- 1Centre for Stem Cells in Cancer and Ageing, Barts Cancer Institute, Queen Mary University of London, London, United Kingdom
- 2Traslational Research Unit, Hospital Universitario Miguel Servet, Aragon Institute for Health Research (IIS Aragon), Zaragoza, Spain
Cancer heterogeneity constitutes the major source of disease progression and therapy failure. Tumors comprise functionally diverse subpopulations, with cancer stem cells (CSCs) as the source of this heterogeneity. Since these cells bear in vivo tumorigenicity and metastatic potential, survive chemotherapy and drive relapse, its elimination may be the only way to achieve long-term survival in patients. Thanks to the great advances in the field over the last few years, we know now that cellular metabolism and stemness are highly intertwined in normal development and cancer. Indeed, CSCs show distinct metabolic features as compared with their more differentiated progenies, though their dominant metabolic phenotype varies across tumor entities, patients and even subclones within a tumor. Following initial works focused on glucose metabolism, current studies have unveiled particularities of CSC metabolism in terms of redox state, lipid metabolism and use of alternative fuels, such as amino acids or ketone bodies. In this review, we describe the different metabolic phenotypes attributed to CSCs with special focus on metabolism-based therapeutic strategies tested in preclinical and clinical settings.
Introduction
Cancer is a highly heterogeneous disease, not only in terms of variability among patients, but also within a single tumor. This heterogeneity constitutes the main cause for therapy resistance and cancer progression in some patients (Hanahan and Weinberg, 2011). We can find different levels of intratumor heterogeneity. First, a tumor is comprised of multiple genotypes, which belong to distinct subclones with diverse features, which may include differential morphology and/or functionality. Additionally, tumors (and the subclones within) are formed of a functionally heterogeneous cell population, where a particular subset of tumor cells have the ability to initiate and propagate tumor growth, survive chemotherapy and drive relapse (Phillips et al., 2006; Diehn et al., 2009; Labelle et al., 2011; Hu et al., 2012; Rhim et al., 2012; Touil et al., 2014; Li et al., 2015b). These cells, the so-called cancer stem cells (CSCs), have self-renewal capacity, and can give rise to a differentiated progeny, leading to the production of all cell types present within a tumor, thereby generating tumor heterogeneity through a differentiation hierarchy (Reya et al., 2001). This distinct population was initially identified in leukemia, but was also found in solid tumors, such as breast, lung, prostate, colon, brain, head and neck, liver, as well as in pancreatic ductal adenocarcinoma (PDAC) (Hermann et al., 2007; Castellanos et al., 2013; Du et al., 2017). Finally, non-cancer cells present in the tumor microenvironment constitute a third level of heterogeneity, since they can directly affect cancer cell plasticity and functionality (Malanchi and Huelsken, 2009; Batlle and Clevers, 2017).
Cancer stem cells can be originated either from a mutation of a normal stem cell or from differentiated cells acquiring stem-like abilities (Reya et al., 2001). Indeed, numerous studies have found an abnormal activation of stem cell regulatory genes and pathways in the CSCs population, such as c-MYC, Bmi-1, Hedgehog, Notch and Wnt (Somervaille et al., 2009; Sancho et al., 2015; Wang et al., 2016). Apart from the well-known developmental pathways such as Wnt, Hedgehog or Jagged, metabolic traits have recently been involved in governing the function of stem cells. Indeed, although stem cells are primarily glycolytic, acquisition of certain metabolic plasticity, together with an increase in oxidative metabolism, primes them for maturation and supports their lineage differentiation (Cho et al., 2006; Chen et al., 2008; Simsek et al., 2010). A parallel mechanism was also postulated for CSCs in different tumors (Dong et al., 2013; Shen et al., 2015; Chen C.L. et al., 2016). However, recent data indicate that CSCs may mainly depend on oxidative metabolism (Sancho et al., 2015). In any case, reported metabolic differences between CSCs and progenies introduce another source of heterogeneity within tumors: metabolic heterogeneity. The latter can be further amplified, since different CSCs subclones can bear different metabolic phenotypes (Gammon et al., 2013) as a result of genetic or microenvironmental factors (Guha et al., 2014; Raj et al., 2015; Sancho et al., 2016).
Cancer (Stem) Cell Metabolism
One of the main cancer characteristics is uncontrolled growth and cell division. To support the abnormal survival and growth, cancer cells need to increase nutrient uptake to supply biosynthesis pathways (Vander Heiden et al., 2009; Kamphorst et al., 2015; Hensley et al., 2016). To achieve that, cancer cells usually modulate the activity of different metabolic pathways in order to produce metabolic precursors to satisfy energetic and anabolic demands, and maintain redox balance (Vazquez et al., 2016). Due to the crucial contribution of diverse metabolic pathways to malignant transformation and tumor progression, metabolic reprogramming recently became one of the cancer hallmarks (Hanahan and Weinberg, 2011).
Aerobic Glycolysis
The best example of reprogrammed metabolism in cancer is aerobic glycolysis (De Berardinis and Chandel, 2016): fast-proliferating tumor cells increase their glucose uptake to produce lactate in the presence of oxygen. This cancer hallmark was discovered by Otto Warburg and, thus, named the Warburg effect (Warburg et al., 1927; Warburg, 1956; Shim et al., 1998; Vander Heiden et al., 2009; Cairns et al., 2011). Glycolysis intermediates are used in diverse reactions to support high proliferation rates. For example, glucose-6-phosphate (G6P) can be used in the pentose phosphate pathway (PPP) to produce NADPH (Horton, 2002; Porporato et al., 2011; Doherty et al., 2014; Liberti and Locasale, 2016) or generate ribose groups, necessary for the synthesis of nucleotides (Lane and Fan, 2015; Vazquez et al., 2016). Alternatively, glycolytic intermediates can be used for anabolic reactions of glycogen or lipid synthesis (Gatenby and Gillies, 2004; Kroemer and Pouyssegur, 2008).
Glycolysis also facilitates survival and fast adaptation to the typically hypoxic tumor environment avoiding toxic Reactive Oxygen Species (ROS) accumulation through both low ROS production and increased detoxification systems (Brand and Hermfisse, 1997; Cairns et al., 2011; Doherty et al., 2014; Liberti and Locasale, 2016). Moreover, favoring glycolysis may bring other advantages to tumor cells, such as creating an acidic environment that can help invasion and suppress the immune response (Fischer et al., 2007; Swietach et al., 2007).
Even though aerobic glycolysis is quite inefficient in terms of ATP production, the rate of glucose uptake can be significantly elevated in cancer cells, resulting in ATP production to levels usually achievable with oxidative phosphorylation (OxPhos) (Liberti and Locasale, 2016). Additionally, although it was originally postulated that aerobic glycolysis is irreversible for tumor cells after cell division (Zu and Guppy, 2004; Jose et al., 2011; Porporato et al., 2011), it is well accepted nowadays that most cancers still produce ATP via OxPhos and modulate the contribution of both pathways in response to environmental factors or in different phases of the cell cycle (Smolková et al., 2011; De Berardinis and Chandel, 2016).
Importantly, glycolytic metabolism supports stemness in normal stem cells and CSCs of several cancer types (Folmes et al., 2011) (Table 1). Indeed, recent pieces of evidence demonstrate the involvement of oncogenes and pluripotency transcription factors, such as MYC, p53, K-Ras, HIF1α, NANOG, MEIS1, Wnt or OCT4 in the metabolic reprogramming from oxidative-dependent metabolism to a glucose dependence in many types of cancer (reviewed in Gabay et al., 2014; Alptekin et al., 2017; Deshmukh et al., 2018). Different studies support the glycolysis dependence of CSCs in diverse types of cancer, such as in radioresistant nasopharyngeal carcinoma spheres with high expression of stage-specific embryonic antigen (SSEA) -3 and -4 compared to parental cells (Shen et al., 2015), CD133+ human hepatocellular carcinoma cells and mouse models (Song et al., 2015; Chen C.L. et al., 2016), ALDH+ (aldehyde dehydrogenase) non-small lung carcinoma cells and side population (SP) cells from human colon cancer (Liu et al., 2014).
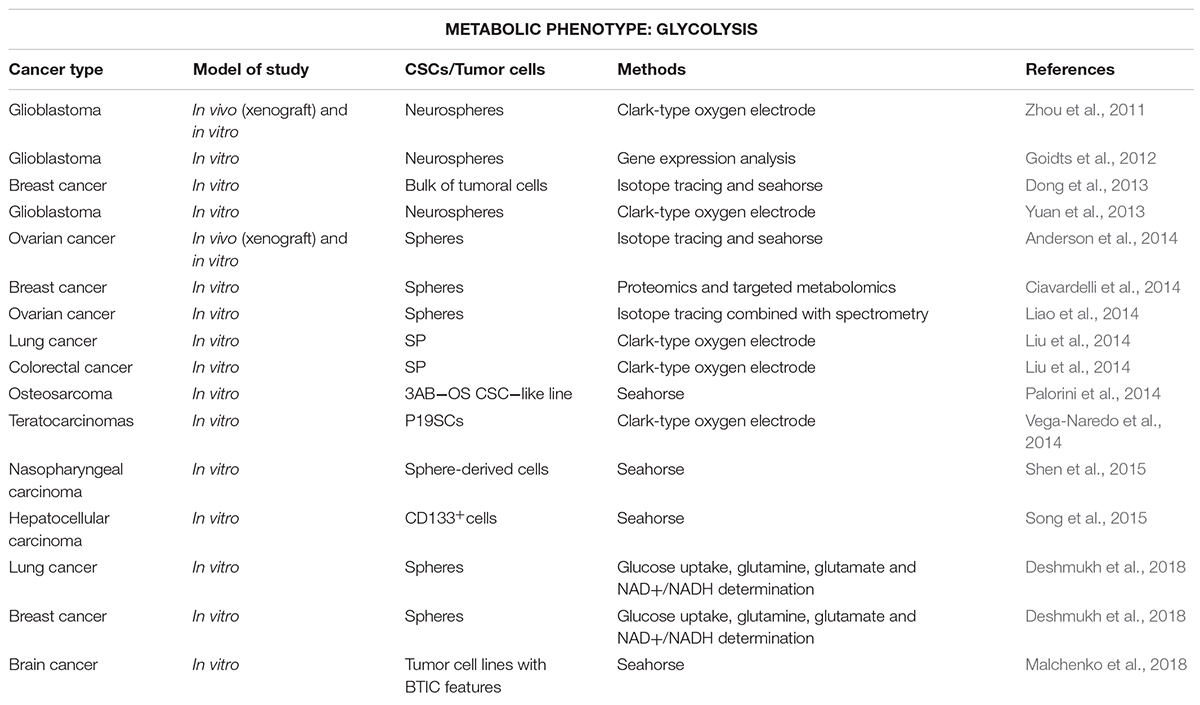
Table 1. Stem-like cells with glycolytic metabolism for various cancer types (in chronological order).
On the other hand, enhanced glycolysis in CSCs could also constitute a secondary response to maintain energy balance, since reduction of mitochondrial metabolism seems to be essential for full stemness in some cancer types, such as osteosarcoma or glioblastoma (Zhou et al., 2011; Yuan et al., 2013; Palorini et al., 2014). Indeed, the downregulation of mitochondrial genes was associated with enhanced increased expression of genes related to epithelial-mesenchymal transition (EMT) usually linked to stemness (Gaude and Frezza, 2016). Importantly, such inverse relationship was functionally proven in embryonal carcinoma cells derived from teratocarcinomas, since the stimulation of mitochondrial function induced cell differentiation and loss of pluripotency (Vega-Naredo et al., 2014). In fact, the occurrence of this metabolic switch, not the final glycolytic phenotype, seems to be key for early state of tumorigenesis and acquisition of stemness-related properties in human mammospheres and brain CSCs in a mouse model of primitive neuroectodermal tumors (Dong et al., 2013; Ciavardelli et al., 2014; Malchenko et al., 2018).
Mitochondrial Metabolism
Mitochondria play a key role in eukaryotic cells coordinating energy production and distribution through OxPhos based on oxygen and substrate availability, although other important metabolic reactions such as fatty acid oxidation (FAO), glutaminolysis, or reductive carboxylation in cells with damaged mitochondria also take place in these organelles. Mitochondrial tricarboxylic acid (TCA) cycle is primarily fueled by acetyl-CoA produced by glycolysis (from pyruvate) or FAO. Alternatively, in highly glycolytic cells, such as Ras-mutant cells, glutamine can be the driving force for OxPhos (Fan et al., 2013) through its conversion to α-ketoglutarate and oxaloacetate, that can be then used for fatty acids (FAs) and nucleotide synthesis (Gaglio et al., 2011). Electron donors produced in the TCA cycle are used by the electron transport chain (ETC) to create a proton motive force to synthesize ATP by the complex V.
As opposed to what we summarized in the previous section, recent literature described OxPhos as the main source of energy in CSCs from a number of cancer types (Table 2). This has been convincingly shown for ROSlow quiescent CD34+ leukemia CSCs (Lagadinou et al., 2013), lung spheroids and CD133+ PDAC cells (Ye et al., 2011; Sancho et al., 2015), as well as CSCs-enriched spheroids form ovarian, cervical and papillary thyroid carcinoma that displayed a reprogrammed metabolism through TCA cycle (Sato et al., 2016; Caria et al., 2018). Since mitochondrial metabolism coupled to OxPhos constitutes a much more efficient energy process, CSCs relying on OxPhos would theoretically make a better use of limited nutrients, which is an important advantage to survive in nutritionally poor environments. Indeed, mitochondria-dependent CD44+CD117+ ovarian CSCs and CD133+ PDAC CSCs showed enhanced resistance to glucose or glutamine deprivation compared to their differentiated counterparts (Pasto et al., 2014; Sancho et al., 2015). On the other hand, a variety of metabolites released by stromal cells can be used by OxPhos-dependent cells to fuel the TCA cycle, conferring them with increased adaptability to the changing conditions of the tumor microenvironment (Anderson et al., 2014). The best known example is lactate uptake from hypoxic tumor cells or cancer-associated fibroblasts (CAFs) via monocarboxylate transporter 1 and 2 (MCT1 and MCT2) in a process known as reverse Warburg effect (Kroemer and Pouyssegur, 2008; Sonveaux et al., 2008; Porporato et al., 2011; Rattigan et al., 2012). Moreover, pancreatic stellate cells release alanine to fuel the TCA cycle and subsequent biosynthetic pathways in pancreatic cancer cells (Sousa et al., 2016). Additionally, recent evidence indicate that microvesicles found in the tumor microenvironment contain several metabolites, including aminoacids, lipids and TCA cycle intermediates to fuel central metabolism of oxidative tumor cells and, consequently, tumor growth (Santi et al., 2015; Zhao et al., 2016). In this sense, stromal cells would play a key role in tumor progression supporting OxPhos-dependent CSCs proliferation and survival in nutrient-deprived environments.
Even though Warburg hypothesized that mitochondrial respiration defects are responsible for cancer cells shifting to glycolysis, it is known today that cancer cells still retain mitochondrial functions and that, in fact, a significant amount of ATP is produced through OxPhos (Tang et al., 2011; Kang et al., 2014; Zong et al., 2016). Indeed, ATP from OxPhos proved to be important for cell movements and invasive/metastatic abilities of cancer (stem) cells (Yu et al., 2017), suggesting that mitochondria contributes to cytoskeletal alterations (Caino et al., 2015). Moreover, OxPhos activation in metastatic breast cancer models is crucial to escape from the metabolic dormancy derived from hormonal therapy (Sansone et al., 2016). Interestingly, OxPhos activation is caused by the horizontal transfer of mitochondrial DNA (mtDNA) in exosomes from CAFs to dormant CSCs, providing a possible mechanism to development of resistance to hormonal therapy and highlighting metabolic interaction between CSCs and their niche (Sansone et al., 2017).
Beyond energy production, mitochondria are involved in controlling cellular redox rate, ROS generation, calcium buffering and the synthesis of intermediate molecules, such as acetyl-CoA and pyrimidines. Additionally, mitochondria have a crucial role in apoptosis initiation through activation of the membrane permeability transport pore, and release of cytochrome C (Wallace, 2012). Furthermore, mitochondria may contribute to malignant transformation and tumor progression through increased ROS production by the ETC (Ishikawa et al., 2008; Liou et al., 2016), abnormal accumulation of specific mitochondrial oncometabolites modifying epigenetic signals (Sciacovelli et al., 2013), and functional deficits in apoptosis (Tomiyama et al., 2006; Izzo et al., 2016). For all these reasons, mitochondrial biogenesis is essential for survival and propagation of CSCs regardless of their metabolic phenotype (Bonuccelli et al., 2010; De Luca et al., 2015; De Francesco et al., 2018). In fact, mitochondrial biogenesis may be a primary driver of stemness since its inhibition efficiently eliminated hypoxic spheroids in breast cancer (Lamb et al., 2015b,c; De Francesco et al., 2017).
The mechanisms driving mitochondrial biogenesis and OxPhos in CSCs described above have not been fully characterized yet, although some studies shed some light on this matter. In fact, findings in glioblastoma spheroids demonstrated the role of the oncofetal insulin-like growth factor 2 mRNA-binding protein 2 (Imp2) in the regulation of OxPhos, and mitochondrial biogenesis and structure (Janiszewska et al., 2012). Interestingly, the metabolic profile and plasticity of PDAC CD133+ cells rely on the balance between the MYC-driven glycolysis and the main regulator of the mitochondrial biogenesis peroxisome proliferator-activated receptor gamma coactivator 1-alpha (PGC-1α) (Sancho et al., 2015). In this study, differentiated PDAC cells exhibited an overexpression of MYC that counteracted stemness maintenance through a negative regulation of PGC-1α. These results apparently contradict the role of MYC as driver of stemness via glycolysis previously exposed, which may be due to a cell context-dependent modulation of stemness/differentiation. On the other hand, PGC-1α overexpression can also lead to different outcomes depending on the cellular context in BRAF driven melanomas: increased PGC-1α expression in primary tumors after BRAF inhibition with vemurafenib causes OxPhos addiction associated with poor patient prognosis (Haq et al., 2013), while it impaired growth rate and invasive abilities in metastatic settings (Luo et al., 2016).
Redox Regulation
It is well known that oncogenic transformation (Trachootham et al., 2009; Zhou et al., 2014), dysfunctional mitochondria (Kudryavtseva et al., 2016) and altered cell signaling (Tachibana et al., 2008; Raza et al., 2017) induce ROS accumulation in cancer cells, further promoting tumorigenesis and mutagenesis. However, due to the potential deleterious effects of ROS, a powerful antioxidant machinery formed of both enzymatic and non-enzymatic antioxidants is often found in cancers (Obrador et al., 2002; Townsend and Tew, 2003; Arnold et al., 2004; Estrela et al., 2006; Valko et al., 2006; Du et al., 2013; Harris et al., 2015; Raza et al., 2017).
Increasing evidence suggests an important role of ROS and redox signaling for CSCs functionality. It was known that quiescent stem cells reside in a low ROS niche that supports their stemness characteristics, like self-renewal capacity. On the other hand, increased ROS content promote stem cell proliferation and differentiation (Ito et al., 2006; Jang and Sharkis, 2007; Naka et al., 2008; Owusu-Ansah and Banerjee, 2009; Yahata et al., 2011; Bigarella et al., 2014). Only recently, it was shown that CSCs share the same redox-related properties (Diehn et al., 2009; Kobayashi and Suda, 2012; Yuan et al., 2015): murine CD44+CD24-/lowLin- and human Thy1+CD24+Lin- breast CSCs (Diehn et al., 2009; Luo et al., 2018), CD44high gastrointestinal cell lines (Ishimoto et al., 2011), tumorigenic ROSlow from head and neck carcinoma cell lines (Chang et al., 2018), human or murine CD133+ glioblastoma cells from cell lines and tumors and chronic myeloid leukemia (CML) CD34+ CSCs (Zhao et al., 2009; Qiang et al., 2012; Zhu et al., 2013) maintain low levels of intracellular ROS coupled to enhanced antioxidant capacity. Apart from stemness maintenance, bearing high antioxidant capacity grants CSCs resistance to ROS inducers, such as chemo and radiotherapy (Diehn et al., 2009; Izumiya et al., 2012; Wang et al., 2017b).
Redox balance can also be achieved through the regulation of ROS-dependent signaling pathways and redox-sensitive transcription factors, such as c-MYC, HIF1α, p53, NF-κB, AP-1, and the master regulator of antioxidant response, nuclear factor erythroid 2–related factor 2 (NRF2) (Chandel et al., 2000; Kamata et al., 2005; Soriano et al., 2009; Liou and Storz, 2010; Boyer-Guittaut et al., 2014; Jiang et al., 2014; Raza et al., 2017). In fact, CSCs regulate ROS levels via antioxidant transcription factors, such as NRF2 or FOXO (Diehn et al., 2009; Zhu et al., 2013; Wu T. et al., 2015; Ryoo et al., 2016; Chang et al., 2018; Luo et al., 2018). Most of these factors affect redox homeostasis by direct or indirect modulation of cellular metabolism. Indeed, NRF2 upregulation in different cancer types (Mitsuishi et al., 2012; Abdul-Aziz et al., 2015; Kim and Keum, 2016; Rocha et al., 2016; Milkovic et al., 2017) influences the switch between anabolic/catabolic glucose metabolism (Mitsuishi et al., 2012; Wallace, 2012; Heiss et al., 2013; Hawkins et al., 2016). In addition, the proto-oncogene c-MYC controls both cellular metabolism and redox homeostasis by increasing glycolysis (Ellwood-Yen et al., 2003; Kim et al., 2007; Wang et al., 2008; Miller et al., 2012; He et al., 2015; Davis-Yadley et al., 2016; Massihnia et al., 2017), and regulating glutamine metabolism (Anderton et al., 2017). Interestigly, both mechanisms could be interconnected, since c-MYC binds to the NRF2 promoter (Levy and Forman, 2010).
Importantly, most of the main signaling pathways governing CSCs functionality are regulated by ROS signaling. That is the case of stemness-regulatory pathways, such as Wnt and Notch (Takubo et al., 2010; Qiang et al., 2012; Paul et al., 2014), or key signaling nodes important for cell survival and growth, such as PTEN (Xia et al., 2013), PI3K (Le Belle et al., 2011), AKT (Zhou et al., 2007; Dey-Guha et al., 2011), ATM (Ito et al., 2004; Yalcin et al., 2008), STAT3 (Qiang et al., 2012; Zhang et al., 2016), and mammalian target of rapamycin (mTOR) (Dubrovska et al., 2009) and their downstream targets. Moreover, those pathways further modulate ROS production/detoxification in a positive feedback loop by activating redox-sensitive transcription factors (Miyamoto et al., 2007; Tothova et al., 2007; Dubrovska et al., 2009; Yeo et al., 2013; Zhang et al., 2016).
Lipid Metabolism
Besides the classical metabolic reprogramming related to glucose, alterations in diverse aspects of lipid metabolism are increasingly gaining attention as determinants of cancer, including CSCs function. In fact, highly proliferating cells require increased amounts of the cell membrane’s main components: lipids and cholesterol. In that cellular location, lipids function as either membrane building blocks or signaling transduction modifiers, since membrane lipid composition modulates protein recruitment and interaction (lipid rafts) (Rysman et al., 2010; Staubach and Hanisch, 2011). In this sense, several reports indicate that CSCs accumulate unsaturated lipids, such as monounsaturated FAs (MUFAs), the precursors of several plasma membrane lipids. In fact, lipid desaturation, mainly via the enzyme stearoyl-CoA desaturase (SCD-1), plays essential functions controlling self-renewal and tumorigenicity in different cancer models (Noto et al., 2013, 2017; Lai et al., 2017; Li F. et al., 2017), possibly through the activation of stemness-related pathways, such as Wnt signaling (Lai et al., 2017). Additionally, differences in plasma membrane lipid composition between CSCs and their differentiated counterparts have been reported, which can be potentially used to identify CSCs. Indeed, even though CSCs may present an overall decrease in glycosphingolipids as described for the glioblastoma CSC-like cell line GSC11 (He et al., 2010), the expression of specific gangliosides, such as GD2 and GD3, identified cells with increased self-renewal capacity and tumorigenicity in breast cancer (Battula et al., 2012; Liang et al., 2013).
Lipids and cholesterol in tumors are either scavenged from exogenous sources or synthesized de novo through FA synthase (FASN) or the mevalonate pathway, respectively (Beloribi-Djefaflia et al., 2016). Thus, different reports suggest that elevated de novo synthesis of lipids and cholesterol contribute to CSCs properties and survival. In fact, the expression of sterol regulatory element-binding protein 1 (SREBP1), master controller of de novo lipogenesis, is increased in CD24-CD44+ESA+ cells from a ductal carcinoma in situ cell line as well as mammospheres and melanospheres (Pandey et al., 2013; Corominas-Faja et al., 2014; Giampietri et al., 2017). This transcription factor may be involved in resistance to hypoxia and nutrient scarce environments, as suggested for glioblastoma sphere-derived cells (Lewis et al., 2015). Moreover, de novo lipogenesis from glycolytic intermediates or acetate via FASN is critical for in vitro self-renewal (Corominas-Faja et al., 2014; Yasumoto et al., 2016), and tumor relapse and metastatic dissemination after withdrawal of anti-angiogenic treatment (Sounni et al., 2014). In the same line of evidence, the activation of the mevalonate pathway is important for self-renewal and tumor formation in breast and pancreatic cancer, as well as glioblastoma (Ginestier et al., 2012; Brandi et al., 2017; Wang et al., 2017a).
Although de novo synthesis has traditionally been considered the preferred source of FAs for tumor cells (Ookhtens et al., 1984), recent reports highlight the crucial role of FAs uptake via CD36 or FA binding proteins (Hale et al., 2014; Pascual et al., 2016). The same is also true for cholesterol uptake within lipoproteins (Guillaumond et al., 2015). Indeed, lipid uptake, either via lipoprotein receptors or CD36, favors proliferation of glioma CD133+ cells (Hale et al., 2014) and label-retaining/CD44+ cells from squamous cell carcinoma (Pascual et al., 2016). Interestingly, increased lipid uptake points to the crucial role of microenvironment supporting cancer (stem) cell functions: tumor-activated adipocytes provide FAs to support leukemia CD34+ cells growth, survival and chemoresistance (Ye et al., 2016; Shafat et al., 2017) as well as omental metastasis from ovarian cancer (Nieman et al., 2011).
