called G loop (Hibino et al., 2010) that plays a crucial role in the regulation of KATP gating kinetics (Shimomura et al., 2009; Li et al., 2016; Lu et al., 2016). Docking simulation of norbormide against either Kir6.1 or Kir6.2 G loop revealed that the compound was unable to dock these binding pockets, owing to its steric hindrance. Instead, a blind docking simulation indicated that norbormide bound KATP channel in the same pocket of glibenclamide (Martin et al., 2017), though with different interaction profiles and binding energy affinities, which seem to be crucial for their different mechanism of action.
Blockade of KATP channels, mediated by PKC activation, characterizes the activity of several vasoconstrictors (Bonev and Nelson, 1996; Liu and Khalil, 2018). Noticeably, norbormide-induced contraction of rat caudal artery is accompanied by the activation of the PLC–PKC pathway (Bova et al., 2001). However, neither mouse caudal artery nor rat vascular and gastric smooth muscle was contracted by exo-specific norbormide, although it is capable to block KATP channels. Therefore, the involvement of PKC in KATP channel current inhibition operated by norbormide and its isomers can be ruled out.
In conclusion, the selective rat toxicant norbormide is a KATP channel blocker likely exerting a direct effect on the channel protein. This activity can, at least in part, underline the vasoconstrictor effect observed in the rat tail artery. In addition, the lack of effect of norbormide on KATP channels in INS-1 cells may suggest a potential selectivity of this compound towards smooth muscle cell channels.
Data Availabilty Statement
All datasets generated for this study are included in the manuscript and/or the supplementary files.
Ethics Statement
All animal care and experimental protocols conformed to the European Union Guidelines for the Care and the Use of Laboratory Animals (European Union Directive 2010/63/EU), and had been approved by the Italian Department of Health (666/2015-PR, 650/2015, 41451.N.ZRS/2018).
Author Contributions
SB, SS, and FF conceived the study and performed the experiments. MB, DR, and BH synthesized norbormide isomers. OS and AT performed the in silico analysis. SB, SS, FF, OS, and AT analyzed and interpreted the data. SB, SS, DR, and BH wrote the manuscript. All authors approved the final version of the manuscript.
Funding
This project was supported by the New Zealand Ministry of Business, Innovation and Employment’s Endeavor Fund C09X1710 (BH, SB, MB, and DR) and by the University of Padua, project no. 148125/14 (SB).
Conflict of Interest Statement
The authors declare that the research was conducted in the absence of any commercial or financial relationships that could be construed as a potential conflict of interest.
Supplementary Material
The Supplementary Material for this article can be found online at: https://www.frontiersin.org/articles/10.3389/fphar.2019.00598/full#supplementary-material
References
Abraham, M. J., Murtola, T., Schulz, R., Páll, S., Smith, J. C., Hess, B., et al. (2015). GROMACS: high performance molecular simulations through multi-level parallelism from laptops to supercomputers. SoftwareX 1–2, 19–25. doi: 10.1016/j.softx.2015.06.001
Asfari, M., Janjic, D., Meda, P., Li, G., Halban, P. A., Wollheim, C. B. (1992). Establishment of 2-mercaptoethanol-dependent differentiated insulin-secreting cell lines. Endocrinology 130, 167–178. doi: 10.1210/endo.130.1.1370150
Ashcroft, F. M., Rorsman, P. (1989). Electrophysiology of the pancreatic beta-cell. Prog. Biophys. Mol. Biol. 54, 87–143. doi: 10.1016/0079-6107(89)90013-8
Ashwood, V. A., Buckingham, R. E., Cassidy, F., Evans, J. M., Faruk, E. A., Hamilton, T. C., et al. (1986). Synthesis and antihypertensive activity of 4-(cyclic amido)-2H-1-benzopyrans. J. Med. Chem. 29, 2194–2201. doi: 10.1021/jm00161a011
Bian, K., Hermsmeyer, K. (1994). Glyburide actions on the dihydropyridine-sensitive Ca2+ channel in rat vascular muscle. J. Vasc. Res. 31, 256–264. doi: 10.1159/000159051
Bonev, A. D., Nelson, M. T. (1996). Vasoconstrictors inhibit ATP-sensitive K+ channels in arterial smooth muscle through protein kinase C. J. Gen. Physiol. 108, 315–323. doi: 10.1085/jgp.108.4.315
Bova, S., Cavalli, M., Cima, L., Luciani, S., Saponara, S., Sgaragli, G., et al. (2003). Relaxant and Ca2+ channel blocking properties of norbormide on rat non-vascular smooth muscles. Eur. J. Pharmacol. 470, 185–191. doi: 10.1016/S0014-2999(03)01797-7
Bova, S., Trevisi, L., Cima, L., Luciani, S., Golovina, V., Cargnelli, G. (2001). Signaling mechanisms for the selective vasoconstrictor effect of norbormide on the rat small arteries. J. Pharmacol. Exp. Ther. 296, 458–463.
