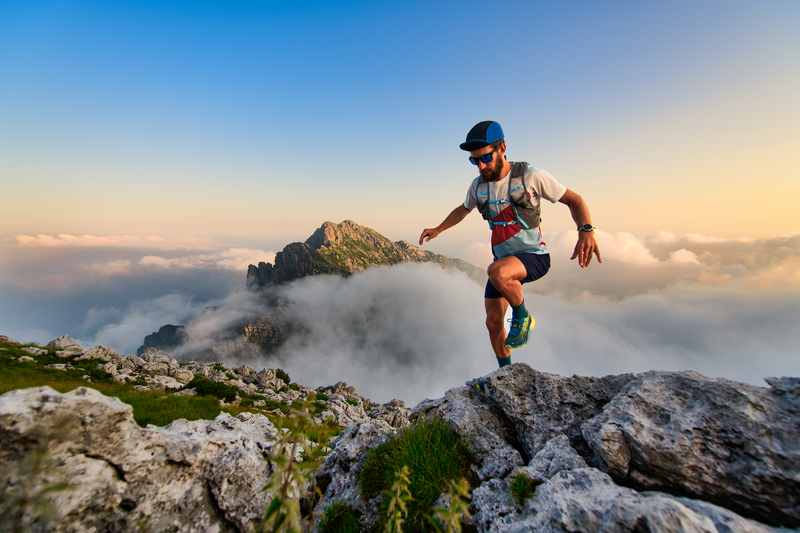
94% of researchers rate our articles as excellent or good
Learn more about the work of our research integrity team to safeguard the quality of each article we publish.
Find out more
REVIEW article
Front. Pharmacol. , 29 October 2018
Sec. Translational Pharmacology
Volume 9 - 2018 | https://doi.org/10.3389/fphar.2018.01199
Extracellular vesicles (EVs)-based therapeutics are based on the premise that EVs shed by stem cells exert similar therapeutic effects and these have been proposed as an alternative to cell therapies. EV-mediated delivery is an effective and efficient system of cell-to-cell communication which can confer therapeutic benefits to their target cells. EVs have been shown to promote tissue repair and regeneration in various animal models such as, wound healing, cardiac ischemia, diabetes, lung fibrosis, kidney injury, and many others. Given the unique attributes of EVs, considerable thought must be given to the preservation, formulation and cold chain strategies in order to effectively translate exciting preclinical observations to clinical and commercial success. This review summarizes current understanding around EV preservation, challenges in maintaining EV quality, and also bioengineering advances aimed at enhancing the long-term stability of EVs.
Interest in extracellular vesicles (EVs) has escalated over the last decade. This has been particularly the case in clinical applications, including the application of EV biology to biomarker discovery, vaccine development, drug delivery, and EV-based therapeutics. EVs are key players in intercellular communication and they are protected from degradation by their lipid bilayer membrane that envelop bioactive cargo. These include proteins, sugars, lipids, and nucleic acids. EVs can be classified based on their size, i.e., apoptotic bodies (>1000 nm), microvesicles (100 – 1000 nm), and exosomes (30 – 150 nm) (Kalra et al., 2016; Tkach and Théry, 2016). The field of EV research is rapidly gaining momentum and overlaps with the newer field of bioengineering where synthetic liposomes, biomimetic vesicles, and nanoparticles have been utilized to package bioactive cargo. In this review, we assess current strategies employed for EV preservation and bioengineering advances aimed at enhancing long term stability of EVs intended for clinical use.
Extracellular vesicles are ideal intercellular transporters of biomolecules. They express surface molecules that enable tissue- or cell-specific targeting. Upon reaching their recipient cells, EVs can induce signaling via receptor-ligand interaction, or be internalized by endocytosis to deliver their cargo. The term “exosomes” is generally used to describe most EVs globally. In an attempt to standardize nomenclature and improve accuracy of data interpretation, the International Society of Extracellular Vesicles (ISEV) published a set of guidelines in 2014 that outlined the so-called minimal requirements to define EVs (Lötvall et al., 2014). The collective term of EVs will be used throughout this review since the definition of exosomes remains contentious. Given the increasing interest in EVs and their potential use in regenerative medicine, isolated EVs must be carefully characterized – this necessarily requires a complex combination of protein profiling (proteomics, western blotting, or flow cytometry), imaging, and nanoparticle tracking analysis.