Fatty acids require covalent modification by CoA by fatty acyl-CoA synthetases to enter the bioactive pool of FAs. Afterward, they will be further esterified to form triacylglycerols or sterol esters and stored in lipid droplets (LDs). Importantly, recent reports correlate accumulation of LDs or stored cholesteryl-ester with tumor progression and aggressiveness (Yue et al., 2014; Guillaumond et al., 2015). In fact, activated and stored lipids play a crucial role supporting tumorigenicity of CSCs in vivo, as demonstrated in cells derived from neurospheres from glioblastoma and ALDH+ CD133+ ovarian cancer cells (Sun et al., 2014; Menard et al., 2016; Li J. et al., 2017). This may be a reflect of adaption to the harsh conditions found in the tumor microenvironment, since those lipids can be mobilized upon metabolic stress, providing ATP via FAO to ensure survival (Maan et al., 2018). Importantly, increased lipid storage in LDs may constitute a useful CSCs marker, as demonstrated in colorectal (CRC) and ovarian cancer (Tirinato et al., 2015; Li J. et al., 2017).
Activated FAs are not only incorporated into membranes or storage, but also used as substrate to synthesize signaling lipids or energy production in FAO. Although FAO is considered the main energy source in non-glycolytic tumors (Liu et al., 2010; Caro et al., 2012), a high activity of this pathway has been reported for aggressive tumor cells and CSCs, especially in nutrient scarce environments (Table 3) (Carracedo et al., 2013; Kamphorst et al., 2013; Daniëls et al., 2014; Pasto et al., 2014). In fact, ATP production and survival of matrix-deprived epithelial cells depend on FAO (Schafer et al., 2009; Carracedo et al., 2012), a metabolic process that also sustains the self-renewal capacity in both leukemia-initiating CFSEhighCD34+ cells and hematopoietic long-term culture initiating cells (Samudio et al., 2010; Ito et al., 2012). Besides its well-known role in energy production, FAs metabolism via mitochondrial FAO regulates multiple functions of CSCs. Indeed, FAO contributes to pluripotency maintenance and chemoresistance (Wang T. et al., 2018), mainly by reducing ROS production (Lee et al., 2015; Chen et al., 2016) and may sustain metastatic properties of sphere-derived cells (Aguilar et al., 2016).
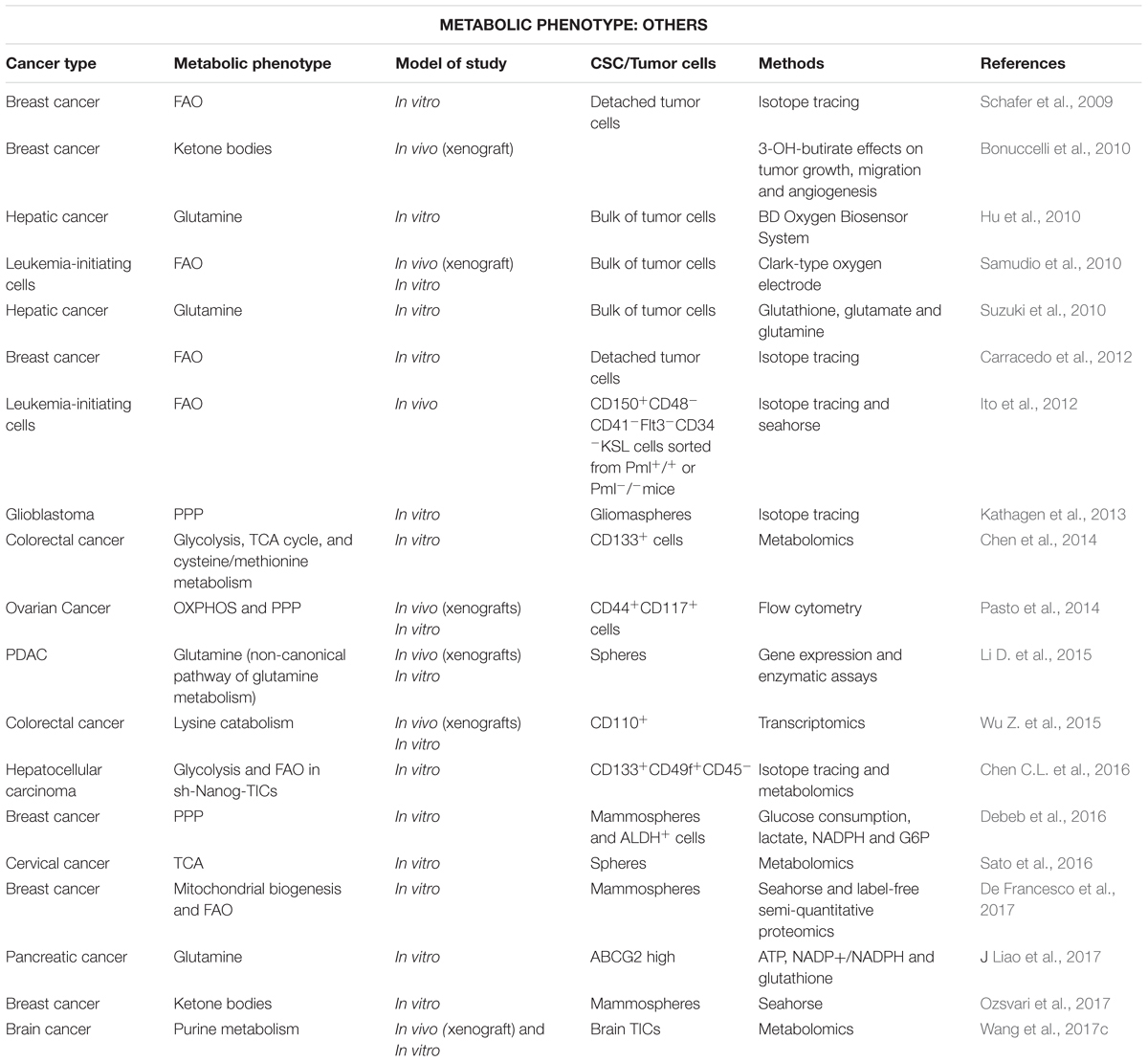
Table 3. Stem-like cells using alternative metabolism for various cancer types (in chronological order).
Finally, lipids can also regulate CSCs functionality in terms of self-renewal and tumorigenic abilities through their function as second messengers in signal transduction pathways, thus becoming potential therapeutic targets. Indeed, sphingolipids, such as sphingosine-1-P (S1P), eicosanoids, such as prostaglandin E2 or glycerophospholipids, such as lysophosphatidic acid (LPA), have been reported to increase CSCs proliferation and in vivo tumorigenicity, activating self-renewal and survival signaling pathways (Notch, AKT, NF-kB) in ALDH1+ from breast cancer, label-retaining cells in bladder cancer, CD133+CD44+ cells in CRC and sphere-derived cells from ovarian cancer (Hirata et al., 2015; Kurtova et al., 2015; Wang et al., 2015; Seo et al., 2016).
Alternative Fuels
Cancer cells require the use of amino acids for their heightened metabolic needs. Indeed, one of the most important metabolic pathways for cancer cells is that related to glutamine (Wise and Thompson, 2010), since it is an important substrate for DNA and fatty acid synthesis, as well as anaplerosis of the TCA cycle. Indeed, glutamine addiction has become a hallmark of glycolytic tumors, especially those with increased c-MYC expression (Deberardinis and Cheng, 2010; Wise and Thompson, 2010; Korangath et al., 2015). In addition, glutamine is related to glutathione synthesis, well known for its powerful antioxidant ability and some other biological activities (Todorova et al., 2004; Son et al., 2013). Although OxPhos-dependent pancreatic CD133+ CSCs are resistant to glutamine deprivation (Sancho et al., 2015), evidence of the involvement of glutamine metabolism in the maintenance of the stem-like SP phenotype has been provided in lung and pancreatic cancer by a β-catenin/redox-mediated mechanism (Liao et al., 2017). In fact, glutamine deprivation in pancreatic cancer cell lines inhibited their self-renewal capacity, reduced their stemness gene signature and increased sensitivity to radiotherapy (Li D. et al., 2015). Additionally, aminoacid metabolism, especially glutamine, is increased in acute myeloid leukemia (AML) ROSlow CSCs to fuel OxPhos and favor survival (Jones et al., 2018). Interestingly, leukemia CSCs may obtain their glutamine supply from neighbor stromal cells, as described for bone marrow adipocytes supporting cancer cells growth after asparaginase treatment in high-risk leukemia patients (Ehsanipour et al., 2013).
Apart from glutamine, the metabolism of amino acids, such as lysine or serine may also support CSCs features. Indeed, CRC CD110+ tumor-initiating cells (TICs) are rich in enzymes implicated in both lysine transport and catabolism, which activates β-catenin-dependent Wnt signaling, ultimately promoting self-renewal and metastasis (Wu Z. et al., 2015). Additionally, accumulation of alpha-aminoadipate, an intermediate of lysine catabolism, on brain TICs correlates with poor survival rate of glioblastoma patients, representing a marker of tumor aggressiveness (Rosi et al., 2015). On the other hand, recent data demonstrate that phosphoglycerate dehydrogenase (PHGDH), catalyzing the first step of the serine biosynthesis, maintains self-renewal and tumorigenicity of lung, breast and brain CD133high sphere-forming cells in a mechanism involving pluripotency gene expression and redox balance (Samanta et al., 2016; Sharif et al., 2018). Finally, endogenous tryptophan derivatives, such as Kyn (kynurenine) and ITE (2-(10H-indole-30- carbonyl)-thiazole-4-carboxylic acid methyl ester), may play opposite roles on cancer progression and stemness, regulating OCT4 expression through aryl hydrocarbon receptor (AhR) modulation: accumulation of the low-affinity AhR agonist Kyn in the tumor microenvironment favor carcinogenesis, whereas the high-affinity AhR agonist ITE promotes its binding to the OCT4 promoter to suppress its transcription and, consequently, inducing cell differentiation in U87 glioblastoma neurospheres (Cheng et al., 2015).
Ketone bodies can also work as fuel to promote tumor growth and play a role in CSCs activity. Reports on breast cancer showed the role of ketone bodies increasing the expression of stemness-related genes, driving recurrence and metastasis, thus related to decreased patient survival (Bonuccelli et al., 2010; Martinez-Outschoorn and Lisanti, 2014).
The PPP has also come up as an alternative way to generate energy in CSCs. For instance, glioblastoma stem-like cells are remarkably metabolically flexibles, switching their metabolism depending on oxygen levels fluctuations: from high levels of PPP activity linked to active proliferation under acute oxygenation, to a glucose-dependent phenotype under hypoxia, when cell migration is stimulated (Kathagen et al., 2013). Additionally, Debeb et al. (2016) described that PPP inhibitors reduced the stemness-related markers in node-positive invasive breast carcinoma and a high rate in PPP activity was also reported in combination with an OxPhos-dependent phenotype in CD44+CD117+ CSCs from epithelial ovarian cancer (Pasto et al., 2014).
Overproduction of hyaluronan, a component of the extracellular microenvironment, supports self-renewal in human head and neck squamous cell carcinoma HSC-3 cells (Bourguignon et al., 2012) and dedifferentiation in breast cancer cells (Chanmee et al., 2014). Using metabolomic approaches, Chanmee et al. (2016) later described that increased hyaluronan production leads to a HIF1α-induced metabolic reprogramming toward glycolysis, thus creating a positive feedback loop through the hexosamine biosynthetic pathway (HBP). Interestingly, HBP inhibition considerably reduced the content of CD44highCD24low cells and mammosphere-forming capacity.
Finally, purine metabolism has also been described to regulate stemness-related properties. Indeed, upregulation of MYC-mediated de novo purine synthesis maintained self-renewal, proliferation and tumor forming capacity in brain TICs, and was associated with poor prognosis in glioblastoma patients (Wang et al., 2017c).
Considerations on the Metabolic Heterogeneity of CSCs
As inferred from the information above, CSCs display a plethora of metabolic phenotypes diverging from the classical OxPhos/Warburg phenotypes (Table 3). However, such diversity cannot be completely attributed to the intrinsic heterogeneity of cancer, since conflicting data can be often found in the literature even for the same tumor entity.
The main source of reported disparities is the utilized model systems. On the one hand, the term CSC tend to be loosely used and include models as dissimilar as established cell lines grown as spheroids in 3D and sorted cells from human tumors expressing one or several surface markers. In fact, although resistance to anoikis and the ability to grow in anchorage-independent conditions are well-accepted characteristics of stem-like cells, the percentage of bona-fide CSCs within a spheroid may be as low as 1%. On the other hand, established cell lines are usually clonal and have been passaged in vitro for dozens or even hundreds of times: resemblance with the genetic and phenotypic heterogeneity found in tumors barely exists. Even when considering sorted cells from fresh tumors, we need to bear in mind that most surface markers are not completely reliable and may be lost or modified in sample preparation: in fact, trypsinization time may greatly affect expression of these markers. Most importantly, most in vitro studies are carried out in artificial metabolic conditions (e.g., high glucose and oxygen) lacking microenvironmental components of the CSC niche. In fact, tumor niche can support metabolic alterations in CSCs (Mateo et al., 2014; Ye et al., 2016) via signaling and metabolic crosstalk.
Interestingly, even with these limitations, different groups have found phenotypically diverse CSCs subpopulations coexisting in the same in vitro or in vivo conditions (Diehn et al., 2009; Sancho et al., 2015; Luo et al., 2018). For instance, although most pancreatic CSCs are dependent on OxPhos, a pre-existing subpopulation of CD133+ resistant to mitochondrial inhibition, due to their increased metabolic plasticity, was detected (Sancho et al., 2015). Importantly, differences in metabolism may be associated to functional diversity inside the CSCs population. Indeed, metabolic heterogeneity, mainly in terms of redox state, has been associated to differences in stemness, as well as chemo and radioresistance (Diehn et al., 2009; Wang et al., 2013; Sancho et al., 2015; Ye et al., 2016). Additionally, several reports link CSCs with enhanced metastatic abilities to specific metabolic traits. Indeed, reduced mitochondrial DNA and function contribute to the acquisition of a metastatic phenotype in spheroid-forming breast cancer cells (Guha et al., 2014). Moreover, alterations in redox balance leading to NRF2 activation mediate a phenotypic switch from glycolytic mesenchymal-like to OxPhos-dependent epithelial-like breast CSCs (Luo et al., 2018). In addition to breast cancer, CD44+ESAlow cells with increased metastatic potential (upon EMT), linked to low ROS levels, as compared to their non-EMT counterparts, have been described for oral and skin carcinomas, as well as prostate cancer (Gammon et al., 2013; Aguilar et al., 2016). On the contrary, metastasis-initiating cells in melanoma bear redox stress, as inferred from their elevated ROS and reduced glutathione content (Porporato et al., 2014; Piskounova et al., 2015).
Therapeutic Targeting of CSCs Metabolism
Considering the involvement of CSCs in chemoresistance, tumor relapse and metastasis, there is a pressing need in cancer therapy to find new strategies to eradicate this aggressive cell population. As summarized in the previous section, the distinct metabolic features of CSCs, compared to non-CSCs, constitute a significant opportunity to targeting specifically the CSCs component of tumors and eradicate the tumor bulk.
Mitochondrial Metabolism
As mentioned above, mitochondria play a key role for CSCs functionality regardless of their dominant metabolic phenotype, suggesting that targeting mitochondrial metabolism may be the most effective therapeutic strategy for their elimination. Noteworthy, highly glycolytic cells with mutations in the TCA cycle or ETC still require functional mitochondria for the generation of metabolites from glutamine via reductive carboxylation (Mullen et al., 2011). For those reasons, pharmacological approaches designed to target different aspects of mitochondrial function for cancer treatment are currently under intense investigation in preclinical and clinical studies (Figure 1).
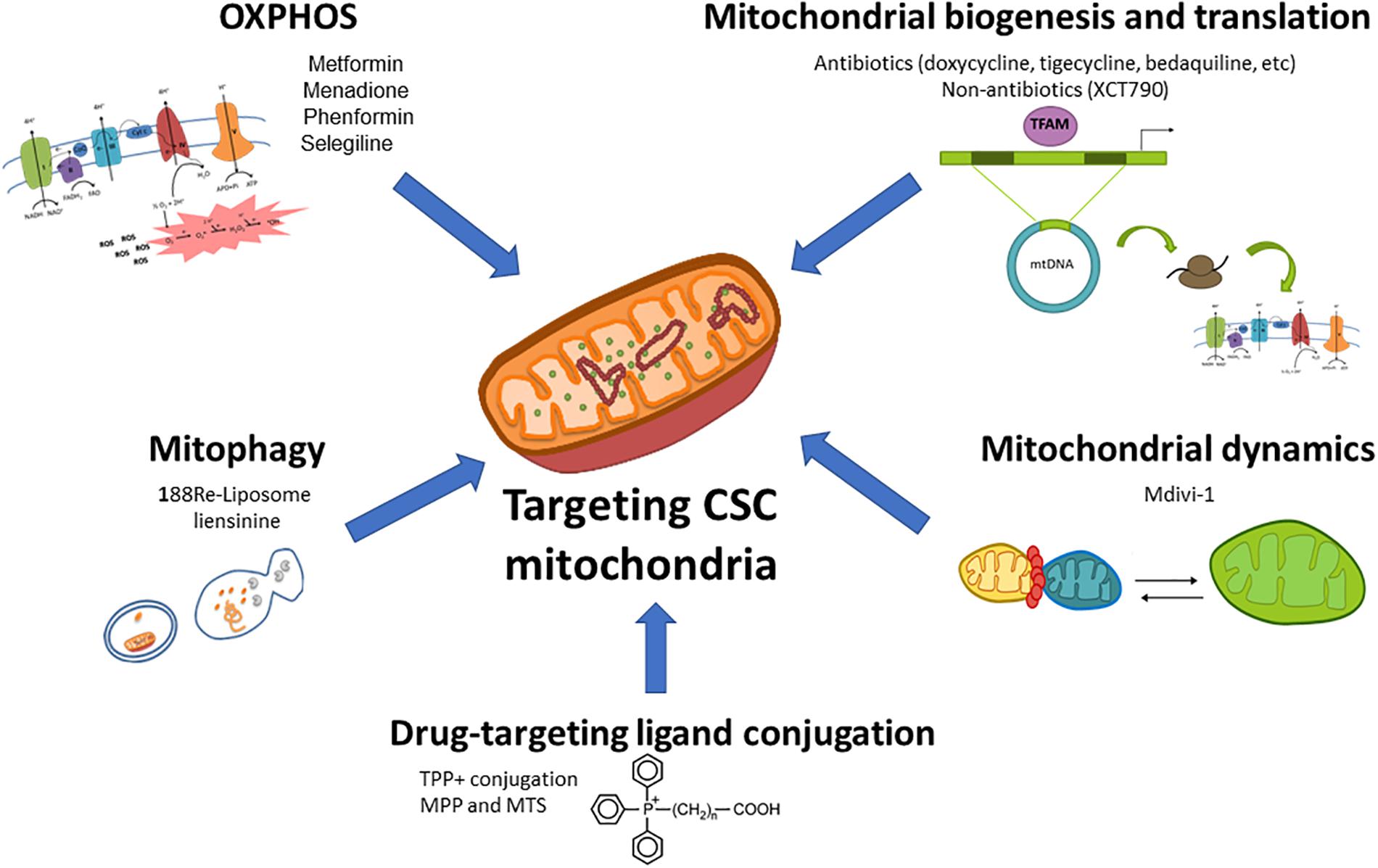
Figure 1. Therapeutic targeting of mitochondrial metabolism in CSCs. Different aspects of the mitochondrial metabolism can be approached to target CSCs: (1) oxidative phosphorylation (OxPhos) can be impaired by ETC inhibitors such as the antidiabetic drugs metformin or phenformin, the reactive oxygen species (ROS) inductor and complex I inhibitor menadione, or the anti-Parkinson compound selegiline; (2) Mitochondrial biogenesis and translation can be targeted by FDA-approved antibiotics such as doxycycline, tigecycline, bedaquiline among others, or non-antibiotic inhibitors; (3) Mitochondrial dynamics can be disrupted by the mitochondrial division inhibitor Mdivi-1; (4) The blockage of mitophagy, an essential mitochondrial quality control system, with nanomedicines such as 188Re-liposome or the inhibitor liensinine may affect CSCs functions; (5) The use of nanocarriers (lipophilic cations, peptides and nanoparticles) conjugated with chemotherapeutics and small drugs may be used for a selective delivery of drugs in mitochondria.
Targeting OxPhos
Inhibition of mitochondrial respiration by compounds blocking ETC complexes is one of the most studied metabolism-based strategies for cancer treatment. In fact, tumor cells in nutrient-deprived environments or displaying limited metabolic plasticity, as described for some gliomaspheres and PDAC CD133+ cells (Janiszewska et al., 2012; Sancho et al., 2015), have restricted ability to cope with decreased mitochondrial ATP production. ETC inhibitors also target glycolytic CSCs, such as CD44+CD24low cells in breast cancer or SP cells in nasopharyngeal carcinoma (Vazquez-Martin et al., 2010; Shen et al., 2013), highlighting the importance of ETC for coupled ATP production, avoiding electron loss in the form of ROS.
One of the most studied ETC inhibitors in the context of CSCs targeting is the antidiabetic drug metformin. Reported antitumoral effects of this drug relate to both systemic glucose decrease, and direct cancer cell targeting via ETC complex I inhibition (Wheaton et al., 2014). Although metformin shows cytostatic properties at low concentration, it induces apoptosis specifically in PDAC CD133+ cells and CD44high CD24low mammospheres (Iliopoulos et al., 2011; Sancho et al., 2015), which, at least for PDAC CD133+ cells, is attributable to their dependency on mitochondrial metabolism. Although metformin clinical testing for pancreatic cancer treatment showed no improvement in patient survival rate (Kordes et al., 2015; Reni et al., 2016), positive clinical data has been reported for breast, endometrial and prostate cancer. On the other hand, the development of resistance to metformin monotherapy in vivo suggests that the design of combinatory treatments (Sancho et al., 2015; Roh et al., 2017) or the use of stronger mitochondrial inhibitors may be needed. This could be the case of the ETC complex I inhibitor phenformin, which is more efficiently delivered to mitochondria (Petrachi et al., 2017) and when combined with the ALDH inhibitor gossypol, suppresses stemness, invasiveness and cell viability in glioblastoma (Park et al., 2018). Additionally, menadione, with dual mechanism of action combining complex I inhibition and ROS induction, prevents the development of resistance (Sancho et al., 2015).
Following metformin’s relative success, a great effort has been put on the drug repurposing for CSCs targeting in cancer treatment. In this sense, several known FDA-approved antibiotics target the ETC at different levels and have proven to selectively decrease CSC content. This is the case of antimycin A, a powerful complex III inhibitor that decreased lung spheroids (Yeh et al., 2013); the antituberculosis agent bedaquiline (complex V inhibitor) that targeted mammospheres (Fiorillo et al., 2016); oligomycin (another complex V inhibitor), which showed drastic synergistic effects suppressing cell growth and motility in glioblastoma cell lines when combined with 2-deoxy-D-glucose (2DG) (Kennedy et al., 2013); and niclosamide, an antihelmintic with ETC uncoupling properties, that inhibited TICs from ovarian and breast cancers (Yo et al., 2012; Wang et al., 2013). Similarly, numerous studies suggest the efficacy of the OxPhos inhibitor salinomycin for CSCs targeting in vitro and in vivo in diverse cancer types (Naujokat and Steinhart, 2012 and references therein). In this last case, however, the final antitumoral effect may be the result of a combination of factors, since salinomycin also interferes with ABC transporters or Wnt signaling (Naujokat and Steinhart, 2012). Besides antibiotics, the agent L-deprenyl (also known as Selegiline), a monoamine oxidase-B (MAO-B) inhibitor typically used for the treatment of Parkinson’s disease, was found to exert antimitochondrial activity and cause apoptotic cell death in AML CSCs through the reduction of ETC and glycolysis-related gene expression, independently of MAO-B inhibition (Ryu et al., 2018).
Considering the therapeutic potential of OxPhos inhibition, compound discovery is currently taking place in order to identify new selective molecules with adequate in vivo properties. As an example, the compound VLX600 showed cytotoxicity in colon cancer spheroid-derived cells both in vivo and in vitro, by directly inhibiting ETC complexes in metabolically compromised microenvironments (Zhang et al., 2014).
Targeting Mitochondrial Translation and Biogenesis
As commented above, several FDA-approved antibiotics can disrupt mitochondrial function. Apart from direct OxPhos inhibition, certain widely prescribed antibiotics target either mitochondrial translation or biogenesis as an “off-target” effect (Lamb et al., 2015c), inhibiting self-renewal ability of multiple tumor types (Lamb et al., 2015a,b). For instance, the use of a tetracycline such as doxycycline induced apoptosis in pancreatic cancer cell lines and human cervical carcinoma tumorspheres (Son et al., 2009; Yang et al., 2015), while azithromycin (erythromycin family) demonstrated to inhibit the self-renewal capacity of PDAC spheroids (Lamb et al., 2015c). On the other hand, the use of the antimicrobial tigecycline selectively killed leukemia CD34+CD38- cells without affecting normal hematopoietic cells through the inhibition of mitochondrial translation (Skrtic et al., 2011).
However, although this research line shows promising results, continuous treatment with antibiotics for cancer therapy may be ineffective (Esner et al., 2017). Indeed, long-term desensitization was reported for human metastatic breast cancer cells treated with different antibiotic classes including streptomycin, tetracycline, kanamycin, G418-geneticin (aminoglycoside), puromycine (aminonucleoside) and blasticidine (Esner et al., 2017). Nonetheless, the design of novel combinatory treatments or stronger derivatives could overcome this setback for future application in the clinical setting, taking advantage of the well-known safety profile of antibiotics.