Bova, S., Trevisi, L., Debetto, P., Cima, L., Furnari, M., Luciani, S., et al. (1996). Vasorelaxant properties of norbormide, a selective vasoconstrictor agent for the rat microvasculature. Br. J. Pharmacol. 117, 1041–1046. doi: 10.1111/j.1476-5381.1996.tb16694.x
Bril, A., Laville, M. P., Gout, B. (1992). Effects of glibenclamide on ventricular arrhythmias and cardiac function in ischaemia and reperfusion in isolated rat heart. Cardiovasc. Res. 26, 1069–1976. doi: 10.1093/cvr/26.11.1069
Brimble, M. A., Muir, V. J., Hopkins, B., Bova, S. (2004). Synthesis and evaluation of vasoconstrictor and vasorelaxant activity of norbormide isomers. ARKIVOC i, 1–11. doi: 10.3998/ark.5550190.0005.101
D’Amore, C., Orso, G., Forgiarini, A., Ceolotto, G., Rennison, D., Ribaudo, G., et al. (2018). Synthesis and biological characterization of a new norbormide derived bodipy FL-conjugated fluorescent probe for cell imaging. Front. Pharmacol. 9, 1055. doi: 10.3389/fphar.2018.01055
D’Amore, C., Orso, G., Fusi, F., Pagano, M. A., Miotto, G., Forgiarini, A., et al. (2016). An NBD derivative of the selective rat toxicant norbormide as a new probe for living cell imaging. Front. Pharmacol. 7, 315. doi: 10.3389/fphar.2016.00315
Forgiarini, A., Wang, Z., D’Amore, C., Jay-Smith, M., Li, F. F., Hopkins, B., et al. (2019). Live applications of norbormide-based fluorescent probes in Drosophila melanogaster. PLoS One 14, e0211169. doi: 10.1371/journal.pone.0211169
Foster, M. N., Coetzee, W. A. (2016). KATP channels in the cardiovascular system. Physiol. Rev. 96, 177–252. doi: 10.1152/physrev.00003.2015
Fusi, F., Manetti, F., Durante, M., Sgaragli, G., Saponara, S. (2016). The vasodilator papaverine stimulates L-type Ca(2+) current in rat tail artery myocytes via a PKA-dependent mechanism. Vascul. Pharmacol. 76, 53–61. doi: 10.1016/j.vph.2015.11.041
Fusi, F., Saponara, S., Gagov, H., Sgaragli, G. P. (2001). Effects of some sterically hindered phenols on whole-cell Ca2+ current of guinea pig gastric fundus smooth muscle cells. Br. J. Pharmacol. 132, 1326–1332. doi: 10.1038/sj.bjp.0703935
Fusi, F., Saponara, S., Sgaragli, G., Cargnelli, G., Bova, S. (2002). Ca(2+) entry blocking and contractility promoting actions of norbormide in single rat caudal artery myocytes. Br. J. Pharmacol. 137, 323–328. doi: 10.1038/sj.bjp.0704877
Fusi, F., Sgaragli, G., Saponara, S. (2005). Mechanism of myricetin stimulation of vascular L-type Ca2+ current. J. Pharmacol. Exp. Ther. 313, 790–797. doi: 10.1124/jpet.104.080135
Fusi, F., Valoti, M., Petkov, G. V., Boev, K. K., Sgaragli, G. P. (1998). Myorelaxant activity of 2-t-butyl-4-methoxyphenol (BHA) in guinea pig gastric fundus. Eur. J. Pharmacol. 360, 43–50. doi: 10.1016/S0014-2999(98)00660-8
Gollasch, M., Bychkov, R., Ried, C., Behrendt, F., Scholze, S., Luft, F. C., et al. (1995). Pinacidil relaxes porcine and human coronary arteries by activating ATP-dependent potassium channels in smooth muscle cells. J. Pharmacol. Exp. Ther. 275, 681–692.