Extracellular vesicles are secreted by virtually all cell types and present in all bodily fluids. An online public database, ExoCarta1 (Keerthikumar et al., 2016) has been created to curate this diverse body of data, with the goal of facilitating and encouraging collaborative research. This public repository is being continuously updated with new contributions from various EV researchers.
Extracellular vesicles are enriched in membrane proteins and cellular proteins, including the tetraspanins CD63, CD9, CD81, Alix, Tsg101, MHC1 and heat shock proteins (van Niel et al., 2006; Raposo and Stoorvogel, 2013). The protein cargo of EVs include cell-specific proteins which are responsible for specific fates and functions, such as: cell adhesion (integrins, ICAM), signal transductions (G proteins, β-catenin, protein kinases), and intracellular trafficking (RAB, GTPases, annexins) (van Niel et al., 2018). The lipid contents of EVs include ceramides, sphingomyelins, phosphatidylserine, and cholesterol (Laulagnier et al., 2004; Subra et al., 2007). This unique lipid composition is thought to facilitate the uptake of EVs by recipient cells. The lipid composition of EV membranes also play significant roles in intercellular signaling and provide structural stability (Skotland et al., 2017). Furthermore, the surfaces of EVs are surrounded by polysaccharides and glycan (Batista et al., 2011). The nucleic acid cargo in EVs such as mitochondrial DNA, genomic DNA, mRNA, miRNA, and long non-coding RNA have already been documented extensively. Importantly, exosomal RNA play functional roles in EV-mediated cellular communication where exosomal mRNA can be translated into proteins in recipient cells and exosomal microRNA (miR) may regulate gene expression in recipient cells (Valadi et al., 2007).
EV-mediated delivery is an effective and efficient system to confer therapeutic benefits to their target destinations. The contents of EV cargo can be heavily influenced by their producer cells and different cell types will secrete a range of functional effects on recipient cells. The ability of EVs to interact with recipient cells is likely to be affected by the presence of adhesion molecules (e.g., integrins) on the surface of EVs, and this will contribute to the cell or tissue specificity of EVs (van Niel et al., 2018).
Extracellular vesicles exhibit intrinsic therapeutic benefits, for example, EVs can be used as gene delivery vehicles without inducing adverse immune reactions. This contrasts with the more commonly used gene therapy vehicles such as viral vectors and lipid nanoparticles which are immunogenic (Kumar et al., 2014).
There are a number of different strategies to identify and validate EV-mediated cargo delivery into recipient cells. For example, labeling EVs with a tracking dye can result in a quantifiable increase in fluorescence in the recipient cells once exosome uptake occurs. Alternatively, EV-associated RNA labeled with a radioactive tracer can be used to demonstrate uptake by recipient cells (Valadi et al., 2007). For the purposes of this review, we have summarized recent studies describing the therapeutic use of EVs from human cell types in Table 1.
EV-based therapeutics have been proposed as an alternative to cellular therapy, where the latter refers to the use of intact, living cells. In particular, cell therapy exploits the ability of the cellular products to secrete a complex repertoire of bioactive factors including EVs. However, the widespread use of cell therapies has been limited by challenges in the scalability and reproducibility of cell manufacturing. A paradigm shift toward cell-free therapies has now captured the attention of this sector, where the potential of EVs is being explored (Gnecchi et al., 2016; Kusuma et al., 2017). In comparison to cells, EVs have a simplified cold chain process, and have a lower risk profile due to the unlikelihood of spontaneous DNA transformation or immune rejection. Furthermore, EVs can be used directly, either alone or in combination with other pharmacological agents (Fais et al., 2016).
Stem cell-derived EVs have been shown to modulate the immune response from both the innate and adaptive immunity. Favaro et al. (2014, 2016) showed that BMMSCs-EVs induced regulatory dendritic cell (DC) phenotypes with the ability to inhibit T cell activity, while ESC-EVs can reportedly promote M2 macrophage polarization, upregulate Treg numbers and downregulate splenocyte proliferation (Zhang et al., 2014a). Additionally, MSC-EVs were reported to promote Treg proliferation and inhibit autoreactive T cell activity (Del Fattore et al., 2015), as well as induce polymyxin-resistant MyD88-dependent secreted embryonic alkaline phosphatase expression in THP-1 cells (Zhang et al., 2014b). In a mouse model of myasthenia gravis, MSC-EVs reduced T cell-dependent immunoactivation, ameliorated autoimmune injury, and prolonged survival time (Sudres et al., 2017). Additionally, Shigemoto-Kuroda et al. (2017) showed that MSC-EVs modulate immune responses in two different autoimmune mouse models. In a mouse model of type I diabetes, they showed that MSC-EVs delayed the onset of type I diabetes through modulation of IL-1β mediated pancreatic B-cell destruction. Similarly, they showed that 30 μg of MSC-EVs attenuated uveoretinitis triggered by Th1/Th17 activation (Shigemoto-Kuroda et al., 2017).