On the other hand, non-antibiotic inhibitors of mitochondrial biogenesis are already available: XCT790, a specific inhibitor of the estrogen-related receptor alpha (ERRα)/PGC-1α, signaling pathway (responsible for mitochondrial biogenesis), inhibited breast CD44+/highCD24-/low TICs and mammosphere survival and propagation by reducing OxPhos. These effects were prevented or reversed by stimulating mitochondrial biogenesis with the mitochondrial fuel acetyl-L-carnitine (ALCAR9) (De Luca et al., 2015).
Targeting Mitochondrial Dynamics
Several types of cancer show downregulation of mitochondrial fusion proteins (Zhang et al., 2013; Chen and Chan, 2017) or upregulation of fission proteins (Rehman et al., 2012; Kashatus et al., 2015; Wieder et al., 2015; Zhang et al., 2017). In fact, increased mitochondrial fragmentation has also been involved in malignancy, promoting tumor migration and invasion in breast cancer (Zhao et al., 2013). On the other hand, mitochondrial dynamics seem to regulate proliferation and survival of CSCs, similar to what is known in embryonic stem cells (Chen and Chan, 2017). Indeed, dynamin-related protein 1 (DRP1)-dependent fission regulates mitochondrial distribution in asymmetrical division, ensuring maximal mitochondrial fitness in the daughter stem cell (Katajisto et al., 2015).
Currently, the only available pharmacological strategy to target mitochondrial dynamics is the DRP1 inhibitor mdivi-1. On the one hand, mdivi-1 or DRP-1 knockdown reduced proliferation and induced apoptosis in lung cancer cells, whose mitochondria were in a situation of constant fission in vitro and in vivo (Rehman et al., 2012). This inhibitor also attenuates lung cancer and mesothelioma proliferation when combined with the MET inhibitor MGCD516 (Wang J. et al., 2018). Importantly, mdivi-1 reduced tumorsphere-forming ability of breast, lung, and melanoma cancer cell lines (Peiris-Pagès et al., 2018) and reduced tumorigenicity of brain TICs in vitro and in vivo (Xie et al., 2015).
Targeting Mitophagy
Mitophagy is an essential quality control system to selectively remove damaged, non-functional or unnecessary mitochondria in cells. However, its involvement in cancer is controversial, since contradictory reports on the role of this process in tumorigenesis have been published (Chourasia et al., 2015a).
On the one hand, the mitophagy promoter Parkin is frequently deleted in many cancer types (Cesari et al., 2003). In addition, defective mitophagy caused by BNip3 loss/inhibition promote invasion and metastasis in breast, pancreatic or CRC (Okami et al., 2004; Chourasia et al., 2015b; Li et al., 2018). Moreover, the induction of mitophagy and mitoptosis by salinomycin treatment led to decreased mitochondrial mass and ATP depletion in prostate cancer and breast cancer CD44highCD24low cells triggering a cytotoxic effect specific to tumor cells without damaging normal fibroblasts (Jangamreddy et al., 2013).
On the other hand, mitophagy can be triggered as a stress response against nutrient deprivation or hypoxia, promoting cell survival and tumorigenesis in hostile environments and contributing to drug resistance in human CRC CD133+CD44+ cells (Jangamreddy et al., 2013; Yan et al., 2017). In agreement with this notion, mitophagy is upregulated in esophageal squamous cell carcinoma undergoing EMT (Whelan et al., 2017). Accordingly, the use of mitophagy blockers, such as the nanomedicine 188Re-Liposome or the inhibitor liensinine, reversed drug resistance in ovarian cancer cells in vitro (Chang et al., 2017) and in breast cancer xenografts in vivo (Zhou et al., 2015), respectively. Additionally, the alkaloid matrine induced mitochondrial dysfunction and apoptosis by inhibiting mitophagy in HepG2 hepatoblastoma cells (Wei et al., 2018). Interestingly, a link between autophagy and mitochondrial respiration has recently been reported: the novel autophagy inhibitor aumitin blocks complex I activity (Robke et al., 2018), while pharmacologic or genetic inhibition of complex I inhibitor impairs autophagy (Thomas et al., 2018).
Mitochondrial Drug Delivery
In order to ensure an efficient and selective delivery in mitochondria, different small compounds or chemotherapeutic drugs can be conjugated with nanocarriers, including lipophilic cations, peptides and nanoparticles, with preferential accumulation in mitochondria.
One of the most studied strategies involves the conjugation of small compounds with delocalized lipophilic cations, such as triphenylphosphonium (TPP), dequalinium or rhodamine 123, that possess both lipophilicity and a positive charge, and accumulate in the mitochondrial matrix (Murphy, 2008). Importantly, as OxPhos-dependent CSCs show an elevated mitochondrial membrane potential (ΔΨm), indicative of increased activity (Sancho et al., 2015), conjugated compounds will be delivered primarily to these cells. For example, MitoChromanol (vitamin E analog) or Gamitrinib (chaperone inhibitor) combined with TPP inhibit OxPhos and ATP production selectively in cancer cells (Chae et al., 2012; Cheng et al., 2013).
Commonly used chemotherapeutic agents can also be joined with lipophilic cations to exert their therapeutic action in the mitochondria and improve their effect. For example, doxorubicin (DOX) combined with TPP showed enhanced toxicity against DOX-resistant MDA-MB-453 breast cancer cells, even though TPP-DOX was as toxic as free DOX in wild type cells (Han et al., 2014). Interestingly, DOX fused with TPP-conjugated chitosan nanoparticles exhibited higher cytotoxicity than free doxorubicin in A549 and Hela cells (Hou et al., 2017). Additionally, the development of a cisplatin prodrug combined with TPP showed promising results treating cisplatin-resistant, aggressive cancers, such as neuroblastoma, since its delivery into the mitochondrial matrix circumvents the nucleotide excision repair pathway present in the nucleus (Marrache et al., 2014). Moreover, the union of paclitaxel with TPP also resulted in enhanced antitumor effects in Hela and in mouse mammary carcinoma cells (4T1) in vitro and in vivo (Biswas et al., 2012).
An alternative delivery strategy tested for chemotherapeutic agents involved their conjugation with mitochondria-penetrating peptide (MPP) or mitochondria-targeting sequences (MTS), which act independently of mitochondrial potential. This approach directs their activity toward mtDNA, thus promoting drug selectivity for cancer cells with reduced mtDNA integrity, while their stable mitochondrial location prevents the acquisition of resistance due to drug efflux (Chamberlain et al., 2013). As an example, cisplatin linked to MMP overcomes tolerance in cisplatin-resistant ovarian cancer 2780/CP70 cells (Marrache et al., 2014).
Finally, the combination of the antibiotic salinomycin with reduced graphene oxide-silver nanocomposites synergistically enhanced the activity of either compound alone, leading to mitochondrial dysfunction and selectively killing human ovarian CD133+ cells (Choi et al., 2018).
Targeting Redox Homeostasis
It is well established that intracellular ROS accumulation induces cancer cell death, a strategy widely used in the clinics associated to classical chemo and radiotherapy. However, recent evidence suggests that this approach may not be effective against CSCs, due to their increased antioxidant potential (Diehn et al., 2009; Ishimoto et al., 2011; Yuan et al., 2015). Moreover, ROS can be a double-edged sword, since they may promote CSCs survival and invasive abilities acting as signaling molecules (Luo et al., 2018).
As previously mentioned, CSCs are characterized by a finely regulated redox metabolism (Le Belle et al., 2011; Paul et al., 2014; Chang et al., 2018), where glutathione plays an essential role to maintain stemness characteristics (Diehn et al., 2009; Ishimoto et al., 2011). For that reason, increasing oxidative stress by blocking glutathione synthesis could represent a novel therapeutic strategy for eliminating CSCs population and diminishing tumor growth (Diehn et al., 2009; Rodman et al., 2016). Thus far, buthionine sulfoximine (BSO), an inhibitor of glutathione biosynthesis, has proven (Marengo et al., 2008) to be very effective in decreasing clonogenicity and enhancing response of CSCs to radiotherapy in vitro and in vivo (Diehn et al., 2009; Boivin et al., 2011; Rodman et al., 2016). Due to its importance for glutathione biosynthesis, especially in glutamine-addicted cancer cells, deprivation of glutamine increased oxidative stress and reduced SP cells in non-small lung and pancreatic cancer cell lines (Liao et al., 2017). Glutamine deprivation also inhibited metastatic potential of cancer cells, one of the main characteristics of CSCs (Wang et al., 2010).
Besides glutathione, strategies aimed at inhibiting cellular antioxidants are currently applied with relative success, mostly improving response to conventional therapies (Figure 2). For example, treatment with auranofin, a thioredoxin reductase inhibitor, increased the sensitivity of human breast CSCs to radiotherapy (Rodman et al., 2016), while a synergistic reduction of the CD44v9+ cells content was achieved by inhibiting glutathione-S-transferase and thioredoxin reductase in patient-derived xenograft (PDX) models of CRC (Tanaka et al., 2016). Arsenic trioxide (ATO), an FDA-approved drug for acute promyelocytic leukemia that increases ROS content and depletes superoxide dismutase (SOD) and glutathione peroxidase (GPX) (Li et al., 2006), proved to reduce CSCs content in different cancer types (Ding et al., 2014; Li et al., 2015a; Chang et al., 2016; Bell et al., 2018). Moreover, the synergistic effect of ATO with glutathione depletion could present a novel treatment for cancers unresponsive to ATO treatment alone (Miller, 2002; Davison et al., 2003; Bhalla et al., 2009; Matulis et al., 2012). The anti-alcohol addiction drug disulfiram has been widely used as anticancer agent since it can increase oxidative stress by blocking SOD activation (Calderon-Aparicio et al., 2015) and inhibiting NRF2 (Xu et al., 2017). In studies with breast cancer cell lines in vitro and in vivo, disulfiram not only diminished mammosphere formation (Yip et al., 2011; Kim et al., 2017) and reduced CD44+CD24- and CD49f+CD24+ subpopulations, but also managed to reverse paclitaxel and cisplatin resistance of triple-negative breast cancer (TNBC) (Liu et al., 2013). Moreover, disulfiram proved to diminish the ALDH1+ population from non-small cell lung cancer cell lines (Liu et al., 2016) and CD34+CD38+ cells in AML cell lines and primary samples in vitro and in vivo (Xu et al., 2017). Now entering phase III in clinical trials, disulfiram may present a potential adjuvant therapy for cancer treatment, although it is highly unstable in blood. For that reason, disulfiram-containing nanoparticles have also been developed. Even though these nanoparticles proved to increase disulfiram blood levels (Song et al., 2016), further studies are needed to establish its full in vivo antioxidant and biological properties.
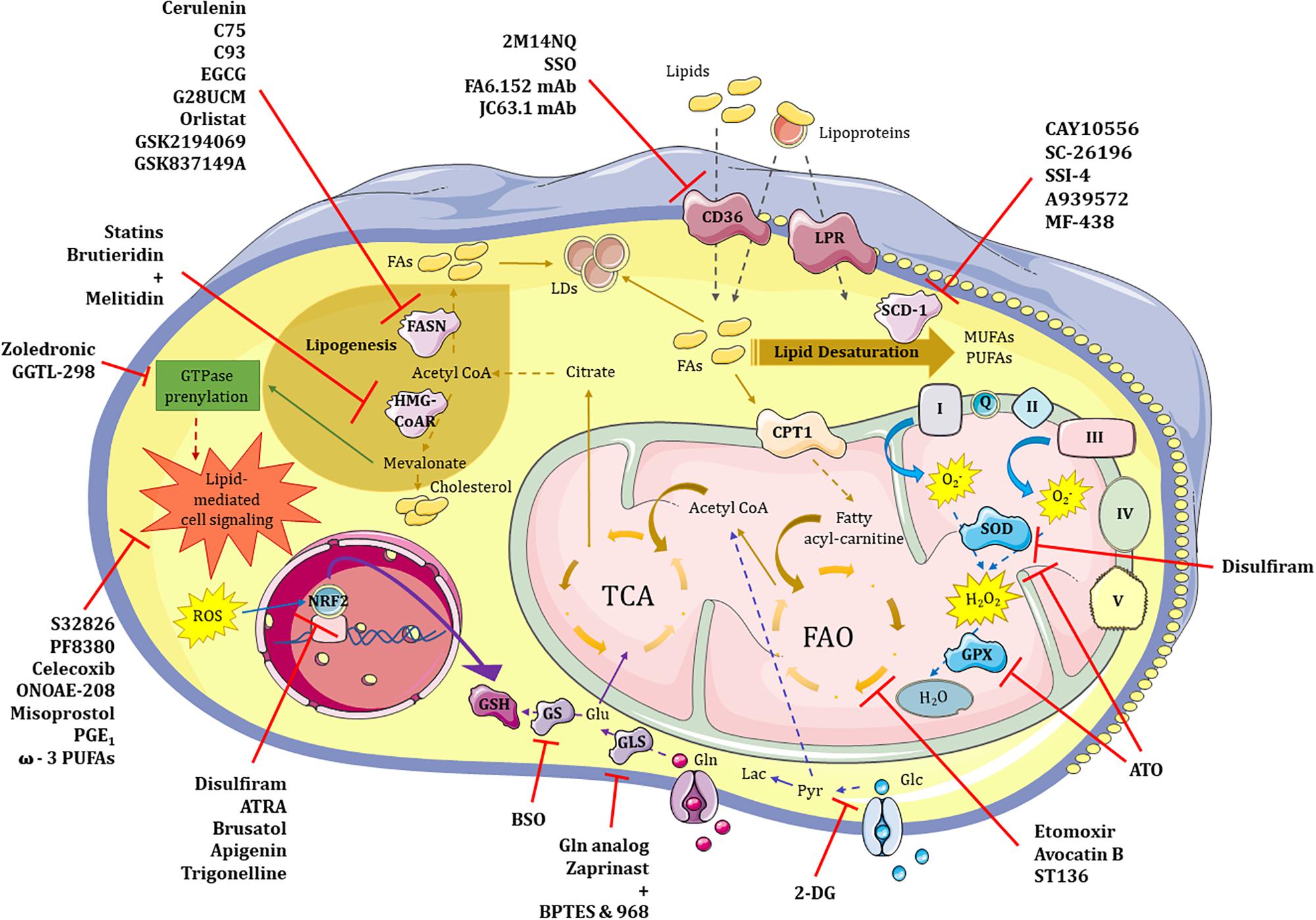
Figure 2. Therapeutic targeting of glycolysis, lipid and redox metabolism in CSCs. Metabolic pathways such those involving glucose, lipids and redox balance are potentially targetable in CSCs. (1) Glycolysis. 2-DG represent the most promising therapeutic approach to neutralize highly glycolytic CSCs in combination treatments. (2) Lipid metabolism. 2M14NQ, SSO and the monoclonal antibodies FA6.152 and JC63.1 can block CD36 activity; substances like Etomoxir, Avocatin B or ST136 block fatty acid oxidation (FAO) in the mitochondria; FASN can be inhibited by drugs such as Cerulenin, C75, C93, EGCG, G28UCM, Orlistat, GSK2194069 or GSK837149A; while HMG-CoAR enzyme may be inhibited by either Statins or the combination of Brutieridin plus Melitidin; GTPase prenylation pathway in which mevalonate is involved can be targeted by both Zoledronic acid and GGTL-298; and different steps of the lipid-mediated cell signaling may be blocked with molecules such as S32826, PF8380, Celecoxib, ONOAE-208, Misoprostol, PGE1 and ω-3 PUFAs; finally, targeting of the main enzyme of lipid desaturation route, SCD-1, can be achieved by CAY10556, SC-26196, SSI-4, A939572 or MF-438. (3) Redox metabolism. Antioxidant features of CSCs may be inhibited at different levels including SOD and GPX proteins with Disulfiram and/or ATO, respectively; ROS-induced NRF2 activity can be neutralized by Disulfiram, ATRA, Brusatol, Apigenin and Trigonelline; finally, glutathione synthesis may be inhibited either directly or indirectly by blocking GS or GLS enzymes with BSO or a glutamine analog, and a mixture of Zaprinast with BPTES or 968 compounds, respectively. 2-DG – 2-deoxy-D-glucose, Pyr – pyruvate, LDs – lipid droplets, LPR – lipoprotein receptor, FAs – fatty acids, FASN – fatty acid synthetase, HMG-CoAR – 3-hydroxy-3-methyl-glutaryl-coenzyme A reductase, SCD-1 – stearoyl-CoA desaturase, MUFAs – monounsaturated fatty acids, PUFAs – polyunsaturated fatty acids, FAO – fatty acid oxidation, TCA – tricarbolxylic acid cycle, CPT1 – carnitine palmitoyltransferase I, GTPase – guanosin triphosphatase, I/Q/II/III/IV/V – complexes of the electron transport chain, O2- – superoxide anion, H2O2 – oxygen peroxide, SOD – superoxide dismutase, GPX – glutathione peroxidase, ROS – reactive oxygen species, NRF2 – nuclear factor erythroid 2–related factor 2, GSH – glutathione, Glu – glutamate, Gln – glutamine, GS – glutathione synthase, GLS – glutaminase, 2M14NQ – 2-methylthio-1,4-naphtoquinone, SSO – sulfosuccinimidyl oleate, mAb – monoclonal antibody, EGCG – epigallocatechin gallate, ATRA – all-trans retinoic acid, BSO – L-buthionine-S,R-sulfoximine, ATO – arsenic trioxide.
Increased NRF2 levels turned out to play a role in CSCs survival and chemoresistance (Kwak et al., 2001; Zhu et al., 2013; Ryoo et al., 2016), representing another potential target for eradicating CSCs. All-trans retinoic acid (ATRA) blocked NRF2 activation, diminishing self-renewal and tumorigenic capacity of ALDH1+ lung cancer cells (Moreb et al., 2004) and ovarian cancer cell lines (Kim D. et al., 2018). Moreover, brusatol, which decreases NRF2 protein levels, was demonstrated to inhibit mammospheres formation and increase the sensitivity of human breast CSCs to Taxol (Wu T. et al., 2015). Similarly, the natural flavonoid apigenin (Kim et al., 2016; Erdogan et al., 2017) or the alkaloid trigonelline (Arlt et al., 2013; Roh et al., 2017), which inhibits NRF2 at transcriptional and translational level, can sensitize CSCs toward chemotherapeutic drugs. On the other hand, the combination of ROS inducers and NRF2 (or downstream targets) inhibition could represent a potential strategy for CSCs elimination (Diehn et al., 2009; Ishimoto et al., 2011; Kwak et al., 2016; Liu et al., 2016; Kim et al., 2017; Kim D. et al., 2018; Luo et al., 2018).
Paradoxically, some natural antioxidants that can increase NRF2 expression levels have also shown therapeutic potential. The NRF2-inducer sulforaphane, a dietary component from broccoli, inhibited self-renewal capacity of CD44+ LDH1+ pancreatic (Rausch et al., 2010) and ALDH1+ breast cancer cells in vivo and in vitro (Burnett et al., 2017). Curcumin, an active ingredient of turmeric, diminished self-renewal capacity of CD44+ EpCAM+ pancreatic cancer cells in vitro and in vivo (Bao et al., 2012) and reduced proliferation and mammosphere formation of ALDH1+ breast cancer cells (Kakarala et al., 2010). Additionally, resveratrol, oleanane triterpenoid or carnosol also proved to active and increase NRF2 expression which could have a positive effect on diminishing the CSCs population (Probst et al., 2015a,b; Sancho et al., 2015; Giacomelli et al., 2017). Treatment with naturally occurring antioxidants, such as vitamin C or phenethyl isothiocyanates (PEITCs), found in broccoli or Brussel sprouts, also diminished self-renewal capacity and clonogenicity of NCCIT human embryonic carcinoma and human colon cancer cell lines, and reduced CD133+, EpCAM+ and OV6+ cells while inhibiting tumorspheres formation and growth of hepatocellular carcinoma cell lines and PDX models in vivo (Yun et al., 2017; Lv et al., 2018). However, even though natural antioxidants could represent an exciting strategy in anticancer therapy, clinical trials thus far showed no positive effect on patient survival. Indeed, published data highlighted the lack of specificity of antioxidant treatments. This fact, together with the possible contribution of antioxidants to stemness maintenance and cancer development, weakens the translation potential of this approach.
Targeting Lipid Metabolism
Lipid metabolism has become an interesting target in order to design new anti-CSC strategies, and a number of compounds have been tested during the last years (Figure 2).
Lipid Desaturation
Over the last few years, several SCD-1 inhibitors have demonstrated their effectiveness in different preclinical in vitro and in vivo models of cancer, by specifically targeting stemness-related properties. Indeed, the inhibitors CAY10556 and SC-26196 reduced stem cell-related markers and signaling pathways by downregulating Hedgehog and Notch expression in ovarian ALDH+CD133+ cells (Li J. et al., 2017). Interestingly, this led to the inhibition of sphere formation in vitro and tumorigenicity in vivo, with no effect on differentiated cells, suggesting the selectivity of this approach. In the same line of evidence, inhibition of SCD-1 with the compounds SSI-4 or A939572 modulates endoplasmic reticulum-stress-mediated differentiation in liver chemoresistant hepatospheres, sensitizing resistant PDXs to sorafenib treatment with low side toxicity in vivo (Ma et al., 2017). In parallel, effects of the inhibitor A939572 in CD133+CD49f+ liver CSCs have also been linked to Wnt-mediated self-renewal and in vivo tumorigenicity (Lai et al., 2017). Finally, MF-438 treatment induced anoikis in lung ALDH1+ cells, decreasing self-renewal and pluripotency markers expression (Pisanu et al., 2017). Interestingly, these in vitro effects translated into reduced tumorigenic potential and reversion of chemoresistance in vivo.
Lipogenesis
Given the important involvement of the enzyme FASN in numerous tumor types, a number of inhibitors have been designed and/or tested in diverse cancer models: cerulenin, C75, C93, epigallocatechin gallate (EGCG), G28UCM, orlistat, GSK2194069 and GSK837149A. In fact, cerulenin treatment prevents proliferation in vitro of pancreatic spheres (Brandi et al., 2017) and neurospheres established from glioma patients (Yasumoto et al., 2016) CSCs. On the other hand, C75 at non-cytotoxic concentrations significantly reduced self-renewal in HER2+ breast cancer cells (Corominas-Faja et al., 2017). However, it is important to highlight the critical selectivity and toxicity issues found for FASN inhibitors in vivo, which have compromised their translation to clinical trials. Only the inhibitor TVB-2640 is being currently tested in clinical trials for HER2+ advanced breast cancer, high grade astrocytoma and colon cancer (NCT03179904, NCT03032484, NCT02980029, respectively).
Cholesterol Synthesis
Cholesterol synthesis through the mevalonate pathway can be inhibited by statins, for which the molecular target is the enzyme 3-hydroxy-3-methylglutharyl-coenzyme A reductase (HMG-COAR). In fact, treatment with different statins decreased self-renewal and CSCs content in breast (Ginestier et al., 2012) and nasopharyngeal (Peng et al., 2017) carcinomas. Interestingly, similar effects were detected in CD133+ brain TICs (Wang et al., 2017a) where overexpression of mevalonate pathway genes was controlled by MYC, highlighting the variety of metabolic pathways controlled by the oncogene. However, anti-CSCs effects of statins could also be related to inhibition of cellular signaling via small GTPases (e.g., Rho and Rac), since they require prenylation using mevalonate pathway intermediates. In fact, impaired self-renewal ability achieved with simvastatin treatment in breast tumorspheres was recapitulated by zoledronic acid and GGTI-298, inhibitors of the prenylation pathway (Ginestier et al., 2012). Moreover, a mixture of brutieridin and melitidin (natural products derived from bergamot with statin-like properties) impaired breast ALDH1+ CSCs proliferation inhibiting both FAO and Rho-related signaling pathways (Fiorillo et al., 2018).
Lipid Uptake
Strategies targeting lipid uptake are mainly designed to inhibit the transporter CD36, by either pharmacological inhibition or blocking antibodies. CD36 blockade with 2-methylthio-1,4-naphtoquinone decreases self-renewal ability and induces apoptosis in glioblastoma CD133+ (Hale et al., 2014). Another CD36 inhibitory compound, sulfosuccinimidyl oleate, decreases chemoresistant leukemic stem cells (Ye et al., 2016). Interestingly, CD36-neutralizing antibodies against either all known functions of CD36 (FA6.152) or the ones reported to block active FA and lipoprotein uptake (JC63.1) induced lipotoxicity in label-retaining/CD44+ metastasis-initiating cells, thus, inhibiting metastasis initiation and progression in oral squamous cell carcinoma, with no reported toxicity in vivo (Pascual et al., 2016).
FAO
Fatty acid oxidation inhibition with etomoxir has been studied in preclinical in vitro and in vivo cancer models. Indeed, etomoxir treatment inhibits mammosphere formation and tumor growth in vivo in TNBC tumors bearing high MYC expression (Camarda et al., 2016). In addition, etomoxir treatment sensitizes hepatocarcinoma CD133+CD49f+ CSCs to standard chemotherapy with sorafenib (Chen C.L. et al., 2016). Moreover, etomoxir decreases the number of quiescent leukemia CSCs in AML patients and, combined with the BCl-2 inhibitor ABT-737, substantially decreases tumor burden (Samudio et al., 2010). However, etomoxir treatment induces normal hematopoietic stem cell exhaustion invalidating this compound for further clinical studies (Ito et al., 2012). Interestingly, alternative FAO inhibitors with higher selectivity for malignant cells are under investigation currently. For example, avocatin B is a lipid that accumulates in mitochondria inhibiting FAO and targets AML cells and leukemia CD34+ CSCs with no effect on hematopoietic stem cells (Lee et al., 2014, 2015). Additionally, the compound ST136 showed antileukemic activity with no effect on normal CD34+ stem cells (Ricciardi et al., 2015).