Haas, J., Roth, S., Arnold, K., Kiefer, F., Schmidt, T., Bordoli, L. (2013). The Protein Model Portal—a comprehensive resource for protein structure and model information. Database (Oxford) 2013, bat031. doi: 10.1093/database/bat031
Hibino, H., Inanobe, A., Furutani, K., Murakami, S., Findlay, I., Kurachi, Y. (2010). Inwardly rectifying potassium channels: their structure, function, and physiological roles. Physiol. Rev. 90, 291–366. doi: 10.1152/physrev.00021.2009
Imamura, Y., Tomoike, H., Narishige, T., Takahashi, T., Kasuya, H., Takeshita, A. (1992). Glibenclamide decreases basal coronary blood flow in anesthetized dogs. Am. J. Physiol. 263, 399–404. doi: 10.1152/ajpheart.1992.263.2.H399
Jackson, W. F., König, A., Dambacher, T., Busse, R. (1993). Prostacyclin-induced vasodilation in rabbit heart is mediated by ATP-sensitive potassium channels. Am. J. Physiol. 264, 238–243. doi: 10.1152/ajpheart.1993.264.1.H238
Janson, G., Zhang, C., Prado, M. G., Paiardini, A. (2017). PyMod 2.0: Improvements in protein sequence-structure analysis and homology modeling within PyMOL. Bioinformatics 33, 444–446. doi: 10.1093/bioinformatics/btw638
Jeanmougin, F., Thompson, J. D., Gouy, M., Higgins, D. G., Gibson, T. J. (1998). Multiple sequence alignment with Clustal X. Trends Biochem. Sci. 23, 403–405. doi: 10.1016/S0968-0004(98)01285-7
Kittl, M., Beyreis, M., Tumurkhuu, M., Fürst, J., Helm, K., Pitschmann, A., et al. (2016). Quercetin stimulates insulin secretion and reduces the viability of rat INS-1 beta-cells. Cell. Physiol. Biochem. 39, 278–293. doi: 10.1159/000445623
Ko, E. A., Han, J., Jung, I. D., Park, W. S. (2008). Physiological roles of K+ channels in vascular smooth muscle cells. J. Smooth Muscle Res. 44, 65–81. doi: 10.1540/jsmr.44.65
Koebel, M. R., Schmadeke, G., Posner, R. G., Sirimulla, S. (2016). AutoDock VinaXB: implementation of XBSF, new empirical halogen bond scoring function, into AutoDock Vina. J Cheminform. 8, 27. doi: 10.1186/s13321-016-0139-1
Li, N., Wu, J. X., Ding, D., Cheng, J., Gao, N., Chen, L. (2016). Structure of a pancreatic ATP sensitive potassium channel. Cell 168, 101–110. doi: 10.1016/j.cell.2016.12.028
Lu, Y., Zhong, C., Wang, L., Wei, P., He, W., Huang, K., et al. (2016). Optogenetic dissection of ictal propagation in the hippocampal–entorhinal cortex structures. Nat. Commun. 21, 10962. doi: 10.1038/ncomms10962
Liu, Z., Khalil, R. A. (2018). Evolving mechanisms of vascular smooth muscle contraction highlight key targets in vascular disease. Biochem. Pharmacol. 153, 91–122. doi: 10.1016/j.bcp.2018.02.012
Martin, G. M., Kandasamy, B., DiMaio, F., Yoshioka, C., Shyng, S. L. (2017). Anti-diabetic drug binding site in a mammalian K(ATP) channel revealed by cryo-EM. Elife 6, e31054. doi: 10.7554/eLife.31054
Mugnai, P., Durante, M., Sgaragli, G., Saponara, S., Paliuri, G., Bova, S., et al. (2014). L-type Ca(2+) channel current characteristics are preserved in rat tail artery myocytes after one-day storage. Acta Physiol. (Oxford) 211, 334–345. doi: 10.1111/apha.12282
O’Boyle, N. M., Banck, M., James, C. A., Morley, C., Vandermeersch, T., Hutchison, G. R. (2011). Open Babel: an open chemical toolbox. J. Cheminform. 3, 33. doi: 10.1186/1758-2946-3-33
Pettersen, E. F., Goddard, T. D., Huang, C. C., Couch, G. S., Greenblatt, D. M., Meng, E. C., et al. (2004). UCSF Chimera—a visualization system for exploratory research and analysis. J. Comput. Chem. 25, 1605–1612. doi: 10.1002/jcc.20084
Poos, G. I., Mohrbacher, R. J., Carson, E. L., Paragamian, V., Puma, B. M., Rasmussen, C. R., et al. (1966). Structure–activity studies with the selective rat toxicant norbormide. J. Med. Chem. 9, 537–540. doi: 10.1021/jm00322a021
Ricchelli, F., Dabbeni-Sala, F., Petronilli, V., Bernardi, P., Hopkins, B., Bova, S. (2005). Species-specific modulation of the mitochondrial permeability transition by norbormide. Biochim. Biophys. Acta 1708, 178–186. doi: 10.1016/j.bbabio.2005.03.002
Roszkowski, A. P. (1965). The pharmacological properties of norbormide, a selective rat toxicant. J. Pharmacol. Exp. Ther. 149, 288–299.