In murine models of kidney injury, MSC-derived EVs protected against renal injury by reducing levels of creatinine, uric acid, lymphocyte response and fibrosis through shuttling miR-let7c to induce renal tubular cell proliferation (Wang et al., 2016). In a murine model of carbon tetrachloride-induced hepatic injury, concurrent treatments of MSC-EVs attenuated the injury by increasing the proliferation, survival and prevented the apoptosis of hepatocytes (Tan et al., 2014). In animal models of lung injury, MSC and hAEC-EVs have been shown to reduce pulmonary inflammation, improved lung tissue recovery and supported the proliferation of alveolar type II and bronchioalveolar stem cells (Rubenfeld et al., 2005; Cruz et al., 2015; Monsel et al., 2015; Tan et al., 2018). In models of stroke, MSC-EVs delivery of miR-133b directly to neurite cells reportedly enhanced the outgrowth of neurites resulting in increased proliferation of neuroblasts and endothelial cells (Xin et al., 2013). Additionally, Anderson et al. showed through a comprehensive proteomic analysis that MSC-derived EVs mediated angiogenesis via NF-κB signaling (Anderson et al., 2016), while Zhang et al. (2015b) showed that UC MSC-EVs mediated angiogenesis via the Wnt4/β-catenin pathway.
The possibility for EV-based therapeutics to be developed from immune cells is also currently being explored. EVs from dendritic cells have been engineered in various ways to help combat autoimmune diseases. These include stimulating DCs with IFNγ to express miRNAs which stimulate myelination, and reduce oxidative stress (Pusic et al., 2014). Immature DCs (iDCs)-EVs, which have not conformed to their mature role in expressing MHC and co-stimulatory molecules, displayed immunosuppressive properties in autoimmune diseases. For instance, in a mouse model of autoimmune neuromuscular disorder; myasthenia gravis (MG) iDC-derived EVs prevented MG disorder by suppressing lymphocyte reactivity in vivo (Bu et al., 2015). Immune cell-derived EVs are relatively easy to isolate and as such can be beneficial as potential targets for autoimmune and cancer treatments.
There is currently only a handful of clinical trials based on therapeutic EVs registered; all of which are currently still recruiting (Fais et al., 2016; Lener et al., 2015). However only one official trial has been reported to date using ascites-derived exosomes for the treatment of colorectal cancer (Dai et al., 2008). Additionally, in a letter to the editor, the use of stem cell-derived EV administered under compassionate care to patients suffering from graft vs. host disease (GvHD) recorded no adverse effects (Kordelas et al., 2014). The first study was dated back to 2008 (Dai et al., 2008), while the second was published in 2014 (Kordelas et al., 2014). Since then, there is a modest increase in the number of clinical trials with five out of seven using biologically derived EVs while the remaining are plant based EVs. These trials are currently recruiting and are expected to commence in the near future.
Current methods for EV manufacturing are inadequate. Indeed, scalable manufacturing of clinical grade EVs to meet market demands will be a major challenge for this emerging sector for the foreseeable future (Figure 1). Given the unique attributes of EVs, considerable thought must be given to the preservation, formulation, and cold chain strategies in order to effectively translate exciting preclinical observations to clinical and commercial success.
FIGURE 1. Workflow summary of EVs production for clinical use. Schematic of the development of EV therapeutics from preclinical testing to scalable bioprocesses including (A) development of large scale manufacturing of clinical grade EVs through various types of bioreactors, (B) characterization, quality analysis and content screening including factors involved in immunomodulation, angiogenesis, regeneration, tumor antigen presentation, (C) preservation in appropriate storage conditions to maintain the stability and integrity of these factors to meet clinical-scale demands.