Lipid-Mediated Signaling
As stated in the previous section, lipid-mediated signaling plays an important role in cancer and, specifically, in CSCs functions. For that reason, several therapeutic approaches, including inhibitors and indirect modulation via dietary supplements, have been studied over the last few years. For example, several stemness-related functions of ovarian spheroid-derived cells from cell lines and primary cells from patients were dependent on LPA synthesis. Thus, inhibition of the LPA-producing enzyme autotaxin with the small molecules S32826 or PF8380 decreased tumorigenicity and chemoresistance in vivo (Seo et al., 2016). Interestingly, inhibition of LPA production not only affects cancer cells, but could also play an important role modulating the immune system and supporting tumor progression. Indeed, LPA induces the differentiation of monocytes into macrophages and favors the activation of CAFs phenotype (Ray and Rai, 2017; Radhakrishnan et al., 2019).
The most studied lipid mediator class in relation to CSCs is prostaglandins. Indeed, treatment of ApcMin/þ mice with celecoxib, the prostaglandin-endoperoxide synthase 2 selective inhibitor, or the EP4 receptor (prostaglandin receptor) antagonist ONOAE-208 resulted in a reduction of tumor CD133+CD44+ cells and tumor burden (Wang et al., 2015). Importantly, celecoxib inhibited CSCs content and the number of liver metastatic tumors upon orthotopic injection of patient-derived CRC into NSG mice. Additionally, celecoxib impaired chemoresistance in bladder carcinomas, suggesting its utility as adjuvant therapy (Kurtova et al., 2015). On the contrary, activation of EP4 with the FDA-approved agonist misoprostol or PGE1 reduced CD34+ cells in a xenograft model of chronic myelogenous leukemia (CML) (Li F. et al., 2017), suggesting a context-dependent effect of prostaglandins in stemness.
On the other hand, preclinical and human observational studies suggest that dietary omega-3 polyunsaturated fatty acids (ω-3 PUFA), including eicosapentaenoic acid (EPA) and docosahexaenoic acid (DHA), decrease CRC risk and may be effective as adjuvant treatment of advanced CRC. Indeed, EPA and DHA reduced the CD133+ content or stem properties in two different in vitro studies using CRC cell lines (De Carlo et al., 2013; Yang et al., 2013). Interestingly, EPA alone or in combination with chemotherapy decreased sphere-forming ability and suppressed tumor growth, likely through inhibition of proinflammatory metabolites in mice (Vasudevan et al., 2014). Importantly, studies in other tumor entities also suggest an anti-CSCs effect of ω-3 PUFAs besides CRC. Indeed, EPA and DHA supplementation also reduced proliferation and induced toxicity in breast tumorspheres, likely through alteration of the prostaglandin profile (Erickson and Hubbard, 2010). In addition, a metabolite derived from EPA eradicated leukemia M34+Kit+Sca1+ CSCs in PDXs of CML (Hegde et al., 2011).
Targeting Metabolism of Alternative Fuels
As mentioned in the previous section, CSCs may utilize a number of different substrates, such as amino acids and ketone bodies, in order to support self-renewal and tumorigenicity. For that reason, diverse compounds which target the metabolism of these alternative fuels are currently under investigation.
On the one hand, the use of a glutamine analog reduced 20 times tumor growth and inhibited metastasis in the VM-M3 murine tumor model of systemic metastasis, when compared with non-treated mice (Shelton et al., 2010). Interestingly, the anti-asthma compound Zaprinast was identified as a novel glutaminase inhibitor that, together with BPTES and 968, inhibited clonogenicity of pancreatic cancer cells in response to radiation (Elhammali et al., 2014).
Interestingly, Ozsvari et al. (2017) unveiled the potential anti-CSCs activity of a novel class of compounds denominated “mitoketoscins.” These compounds block the active site of the enzymes involved in the recycling of ketone bodies into acetyl-CoA (OXCT1 and ACAT1), leading to inhibition of the CSCs activity and propagation in breast cancer spheroids. However, considering the sometimes contradictory results of diverse studies on the antitumor effects of ketogenic diet (high-fat/low-carbohydrate intake) (Vidali et al., 2015; Weber et al., 2018), the anti-tumor effect of these inhibitors would need to be carefully tested as dependency on ketone bodies strongly varies across tumor entities and specific genotypes.
Combination Treatments: Targeting Glycolysis
Considering the great intratumoral metabolic heterogeneity and plasticity found in tumors, mitochondrial inhibitors as single agents will unlikely become an effective therapy for cancer treatment. In fact, combination treatments, where two or more metabolic pathways are inhibited simultaneously, would block relapse and development of resistances. For instance, a dual inhibition of the main metabolic pathway together with its main escape mechanism will completely erase CSCs within the tumor. This has been reported for the combinations of metformin with either the bromodomain and extraterminal motif (BET) inhibitor JQ-1 in pancreatic cancer (Sancho et al., 2015) or PI3K inhibition for ovarian cancer (Li et al., 2012), which blocks OxPhos and indirectly inhibits glycolysis simultaneously.
In fact, direct glycolysis inhibition for cancer treatment has been studied intensively in preclinical and clinical settings over the last few years, although with low success rates. On the one hand, glucose transport inhibitors, such as silybin/silibinin [tested in a phase I/II clinical trials for prostate cancer and advanced hepatocellular carcinoma (Flaig et al., 2006)], phloretin, WZB117 and fasentin caused important side effects, since GLUT transporters are present in all the cells of the organism. Similarly, inhibition of glycolytic enzymes, such as hexokinase II with lonidamine, has been tested in several types of cancers, including breast, lung and ovarian cancer (Gadducci et al., 1994; De Lena et al., 1997; De Marinis et al., 1999; Berruti et al., 2002). However, there was no significant improvement in overall survival and many cases presented with elevated toxicity. Additionally, the glucose analog 2-DG was shown to be a promising agent in preclinical studies (Maschek et al., 2004; Coleman et al., 2008). In fact, it has been recently tested in phase II/III of a clinical trial for prostate cancer (NCT00633087), although no results are available, since the trial was terminated due to the slow accrual.
Apart from direct inhibition, targeting tumor drivers affecting cellular metabolism might hinder glycolysis. For example, KRAS mutation is present in more than 90% of pancreatic cancer cases (Bailey et al., 2016) and controls both tumorigenesis and metabolic reprogramming (Ying et al., 2012; Son et al., 2013; Liou et al., 2016). In fact, KRAS drives glycolysis and the diversion of glycolysis intermediates into the non-oxidative branch of PPP, essential for the synthesis of nucleic acids (Yun et al., 2009; Ying et al., 2012; Blum and Kloog, 2014). However, even though small molecule inhibitors of KRAS proved to be promising in preclinical studies (Xie et al., 2017; Zeng et al., 2017), targeting KRAS or its downstream pathways showed no effect in overall survival and overall response rate in pancreatic cancer patients (Kindler et al., 2012; Infante et al., 2014; Chung et al., 2017).
Alternatively, c-MYC is another essential driver of tumorigenesis and glycolysis in cancer (Miller et al., 2012; Lin et al., 2013; He et al., 2015). For that reason, different compounds targeting MYC are currently undergoing clinical trials. Noteworthy, inhibitors of BET proteins directly downregulate MYC expression and suppress tumor growth in vivo (Delmore et al., 2011; Mazur et al., 2015; Garcia et al., 2016). Importantly, since MYC suppression blocks development of resistance to mitochondrial inhibitors (Sancho et al., 2015; Kim J.H. et al., 2018), combinatory approaches using this strategy can represent a promising anticancer therapy.
Concluding Remarks
Over the last few years, a huge collective effort to decipher metabolic reprogramming occurring in cancer has taken place. Technical advances have allowed the determination of the great metabolic heterogeneity, not only among individuals suffering from one type of cancer, but within a single tumor. Collectively, present literature indicates that both metabolic and redox state diversity define CSCs phenotype and fate, determining response to therapy. Importantly, most of the metabolic dependencies described for CSCs in diverse tumor entities have the tight control of redox state as a common factor (Figure 3), unveiling an important vulnerability that could provide new therapeutic opportunities. Other factors, such as tumor metabolic heterogeneity, microenvironmental cues or a cross-talk through metabolic and redox signaling between CSCs and cancer cells or stromal components (Riemann et al., 2011; Chen X. et al., 2016; Chang et al., 2018; Luo et al., 2018) can play an additional role in cancer progression and chemoresistance. Therefore, current knowledge suggests that carefully designed therapies, which target metabolically diverse populations and consider the tumor microenvironment may be crucial in order to develop more effective metabolism-focused treatment strategies.
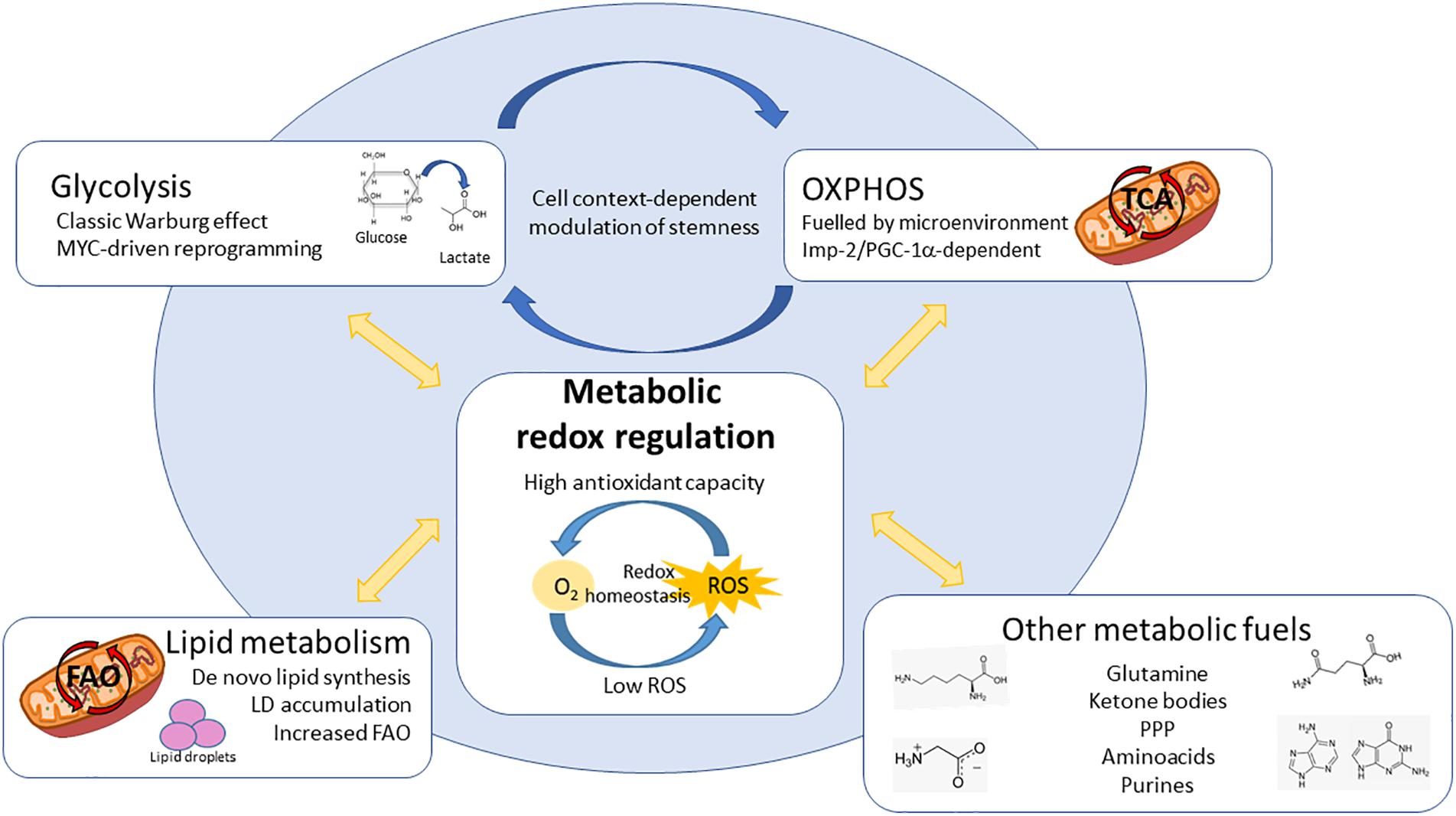
Figure 3. Redox involvement in the different metabolic dependencies described for CSCs. CSCs bear diverse metabolic dependencies in a tumor and context-dependent manner: (1) Aerobic glycolysis, controlled by MYC; (2) OxPhos, fuelled by different microenvironmental substrates and controlled by Imp2 or PGC-1α; (3) Lipid metabolism, increasing either fatty acid synthesis and storage in lipid droplets (LDs) or utilization via mitochondrial FAO; (4) CSCs can be dependent on alternative substrates and pathways such as aminoacids, ketone bodies, PPP or purines. Interestingly, the metabolic phenotypes described for CSCs ensure the maintenance of cellular redox state. Keeping redox balance is crucial for CSCs in order to maintain their stemness characteristics, differentiation ability and resistance to chemo and radiotherapy, constituting one of the most important vulnerabilities independently of their origin or cellular context. ROS – reactive oxygen species, OxPhos – oxidative phosphorylation, LDs – lipid droplets, PPP – pentose phosphate pathway, FAO – fatty acid oxidation, TCA – tricarboxylic acid cycle.
Author Contributions
PJ, BdL-D, and BP-A designed the figures. PS developed the study concept, obtained funding and wrote the final version of the manuscript. All authors contributed to conception and design of the manuscript, wrote sections, revised, read and approved the submitted version.
Funding
This work was supported by the Pancreatic Cancer Research Fund (2015 Award Round), the Miguel Servet Program (CP16/00121) and a FIS (Fondo Investigaciones Sanitarias) grant (PI17/00082), both from the Instituto de Salud Carlos III and cofinanced by European funds (FSE: “el FSE invierte en tu futuro” and FEDER: “una manera de hacer Europa,” respectively).
Conflict of Interest Statement
The authors declare that the research was conducted in the absence of any commercial or financial relationships that could be construed as a potential conflict of interest.
Acknowledgments
We would like to thank Laura Sancho Andrés for language and manuscript editing.
Abbreviations
α-ADD, alpha-aminoadipate; 2DG, 2-deoxy-D-glucose; AML, acute myeloid leukemia; ALDH1, aldehyde dehydrogenase 1; ATO, arsenic trioxide; BET, bromodomain and extra-terminal motif; BSO, buthionine sulfoximide; CAFs, cancer associated fibroblast; CML, chronic myeloid leukemia; CSCs, cancer stem cells; DHA, docosahexaenoic acid; DOX, doxorubicin; DQA, dequalinium; DRP-1, dynamin-related protein 1; EGCG, epigallocatechin gallate; EPA, eicosapentaenoic acid; ERRα, estrogen-related receptor alpha; EMT, epithelial-mesenchymal transition; ETC, electron transport chain; FAs, fatty acids; FAO, fatty acid oxidation; FASN, fatty synthase; FOXO, forkhead box; G6P, glucose-6-phosphate; GPx, glutathione peroxidase; HBP, hexosamine biosynthetic pathway; LDs, lipid droplets; LPA, lysophosphatidic acid; MAO-B, monoamine oxidase-B inhibitor; MCT 1/2, monocarboxylate transporter 1 and 2; Mdivi-1, mitochondrial division inhibitor; MPP, mitochondria penetrating peptide; mtDNA, mitochondrial DNA; mTOR, mammalian target of rapamycin; MTS, mitochondria targeting sequences; MUFAs, monounsaturated fatty acids; NER, nucleotide excision repair; NRF2, nuclear factor erythroid 2–related factor 2; OxPhos, oxidative phosphorylation; PDAC, pancreatic ductal adenocarcinoma; PDX, patient-derived xenograft; PEITCs, phenethyl isothiocyanates; PGC-1α, peroxisome proliferator-activated receptor gamma coactivator 1-alpha; PHGDH, phosphoglycerate dehydrogenase; PI3K, phosphoinositide 3-kinase; PPP, pentose phosphate pathway; ROS, reactive oxygen species; S1P, sphingosine-1-P; SOD, superoxide dismutase; SREBP1, sterol regulatory element-binding protein 1; SCD-1, stearoyl-CoA desaturase; TCA, tricarboxylic acid; TICs, tumor initiating cells; TNBC, triple negative breast cancer; TPP, triphenylphosphonium.
References
Abdul-Aziz, A., Macewan, D. J., Bowles, K. M., and Rushworth, S. A. (2015). Oxidative stress responses and NRF2 in human leukaemia. Oxid. Med. Cell. Longev. 2015:454659. doi: 10.1155/2015/454659
Aguilar, E., de Mas, I. G., Zodda, E., Marin, S., Morrish, F., Selivanov, V., et al. (2016). Metabolic reprogramming and dependencies associated with epithelial cancer stem cells independent of the epithelial-mesenchymal transition program. Stem Cells 34, 1163–1176. doi: 10.1002/stem.2286
Alptekin, A., Ye, B., and Ding, H. F. (2017). Transcriptional regulation of stem cell and cancer stem cell metabolism. Curr. Stem Cell Rep. 3, 19–27. doi: 10.1007/s40778-017-0071-y
Anderson, A. S., Roberts, P. C., Frisard, M. I., Hulver, M. W., and Schmelz, E. M. (2014). Ovarian tumor-initiating cells display a flexible metabolism. Exp. Cell Res. 328, 44–57. doi: 10.1016/j.yexcr.2014.08.028
Anderton, B., Camarda, R., Balakrishnan, S., Balakrishnan, A., Kohnz, R. A., Lim, L., et al. (2017). MYC-driven inhibition of the glutamate-cysteine ligase promotes glutathione depletion in liver cancer. EMBO Rep. 18, 569–585. doi: 10.15252/embr.201643068
Arlt, A., Sebens, S., Krebs, S., Geismann, C., Grossmann, M., Kruse, M. L., et al. (2013). Inhibition of the Nrf2 transcription factor by the alkaloid trigonelline renders pancreatic cancer cells more susceptible to apoptosis through decreased proteasomal gene expression and proteasome activity. Oncogene 32, 4825–4835. doi: 10.1038/onc.2012.493
Arnold, N. B., Ketterer, K., Kleeff, J., Friess, H., Büchler, M. W., and Korc, M. (2004). Thioredoxin is downstream of Smad7 in a pathway that promotes growth and suppresses cisplatin-induced apoptosis in pancreatic cancer. Cancer Res. 64, 3599–3606. doi: 10.1158/0008-5472.CAN-03-2999
Bailey, P., Chang, D. K., Nones, K., Johns, A. L., Patch, A. M., Gingras, M. C., et al. (2016). Genomic analyses identify molecular subtypes of pancreatic cancer. Nature 531, 47–52. doi: 10.1038/nature16965
Bao, B., Ali, S., Banerjee, S., Wang, Z., Logna, F., Azmi, A. S., et al. (2012). Curcumin analogue CDF inhibits pancreatic tumor growth by switching on suppressor microRNAs and attenuating EZH2 expression. Cancer Res. 72, 335–345. doi: 10.1158/0008-5472.CAN-11-2182
Batlle, E., and Clevers, H. (2017). Cancer stem cells revisited. Nat. Med. 23, 1124–1134. doi: 10.1038/nm.4409
Battula, V. L., Shi, Y., Evans, K. W., Wang, R.-Y., Spaeth, E. L., Jacamo, R. O., et al. (2012). Ganglioside GD2 identifies breast cancer stem cells and promotes tumorigenesis. J. Clin. Investig. 122, 2066–2078. doi: 10.1172/JCI59735
Bell, J. B., Eckerdt, F., Dhruv, H. D., Finlay, D., Peng, S., Kim, S., et al. (2018). Differential response of glioma stem cells to arsenic trioxide therapy is regulated by MNK1 and mRNA translation. Mol. Cancer Res. 16, 32–46. doi: 10.1158/1541-7786.MCR-17-0397
Beloribi-Djefaflia, S., Vasseur, S., and Guillaumond, F. (2016). Lipid metabolic reprogramming in cancer cells. Oncogenesis 5:e189. doi: 10.1038/oncsis.2015.49
Berruti, A., Bitossi, R., Gorzegno, G., Bottini, A., Alquati, P., De Matteis, A., et al. (2002). Time to progression in metastatic breast cancer patients treated with epirubicin is not improved by the addition of either cisplatin or lonidamine: final results of a phase III study with a factorial design. J. Clin. Oncol. 20, 4150–4159. doi: 10.1200/JCO.2002.08.012
Bhalla, S., Gordon, L. I., David, K., Prachand, S., Singh, A. T. K., Yang, S., et al. (2009). Glutathione depletion enhances arsenic trioxide-induced apoptosis in lymphoma cells through mitochondrial and caspase-independent mechanisms. Blood 114:2708.
Bigarella, C. L., Liang, R., and Ghaffari, S. (2014). Stem cells and the impact of ROS signaling. Development 141, 4206–4218. doi: 10.1242/dev.107086
Biswas, S., Dodwadkar, N. S., Deshpande, P. P., and Torchilin, V. P. (2012). Liposomes loaded with paclitaxel and modified with novel triphenylphosphonium-PEG-PE conjugate possess low toxicity, target mitochondria and demonstrate enhanced antitumor effects in vitro and in vivo. J. Controll. Release 159, 393–402. doi: 10.1016/j.jconrel.2012.01.009
Blum, R., and Kloog, Y. (2014). Metabolism addiction in pancreatic cancer. Cell Death Dis. 5:e1065. doi: 10.1038/cddis.2014.38
Boivin, A., Hanot, M., Malesys, C., Maalouf, M., Rousson, R., Rodriguez-Lafrasse, C., et al. (2011). Transient alteration of cellular redox buffering before irradiation triggers apoptosis in head and neck carcinoma stem and non-stem cells. PLoS One 6:e14558. doi: 10.1371/journal.pone.0014558
Bonuccelli, G., Tsirigos, A., Whitaker-Menezes, D., Pavlides, S., Pestell, R. G., Chiavarina, B., et al. (2010). Ketones and lactate “fuel” tumor growth and metastasis: evidence that epithelial cancer cells use oxidative mitochondrial metabolism. Cell Cycle 9, 3506–3514. doi: 10.4161/cc.9.17.12731
Bourguignon, L. Y., Wong, G., Earle, C., and Chen, L. (2012). Hyaluronan-CD44v3 interaction with Oct4-Sox2-Nanog promotes miR-302 expression leading to self-renewal, clonal formation, and cisplatin resistance in cancer stem cells from head and neck squamous cell carcinoma. J. Biol. Chem. 287, 32800–32824. doi: 10.1074/jbc.M111.308528
Boyer-Guittaut, M., Poillet, L., Liang, Q., Bôle-Richard, E., Ouyang, X., Benavides, G. A., et al. (2014). The role of GABARAPL1/GEC1 in autophagic flux and mitochondrial quality control in MDA-MB-436 breast cancer cells. Autophagy 10, 986–1003. doi: 10.4161/auto.28390
Brand, K. A., and Hermfisse, U. (1997). Aerobic glycolysis by proliferating cells: a protective strategy against reactive oxygen species. FASEB J. 11, 388–395. doi: 10.1096/fasebj.11.5.9141507
Brandi, J., Dando, I., Pozza, E. D., Biondani, G., Jenkins, R., Elliott, V., et al. (2017). Proteomic analysis of pancreatic cancer stem cells: functional role of fatty acid synthesis and mevalonate pathways. J. Proteomics 150, 310–322. doi: 10.1016/j.jprot.2016.10.002
Burnett, J. P., Lim, G., Li, Y., Shah, R. B., Lim, R., Paholak, H. J., et al. (2017). Sulforaphane enhances the anticancer activity of taxanes against triple negative breast cancer by killing cancer stem cells. Cancer Lett. 394, 52–64. doi: 10.1016/j.canlet.2017.02.023
Caino, M. C., Ghosh, J. C., Chae, Y. C., Vaira, V., Rivadeneira, D. B., Faversani, A., et al. (2015). PI3K therapy reprograms mitochondrial trafficking to fuel tumor cell invasion. Proc. Natl. Acad. Sci. U.S.A. 112, 8638–8643. doi: 10.1073/pnas.1500722112
Cairns, R. A., Harris, I. S., and Mak, T. W. (2011). Regulation of cancer cell metabolism. Nat. Rev. Cancer 11, 85–95. doi: 10.1038/nrc2981
Calderon-Aparicio, A., Strasberg-Rieber, M., and Rieber, M. (2015). Disulfiram anti-cancer efficacy without copper overload is enhanced by extracellular H2O2 generation: antagonism by tetrathiomolybdate. Oncotarget 6, 29771–29781. doi: 10.18632/oncotarget.4833
Camarda, R., Zhou, Z., Kohnz, R. A., Balakrishnan, S., Mahieu, C., Anderton, B., et al. (2016). Inhibition of fatty acid oxidation as a therapy for MYC-overexpressing triple-negative breast cancer. Nat. Med. 22, 427–432. doi: 10.1038/nm.4055
Caria, P., Tronci, L., Dettori, T., Murgia, F., Santoru, M. L., Griffin, L. L., et al. (2018). Metabolomic alterations in thyrospheres and adherent parental cells in papillary thyroid carcinoma cell lines: a pilot study. Int. J. Mol. Sci. 19:E2948. doi: 10.3390/ijms19102948
Caro, P., Kishan, A. U., Norberg, E., Stanley, I. A., Chapuy, B., Ficarro, S. B., et al. (2012). Metabolic signatures uncover distinct targets in molecular subsets of diffuse large B cell lymphoma. Cancer Cell 22, 547–560. doi: 10.1016/j.ccr.2012.08.014
Carracedo, A., Cantley, L. C., and Pandolfi, P. P. (2013). Cancer metabolism: fatty acid oxidation in the limelight. Nat. Rev. Cancer 13, 227–232. doi: 10.1038/nrc3483
Carracedo, A., Weiss, D., Leliaert, A. K., Bhasin, M., de Boer, V. C., Laurent, G., et al. (2012). A metabolic prosurvival role for PML in breast cancer. J. Clin. Investig. 122, 3088–3100. doi: 10.1172/JCI62129
Castellanos, J. A., Merchant, N. B., and Nagathihalli, N. S. (2013). Emerging targets in pancreatic cancer: epithelial-mesenchymal transition and cancer stem cells. Onco Targets Ther. 6, 1261–1267. doi: 10.2147/OTT.S34670
Cesari, R., Martin, E. S., Calin, G. A., Pentimalli, F., Bichi, R., McAdams, H., et al. (2003). Parkin, a gene implicated in autosomal recessive juvenile parkinsonism, is a candidate tumor suppressor gene on chromosome 6q25-q27. Proc. Natl. Acad. Sci. U.S.A. 100, 5956–5961. doi: 10.1073/pnas.0931262100
Chae, Y. C., Caino, M. C., Lisanti, S., Ghosh, J. C., Dohi, T., Danial, N. N., et al. (2012). Control of tumor bioenergetics and survival stress signaling by mitochondrial HSP90s. Cancer Cell 22, 331–344. doi: 10.1016/j.ccr.2012.07.015
Chamberlain, G. R., Tulumello, D. V., and Kelley, S. O. (2013). Targeted delivery of doxorubicin to mitochondria. ACS Chem. Biol. 8, 1389–1395. doi: 10.1021/cb400095v
Chandel, N. S., McClintock, D. S., Feliciano, C. E., Wood, T. M., Melendez, J. A., Rodriguez, A. M., et al. (2000). Reactive oxygen species generated at mitochondrial Complex III stabilize hypoxia-inducible factor-1α during hypoxia: a mechanism of O2-sensing. J. Biol. Chem. 275, 25130–25138. doi: 10.1074/jbc.M001914200
Chang, C. M., Lan, K. L., Huang, W. S., Lee, Y. J., Lee, T. W., Chang, C. H., et al. (2017). (188)Re-liposome can induce mitochondrial autophagy and reverse drug resistance for ovarian cancer: from bench evidence to preliminary clinical proof-of-concept. Int. J. Mol. Sci. 18:E903. doi: 10.3390/ijms18050903
Chang, C. W., Chen, Y. S., Tsay, Y. G., Han, C. L., Chen, Y. J., Yang, C. C., et al. (2018). ROS-independent ER stress-mediated NRF2 activation promotes warburg effect to maintain stemness-associated properties of cancer-initiating cells. Cell Death Dis. 9:194. doi: 10.1038/s41419-017-0250-x
Chang, K. J., Yang, M. H., Zheng, J. C., Li, B., and Nie, W. (2016). Arsenic trioxide inhibits cancer stem-like cells via down-regulation of Gli1 in lung cancer. Am. J. Transl. Res. 8, 1133–1143.