Rydén, L., Grant, P. J., Anker, S. D., Berne, C., Cosentino, F., Danchin, N., et al. (2014). Task Force on diabetes, pre-diabetes, and cardiovascular diseases of the European Society of Cardiology (ESC); European Association for the Study of Diabetes (EASD), ESC guidelines on diabetes, pre-diabetes, and cardiovascular diseases developed in collaboration with the EASD. Diab. Vasc. Dis. Res. 11, 133–173. doi: 10.1177/1479164114525548
Salentin, S., Schreiber, S., Haupt, V. J., Adasme, M. F., Schroeder, M. (2015). PLIP: fully automated protein–ligand interaction profiler. Nucleic Acids Res. 43, W443–W447. doi: 10.1093/nar/gkv315
Saponara, S., Carosati, E., Mugnai, P., Sgaragli, G., Fusi, F. (2011). The flavonoid scaffold as a template for the design of vascular Ca(v) 1.2 channels modulators. Br. J. Pharmacol. 164, 1684–1697. doi: 10.1111/j.1476-5381.2011.01476.x
Skalska, J., Debska, G., Kunz, W. S., Szewczyk, A. (2005). Antidiabetic sulphonylureas activate mitochondrial permeability transition in rat skeletal muscle. Br. J. Pharmacol. 145, 785–791. doi: 10.1038/sj.bjp.0706214
Shi, N. Q., Ye, B., Makielski, J. C. (2005). Function and distribution of the SUR isoforms and splice variants. J. Mol. Cell. Cardiol. 39, 51–60. doi: 10.1016/j.yjmcc.2004.11.024
Shimomura, K., Flanagan, S. E., Zadek, B., Lethby, M., Zubcevic, L., Girard, C. A., et al. (2009). Adjacent mutations in the gating loop of Kir6.2 produce neonatal diabetes and hyperinsulinism. EMBO Mol. Med. 1, 166–177. doi: 10.1002/emmm.200900018
Sievers, F., Wilm, A., Dineen, D. G., Gibson, T. J., Karplus, K., Li, W., et al. (2011). Fast, scalable generation of high-quality protein multiple sequence alignments using Clustal Omega. Mol. Syst. Biol. 7, 539. doi: 10.1038/msb.2011.75
Tinker, A., Aziz, Q., Thomas, A. (2014). The role of ATP-sensitive potassium channels in cellular function and protection in the cardiovascular system. Br. J. Pharmacol. 171, 12–23. doi: 10.1111/bph.12407
Trezza, A., Cicaloni, V., Porciatti, P., Langella, A., Fusi, F., Saponara, S., et al. (2018). From in silico to in vitro: a trip to reveal flavonoid binding on the Rattus norvegicus Kir6.1 ATP-sensitive inward rectifier potassium channel. Peer J. 2 (6), e4680. doi: 10.7717/peerj.4680
Yokoshiki, H., Sunagawa, M., Seki, T., Sperelakis, N. (1998). ATP-sensitive K+ channels in pancreatic, cardiac, and vascular smooth muscle cells. Am. J. Physiol. 274, 25–37. doi: 10.1152/ajpcell.1998.274.1.C25
Zulian, A., Sileikytė, J., Petronilli, V., Bova, S., Dabbeni-Sala, F., Cargnelli, G., et al. (2011). The translocator protein (peripheral benzodiazepine receptor) mediates rat-selective activation of the mitochondrial permeability transition by norbormide. Biochim. Biophys. Acta 1807, 1600–1605. doi: 10.1016/j.bbabio.2011.08.007
Keywords: norbormide, glibenclamide, KATP channel, patch-clamp, molecular modeling, docking
Citation: Saponara S, Fusi F, Spiga O, Trezza A, Hopkins B, Brimble MA, Rennison D and Bova S (2019) The Selective Rat Toxicant Norbormide Blocks KATP Channels in Smooth Muscle Cells But Not in Insulin-Secreting Cells. Front. Pharmacol. 10:598. doi: 10.3389/fphar.2019.00598
Received: 15 February 2019; Accepted: 09 May 2019;
Published: 23 May 2019.
Edited by:
Elisabetta Cerbai, University of Florence, ItalyReviewed by:
Domenico Tricarico, University of Bari Aldo Moro, ItalyTim Murphy, University of New South Wales, Australia
Copyright © 2019 Saponara, Fusi, Spiga, Trezza, Hopkins, Brimble, Rennison and Bova. This is an open-access article distributed under the terms of the Creative Commons Attribution License (CC BY). The use, distribution or reproduction in other forums is permitted, provided the original author(s) and the copyright owner(s) are credited and that the original publication in this journal is cited, in accordance with accepted academic practice. No use, distribution or reproduction is permitted which does not comply with these terms.
*Correspondence: Fabio Fusi, ZmFiaW8uZnVzaUB1bmlzaS5pdA==