Since the commercial and clinical applications of EVs require standard criteria for long-term storage, cryopreservation methods have become a subject of growing interest. This section will describe the current understanding around EV preservation, challenges in maintaining EV stability, and their impact on long term storage and cold chain processes. Table 2 highlights the current preservation methods used in EV for therapeutics purposes.
Cryopreservation with cryoprotectants (CPAs) is a widely accepted procedure to maintain protein stability and prevent osmotic damage (Elliott et al., 2017). Optimum EV dehydration can be achieved in the presence of CPAs by increasing viscosity, impacting the kinetics of ice nucleation, and allowing regulated extracellular ice growth during controlled cooling. However, excessively low concentrations of CPAs may result in chilling shock, which is defined as the damage caused by the freezing process. On the other hand, excessively high concentrations of CPAs can be toxic. Thus, a balance is needed to achieve optimal cryopreservation (Best, 2015).
CPAs refer to a diverse range of sugars, diols, and amino acids which work to stabilize biomolecules in a variety of ways depending on their molecular mass, examples of CPA application in molecular and cell biology is described on Table 3. Penetrating CPAs (pCPA) have low molecular weights (<100 Da) and work by permeating across the lipid bilayer membranes to stabilize the biomolecules (Figure 2). In contrast, non-penetrating CPAs (npCPA) remain external to the vesicle due to their high molecular mass (180–594 Da) and prevent cryodamage from hyperosmotic lysis (Jan et al., 2008; Motta et al., 2014). Notably, there is a growing body of evidence suggesting a combination of both pCPAs and npCPAs is more effective (Table 3) (Willison and Rowe, 1980; Ha et al., 2005).
FIGURE 2. Penetrating vs. non-penetrating CPAs. Penetrating CPAs are low molecular weight molecules that can cross the lipid bilayer membrane and typically must be soluble in water, non-toxic, and can remain in solution at very low temperature. Non-penetrating CPAs have higher molecular weight; and by definition they do not permeate through the membrane and generally utilized at lower concentrations.
A wide range of substances have been used as stabilizers in conventional cryopreservation methods. Specifically, disaccharides are a safe choice for EV-based therapeutics. Trehalose, a natural non-reducing disaccharide, is an FDA-approved CPA for a wide range of proteins and cell products (Eroglu et al., 2000; Buchanan et al., 2004; Motta et al., 2014; Bosch et al., 2016). Following reports showing the importance of adding pCPAs and npCPAs, trehalose was suggested as an ideal candidate to preserve hematopoietic and embryonic stem cells as well as other progenitor cells for therapeutic applications (Buchanan et al., 2004, 2005). Trehalose prevented aggregation by avoiding internal ice formation in biological particles such as liposomes and EVs (Bosch et al., 2016). The addition of trehalose also increased the colloidal stability of EVs (Hood et al., 2014).
Freeze-drying or lyophilisation is currently thought to be the most reliable method to preserve thermolabile materials such as proteins, peptides, vaccines, colloidal carriers, EVs and viruses (Khairnar et al., 2013; Hansen et al., 2015). The first step in lyophilisation involves the freezing or solidification of the EVs, when cooling rate correlates inversely with the size of the ice crystal. The crystallized material is then sublimated directly into water vapor. Freezing and dehydration stresses generated during lyophilisation may result in destructive effects on the structure of biomolecules within the EV, and thus necessitates the use of CPAs in the formulation to protect the EVs and their cargo (Wu et al., 2015a).
The stability of lyophilised EVs significantly extends their shelf life, lowers storage demands, and costs owing to a simplified cold chain. For example, the best storage temperature reported for lyophilised EVs isolated from cardiospheres was 4°C (Kreke et al., 2016). The most common stabilizers used in lyophilisation are disaccharides such as glucose, lactose, sucrose and trehalose, which work by replacing the hydration sphere around the EVs through a hydrogen bonding interaction with phospholipid head groups to form an amorphous sugar glass. The glassy state produced in the presence of disaccharides prevent fusion of products or protein destabilization (Jain and Roy, 2009).
Trehalose has been suggested as the most effective disaccharide to preserve EVs during lyophilisation (Chen et al., 2010; Bosch et al., 2016). This promising technique is an FDA-approved method for a range of proteins, liposomes and nanoparticles that enables their use in the pharmaceutical industry (Van Backstal et al., 2017).