Chanmee, T., Ontong, P., Izumikawa, T., Higashide, M., Mochizuki, N., Chokchaitaweesuk, C., et al. (2016). Hyaluronan production regulates metabolic and cancer stem-like properties of breast cancer cells via hexosamine biosynthetic pathway-coupled HIF-1 signaling. J. Biol. Chem. 291, 24105–24120. doi: 10.1074/jbc.M116.751263
Chanmee, T., Ontong, P., Mochizuki, N., Kongtawelert, P., Konno, K., and Itano, N. (2014). Excessive hyaluronan production promotes acquisition of cancer stem cell signatures through the coordinated regulation of twist and the transforming growth factor β (TGF-β)-snail signaling axis. J. Biol. Chem. 289, 26028–26056. doi: 10.1074/jbc.M114.564120
Chen, C. L., Uthaya Kumar, D. B., Punj, V., Xu, J., Sher, L., Tahara, S. M., et al. (2016). NANOG metabolically reprograms tumor-initiating stem-like cells through tumorigenic changes in oxidative phosphorylation and fatty acid metabolism. Cell Metab. 23, 206–219. doi: 10.1016/j.cmet.2015.12.004
Chen, X., Song, M., Zhang, B., and Zhang, Y. (2016). Reactive oxygen species regulate T cell immune response in the tumor microenvironment. Oxid. Med. Cell. Longev. 2016:1580967. doi: 10.1155/2016/1580967
Chen, C. T., Shih, Y. R., Kuo, T. K., Lee, O. K., and Wei, Y. H. (2008). Coordinated changes of mitochondrial biogenesis and antioxidant enzymes during osteogenic differentiation of human mesenchymal stem cells. Stem Cells 26, 960–968. doi: 10.1634/stemcells.2007-0509
Chen, H., and Chan, D. C. (2017). Mitochondrial dynamics in regulating the unique phenotypes of cancer and stem cells. Cell Metab. 26, 39–48. doi: 10.1016/j.cmet.2017.05.016
Chen, K. Y., Liu, X., Bu, P., Lin, C. S., Rakhilin, N., Locasale, J. W., et al. (2014). A metabolic signature of colon cancer initiating cells. Conf. Proc. IEEE Eng. Med. Biol. Soc. 2014, 4759–4762. doi: 10.1109/EMBC.2014.6944688
Cheng, G., Zielonka, J., McAllister, D. M., Mackinnon, A. C. Jr., Joseph, J., Dwinell, M. B., et al. (2013). Mitochondria-targeted vitamin E analogs inhibit breast cancer cell energy metabolism and promote cell death. BMC Cancer 13:285. doi: 10.1186/1471-2407-13-285
Cheng, J., Li, W., Kang, B., Zhou, Y., Song, J., Dan, S., et al. (2015). Tryptophan derivatives regulate the transcription of Oct4 in stem-like cancer cells. Nat. Commun. 6:7209. doi: 10.1038/ncomms8209
Cho, Y. M., Kwon, S., Pak, Y. K., Seol, H. W., Choi, Y. M., Park, D. J., et al. (2006). Dynamic changes in mitochondrial biogenesis and antioxidant enzymes during the spontaneous differentiation of human embryonic stem cells. Biochem. Biophys. Res. Commun. 348, 1472–1478. doi: 10.1016/j.bbrc.2006.08.020
Choi, Y. J., Gurunathan, S., and Kim, J. H. (2018). Graphene oxide-silver nanocomposite enhances cytotoxic and apoptotic potential of salinomycin in human ovarian cancer stem cells (OvCSCs): a novel approach for cancer therapy. Int. J. Mol. Sci. 19:E710. doi: 10.3390/ijms19030710
Chourasia, A. H., Boland, M. L., and Macleod, K. F. (2015a). Mitophagy and cancer. Cancer Metab. 3:4. doi: 10.1186/s40170-015-0130-8
Chourasia, A. H., Tracy, K., Frankenberger, C., Boland, M. L., Sharifi, M. N., Drake, L. E., et al. (2015b). Mitophagy defects arising from BNip3 loss promote mammary tumor progression to metastasis. EMBO Rep. 16, 1145–1163. doi: 10.15252/embr.201540759
Chung, V., McDonough, S., Philip, P. A., Cardin, D., Wang-Gillam, A., Hui, L., et al. (2017). Effect of selumetinib and MK-2206 vs oxaliplatin and fluorouracil in patients with metastatic pancreatic cancer after prior therapy. JAMA Oncol. 3, 516–522. doi: 10.1001/jamaoncol.2016.5383
Ciavardelli, D., Rossi, C., Barcaroli, D., Volpe, S., Consalvo, A., Zucchelli, M., et al. (2014). Breast cancer stem cells rely on fermentative glycolysis and are sensitive to 2-deoxyglucose treatment. Cell Death Dis. 5:e1336. doi: 10.1038/cddis.2014.285
Coleman, M. C., Asbury, C. R., Daniels, D., Du, J., Aykin-Burns, N., Smith, B. J., et al. (2008). 2-Deoxy-d-glucose causes cytotoxicity, oxidative stress, and radiosensitization in pancreatic cancer. Free Radic. Biol. Med. 44, 322–331. doi: 10.1016/j.freeradbiomed.2007.08.032
Corominas-Faja, B., Cuyàs, E., Gumuzio, J., Bosch-Barrera, J., Leis, O., Martin, A. G., et al. (2014). Chemical inhibition of acetyl-CoA carboxylase suppresses self-renewal growth of cancer stem cells. Oncotarget 5, 8306–8316. doi: 10.18632/oncotarget.2059
Corominas-Faja, B., Vellon, L., Cuyàs, E., Buxó, M., Martin-Castillo, B., Serra, D., et al. (2017). Clinical and therapeutic relevance of the metabolic oncogene fatty acid synthase in HER2+ breast cancer. Histol. Histopathol. 32, 687–698. doi: 10.14670/HH-11-830
Daniëls, V. W., Smans, K., Royaux, I., Chypre, M., Swinnen, J. V., Zaidi, N., et al. (2014). Cancer cells differentially activate and thrive on de novo lipid synthesis pathways in a low-lipid environment. PLoS One 9:e106913. doi: 10.1371/journal.pone.0106913
Davison, K., Côté, S., Mader, S., and Miller, W. H. (2003). Glutathione depletion overcomes resistance to arsenic trioxide in arsenic-resistant cell lines. Leukemia 17, 931–940. doi: 10.1038/sj.leu.2402876
Davis-Yadley, A. H., Abbott, A. M., Pimiento, J. M., Chen, D. T., and Malafa, M. P. (2016). Increased expression of the glucose transporter type 1 gene is associated with worse overall survival in resected pancreatic adenocarcinoma. Pancreas 45, 974–979. doi: 10.1097/MPA.0000000000000580
De Berardinis, R. J., and Chandel, N. S. (2016). Fundamentals of cancer metabolism. Sci. Adv. 2:e1600200. doi: 10.1126/sciadv.1600200
De Carlo, F., Witte, T. R., Hardman, W. E., and Claudio, P. P. (2013). Omega-3 eicosapentaenoic acid decreases CD133 colon cancer stem-like cell marker expression while increasing sensitivity to chemotherapy. PLoS One 8:e69760. doi: 10.1371/journal.pone.0069760
De Francesco, E. M., Maggiolini, M., Tanowitz, H. B., Sotgia, F., and Lisanti, M. P. (2017). Targeting hypoxic cancer stem cells (CSCs) with Doxycycline: implications for optimizing anti-angiogenic therapy. Oncotarget 8, 56126–56142. doi: 10.18632/oncotarget.18445
De Francesco, E. M., Sotgia, F., and Lisanti, M. P. (2018). Cancer stem cells (CSCs): metabolic strategies for their identification and eradication. Biochem. J. 475, 1611–1634. doi: 10.1042/BCJ20170164
De Lena, M., Lorusso, V., Bottalico, C., Brandi, M., De Mitrio, A., Catino, A., et al. (1997). Revertant and potentiating activity of lonidamine in patients with ovarian cancer previously treated with platinum. J. Clin. Oncol. 15, 3208–3213. doi: 10.1200/JCO.1997.15.10.3208
De Luca, A., Fiorillo, M., Peiris-Pagès, M., Ozsvari, B., Smith, D. L., Sanchez-Alvarez, R., et al. (2015). Mitochondrial biogenesis is required for the anchorage-independent survival and propagation of stem-like cancer cells. Oncotarget 6, 14777–14795. doi: 10.18632/oncotarget.4401
De Marinis, F., Rinaldi, M., Ardizzoni, A., Bruzzi, P., Pennucci, M. C., Portalone, L., et al. (1999). The role of vindesine and lonidamine in the treatment of elderly patients with advanced non-small cell lung cancer: a phase III randomized fonicap trial. Tumori 85, 177–182. doi: 10.1177/030089169908500306
Debeb, B. G., Lacerda, L., Larson, R., Wolfe, A. R., Krishnamurthy, S., Reuben, J. M., et al. (2016). Histone deacetylase inhibitor-induced cancer stem cells exhibit high pentose phosphate pathway metabolism. Oncotarget 7, 28329–28339. doi: 10.18632/oncotarget.8631
Deberardinis, R. J., and Cheng, T. (2010). Qs next: the diverse functions of glutamine in metabolism, cell biology and cancer. Oncogene 29, 313–324. doi: 10.1038/onc.2009.358
Delmore, J. E., Issa, G. C., Lemieux, M. E., Rahl, P. B., Shi, J., Jacobs, H. M., et al. (2011). BET bromodomain inhibition as a therapeutic strategy to target c-Myc. Cell 146, 904–917. doi: 10.1016/j.cell.2011.08.017
Deshmukh, A., Arfuso, F., Newsholme, P., and Dharmarajan, A. (2018). Regulation of cancer stem cell metabolism by secreted frizzled-related protein 4 (sFRP4). Cancers 10:E40. doi: 10.3390/cancers10020040
Dey-Guha, I., Wolfer, A., Yeh, A. C., G Albeck, J., Darp, R., Leon, E., et al. (2011). Asymmetric cancer cell division regulated by AKT. Proc. Natl. Acad. Sci. U.S.A. 108, 12845–12850. doi: 10.1073/pnas.1109632108
Diehn, M., Cho, R. W., Lobo, N. A., Kalisky, T., Dorie, M. J., Kulp, A. N., et al. (2009). Association of reactive oxygen species levels and radioresistance in cancer stem cells. Nature 458, 780–783. doi: 10.1038/nature07733
Ding, D., Lim, K. S., and Eberhart, C. G. (2014). Arsenic trioxide inhibits Hedgehog, Notch and stem cell properties in glioblastoma neurospheres. Acta Neuropathol. Commun. 2:31. doi: 10.1186/2051-5960-2-31
Doherty, J. R., Yang, C., Scott, K. E., Cameron, M. D., Fallahi, M., Li, W., et al. (2014). Blocking lactate export by inhibiting the Myc target MCT1 disables glycolysis and glutathione synthesis. Cancer Res. 74, 908–920. doi: 10.1158/0008-5472.CAN-13-2034
Dong, C., Yuan, T., Wu, Y., Wang, Y., Fan, T. W., Miriyala, S., et al. (2013). Loss of FBP1 by snail-mediated repression provides metabolic advantages in basal-like breast cancer. Cancer Cell 23, 316–331. doi: 10.1016/j.ccr.2013.01.022
Du, J., Nelson, E. S., Simons, A. L., Olney, K. E., Moser, J. C., Schrock, H. E., et al. (2013). Regulation of pancreatic cancer growth by superoxide. Mol. Carcinog. 52, 555–567. doi: 10.1002/mc.21891
Du, Y., Zhao, B., Liu, Z., Ren, X., Zhao, W., Li, Z., et al. (2017). Molecular subtyping of pancreatic cancer: translating genomics and transcriptomics into the clinic. J. Cancer 8, 513–522. doi: 10.7150/jca.17622
Dubrovska, A., Kim, S., Salamone, R. J., Walker, J. R., Maira, S. M., García-Echeverría, C., et al. (2009). The role of PTEN/Akt/PI3K signaling in the maintenance and viability of prostate cancer stem-like cell populations. Proc. Natl. Acad. Sci. U.S.A. 106, 268–273. doi: 10.1073/pnas.0810956106
Ehsanipour, E. A., Sheng, X., Behan, J. W., Wang, X., Butturini, A., Avramis, V. I., et al. (2013). Adipocytes cause leukemia cell resistance to l-asparaginase via release of glutamine. Cancer Res. 73, 2998–3006. doi: 10.1158/0008-5472.CAN-12-4402
Elhammali, A., Ippolito, J. E., Collins, L., Crowley, J., Marasa, J., and Piwnica-Worms, D. (2014). A high-throughput fluorimetric assay for 2-hydroxyglutarate identifies zaprinast as a glutaminase inhibitor. Cancer Discov. 4, 828–839. doi: 10.1158/2159-8290.CD-13-0572
Ellwood-Yen, K., Graeber, T. G., Wongvipat, J., Iruela-Arispe, M. L., Zhang, J., Matusik, R., et al. (2003). Myc-driven murine prostate cancer shares molecular features with human prostate tumors. Cancer Cell 4, 223–238. doi: 10.1016/S1535-6108(03)00197-1
Erdogan, S., Turkekul, K., Serttas, R., and Erdogan, Z. (2017). The natural flavonoid apigenin sensitizes human CD44 + prostate cancer stem cells to cisplatin therapy. Biomed. Pharmacother. 88, 210–217. doi: 10.1016/j.biopha.2017.01.056
Erickson, K. L., and Hubbard, N. E. (2010). Fatty acids and breast cancer: the role of stem cells. Prostaglandins Leukot. Essent. Fatty Acids 82, 237–241. doi: 10.1016/j.plefa.2010.02.019
Esner, M., Graifer, D., Lleonart, M. E., and Lyakhovich, A. (2017). Targeting cancer cells through antibiotics-induced mitochondrial dysfunction requires autophagy inhibition. Cancer Lett. 384, 60–69. doi: 10.1016/j.canlet.2016.09.023
Estrela, J. M., Ortega, A., and Obrador, E. (2006). Glutathione in cancer biology and therapy. Crit. Rev. Clin. Lab. Sci. 43, 143–181. doi: 10.1080/10408360500523878
Fan, J., Kamphorst, J. J., Mathew, R., Chung, M. K., White, E., Shlomi, T., et al. (2013). Glutamine-driven oxidative phosphorylation is a major ATP source in transformed mammalian cells in both normoxia and hypoxia. Mol. Syst. Biol. 9:712. doi: 10.1038/msb.2013.65
Fiorillo, M., Lamb, R., Tanowitz, H. B., Cappello, A. R., Martinez-Outschoorn, U. E., Sotgia, F., et al. (2016). Bedaquiline, an FDA-approved antibiotic, inhibits mitochondrial function and potently blocks the proliferative expansion of stem-like cancer cells (CSCs). Aging 8, 1593–1607. doi: 10.18632/aging.100983
Fiorillo, M., Peiris-Pagès, M., Sanchez-Alvarez, R., Bartella, L., Di Donna, L., Dolce, V., et al. (2018). Bergamot natural products eradicate cancer stem cells (CSCs) by targeting mevalonate, Rho-GDI-signalling and mitochondrial metabolism. Biochim. Biophys. Acta 1859, 984–996. doi: 10.1016/j.bbabio.2018.03.018
Fischer, K., Hoffmann, P., Voelkl, S., Meidenbauer, N., Ammer, J., Edinger, M., et al. (2007). Inhibitory effect of tumor cell-derived lactic acid on human T cells. Blood 109, 3812–3819. doi: 10.1182/blood-2006-07-035972
Flaig, T. W., Gustafson, D. L., Su, L. J., Zirrolli, J. A., Crighton, F., Harrison, G. S., et al. (2006). A phase I and pharmacokinetic study of silybin-phytosome in prostate cancer patients. Invest. New Drugs 25, 139–146. doi: 10.1007/s10637-006-9019-2
Folmes, C. D., Nelson, T. J., Martinez-Fernandez, A., Arrell, D. K., Lindor, J. Z., Dzeja, P. P., et al. (2011). Somatic oxidative bioenergetics transitions into pluripotency-dependent glycolysis to facilitate nuclear reprogramming. Cell Metab. 14, 264–271. doi: 10.1016/j.cmet.2011.06.011
Gabay, M., Li, Y., and Felsher, D. W. (2014). MYC activation is a hallmark of cancer initiation and maintenance. Cold Spring Harb. Perspect. Med. 4:a014241. doi: 10.1101/cshperspect.a014241
Gadducci, A., Brunetti, I., Muttini, M. P., Fanucchi, A., Dargenio, F., Giannessi, P. G., et al. (1994). Epidoxorubicin and lonidamine in refractory or recurrent epithelial ovarian cancer. Eur. J. Cancer 30, 1432–1435. doi: 10.1016/0959-8049(94)00231-S
Gaglio, D., Metallo, C. M., Gameiro, P. A., Hiller, K., Danna, L. S., Balestrieri, C., et al. (2011). Oncogenic K-Ras decouples glucose and glutamine metabolism to support cancer cell growth. Mol. Syst. Biol. 7:523. doi: 10.1038/msb.2011.56
Gammon, L., Biddle, A., Heywood, H. K., Johannessen, A. C., and Mackenzie, I. C. (2013). Sub-sets of cancer stem cells differ intrinsically in their patterns of oxygen metabolism. PLoS One 8:e62493. doi: 10.1371/journal.pone.0062493
Garcia, P. L., Miller, A. L., Kreitzburg, K. M., Council, L. N., Gamblin, T. L., Christein, J. D., et al. (2016). The BET bromodomain inhibitor JQ1 suppresses growth of pancreatic ductal adenocarcinoma in patient-derived xenograft models. Oncogene 35, 833–845. doi: 10.1038/onc.2015.126
Gatenby, R. A., and Gillies, R. J. (2004). Why do cancers have high aerobic glycolysis? Nat. Rev. Cancer 4, 891–899. doi: 10.1038/nrc1478
Gaude, E., and Frezza, C. (2016). Tissue-specific and convergent metabolic transformation of cancer correlates with metastatic potential and patient survival. Nat. Commun. 7:13041. doi: 10.1038/ncomms13041
Giacomelli, C., Daniele, S., Natali, L., Iofrida, C., Flamini, G., Braca, A., et al. (2017). Carnosol controls the human glioblastoma stemness features through the epithelial-mesenchymal transition modulation and the induction of cancer stem cell apoptosis. Sci. Rep. 7:15174. doi: 10.1038/s41598-017-15360-2
Giampietri, C., Petrungaro, S., Cordella, M., Tabolacci, C., Tomaipitinca, L., Facchiano, A., et al. (2017). Lipid storage and autophagy in melanoma cancer cells. Int. J. Mol. Sci. 18:E1271. doi: 10.3390/ijms18061271
Ginestier, C., Monville, F., Wicinski, J., Cabaud, O., Cervera, N., Josselin, E., et al. (2012). Mevalonate metabolism regulates basal breast cancer stem cells and is a potential therapeutic target. Stem Cells 30, 1327–1337. doi: 10.1002/stem.1122
Goidts, V., Bageritz, J., Puccio, L., Nakata, S., Zapatka, M., Barbus, S., et al. (2012). RNAi screening in glioma stem-like cells identifies PFKFB4 as a key molecule important for cancer cell survival. Oncogene 31, 3235–3243. doi: 10.1038/onc.2011.490
Guha, M., Srinivasan, S., Ruthel, G., Kashina, A. K., Carstens, R. P., Mendoza, A., et al. (2014). Mitochondrial retrograde signaling induces epithelial–mesenchymal transition and generates breast cancer stem cells. Oncogene 33, 5238–5250. doi: 10.1038/onc.2013.467
Guillaumond, F., Bidaut, G., Ouaissi, M., Servais, S., Gouirand, V., Olivares, O., et al. (2015). Cholesterol uptake disruption, in association with chemotherapy, is a promising combined metabolic therapy for pancreatic adenocarcinoma. Proc. Natl. Acad. Sci. U.S.A. 112, 2473–2478. doi: 10.1073/pnas.1421601112
Hale, J. S., Otvos, B., Sinyuk, M., Alvarado, A. G., Hitomi, M., Stoltz, K., et al. (2014). Cancer stem cell-specific scavenger receptor CD36 drives glioblastoma progression. Stem Cells 32, 1746–1758. doi: 10.1002/stem.1716
Han, M., Vakili, M. R., Soleymani Abyaneh, H., Molavi, O., Lai, R., and Lavasanifar, A. (2014). Mitochondrial delivery of doxorubicin via triphenylphosphine modification for overcoming drug resistance in MDA-MB-435/DOX cells. Mol. Pharmacol. 11, 2640–2649. doi: 10.1021/mp500038g
Hanahan, D., and Weinberg, R. A. (2011). Hallmarks of cancer: the next generation. Cell 144, 646–674. doi: 10.1016/j.cell.2011.02.013
Haq, R., Shoa, G. J., Andreu-Perez, P., Yokoyama, S., Edelman, H., Rowe, G. C., et al. (2013). Oncogenic BRAF regulates oxidative metabolism via PGC1α and MITF. Cancer Cell 23, 302–315. doi: 10.1016/j.ccr.2013.02.003
Harris, I. S., Treloar, A. E., Inoue, S., Sasaki, M., Gorrini, C., Lee, K. C., et al. (2015). Glutathione and thioredoxin antioxidant pathways synergize to drive cancer initiation and progression. Cancer Cell 27, 211–222. doi: 10.1016/j.ccell.2014.11.019
Hawkins, K. E., Joy, S., Delhove, J. M., Kotiadis, V. N., Fernandez, E., Fitzpatrick, L. M., et al. (2016). NRF2 orchestrates the metabolic shift during induced pluripotent stem cell reprogramming. Cell Rep. 14, 1883–1891. doi: 10.1016/J.CELREP.2016.02.003
He, H., Nilsson, C. L., Emmett, M. R., Marshall, A. G., Kroes, R. A., Moskal, J. R., et al. (2010). Glycomic and transcriptomic response of GSC11 glioblastoma stem cells to STAT3 phosphorylation inhibition and serum-induced differentiation. J. Proteome Res. 9, 2098–2108. doi: 10.1021/pr900793a
He, T.-L., Zhang, Y. J., Jiang, H., Li, X. H., Zhu, H., and Zheng, K. L. (2015). The c-Myc–LDHA axis positively regulates aerobic glycolysis and promotes tumor progression in pancreatic cancer. Med. Oncol. 32:187. doi: 10.1007/s12032-015-0633-8
Hegde, S., Kaushal, N., Ravindra, K. C., Chiaro, C., Hafer, K. T., Gandhi, U. H., et al. (2011). Δ 12-prostaglandin J 3, an omega-3 fatty acid-derived metabolite, selectively ablates leukemia stem cells in mice. Blood 118, 6909–6919. doi: 10.1182/blood-2010-11-317750
Heiss, E. H., Schachner, D., Zimmermann, K., and Dirsch, V. M. (2013). Glucose availability is a decisive factor for Nrf2-mediated gene expression. Redox Biol. 1, 359–365. doi: 10.1016/j.redox.2013.06.001
Hensley, C. T., Faubert, B., Yuan, Q., Lev-Cohain, N., Jin, E., Kim, J., et al. (2016). Metabolic heterogeneity in human lung tumors. Cell 164, 681–694. doi: 10.1016/j.cell.2015.12.034
Hermann, P. C., Huber, S. L., Herrler, T., Aicher, A., Ellwart, J. W., Guba, M., et al. (2007). Distinct populations of cancer stem cells determine tumor growth and metastatic activity in human pancreatic cancer. Cell Stem Cell 1, 313–323. doi: 10.1016/j.stem.2007.06.002
Hirata, N., Yamada, S., Shoda, T., Kurihara, M., Sekino, Y., and Kanda, Y. (2015). Sphingosine-1-phosphate promotes expansion of cancer stem cells via S1PR3 by a ligand-independent Notch activation. Nat. Commun. 5:4806. doi: 10.1038/ncomms5806
Horton, J. D. (2002). Sterol regulatory element-binding proteins: transcriptional activators of lipid synthesis. Biochem. Soc. Trans. 30(Pt 6), 1091–1095. doi: 10.1042/bst0301091
Hou, J., Yu, X., Shen, Y., Shi, Y., Su, C., and Zhao, L. (2017). Triphenylphosphine-functionalized chitosan nanoparticles enhanced antitumor efficiency through targeted delivery of doxorubicin to mitochondria. Nanoscale Res. Lett. 12:158. doi: 10.1186/s11671-017-1931-1
Hu, W., Zhang, C., Wu, R., Sun, Y., Levine, A., and Feng, Z. (2010). Glutaminase 2, a novel p53 target gene regulating energy metabolism and antioxidant function. Proc. Natl. Acad. Sci. U.S.A. 107, 7455–7460. doi: 10.1073/pnas.1001006107
Hu, X., Ghisolfi, L., Keates, A. C., Zhang, J., Xiang, S., Lee, D. K., et al. (2012). Induction of cancer cell stemness by chemotherapy. Cell Cycle 11, 2691–2698. doi: 10.4161/cc.21021
Iliopoulos, D., Hirsch, H. A., and Struhl, K. (2011). Metformin decreases the dose of chemotherapy for prolonging tumor remission in mouse xenografts involving multiple cancer cell types. Cancer Res. 71, 3196–3201. doi: 10.1158/0008-5472.CAN-10-3471
Infante, J. R., Somer, B. G., Park, J. O., Li, C. P., Scheulen, M. E., Kasubhai, S. M., et al. (2014). A randomised, double-blind, placebo-controlled trial of trametinib, an oral MEK inhibitor, in combination with gemcitabine for patients with untreated metastatic adenocarcinoma of the pancreas. Eur. J. Cancer 50, 2072–2081. doi: 10.1016/j.ejca.2014.04.024
Ishikawa, K., Takenaga, K. M., Akimoto, M. M., Koshikawa, N., Yamaguchi, A., Imanishi, H., et al. (2008). ROS-generating mitochondrial DNA mutations can regulate tumor cell metastasis. Science 320, 661–664. doi: 10.1126/science.1156906
Ishimoto, T., Nagano, O., Yae, T., Tamada, M., Motohara, T., Oshima, H., et al. (2011). CD44 variant regulates redox status in cancer cells by stabilizing the xCT subunit of system xc-and thereby promotes tumor growth. Cancer Cell 19, 387–400. doi: 10.1016/j.ccr.2011.01.038
Ito, K., Carracedo, A., Weiss, D., Arai, F., Ala, U., Avigan, D. E., et al. (2012). A PML-PPAR-δ pathway for fatty acid oxidation regulates hematopoietic stem cell maintenance. Nat. Med. 18, 1350–1358. doi: 10.1038/nm.2882
Ito, K., Hirao, A., Arai, F., Matsuoka, S., Takubo, K., Hamaguchi, I., et al. (2004). Regulation of oxidative stress by ATM is required for self-renewal of haematopoietic stem cells. Nature 431, 997–1002. doi: 10.1038/nature02989
Ito, K., Hirao, A., Arai, F., Takubo, K., Matsuoka, S., Miyamoto, K., et al. (2006). Reactive oxygen species act through p38 MAPK to limit the lifespan of hematopoietic stem cells. Nat. Med. 12, 446–451. doi: 10.1038/nm1388
Izumiya, M., Kabashima, A., Higuchi, H., Igarashi, T., Sakai, G., Iizuka, H., et al. (2012). Chemoresistance is associated with cancer stem cell-like properties and epithelial-to-mesenchymal transition in pancreatic cancer cells. Anticancer Res. 32, 3847–3853.
Izzo, V., Bravo-San Pedro, J. M., Sica, V., Kroemer, G., and Galluzzi, L. (2016). Mitochondrial permeability transition: new findings and persisting uncertainties. Trends Cell Biol. 26, 655–667. doi: 10.1016/j.tcb.2016.04.006
Jang, Y. Y., and Sharkis, S. J. (2007). A low level of reactive oxygen species selects for primitive hematopoietic stem cells that may reside in the low-oxygenic niche. Blood 110, 3056–3063. doi: 10.1182/blood-2007-05-087759
Jangamreddy, J. R., Ghavami, S., Grabarek, J., Kratz, G., Wiechec, E., Fredriksson, B. A., et al. (2013). Salinomycin induces activation of autophagy, mitophagy and affects mitochondrial polarity: differences between primary and cancer cells. Biochim. Biophys. Acta 1833, 2057–2069. doi: 10.1016/j.bbamcr.2013.04.011
Janiszewska, M., Suvà, M. L., Riggi, N., Houtkooper, R. H., Auwerx, J., Clément-Schatlo, V., et al. (2012). Imp2 controls oxidative phosphorylation and is crucial for preserving glioblastoma cancer stem cells. Genes Dev. 26, 1926–1944. doi: 10.1101/gad.188292.112
Jiang, H., Wu, L., Mishra, M., Chawsheen, H. A., and Wei, Q. (2014). Expression of peroxiredoxin 1 and 4 promotes human lung cancer malignancy. Am. J. Cancer Res. 4, 445–460.
Jones, C. L., Stevens, B. M., DAlessandro, A., Reisz, J. A., Culp-Hill, R., Nemkov, T., et al. (2018). Inhibition of amino acid metabolism selectively targets human leukemia stem cells. Cancer Cell 34, 724–740.e4. doi: 10.1016/j.ccell.2018.10.005
Jose, C., Bellance, N., and Rossignol, R. (2011). Choosing between glycolysis and oxidative phosphorylation: a tumors dilemma? Biochim. Biophys. Acta 1807, 552–561. doi: 10.1016/j.bbabio.2010.10.012
Kakarala, M., Brenner, D. E., Korkaya, H., Cheng, C., Tazi, K., Ginestier, C., et al. (2010). Targeting breast stem cells with the cancer preventive compounds curcumin and piperine. Breast Cancer Res. Treat. 122, 777–785. doi: 10.1007/s10549-009-0612-x
Kamata, H., Honda, S., Maeda, S., Chang, L., Hirata, H., and Karin, M. (2005). Reactive oxygen species promote TNFα-induced death and sustained JNK activation by inhibiting MAP kinase phosphatases. Cell 120, 649–661. doi: 10.1016/j.cell.2004.12.041
Kamphorst, J. J., Cross, J. R., Fan, J., de Stanchina, E., Mathew, R., White, E. P., et al. (2013). Hypoxic and Ras-transformed cells support growth by scavenging unsaturated fatty acids from lysophospholipids. Proc. Natl. Acad. Sci. U.S.A. 110, 8882–8887. doi: 10.1073/pnas.1307237110
Kamphorst, J. J., Nofal, M., Commisso, C., Hackett, S. R., Lu, W., Grabocka, E., et al. (2015). Human pancreatic cancer tumors are nutrient poor and tumor cells actively scavenge extracellular protein. Cancer Res. 75, 544–553. doi: 10.1158/0008-5472.CAN-14-2211
Kang, R., Tang, D., Schapiro, N. E., Loux, T., Livesey, K. M., Billiar, T. R., et al. (2014). The HMGB1/RAGE inflammatory pathway promotes pancreatic tumor growth by regulating mitochondrial bioenergetics. Oncogene 33, 567–577. doi: 10.1038/onc.2012.631
Kashatus, J. A., Nascimento, A., Myers, L. J., Sher, A., Byrne, F. L., Hoehn, K. L., et al. (2015). Erk2 phosphorylation of Drp1 promotes mitochondrial fission and MAPK-driven tumor growth. Mol. Cell 57, 537–551. doi: 10.1016/j.molcel.2015.01.002
Katajisto, P., Döhla, J., Chaffer, C. L., Pentinmikko, N., Marjanovic, N., Iqbal, S., et al. (2015). Asymmetric apportioning of aged mitochondria between daughter cells is required for stemness. Science 348, 340–343. doi: 10.1126/science.1260384
Kathagen, A., Schulte, A., Balcke, G., Phillips, H. S., Martens, T., Matschke, J., et al. (2013). Hypoxia and oxygenation induce a metabolic switch between pentose phosphate pathway and glycolysis in glioma stem-like cells. Acta Neuropathol. 126, 763–780. doi: 10.1007/s00401-013-1173-y
Kennedy, C. R., Tilkens, S. B., Guan, H., Garner, J. A., Or, P. M., and Chan, A. M. (2013). Differential sensitivities of glioblastoma cell lines towards metabolic and signaling pathway inhibitions. Cancer Lett. 336, 299–306. doi: 10.1016/j.canlet.2013.03.020
Kim, B., Jung, N., Lee, S., Sohng, J. K., and Jung, H. J. (2016). Apigenin inhibits cancer stem cell-like phenotypes in human glioblastoma cells via suppression of c-met signaling. Phytother. Res. 30, 1833–1840. doi: 10.1002/ptr.5689
Kim, D., Choi, B. H., Ryoo, I. G., and Kwak, M. K. (2018). High NRF2 level mediates cancer stem cell-like properties of aldehyde dehydrogenase (ALDH)-high ovarian cancer cells: inhibitory role of all-trans retinoic acid in ALDH/NRF2 signaling. Cell Death Dis. 9:896. doi: 10.1038/s41419-018-0903-4
Kim, J. H., Lee, K. J., Seo, Y., Kwon, J. H., Yoon, J. P., Kang, J. Y., et al. (2018). Effects of metformin on colorectal cancer stem cells depend on alterations in glutamine metabolism. Sci. Rep. 8:409. doi: 10.1038/s41598-017-18762-4
Kim, J., and Keum, Y. S. (2016). NRF2, a key regulator of antioxidants with two faces towards cancer. Oxid. Med. Cell. Longev. 2016:2746457. doi: 10.1155/2016/2746457
Kim, J. W., Gao, P., Liu, Y. C., Semenza, G. L., and Dang, C. V. (2007). Hypoxia-inducible factor 1 and dysregulated c-Myc cooperatively induce vascular endothelial growth factor and metabolic switches hexokinase 2 and pyruvate dehydrogenase kinase 1. Mol. Cell. Biol. 27, 7381–7393. doi: 10.1128/MCB.00440-07
Kim, Y. J., Kim, J. Y., Lee, N., Oh, E., Sung, D., Cho, T. M., et al. (2017). Disulfiram suppresses cancer stem-like properties and STAT3 signaling in triple-negative breast cancer cells. Biochem. Biophys. Res. Commun. 486, 1069–1076. doi: 10.1016/j.bbrc.2017.03.164
Kindler, H. L., Wroblewski, K., Wallace, J. A., Hall, M. J., Locker, G., Nattam, S., et al. (2012). Gemcitabine plus sorafenib in patients with advanced pancreatic cancer: a phase II trial of the University of Chicago Phase II Consortium. Investig. New Drugs 30, 382–386. doi: 10.1007/s10637-010-9526-z
Kobayashi, C. I., and Suda, T. (2012). Regulation of reactive oxygen species in stem cells and cancer stem cells. J. Cell. Physiol. 227, 421–430. doi: 10.1002/jcp.22764
Korangath, P., Teo, W. W., Sadik, H., Han, L., Mori, N., Huijts, C. M., et al. (2015). Targeting glutamine metabolism in breast cancer with aminooxyacetate. Clin. Cancer Res. 21, 3263–3273. doi: 10.1158/1078-0432.CCR-14-1200
Kordes, S., Pollak, M. N., Zwinderman, A. H., Mathôt, R. A., Weterman, M. J., Beeker, A., et al. (2015). Metformin in patients with advanced pancreatic cancer: a double-blind, randomised, placebo-controlled phase 2 trial. Lancet Oncol. 16, 839–847. doi: 10.1016/S1470-2045(15)00027-3
Kroemer, G., and Pouyssegur, J. (2008). Tumor cell metabolism: cancers Achilles heel. Cancer Cell 13, 472–482. doi: 10.1016/j.ccr.2008.05.005
Kudryavtseva, A. V., Krasnov, G. S., Dmitriev, A. A., Alekseev, B. Y., Kardymon, O. L., Sadritdinova, A. F., et al. (2016). Mitochondrial dysfunction and oxidative stress in aging and cancer. Oncotarget 7, 44879–44905. doi: 10.18632/oncotarget.9821
Kurtova, A. V., Xiao, J., Mo, Q., Pazhanisamy, S., Krasnow, R., Lerner, S. P., et al. (2015). Blocking PGE2-induced tumour repopulation abrogates bladder cancer chemoresistance. Nature 517, 209–213. doi: 10.1038/nature14034
Kwak, M. K., Itoh, K., Yamamoto, M., Sutter, T. R., and Kensler, T. W. (2001). Role of transcription factor Nrf2 in the induction of hepatic phase 2 and antioxidative enzymes in vivo by the cancer chemoprotective agent, 3H-1, 2-dimethiole-3-thione. Mol. Med. 7, 135–145. doi: 10.1007/BF03401947
Kwak, M.-K., Ryoo, I., and Lee, S. (2016). Activation of NRF2 signaling in breast cancer stem cell-enriched population. Free Radic. Biol. Med. 100:S125. doi: 10.1016/J.FREERADBIOMED.2016.10.325
Labelle, M., Begum, S., and Hynes, R. O. (2011). Direct signaling between platelets and cancer cells induces an epithelial-mesenchymal-like transition and promotes metastasis. Cancer Cell 20, 576–590. doi: 10.1016/j.ccr.2011.09.009
Lagadinou, E. D., Sach, A., Callahan, K., Rossi, R. M., Neering, S. J., and Minhajuddin, M. (2013). BCL-2 inhibition targets oxidative phosphorylation and selectively eradicates quiescent human leukemia stem cells. Cell Stem Cell 12, 329–341. doi: 10.1016/j.stem.2012.12.013
Lai, K. K. Y., Kweon, S. M., Chi, F., Hwang, E., Kabe, Y., and Higashiyama, R. (2017). Stearoyl-CoA desaturase promotes liver fibrosis and tumor development in mice via a Wnt positive-signaling loop by stabilization of low-density lipoprotein-receptor-related proteins 5 and 6. Gastroenterology 152, 1477–1491. doi: 10.1053/J.GASTRO.2017.01.021
Lamb, R., Bonuccelli, G., Ozsvári, B., Peiris-Pagès, M., Fiorillo, M., Smith, D. L., et al. (2015a). Mitochondrial mass, a new metabolic biomarker for stem-like cancer cells: understanding WNT/FGF-driven anabolic signaling. Oncotarget 6, 30453–30471. doi: 10.18632/oncotarget.5852
Lamb, R., Fiorillo, M., Fiorillo, M., Chadwick, A., Ozsvari, B., Reeves, K. J., et al. (2015b). Doxycycline down-regulates DNA-PK and radiosensitizes tumor initiating cells: implications for more effective radiation therapy. Oncotarget 6, 14005–14025. doi: 10.18632/oncotarget.4159
Lamb, R., Ozsvari, B., Ozsvari, B., Lisanti, C. L., Tanowitz, H. B., Howell, A., et al. (2015c). Antibiotics that target mitochondria effectively eradicate cancer stem cells, across multiple tumor types: treating cancer like an infectious disease. Oncotarget 6, 4569–4584. doi: 10.18632/oncotarget.3174
Lane, A. N., and Fan, T. W. M. (2015). Regulation of mammalian nucleotide metabolism and biosynthesis. Nucleic Acids Res. 43, 2466–2485. doi: 10.1093/nar/gkv047
Le Belle, J. E., Orozco, N. M., Paucar, A. A., Saxe, J. P., Mottahedeh, J., Pyle, A. D., et al. (2011). Proliferative neural stem cells have high endogenous ROS levels that regulate self-renewal and neurogenesis in a PI3K/Akt-dependant manner. Cell Stem Cell 8, 59–71. doi: 10.1016/j.stem.2010.11.028
Lee, E. A., Angka, L., Rota, S.-G., Hanlon, T., Hurren, R., Wang, X. M., et al. (2014). Inhibition of fatty acid oxidation with Avocatin B selectively targets AML cells and leukemia stem cells. Blood 124:268.
Lee, E. A., Angka, L., Rota, S. G., Hanlon, T., Mitchell, A., Hurren, R., et al. (2015). Targeting mitochondria with avocatin B induces selective leukemia cell death. Cancer Res. 75, 2478–2488. doi: 10.1158/0008-5472.CAN-14-2676
Levy, S., and Forman, H. J. (2010). C-Myc is a Nrf2-interacting protein that negatively regulates phase II genes through their electrophile responsive elements. IUBMB Life 62, 237–246. doi: 10.1002/iub.314
Lewis, C. A., Brault, C., Peck, B., Bensaad, K., Griffiths, B., Mitter, R., et al. (2015). SREBP maintains lipid biosynthesis and viability of cancer cells under lipid- and oxygen-deprived conditions and defines a gene signature associated with poor survival in glioblastoma multiforme. Oncogene 34, 5128–5140. doi: 10.1038/onc.2014.439
Li, C., Liu, V. W., Chan, D. W., Yao, K. M., and Ngan, H. Y. (2012). LY294002 and metformin cooperatively enhance the inhibition of growth and the induction of apoptosis of ovarian cancer cells. Int. J. Gynecol. Cancer 22, 15–22. doi: 10.1097/IGC.0b013e3182322834
Li, D., Fu, Z., Chen, R., Zhao, X., Zhou, Y., Zeng, B., et al. (2015). Inhibition of glutamine metabolism counteracts pancreatic cancer stem cell features and sensitizes cells to radiotherapy. Oncotarget 6, 31151–31163. doi: 10.18632/oncotarget.5150
Li, F., He, B., Ma, X., Yu, S., Bhave, R. R., Lentz, S. R., et al. (2017). Prostaglandin E1 and its analog misoprostol inhibit human CML stem cell self-renewal via EP4 receptor activation and repression of AP-1. Cell Stem Cell 21, 359–373.e5. doi: 10.1016/j.stem.2017.08.001
Li, J., Tang, Q., Li, Y., Hu, B.-R., Ming, Z.-Y., Fu, Q., et al. (2017). Lipid desaturation is a metabolic marker and therapeutic target of ovarian cancer stem cells. Cell Stem Cell 20, 303–314. doi: 10.1016/j.stem.2016.11.004
Li, J.-J., Tang, Q., Li, Y., Hu, B.-R., Ming, Z.-Y., Fu, Q., et al. (2006). Role of oxidative stress in the apoptosis of hepatocellular carcinoma induced by combination of arsenic trioxide and ascorbic acid. Acta Pharmacol. Sin. 27, 1078–1084. doi: 10.1111/j.1745-7254.2006.00345.x
Li, Q., Qi, F., Meng, X., Zhu, C., and Gao, Y. (2018). Mst1 regulates colorectal cancer stress response via inhibiting Bnip3-related mitophagy by activation of JNK/p53 pathway. Cell Biol. Toxicol. 34, 263–277. doi: 10.1007/s10565-017-9417-6
Li, Y., Jiang, F., Liu, Q., Shen, J., Wang, X., Li, Z., et al. (2015a). Inhibition of the cancer stem cells-like properties by arsenic trioxide, involved in the attenuation of endogenous transforming growth factor beta signal. Toxicol. Sci. 143, 156–164. doi: 10.1093/toxsci/kfu218
Li, Y., Rogoff, H. A., Keates, S., Gao, Y., Murikipudi, S., Mikule, K., et al. (2015b). Suppression of cancer relapse and metastasis by inhibiting cancer stemness. Proc. Natl. Acad. Sci. U.S.A. 112, 1839–1844. doi: 10.1073/pnas.1424171112
Liang, Y.-J., Ding, Y., Levery, S. B., Lobaton, M., Handa, K., and Hakomori, S.-I. (2013). Differential expression profiles of glycosphingolipids in human breast cancer stem cells vs. cancer non-stem cells. Proc. Natl. Acad. Sci. U.S.A. 110, 4968–4973. doi: 10.1073/pnas.1302825110
Liao, J., Liu, P.-P., Hou, G., Shao, J., Yang, J., Liu, K., et al. (2017). Regulation of stem-like cancer cells by glutamine through beta-catenin pathway mediated by redox signaling. Mol. Cancer 16:51. doi: 10.1186/s12943-017-0623-x
Liao, J., Qian, F., Tchabo, N., Mhawech-Fauceglia, P., Beck, A., Qian, Z., et al. (2014). Ovarian cancer spheroid cells with stem cell-like properties contribute to tumor generation, metastasis and chemotherapy resistance through hypoxia-resistant metabolism. PLoS One 9:e84941. doi: 10.1371/journal.pone.0084941
Liberti, M. V., and Locasale, J. W. (2016). The warburg effect: how does it benefit cancer cells? Trends Biochem. Sci. 41, 211–218. doi: 10.1016/j.tibs.2015.12.001
Lin, W.-C., Rajbhandari, N., Liu, C., Sakamoto, K., Zhang, Q., Triplett, A. A., et al. (2013). Dormant cancer cells contribute to residual disease in a model of reversible pancreatic cancer. Cancer Res. 73, 1821–1830. doi: 10.1158/0008-5472.CAN-12-2067
Liou, G. Y., Döppler, H., DelGiorno, K. E., Zhang, L., Leitges, M., Crawford, H. C., et al. (2016). Mutant KRas-induced mitochondrial oxidative stress in acinar cells upregulates EGFR signaling to drive formation of pancreatic precancerous lesions. Cell Rep. 14, 2325–2336. doi: 10.1016/j.celrep.2016.02.029
Liou, G.-Y., and Storz, P. (2010). Reactive oxygen species in cancer. Free Radic. Res. 44, 479–479. doi: 10.3109/10715761003667554
Liu, P., Kumar, I. S., Brown, S., Kannappan, V., Tawari, P. E., Tang, J. Z., et al. (2013). Disulfiram targets cancer stem-like cells and reverses resistance and cross-resistance in acquired paclitaxel-resistant triple-negative breast cancer cells. Br. J. Cancer 109, 1876–1885. doi: 10.1038/bjc.2013.534
Liu, P.-P., Liao, J., Tang, Z.-J., Wu, W.-J., Yang, J., Zeng, Z.-L., et al. (2014). Metabolic regulation of cancer cell side population by glucose through activation of the Akt pathway. Cell Death Differ. 21, 124–135. doi: 10.1038/cdd.2013.131
Liu, X., Wang, L., Cui, W., Yuan, X., Lin, L., Cao, Q., et al. (2016). Targeting ALDH1A1 by disulfiram/copper complex inhibits non-small cell lung cancer recurrence driven by ALDH-positive cancer stem cells. Oncotarget 7, 58516–58530. doi: 10.18632/oncotarget.11305
Liu, Y., Zuckier, L. S., and Ghesani, N. V. (2010). Dominant uptake of fatty acid over glucose by prostate cells: a potential new diagnostic and therapeutic approach. Anticancer Res. 30, 369–374.
Luo, C., Lim, J.-H., Lee, Y., Granter, S. R., Thomas, A., Vazquez, F., et al. (2016). A PGC1α-mediated transcriptional axis suppresses melanoma metastasis. Nature 537, 422–426. doi: 10.1038/nature19347
Luo, M., Shang, L., Brooks, M. D., Jiagge, E., Zhu, Y., Buschhaus, J. M., et al. (2018). Targeting breast cancer stem cell state equilibrium through modulation of redox signaling. Cell Metab. 28, 69–86.e6. doi: 10.1016/j.cmet.2018.06.006
Lv, H., Wang, C., Fang, T., Li, T., Lv, G., Han, Q., et al. (2018). Vitamin C preferentially kills cancer stem cells in hepatocellular carcinoma via SVCT-2. Precis. Oncol. 2:1. doi: 10.1038/s41698-017-0044-8
Ma, M. K. F., Lau, E. Y. T., Leung, D. H. W., Lo, J., Ho, N. P. Y., Cheng, L. K. W., et al. (2017). Stearoyl-CoA desaturase regulates sorafenib resistance via modulation of ER stress-induced differentiation. J. Hepatol. 67, 979–990. doi: 10.1016/j.jhep.2017.06.015
Maan, M., Peters, J. M., Dutta, M., and Patterson, A. D. (2018). Lipid metabolism and lipophagy in cancer. Biochem. Biophys. Res. Commun. 504, 582–589. doi: 10.1016/j.bbrc.2018.02.097
Malanchi, I., and Huelsken, J. (2009). Cancer stem cells: never Wnt away from the niche. Curr. Opin. Oncol. 21, 41–46. doi: 10.1097/CCO.0b013e32831d1faf
Malchenko, S., Sredni, S. T., Boyineni, J., Bi, Y., Margaryan, N. V., Guda, M. R., et al. (2018). Characterization of brain tumor initiating cells isolated from an animal model of CNS primitive neuroectodermal tumors. Oncotarget 9, 13733–13747. doi: 10.18632/oncotarget.24460
Marengo, B., De Ciucis, C., Verzola, D., Pistoia, V., Raffaghello, L., Patriarca, S., et al. (2008). Mechanisms of BSO (L-buthionine-S,R-sulfoximine)-induced cytotoxic effects in neuroblastoma. Free Radic. Biol. Med. 44, 474–482. doi: 10.1016/j.freeradbiomed.2007.10.031
Marrache, S., Pathak, R. K., and Dhar, S. (2014). Detouring of cisplatin to access mitochondrial genome for overcoming resistance. Proc. Natl. Acad. Sci. U.S.A. 111, 10444–10449. doi: 10.1073/pnas.1405244111
Martinez-Outschoorn, U. E., and Lisanti, M. P. (2014). Tumor microenvironment: introduction. Semin. Oncol. 41:145. doi: 10.1053/j.seminoncol.2014.03.007
Maschek, G., Savaraj, N., Priebe, W., Braunschweiger, P., Hailton, K., Tidmarsh, G. F., et al. (2004). 2-deoxy-D-glucose increases the efficacy of adriamycin and paclitaxel in human osteosarcoma and non-small cell lung cancers in vivo. Cancer Res. 64, 31–34. doi: 10.1158/0008-5472.CAN-03-3294
Massihnia, D., Avan, A., Funel, N., Maftouh, M., Van Krieken, A., Granchi, C., et al. (2017). Phospho-Akt overexpression is prognostic and can be used to tailor the synergistic interaction of Akt inhibitors with gemcitabine in pancreatic cancer. J. Hematol. Oncol. 10:9. doi: 10.1186/s13045-016-0371-1
Mateo, F., Meca-Cortés,Ó., Celià-Terrassa, T., Fernández, Y., Abasolo, I., Sánchez-Cid, L., et al. (2014). SPARC mediates metastatic cooperation between CSC and non-CSC prostate cancer cell subpopulations. Mol. Cancer 13:237. doi: 10.1186/1476-4598-13-237
Matulis, S. M., Morales, A. A., Yehiayan, L., Lee, K. P., Cai, Y., and Boise, L. H. (2012). Alterations in glutathione levels and apoptotic regulators are associated with acquisition of arsenic trioxide resistance in multiple myeloma. PLoS One 7:e52662. doi: 10.1371/journal.pone.0052662
Mazur, P. K., Herner, A., Mello, S. S., Wirth, M., Hausmann, S., Sánchez-Rivera, F. J., et al. (2015). Combined inhibition of BET family proteins and histone deacetylases as a potential epigenetics-based therapy for pancreatic ductal adenocarcinoma. Nat. Med. 21, 1163–1171. doi: 10.1038/nm.3952
Menard, J. A., Christianson, H. C., Kucharzewska, P., Bourseau-Guilmain, E., Svensson, K. J., Lindqvist, E., et al. (2016). Metastasis stimulation by hypoxia and acidosis-induced extracellular lipid uptake is mediated by proteoglycan-dependent endocytosis. Cancer Res. 76, 4828–4840. doi: 10.1158/0008-5472.CAN-15-2831
Milkovic, L., Zarkovic, N., and Saso, L. (2017). Controversy about pharmacological modulation of Nrf2 for cancer therapy. Redox Biol. 12, 727–732. doi: 10.1016/j.redox.2017.04.013
Miller, D. M., Thomas, S. D., Islam, A., Muench, D., and Sedoris, K. (2012). c-Myc and cancer metabolism. Clin. Cancer Res. 18, 5546–5553. doi: 10.1158/1078-0432.CCR-12-0977
Miller, W. H. (2002). Molecular targets of arsenic trioxide in malignant cells. Oncologist 7(Suppl. 1), 14–19. doi: 10.1634/theoncologist.7-suppl_1-14
Mitsuishi, Y., Taguchi, K., Kawatani, Y., Shibata, T., Nukiwa, T., Aburatani, H., et al. (2012). Nrf2 redirects glucose and glutamine into anabolic pathways in metabolic reprogramming. Cancer Cell 22, 66–79. doi: 10.1016/j.ccr.2012.05.016
Miyamoto, K., Araki, K. Y., Naka, K., Arai, F., Takubo, K., Yamazaki, S., et al. (2007). Foxo3a is essential for maintenance of the hematopoietic stem cell pool. Cell Stem Cell 1, 101–112. doi: 10.1016/j.stem.2007.02.001
Moreb, J. S., Gabr, A., Vartikar, G. R., Gowda, S., Zucali, J. R., Mohuczy, D., et al. (2004). Retinoic acid down-regulates aldehyde dehydrogenase and increases cytotoxicity of 4-hydroperoxycyclophosphamide and acetaldehyde. J. Pharmacol. Exp. Ther. 312, 339–345. doi: 10.1124/jpet.104.072496
Mullen, A. R., Wheaton, W. W., Jin, E. S., Chen, P. H., Sullivan, L. B., Cheng, T., et al. (2011). Reductive carboxylation supports growth in tumour cells with defective mitochondria. Nature 481, 385–388. doi: 10.1038/nature10642
Murphy, M. P. (2008). Targeting lipophilic cations to mitochondria. Biochim. Biophys. Acta 1777, 1028–1031. doi: 10.1016/j.bbabio.2008.03.029
Naka, K., Muraguchi, T., Hoshii, T., and Hirao, A. (2008). Regulation of reactive oxygen species and genomic stability in hematopoietic stem cells. Antioxid. Redox Signal. 10, 1883–1894. doi: 10.1089/ars.2008.2114
Naujokat, C., and Steinhart, R. (2012). Salinomycin as a drug for targeting human cancer stem cells. J. Biomed. Biotechnol. 2012:950658. doi: 10.1155/2012/950658
Nieman, K. M., Kenny, H. A., Penicka, C. V., Ladanyi, A., Buell-Gutbrod, R., Zillhardt, M. R., et al. (2011). Adipocytes promote ovarian cancer metastasis and provide energy for rapid tumor growth. Nat. Med. 17, 1498–1503. doi: 10.1038/nm.2492
Noto, A., De Vitis, C., Pisanu, M. E., Roscilli, G., Ricci, G., Catizone, A., et al. (2017). Stearoyl-CoA-desaturase 1 regulates lung cancer stemness via stabilization and nuclear localization of YAP/TAZ. Oncogene 36, 4573–4584. doi: 10.1038/onc.2017.75
Noto, A., Raffa, S., De Vitis, C., Roscilli, G., Malpicci, D., Coluccia, P., et al. (2013). Stearoyl-CoA desaturase-1 is a key factor for lung cancer-initiating cells. Cell Death Dis. 4:e947. doi: 10.1038/cddis.2013.444
Obrador, E., Carretero, J., Ortega, A., Medina, I., Rodilla, V., Pellicer, J. A., et al. (2002). γ-Glutamyl transpeptidase overexpression increases metastatic growth of B16 melanoma cells in the mouse liver. Hepatology 35, 74–81. doi: 10.1053/jhep.2002.30277
Okami, J., Simeone, D. M., and Logsdon, C. D. (2004). Silencing of the hypoxia-inducible cell death protein BNIP3 in pancreatic cancer. Cancer Res. 64, 5338–5346. doi: 10.1158/0008-5472.CAN-04-0089
Ookhtens, M., Kannan, R., Lyon, I., and Baker, N. (1984). Liver and adipose tissue contributions to newly formed fatty acids in an ascites tumor. Am. J. Physiol. Regul. Integr. Comp. Physiol. 247, R146–R153. doi: 10.1152/ajpregu.1984.247.1.R146
Owusu-Ansah, E., and Banerjee, U. (2009). Reactive oxygen species prime Drosophila haematopoietic progenitors for differentiation. Nature 461, 537–541. doi: 10.1038/nature08313
Ozsvari, B., Sotgia, F., Simmons, K., Trowbridge, R., Foster, R., and Lisanti, M. P. (2017). Mitoketoscins: novel mitochondrial inhibitors for targeting ketone metabolism in cancer stem cells (CSCs). Oncotarget 8, 78340–78350. doi: 10.18632/oncotarget.21259
Palorini, R., Votta, G., Balestrieri, C., Monestiroli, A., Olivieri, S., and Vento, R. (2014). Energy metabolism characterization of a novel cancer stem cell-like line 3AB-OS. J. Cell. Biochem. 115, 368–379. doi: 10.1002/jcb.24671
Pandey, P. R., Xing, F., Sharma, S., Watabe, M., Pai, S. K., Iiizumi-Gairani, M., et al. (2013). Elevated lipogenesis in epithelial stem-like cell confers survival advantage in ductal carcinoma in situ of breast cancer. Oncogene 32, 5111–5122. doi: 10.1038/onc.2012.519
Park, J., Shim, J. K., Kang, J. H., Choi, J., Chang, J. H., Kim, S. Y., et al. (2018). Regulation of bioenergetics through dual inhibition of aldehyde dehydrogenase and mitochondrial complex I suppresses glioblastoma tumorspheres. Neuro Oncol. 20, 954–965. doi: 10.1093/neuonc/nox243
Pascual, G., Avgustinova, A., Mejetta, S., Martín, M., Castellanos, A., Attolini, C. S.-O., et al. (2016). Targeting metastasis-initiating cells through the fatty acid receptor CD36. Nature 541, 41–45. doi: 10.1038/nature20791
Pasto, A., Bellio, C., Pilotto, G., Ciminale, V., Silic-Benussi, M., Guzzo, G., et al. (2014). Cancer stem cells from epithelial ovarian cancer patients privilege oxidative phosphorylation, and resist glucose deprivation. Oncotarget 5, 4305–4319. doi: 10.18632/oncotarget.2010
Paul, M. K., Bisht, B., Darmawan, D. O., Chiou, R., Ha, V. L., Wallace, W. D., et al. (2014). Dynamic changes in intracellular ROS levels regulate airway basal stem cell homeostasis through Nrf2-dependent notch signaling. Cell Stem Cell 15, 199–214. doi: 10.1016/j.stem.2014.05.009
Peiris-Pagès, M., Bonuccelli, G., Sotgia, F., and Lisanti, M. P. (2018). Mitochondrial fission as a driver of stemness in tumor cells: mDIVI1 inhibits mitochondrial function, cell migration and cancer stem cell (CSC) signalling. Oncotarget 9, 13254–13275. doi: 10.18632/oncotarget.24285
Peng, Y., He, G., Tang, D., Xiong, L., Wen, Y., Miao, X., et al. (2017). Lovastatin inhibits cancer stem cells and sensitizes to chemo- and photodynamic therapy in nasopharyngeal carcinoma. J. Cancer 8, 1655–1664. doi: 10.7150/jca.19100
Petrachi, T., Romagnani, A., Albini, A., Longo, C., Argenziano, G., Grisendi, G., et al. (2017). Therapeutic potential of the metabolic modulator phenformin in targeting the stem cell compartment in melanoma. Oncotarget 8, 6914–6928. doi: 10.18632/oncotarget.14321
Phillips, T. M., McBride, W. H., and Pajonk, F. (2006). The response of CD24-/low/CD44+ breast cancer-initiating cells to radiation. J. Natl. Cancer Inst. 98, 1777–1785. doi: 10.1093/jnci/djj495
Pisanu, M. E., Noto, A., De Vitis, C., Morrone, S., Scognamiglio, G., Botti, G., et al. (2017). Blockade of Stearoyl-CoA-desaturase 1 activity reverts resistance to cisplatin in lung cancer stem cells. Cancer Lett. 406, 93–104. doi: 10.1016/J.CANLET.2017.07.027
Piskounova, E., Agathocleous, M., Murphy, M. M., Hu, Z., Huddlestun, S. E., Zhao, Z., et al. (2015). Oxidative stress inhibits distant metastasis by human melanoma cells. Nature 527, 186–191. doi: 10.1038/nature15726
Porporato, P. E., Dhup, S., Dadhich, R. K., Copetti, T., and Sonveaux, P. (2014). A mitochondrial switch promotes tumor metastasis. Cell Rep. 8, 754–766. doi: 10.1016/j.celrep.2014.06.043
Porporato, P. E., Payen, V. L., Pérez-Escuredo, J., De Saedeleer, C. J., Danhier, P., Copetti, T., et al. (2011). Anticancer targets in the glycolytic metabolism of tumors: a comprehensive review. Front. Pharmacol. 2:49. doi: 10.3389/fphar.2011.00049
Probst, B. L., McCauley, L., Trevino, I., Wigley, W. C., and Ferguson, D. A. (2015a). Cancer cell growth is differentially affected by constitutive activation of NRF2 by KEAP1 deletion and pharmacological activation of NRF2 by the synthetic triterpenoid, RTA 405. PLoS One 10:e0135257. doi: 10.1371/journal.pone.0135257
Probst, B. L., Trevino, I., McCauley, L., Bumeister, R., Dulubova, I., and Wigley, W. C. (2015b). RTA 408, a novel synthetic triterpenoid with broad anticancer and anti-inflammatory activity. PLoS One 10:e0122942. doi: 10.1371/journal.pone.0122942
Qiang, L., Wu, L., Zhang, H. W., Lu, N., Hu, R., Wang, Y. J., et al. (2012). HIF-1α is critical for hypoxia-mediated maintenance of glioblastoma stem cells by activating Notch signaling pathway. Cell Death Differ. 19, 284–294. doi: 10.1038/cdd.2011.95
Radhakrishnan, R., Ha, J. H., Jayaraman, M., Liu, J., Moxley, K. M., Isidoro, C., et al. (2019). Ovarian cancer cell-derived lysophosphatidic acid induces glycolytic shift and cancer-associated fibroblast-phenotype in normal and peritumoral fibroblasts. Cancer Lett. 442, 464–474. doi: 10.1016/j.canlet.2018.11.023
Raj, D., Aicher, A., and Heeschen, C. (2015). Concise review: stem cells in pancreatic cancer: from concept to translation. Stem Cells 33, 2893–2902. doi: 10.1002/stem.2114
Rattigan, Y. I., Patel, B. B., Ackerstaff, E., Sukenick, G., Koutcher, J. A., and Glod, J. W. (2012). Lactate is a mediator of metabolic cooperation between stromal carcinoma associated fibroblasts and glycolytic tumor cells in the tumor microenvironment. Exp. Cell Res. 318, 326–335. doi: 10.1016/j.yexcr.2011.11.014
Rausch, V., Liu, L., Kallifatidis, G., Baumann, B., Mattern, J., Gladkich, J., et al. (2010). Synergistic activity of sorafenib and sulforaphane abolishes pancreatic cancer stem cell characteristics. Cancer Res. 70, 5004–5013. doi: 10.1158/0008-5472.CAN-10-0066
Ray, R., and Rai, V. (2017). Lysophosphatidic acid converts monocytes into macrophages in both mice and humans. Blood 129, 1177–1183. doi: 10.1182/blood-2016-10-743757
Raza, M. H., Siraj, S., Arshad, A., Waheed, U., Aldakheel, F., Alduraywish, S., et al. (2017). ROS-modulated therapeutic approaches in cancer treatment. J. Cancer Res. Clin. Oncol. 143, 1789–1809. doi: 10.1007/s00432-017-2464-9
Rehman, J., Zhang, H. J., Toth, P. T., Zhang, Y., Marsboom, G., Hong, Z., et al. (2012). Inhibition of mitochondrial fission prevents cell cycle progression in lung cancer. FASEB J. 26, 2175–2186. doi: 10.1096/fj.11-196543
Reni, M., Dugnani, E., Cereda, S., Belli, C., Balzano, G., Nicoletti, R., et al. (2016). (Ir)relevance of metformin treatment in patients with metastatic pancreatic cancer: an open-label, randomized phase II trial. Clin. Cancer Res. 22, 1076–1085. doi: 10.1158/1078-0432.CCR-15-1722
Reya, T., Morrison, S. J., Clarke, M. F., and Weissman, I. L. (2001). Stem cells, cancer, and cancer stem cells. Nature 414, 105–111. doi: 10.1038/35102167
Rhim, A. D., Mirek, E. T., Aiello, N. M., Maitra, A., Bailey, J. M., McAllister, F., et al. (2012). EMT and dissemination precede pancreatic tumor formation. Cell 148, 349–361. doi: 10.1016/j.cell.2011.11.025
Ricciardi, M. R., Mirabilii, S., Allegretti, M., Licchetta, R., Calarco, A., Torrisi, M. R., et al. (2015). Targeting the leukemia cell metabolism by the CPT1a inhibition: functional preclinical effects in leukemias. Blood 126, 1925–1929. doi: 10.1182/blood-2014-12-617498
Riemann, A., Schneider, B., Ihling, A., Nowak, M., Sauvant, C., Thews, O., et al. (2011). Acidic environment leads to ROS-Induced MAPK signaling in cancer cells. PLoS One 6:e22445. doi: 10.1371/journal.pone.0022445
Robke, L., Futamura, Y., Konstantinidis, G., Wilke, J., Aono, H., Mahmoud, Z., et al. (2018). Discovery of the novel autophagy inhibitor aumitin that targets mitochondrial complex I. Chem. Sci. 9, 3014–3022. doi: 10.1039/c7sc05040b
Rocha, C. R. R., Kajitani, G. S., Quinet, A., Fortunato, R. S., and Menck, C. F. M. (2016). NRF2 and glutathione are key resistance mediators to temozolomide in glioma and melanoma cells. Oncotarget 7, 48081–48092. doi: 10.18632/oncotarget.10129
Rodman, S. N., Spence, J. M., Ronnfeldt, T. J., Zhu, Y., Solst, S. R., ONeill, R. A., et al. (2016). Enhancement of radiation response in breast cancer stem cells by inhibition of thioredoxin- and glutathione-dependent metabolism. Radiat. Res. 186, 385–395. doi: 10.1667/RR14463.1
Roh, J. L., Kim, E. H., Jang, H., and Shin, D. (2017). Nrf2 inhibition reverses the resistance of cisplatin-resistant head and neck cancer cells to artesunate-induced ferroptosis. Redox Biol. 11, 254–262. doi: 10.1016/j.redox.2016.12.010
Rosi, A., Ricci-Vitiani, L., Biffoni, M., Grande, S., Luciani, A. M., Palma, A., et al. (2015). 1H NMR spectroscopy of glioblastoma stem-like cells identifies alpha-aminoadipate as a marker of tumor aggressiveness. NMR Biomed. 28, 317–326. doi: 10.1002/nbm.3254
Ryoo, I. G., Lee, S. H., and Kwak, M. K. (2016). Redox modulating NRF2: a potential mediator of cancer stem cell resistance. Oxid. Med. Cell. Longev. 2016:2428153. doi: 10.1155/2016/2428153
Rysman, E., Brusselmans, K., Scheys, K., Timmermans, L., Derua, R., Munck, S., et al. (2010). De novo lipogenesis protects cancer cells from free radicals and chemotherapeutics by promoting membrane lipid saturation. Cancer Res. 70, 8117–8126. doi: 10.1158/0008-5472.CAN-09-3871
Ryu, I., Ryu, M. J., Han, J., Kim, S. J., Lee, M. J., Ju, X., et al. (2018). L-Deprenyl exerts cytotoxicity towards acute myeloid leukemia through inhibition of mitochondrial respiration. Oncol. Rep. 40, 3869–3878. doi: 10.3892/or.2018.6753
Samanta, D., Park, Y., Andrabi, S. A., Shelton, L. M., Gilkes, D. M., and Semenza, G. L. (2016). PHGDH expression is required for mitochondrial redox homeostasis, breast cancer stem cell maintenance, and lung metastasis. Cancer Res. 76, 4430–4442. doi: 10.1158/0008-5472.CAN-16-0530
Samudio, I., Harmancey, R., Fiegl, M., Kantarjian, H., Konopleva, M., Korchin, B., et al. (2010). Pharmacologic inhibition of fatty acid oxidation sensitizes human leukemia cells to apoptosis induction. J. Clin. Investig. 120, 142–156. doi: 10.1172/JCI38942
Sancho, P., Barneda, D., and Heeschen, C. (2016). Hallmarks of cancer stem cell metabolism. Br. J. Cancer 114, 1305–1312. doi: 10.1038/bjc.2016.152
Sancho, P., Burgos-Ramos, E., Tavera, A., Kheir, T. B., Jagust, P., Schoenhals, M., et al. (2015). MYC/PGC-1α balance determines the metabolic phenotype and plasticity of pancreatic cancer stem cells. Cell Metab. 22, 590–605. doi: 10.1016/j.cmet.2015.08.015
Sansone, P., Ceccarelli, C., Berishaj, M., Chang, Q., Rajasekhar, V. K., Perna, F., et al. (2016). Self-renewal of CD133hi cells by IL6/Notch3 signalling regulates endocrine resistance in metastatic breast cancer. Nat. Commun. 7:10442. doi: 10.1038/ncomms10442
Sansone, P., Savini, C., Kurelac, I., Chang, Q., Amato, L. B., Strillacci, A., et al. (2017). Packaging and transfer of mitochondrial DNA via exosomes regulate escape from dormancy in hormonal therapy-resistant breast cancer. Proc. Natl. Acad. Sci. U.S.A. 114, E9066–E9075. doi: 10.1073/pnas.1704862114
Santi, A., Caselli, A., Ranaldi, F., Paoli, P., Mugnaioni, C., Michelucci, E., et al. (2015). Cancer associated fibroblasts transfer lipids and proteins to cancer cells through cargo vesicles supporting tumor growth. Biochim. Biophys. Acta 1853, 3211–3223. doi: 10.1016/J.BBAMCR.2015.09.013
Sato, M., Kawana, K., Adachi, K., Fujimoto, A., Yoshida, M., Nakamura, H., et al. (2016). Spheroid cancer stem cells display reprogrammed metabolism and obtain energy by actively running the tricarboxylic acid (TCA) cycle. Oncotarget 7, 33297–33305. doi: 10.18632/oncotarget.8947
Schafer, Z. T., Grassian, A. R., Song, L., Jiang, Z., Gerhart-Hines, Z., Irie, H. Y., et al. (2009). Antioxidant and oncogene rescue of metabolic defects caused by loss of matrix attachment. Nature 461, 109–113. doi: 10.1038/nature08268
Sciacovelli, M., Guzzo, G., Morello, V., Frezza, C., Zheng, L., Nannini, N., et al. (2013). The mitochondrial chaperone TRAP1 promotes neoplastic growth by inhibiting succinate dehydrogenase. Cell Metab. 17, 988–999. doi: 10.1016/j.cmet.2013.04.019
Seo, E. J., Kwon, Y. W., Jang, I. H., Kim, D. K., Lee, S. I., Choi, E. J., et al. (2016). Autotaxin regulates maintenance of ovarian cancer stem cells through lysophosphatidic acid-mediated autocrine mechanism. Stem Cells 34, 551–564. doi: 10.1002/stem.2279
Shafat, M. S., Oellerich, T., Mohr, S., Robinson, S. D., Edwards, D. R., Marlein, C. R., et al. (2017). Leukemic blasts program bone marrow adipocytes to generate a protumoral microenvironment. Blood 129, 1320–1332. doi: 10.1182/blood-2016-08-734798
Sharif, T., Martell, E., Dai, C., Ghassemi-Rad, M. S., Lee, K., Singh, S. K., et al. (2018). Phosphoglycerate dehydrogenase inhibition induces p-mTOR-independent autophagy and promotes multilineage differentiation in embryonal carcinoma stem-like cells. Cell Death Dis. 9:990. doi: 10.1038/s41419-018-0997-8
Shelton, L. M., Huysentruyt, L. C., and Seyfried, T. N. (2010). Glutamine targeting inhibits systemic metastasis in the VM-M3 murine tumor model. Int. J. Cancer 127, 2478–2485. doi: 10.1002/ijc.25431
Shen, Y. A., Lin, C. H., Chi, W. H., Wang, C. Y., Hsieh, Y. T., Wei, Y. H., et al. (2013). Resveratrol impedes the stemness, epithelial-mesenchymal transition, and metabolic reprogramming of cancer stem cells in nasopharyngeal carcinoma through p53 activation. Evid. Based Complement. Alternat. Med. 2013:590393. doi: 10.1155/2013/590393
Shen, Y. A., Wang, C. Y., Hsieh, Y. T., Chen, Y. J., and Wei, Y. H. (2015). Metabolic reprogramming orchestrates cancer stem cell properties in nasopharyngeal carcinoma. Cell Cycle 14, 86–98. doi: 10.4161/15384101.2014.974419
Shim, H., Chun, Y. S., Lewis, B. C., and Dang, C. V. (1998). A unique glucose-dependent apoptotic pathway induced by c-Myc. Proc. Natl. Acad. Sci. U.S.A. 95, 1511–1516. doi: 10.1073/pnas.95.4.1511
Simsek, T., Kocabas, F., Zheng, Z., Deberardinis, R. J., Mahmoud, A. I., Olson, E. N., et al. (2010). The distinct metabolic profile of hematopoietic stem cells reflects their location in a hypoxic niche. Cell Stem Cell 7, 380–390. doi: 10.1016/j.stem.2010.07.011
Skrtic, M., Sriskanthadevan, S., Jhas, B., Gebbia, M., Wang, X., Wang, Z., et al. (2011). Inhibition of mitochondrial translation as a therapeutic strategy for human acute myeloid leukemia. Cancer Cell 20, 674–688. doi: 10.1016/j.ccr.2011.10.015
Smolková, K., Plecitá-Hlavatá, L., Bellance, N., Benard, G., Rossignol, R., and Ježek, P. (2011). Waves of gene regulation suppress and then restore oxidative phosphorylation in cancer cells. Int. J. Biochem. Cell Biol. 43, 950–968. doi: 10.1016/j.biocel.2010.05.003
Somervaille, T. C. P., Matheny, C. J., Spencer, G. J., Iwasaki, M., Rinn, J. L., Witten, D. M., et al. (2009). Hierarchical maintenance of MLL myeloid leukemia stem cells employs a transcriptional program shared with embryonic rather than adult stem cells. Cell Stem Cell 4, 129–140. doi: 10.1016/j.stem.2008.11.015
Son, J., Lyssiotis, C. A., Ying, H., Wang, X., Hua, S., Ligorio, M., et al. (2013). Glutamine supports pancreatic cancer growth through a KRAS-regulated metabolic pathway. Nature 496, 101–105. doi: 10.1038/nature12040
Son, K., Fujioka, S., Iida, T., Furukawa, K., Fujita, T., Yamada, H., et al. (2009). Doxycycline induces apoptosis in PANC-1 pancreatic cancer cells. Anticancer Res. 29, 3995–4003.
Song, K., Kwon, H., Han, C., Zhang, J., Dash, S., Lim, K., et al. (2015). Active glycolytic metabolism in CD133(+) hepatocellular cancer stem cells: regulation by MIR-122. Oncotarget 6, 40822–40835. doi: 10.18632/oncotarget.5812
Song, W., Tang, Z., Lei, T., Wen, X., Wang, G., Zhang, D., et al. (2016). Stable loading and delivery of disulfiram with mPEG-PLGA/PCL mixed nanoparticles for tumor therapy. Nanomedicine 12, 377–386. doi: 10.1016/j.nano.2015.10.022
Sonveaux, P., Végran, F., Schroeder, T., Wergin, M. C., Verrax, J., Rabbani, Z. N., et al. (2008). Targeting lactate-fueled respiration selectively kills hypoxic tumor cells in mice. J. Clin. Investig. 118, 3930–3942. doi: 10.1172/JCI36843
Soriano, F. X., Baxter, P., Murray, L. M., Sporn, M. B., Gillingwater, T. H., and Hardingham, G. E. (2009). Transcriptional regulation of the AP-1 and Nrf2 target gene sulfiredoxin. Mol. Cells 27, 279–282. doi: 10.1007/s10059-009-0050-y
Sounni, N. E., Cimino, J., Blacher, S., Primac, I., Truong, A., Mazzucchelli, G., et al. (2014). Blocking lipid synthesis overcomes tumor regrowth and metastasis after antiangiogenic therapy withdrawal. Cell Metab. 20, 280–294. doi: 10.1016/j.cmet.2014.05.022
Sousa, C. M., Biancur, D. E., Wang, X., Halbrook, C. J., Sherman, M. H., Zhang, L., et al. (2016). Pancreatic stellate cells support tumour metabolism through autophagic alanine secretion. Nature 536, 479–483. doi: 10.1038/nature19084
Staubach, S., and Hanisch, F.-G. (2011). Lipid rafts: signaling and sorting platforms of cells and their roles in cancer. Expert Rev. Proteomics 8, 263–277. doi: 10.1586/epr.11.2
Sun, P., Xia, S., Lal, B., Shi, X., Yang, K. S., Watkins, P. A., et al. (2014). Lipid metabolism enzyme ACSVL3 supports glioblastoma stem cell maintenance and tumorigenicity. BMC Cancer 14:401. doi: 10.1186/1471-2407-14-401
Suzuki, S., Tanaka, T., Poyurovsky, M. V., Nagano, H., Mayama, T., and Ohkubo, S. (2010). Phosphate-activated glutaminase (GLS2), a p53-inducible regulator of glutamine metabolism and reactive oxygen species. Proc. Natl. Acad. Sci. U.S.A. 107, 7461–7466. doi: 10.1073/pnas.1002459107
Swietach, P., Vaughan-Jones, R. D., and Harris, A. L. (2007). Regulation of tumor pH and the role of carbonic anhydrase 9. Cancer Metastasis Rev. 26, 299–310. doi: 10.1007/s10555-007-9064-0
Tachibana, K., Yamasaki, D., Ishimoto, K., and Doi, T. (2008). The role of PPARs in cancer. PPAR Res. 2008:102737. doi: 10.1155/2008/102737
Takubo, K., Goda, N., Yamada, W., Iriuchishima, H., Ikeda, E., Kubota, Y., et al. (2010). Regulation of the HIF-1α level is essential for hematopoietic stem cells. Cell Stem Cell 7, 391–402. doi: 10.1016/j.stem.2010.06.020
Tanaka, G., Inoue, K.-I., Shimizu, T., Akimoto, K., and Kubota, K. (2016). Dual pharmacological inhibition of glutathione and thioredoxin systems synergizes to kill colorectal carcinoma stem cells. Cancer Med. 5, 2544–2557. doi: 10.1002/cam4.844
Tang, D., Kang, R., Livesey, K. M., Kroemer, G., Billiar, T. R., Van Houten, B., et al. (2011). High-mobility group box 1 is essential for mitochondrial quality control. Cell Metab. 13, 701–711. doi: 10.1016/j.cmet.2011.04.008
Thomas, H. E., Zhang, Y., Stefely, J. A., Veiga, S. R., Thomas, G., Kozma, S. C., et al. (2018). Mitochondrial complex I activity is required for maximal autophagy. Cell Rep. 24, 2404–2417.e8. doi: 10.1016/j.celrep.2018.07.101
Tirinato, L., Liberale, C., Di Franco, S., Candeloro, P., Benfante, A., La Rocca, R., et al. (2015). Lipid droplets: a new player in colorectal cancer stem cells unveiled by spectroscopic imaging. Stem Cells 33, 35–44. doi: 10.1002/stem.1837
Todorova, V. K., Harms, S. A., Kaufmann, Y., Luo, S., Luo, K. Q., Babb, K., et al. (2004). Effect of dietary glutamine on tumor glutathione levels and apoptosis-related proteins in DMBA-induced breast cancer of rats. Breast Cancer Res. Treat. 88, 247–256. doi: 10.1007/s10549-004-0783-4
Tomiyama, A., Serizawa, S., Tachibana, K., Sakurada, K., Samejima, H., Kuchino, Y., et al. (2006). Critical role for mitochondrial oxidative phosphorylation in the activation of tumor suppressors Bax and Bak. J. Natl. Cancer Inst. 98, 1462–1473. doi: 10.1093/jnci/djj395
Tothova, Z., Kollipara, R., Huntly, B. J., Lee, B. H., Castrillon, D. H., Cullen, D. E., et al. (2007). FoxOs are critical mediators of hematopoietic stem cell resistance to physiologic oxidative stress. Cell 128, 325–339. doi: 10.1016/j.cell.2007.01.003
Touil, Y., Igoudjil, W., Corvaisier, M., Dessein, A.-F., Vandomme, J., Monte, D., et al. (2014). Colon cancer cells escape 5FU chemotherapy-induced cell death by entering stemness and quiescence associated with the c-Yes/YAP axis. Clin. Cancer Res. 20, 837–846. doi: 10.1158/1078-0432.CCR-13-1854
Townsend, D. M., and Tew, K. D. (2003). The role of glutathione-S-transferase in anti-cancer drug resistance. Oncogene 22, 7369–7375. doi: 10.1038/sj.onc.1206940
Trachootham, D., Alexandre, J., and Huang, P. (2009). Targeting cancer cells by ROS-mediated mechanisms: a radical therapeutic approach? Nat. Rev. Drug Discov. 8, 579–591. doi: 10.1038/nrd2803
Valko, M., Rhodes, C. J., Moncol, J., Izakovic, M., and Mazur, M. (2006). Free radicals, metals and antioxidants in oxidative stress-induced cancer. Chem. Biol. Interact. 160, 1–40. doi: 10.1016/j.cbi.2005.12.009
Vander Heiden, M. G., Cantley, L. C., and Thompson, C. B. (2009). Understanding the warburg effect: the metabolic requirements of cell proliferation. Science 324, 1029–1033. doi: 10.1126/science.1160809
Vasudevan, A., Yu, Y., Banerjee, S., Woods, J., Farhana, L., Rajendra, S. G., et al. (2014). Omega-3 fatty acid is a potential preventive agent for recurrent colon cancer. Cancer Prev. Res. 7, 1138–1148. doi: 10.1158/1940-6207.CAPR-14-0177
Vazquez, A., Kamphorst, J. J., Markert, E. K., Schug, Z. T., Tardito, S., and Gottlieb, E. (2016). Cancer metabolism at a glance. J. Cell Sci. 129, 3367–3373. doi: 10.1242/jcs.181016
Vazquez-Martin, A., Oliveras-Ferraros, C., Cufi, S., Del Barco, S., Martin-Castillo, B., and Menendez, J. A. (2010). Metformin regulates breast cancer stem cell ontogeny by transcriptional regulation of the epithelial-mesenchymal transition (EMT) status. Cell Cycle 9, 3807–3814. doi: 10.4161/cc.9.18.13131
Vega-Naredo, I., Loureiro, R., Mesquita, K. A., Barbosa, I. A., Tavares, L. C., Branco, A. F., et al. (2014). Mitochondrial metabolism directs stemness and differentiation in P19 embryonal carcinoma stem cells. Cell Death Differ. 21, 1560–1574. doi: 10.1038/cdd.2014.66
Viale, A., Pettazzoni, P., Lyssiotis, C. A., Ying, H., Sánchez, N., Marchesini, M., et al. (2014). Oncogene ablation-resistant pancreatic cancer cells depend on mitochondrial function. Nature 514, 628–632. doi: 10.1038/nature13611
Vidali, S., Aminzadeh, S., Lambert, B., Rutherford, T., Sperl, W., Kofler, B., et al. (2015). Mitochondria: the ketogenic diet—A metabolism-based therapy. Int. J. Biochem. Cell Biol. 63, 55–59. doi: 10.1016/j.biocel.2015.01.022
Wang, D., Fu, L., Sun, H., Guo, L., and Dubois, R. N. (2015). Prostaglandin E2 promotes colorectal cancer stem cell expansion and metastasis in mice. Gastroenterology 149, 1884–1895.e4. doi: 10.1053/j.gastro.2015.07.064
Wang, H., Mannava, S., Grachtchouk, V., Zhuang, D., Soengas, M. S., Gudkov, A. V., et al. (2008). c-Myc depletion inhibits proliferation of human tumor cells at various stages of the cell cycle. Oncogene 27, 1905–1915. doi: 10.1038/sj.onc.1210823
Wang, J., Mirzapoiazova, T., Tan, Y. H. C., Pang, K. M., Pozhitkov, A., Wang, Y., et al. (2018). Inhibiting crosstalk between MET signaling and mitochondrial dynamics and morphology: a novel therapeutic approach for lung cancer and mesothelioma. Cancer Biol. Ther. 19, 1–10. doi: 10.1080/15384047.2018.1472193
Wang, T., Fahrmann, J. F., Lee, H., Li, Y.-L., Tripathi, S. C., Yue, S., et al. (2018). JAK/STAT3-regulated fatty acid β-oxidation is critical for breast cancer stem cell self-renewal and chemoresistance. Cell Metab. 27, 136–150.e5. doi: 10.1016/J.CMET.2017.11.001
Wang, J.-B., Erickson, J. W., Fuji, R., Ramachandran, S., Gao, P., Dinavahie, R., et al. (2010). Targeting mitochondrial glutaminase activity inhibits oncogenic transformation. Cancer Cell 18, 207–219. doi: 10.1016/j.ccr.2010.08.009
Wang, M.-C., Jiao, M., Wu, T., Jing, L., Cui, J., Guo, H., et al. (2016). Polycomb complex protein BMI-1 promotes invasion and metastasis of pancreatic cancer stem cells by activating PI3K/AKT signaling, an ex vivo, in vitro, and in vivo study. Oncotarget 7, 9586–9599. doi: 10.18632/oncotarget.7078
Wang, X., Huang, Z., Wu, Q., Prager, B. C., Mack, S. C., Yang, K., et al. (2017a). MYC-regulated mevalonate metabolism maintains brain tumor–initiating cells. Cancer Res. 77, 4947–4960. doi: 10.1158/0008-5472.CAN-17-0114
Wang, L., Li, P., Hu, W., Xia, Y., Hu, C., Liu, L., et al. (2017b). CD44+CD24+ subset of PANC-1 cells exhibits radiation resistance via decreased levels of reactive oxygen species. Oncol. Lett. 14, 1341–1346. doi: 10.3892/ol.2017.6301
Wang, X., Yang, K., Xie, Q., Wu, Q., Mack, S. C., Shi, Y., et al. (2017c). Purine synthesis promotes maintenance of brain tumor initiating cells in glioma. Nat. Neurosci. 20, 661–673. doi: 10.1038/nn.4537
Wang, Y. C., Chao, T. K., Chang, C. C., Yo, Y. T., Yu, M. H., and Lai, H. C. (2013). Drug screening identifies niclosamide as an inhibitor of breast cancer stem-like cells. PLoS One 8:e74538. doi: 10.1371/journal.pone.0074538
Warburg, O. (1956). On the origin of cancer cells. Science 123, 309–314. doi: 10.1126/science.123.3191.309
Warburg, O., Wind, F., and Negelein, E. (1927). The metabolism of tumours in the body. J. Gen. Physiol. 8, 519–530. doi: 10.1085/jgp.8.6.519
Weber, D. D., Aminazdeh-Gohari, S., and Kofler, B. (2018). Ketogenic diet in cancer therapy. Aging 10, 164–165. doi: 10.18632/aging.101382
Wei, R., Cao, J., and Yao, S. (2018). Matrine promotes liver cancer cell apoptosis by inhibiting mitophagy and PINK1/Parkin pathways. Cell Stress Chaperones 23, 1295–1309. doi: 10.1007/s12192-018-0937-7
Wheaton, W. W., Weinberg, S. E., Hamanaka, R. B., Soberanes, S., Sullivan, L. B., Anso, E., et al. (2014). Metformin inhibits mitochondrial complex I of cancer cells to reduce tumorigenesis. eLife 3:e02242. doi: 10.7554/eLife.02242
Whelan, K. A., Chandramouleeswaran, P. M., Tanaka, K., Natsuizaka, M., Guha, M., Srinivasan, S., et al. (2017). Autophagy supports generation of cells with high CD44 expression via modulation of oxidative stress and Parkin-mediated mitochondrial clearance. Oncogene 36, 4843–4858. doi: 10.1038/onc.2017.102
Wieder, S. Y., Serasinghe, M. N., Sung, J. C., Choi, D. C., Birge, M. B., Yao, J. L., et al. (2015). Activation of the mitochondrial fragmentation protein DRP1 correlates with BRAF(V600E) melanoma. J. Investig. Dermatol. 135, 2544–2547. doi: 10.1038/jid.2015.196
Wise, D. R., and Thompson, C. B. (2010). Glutamine addiction: a new therapeutic target in cancer. Trends Biochem. Sci. 35, 427–433. doi: 10.1016/j.tibs.2010.05.003
Wu, T., Harder, B. G., Wong, P. K., Lang, J. E., and Zhang, D. D. (2015). Oxidative stress, mammospheres and Nrf2-new implication for breast cancer therapy? Mol. Carcinog. 54, 1494–1502. doi: 10.1002/mc.22202
Wu, Z., Wei, D., Gao, W., Gao, C., Zhu, X., and Li, Q. (2015). TPO-induced metabolic reprogramming drives liver metastasis of colorectal cancer CD110+ tumor-initiating cells. Stem Cell 17, 47–59. doi: 10.1016/j.stem.2015.05.016
Xia, H., Ooi, L. L. P. J., and Hui, K. M. (2013). MicroRNA-216a/217-induced epithelial-mesenchymal transition targets PTEN and SMAD7 to promote drug resistance and recurrence of liver cancer. Hepatology 58, 629–641. doi: 10.1002/hep.26369
Xie, C., Li, Y., Li, L.-L., Fan, X.-X., Wang, Y.-W., Wei, C. L., et al. (2017). Identification of a new potent inhibitor targeting KRAS in non-small cell lung cancer cells. Front. Pharmacol. 8:823. doi: 10.3389/fphar.2017.00823
Xie, Q., Wu, Q., Horbinski, C. M., Flavahan, W. A., Yang, K., Zhou, W., et al. (2015). Mitochondrial control by DRP1 in brain tumor initiating cells. Nat. Neurosci. 18, 501–510. doi: 10.1038/nn.3960
Xu, B., Wang, S., Li, R., Chen, K., He, L., Deng, M., et al. (2017). Disulfiram/copper selectively eradicates AML leukemia stem cells in vitro and in vivo by simultaneous induction of ROS-JNK and inhibition of NF-κB and Nrf2. Cell Death Dis. 8:e2797. doi: 10.1038/cddis.2017.176
Yahata, T., Takanashi, T., Muguruma, Y., Ibrahim, A. A., Matsuzawa, H., Uno, T., et al. (2011). Accumulation of oxidative DNA damage restricts the self-renewal capacity of human hematopoietic stem cells. Blood 118, 2941–2950. doi: 10.1182/blood-2011-01-330050
Yalcin, S., Zhang, X., Luciano, J. P., Mungamuri, S. K., Marinkovic, D., Vercherat, C., et al. (2008). Foxo3 is essential for the regulation of ataxia telangiectasia mutated and oxidative stress-mediated homeostasis of hematopoietic stem cells. J. Biol. Chem. 283, 25692–25705. doi: 10.1074/jbc.M800517200
Yan, C., Luo, L., Guo, C. Y., Goto, S., Urata, Y., Shao, J. H., et al. (2017). Doxorubicin-induced mitophagy contributes to drug resistance in cancer stem cells from HCT8 human colorectal cancer cells. Cancer Lett. 388, 34–42. doi: 10.1016/j.canlet.2016.11.018
Yang, B., Lu, Y., Zhang, A., Zhou, A., Zhang, L., Zhang, L., et al. (2015). Doxycycline induces apoptosis and inhibits proliferation and invasion of human cervical carcinoma stem cells. PLoS One 10:e0129138. doi: 10.1371/journal.pone.0129138
Yang, T., Fang, S., Zhang, H. X., Xu, L. X., Zhang, Z. Q., Yuan, K. T., et al. (2013). N-3 PUFAs have antiproliferative and apoptotic effects on human colorectal cancer stem-like cells in vitro. J. Nutr. Biochem. 24, 744–753. doi: 10.1016/j.jnutbio.2012.03.023
Yasumoto, Y., Miyazaki, H., Vaidyan, L. K., Kagawa, Y., Ebrahimi, K., Yamamoto, Y., et al. (2016). Inhibition of fatty acid synthase decreases expression of stemness markers in glioma stem cells. PLoS One 11:e0147717. doi: 10.1371/journal.pone.0147717
Ye, H., Adane, B., Khan, N., Sullivan, T., Minhajuddin, M., Gasparetto, M., et al. (2016). Leukemic stem cells evade chemotherapy by metabolic adaptation to an adipose tissue niche. Cell Stem Cell 19, 23–37. doi: 10.1016/J.STEM.2016.06.001
Ye, X. Q., Li, Q., Wang, G. H., Sun, F. F., Huang, G. J., Bian, X. W., et al. (2011). Mitochondrial and energy metabolism-related properties as novel indicators of lung cancer stem cells. Int. J. Cancer 129, 820–831. doi: 10.1002/ijc.25944
Yeh, C. T., Su, C. L., Huang, C. Y., Lin, J. K., Lee, W. H., Chang, P. M., et al. (2013). A preclinical evaluation of antimycin a as a potential antilung cancer stem cell agent. Evid. Based Complement. Alternat. Med. 2013:910451. doi: 10.1155/2013/910451
Yeo, H., Lyssiotis, C. A., Zhang, Y., Ying, H., Asara, J. M., Cantley, L. C., et al. (2013). FoxO3 coordinates metabolic pathways to maintain redox balance in neural stem cells. EMBO J. 32, 2589–2602. doi: 10.1038/emboj.2013.186
Ying, H., Kimmelman, A. C., Lyssiotis, C. A., Hua, S., Chu, G. C., Fletcher-Sananikone, E., et al. (2012). Oncogenic kras maintains pancreatic tumors through regulation of anabolic glucose metabolism. Cell 149, 656–670. doi: 10.1016/j.cell.2012.01.058
Yip, N. C., Fombon, I. S., Liu, P., Brown, S., Kannappan, V., Armesilla, A. L., et al. (2011). Disulfiram modulated ROS–MAPK and NFκB pathways and targeted breast cancer cells with cancer stem cell-like properties. Br. J. Cancer 104, 1564–1574. doi: 10.1038/bjc.2011.126
Yo, Y.-T., Lin, Y.-W., Wang, Y.-C., Balch, C., Huang, R.-L., Chan, M. W. Y., et al. (2012). Growth inhibition of ovarian tumor-initiating cells by niclosamide. Mol. Cancer Ther. 11, 1703–1712. doi: 10.1158/1535-7163.MCT-12-0002
Yu, L., Lu, M., Jia, D., Ma, J., Ben-Jacob, E., Levine, H., et al. (2017). Modeling the genetic regulation of cancer metabolism: interplay between glycolysis and oxidative phosphorylation. Cancer Res. 77, 1564–1574. doi: 10.1158/0008-5472.CAN-16-2074
Yuan, S., Lu, Y., Yang, J., Chen, G., Kim, S., Feng, L., et al. (2015). Metabolic activation of mitochondria in glioma stem cells promotes cancer development through a reactive oxygen species-mediated mechanism. Stem Cell Res. Ther. 6:198. doi: 10.1186/s13287-015-0174-2
Yuan, S., Wang, F., Chen, G., Zhang, H., Feng, L., Wang, L., et al. (2013). Effective elimination of cancer stem cells by a novel drug combination strategy. Stem Cells 31, 23–34. doi: 10.1002/stem.1273
Yue, S., Li, J., Lee, S. Y., Lee, H. Y., Shao, T., Song, B., et al. (2014). Cholesteryl ester accumulation induced by PTEN loss and PI3K/AKT activation underlies human prostate cancer aggressiveness. Cell Metab. 19, 393–406. doi: 10.1016/j.cmet.2014.01.019
Yun, J., Rago, C., Cheong, I., Pagliarini, R., Angenendt, P., Rajagopalan, H., et al. (2009). Glucose deprivation contributes to the development of KRAS pathway mutations in tumor cells. Science 325, 1555–1559. doi: 10.1126/science.1174229
Yun, J. H., Kim, K. A., Yoo, G., Kim, S. Y., Shin, J. M., Kim, J. H., et al. (2017). Phenethyl isothiocyanate suppresses cancer stem cell properties in vitro and in a xenograft model. Phytomedicine 30, 42–49. doi: 10.1016/j.phymed.2017.01.015
Zeng, M., Lu, J., Li, J., Feru, F., Quan, C., Gero, T. W., et al. (2017). Potent and selective covalent quinazoline inhibitors of KRAS G12C. Cell Chem. Biol. 24, 1005–1016.e3. doi: 10.1016/j.chembiol.2017.06.017
Zhang, G. E., Jin, H. L., Lin, X. K., Chen, C., Liu, X. S., Zhang, Q., et al. (2013). Anti-tumor effects of Mfn2 in gastric cancer. Int. J. Mol. Sci. 14, 13005–13021. doi: 10.3390/ijms140713005
Zhang, J., Zhang, Y., Wu, W., Wang, F., Liu, X., and Shui, G. (2017). Guanylate-binding protein 2 regulates Drp1-mediated mitochondrial fission to suppress breast cancer cell invasion. Cell Death Dis. 8:e3151. doi: 10.1038/cddis.2017.559
Zhang, X., Fryknas, M., Hernlund, E., Fayad, W., De Milito, A., Olofsson, M. H., et al. (2014). Induction of mitochondrial dysfunction as a strategy for targeting tumour cells in metabolically compromised microenvironments. Nat. Commun. 5:3295. doi: 10.1038/ncomms4295
Zhang, Z., Duan, Q., Zhao, H., Liu, T., Wu, H., Shen, Q., et al. (2016). Gemcitabine treatment promotes pancreatic cancer stemness through the Nox/ROS/NF-κB/STAT3 signaling cascade. Cancer Lett. 382, 53–63. doi: 10.1016/j.canlet.2016.08.023
Zhao, C., Chen, A., Jamieson, C. H., Fereshteh, M., Abrahamsson, A., Blum, J., et al. (2009). Hedgehog signalling is essential for maintenance of cancer stem cells in myeloid leukaemia. Nature 458, 776–779. doi: 10.1038/nature07737
Zhao, H., Yang, L., Baddour, J., Achreja, A., Bernard, V., Moss, T., et al. (2016). Tumor microenvironment derived exosomes pleiotropically modulate cancer cell metabolism. eLife 5:e10250. doi: 10.7554/eLife.10250
Zhao, J., Zhang, J., Yu, M., Xie, Y., Huang, Y., Wolff, D. W., et al. (2013). Mitochondrial dynamics regulates migration and invasion of breast cancer cells. Oncogene 32, 4814–4824. doi: 10.1038/onc.2012.494
Zhou, D., Shao, L., and Spitz, D. R. (2014). Reactive oxygen species in normal and tumor stem cells. Adv. Cancer Res. 122, 1–67. doi: 10.1016/B978-0-12-420117-0.00001-3
Zhou, J., Li, G., Zheng, Y., Shen, H. M., Hu, X., Ming, Q. L., et al. (2015). A novel autophagy/mitophagy inhibitor liensinine sensitizes breast cancer cells to chemotherapy through DNM1L-mediated mitochondrial fission. Autophagy 11, 1259–1279. doi: 10.1080/15548627.2015.1056970
Zhou, J., Wulfkuhle, J., Zhang, H., Gu, P., Yang, Y., Deng, J., et al. (2007). Activation of the PTEN/mTOR/STAT3 pathway in breast cancer stem-like cells is required for viability and maintenance. Proc. Natl. Acad. Sci. U.S.A. 104, 16158–16163. doi: 10.1073/pnas.0702596104
Zhou, Y., Shingu, T., Feng, L., Chen, Z., Ogasawara, M., Keating, M. J., et al. (2011). Metabolic alterations in highly tumorigenic glioblastoma cells: preference for hypoxia and high dependency on glycolysis. J. Biol. Chem. 286, 32843–32853. doi: 10.1074/jbc.M111.260935
Zhu, J., Wang, H., Sun, Q., Ji, X., Zhu, L., Cong, Z., et al. (2013). Nrf2 is required to maintain the self-renewal of glioma stem cells. BMC Cancer 13:380. doi: 10.1186/1471-2407-13-380
Zong, W.-X., Rabinowitz, J. D., and White, E. (2016). Mitochondria and cancer. Mol. Cell 61, 667-676. doi: 10.1016/j.molcel.2016.02.011
Keywords: cancer stem cells, metabolism, mitochondria, oxidative phosphorylation, lipid metabolism, redox regulation
Citation: Jagust P, de Luxán-Delgado B, Parejo-Alonso B and Sancho P (2019) Metabolism-Based Therapeutic Strategies Targeting Cancer Stem Cells. Front. Pharmacol. 10:203. doi: 10.3389/fphar.2019.00203
Received: 17 December 2018; Accepted: 18 February 2019;
Published: 22 March 2019.
Edited by:
Cyril Corbet, Catholic University of Louvain, BelgiumReviewed by:
Persio Dello Sbarba, Università degli Studi di Firenze, ItalyAnna Rita Cantelmo, Université Lille Nord de France, France
Ivana Kurelac, University of Bologna, Italy
Copyright © 2019 Jagust, de Luxán-Delgado, Parejo-Alonso and Sancho. This is an open-access article distributed under the terms of the Creative Commons Attribution License (CC BY). The use, distribution or reproduction in other forums is permitted, provided the original author(s) and the copyright owner(s) are credited and that the original publication in this journal is cited, in accordance with accepted academic practice. No use, distribution or reproduction is permitted which does not comply with these terms.
*Correspondence: Patricia Sancho, cHNhbmNob0BpaXNhcmFnb24uZXM=