Spray drying is a common method for producing a wide variety of therapeutic agents including vaccines, peptides and proteins for inhaled delivery (Broadhead et al., 1992; Chan et al., 1997; Salama et al., 2009). This single-step process substantially reduces the need for expensive equipment and lengthier multi-step processes. Spray drying is scalable and operators are able to tune the particle size of the final product by controlling the spray droplet size and solute concentration, thereby providing a major point of difference from lyophilisation where the particle size reduction can occur only through mechanical milling (Costantino et al., 2000).
Spray drying involves an initial step of atomising the solution containing EVs. These droplets are rapidly converted into a dry powder using heated gas (Lee, 2002). Spray drying is a continuous process and can be both automated and instrumented for enhanced process control. The reduction in moisture content of particles formed during the spray drying process generally increases the stability of the biopharmaceuticals in these particles: the residual moisture acts as a plasticiser to reduce glass transition temperature of the particle solid state, and its presence may also enhance chemical instability. Critical process parameters such as the rate at which EV solution is being fed into the system, the atomisation pressure and outlet temperature, can all affect the stability of the EVs and their cargo. These critical process parameters must therefore be identified and maintained within a narrow window (Masters, 1972). Behfar (2016) patented a technique to encapsulate the platelet rich solution EVs as a candidate for wound healing (US20160324794A1). However, further investigation is needed to apply this technique more broadly to the manufacturing and storage of EV-based therapeutics.
In order for EV-based therapeutics to be manufactured and used reproducibly, storage conditions must have minimal impact on EV structural integrity. The following section will discuss parameters known to affect EV composition, biological potency and structural integrity.
There have been a number of studies conducted to determine the most favorable storage conditions for EVs. Focusing on EVs with intended therapeutic applications, EVs from human embryonic kidney (HEK) 293T cells, endothelial colony forming cells (ECFCs) and MSCs report -20°C as the highest temperature in which EVs are stable (Sokolova et al., 2011). These results are in line with the standard preservation temperature reported by ISEV for EVs storage. In contrast, another study has reported that -70°C is the best long-term storage temperature for EVs isolated using the Exo-Quick kit (System Biosciences, Palo Alto, CA, United States) (Lee et al., 2016).
While freeze-thaw cycles do not affect the stability of EVs isolated from plasma and exosomal miRNA and different cell types like HEK293T, ECFCs and MSCs (Sokolova et al., 2011; Lv et al., 2013; Ge et al., 2014), other studies show that EVs can be structurally susceptible due to the exposure of vulnerable phosphatidylserine to repeated freeze-thaw cycles (Wu et al., 2015b; Maroto et al., 2017). This is an area that must be deconvoluted as EV-based therapeutics are being developed, in order to establish a clear product stability profile as required by regulatory bodies.
A preparation of EVs can be considered as a colloid – a solution in which microscopically dispersed particles are suspended (Hood et al., 2014). From this perspective, there are several known phenomena that can be applied to EVs, providing a rationale underlying the basis of possible approaches that can be used to increase the stability and quality of stored EVs. One of the major challenges in EV storage, particle aggregation, occurs when inter-particle attraction is greater than repulsion. Such interactions are governed by factors such as surface charge, hydrophobicity and fluidity (Takeuchi et al., 2000). Strategies to prevent EV aggregation must therefore modify these factors to increase inter-particle repulsion and stabilize the colloidal solution.
Although EV biology is a relatively new field, EVs share many overarching structural features with liposomes – lipid bilayered vesicles that have been well-studied due to their utility as drug delivery vehicles. Looking toward liposome studies, the use of hydrophilic polymers as steric stabilizers may be a good strategy for preservation of a colloidal system. When using polymers, it is thought that the hydrophilic chains extend from the liposomes out into the solution thereby stabilizing the system so that the individual particles remain well-dispersed (Figure 3).
FIGURE 3. Stabilization strategy for EVs. (A) Particles in colloidal suspension. (B) Steric stabilization achieved by polymer chains attached to particles to decrease inter-particle interactions.
The most common polymer used in liposome stabilization is PEG (polyethylene glycol). Advantages of PEG include the fact that it is non-fouling, well-tolerated by the body, can be obtained in a wide range of molecular weights and end-group chemistries and that it is FDA approved for a range of medical applications (Hasan, 2017). There are many examples in which liposomes have been PEGylated, i.e., the PEG chains are incorporated within the lipid bilayer during synthesis (e.g., PEGlyated liposomes to incorporate itraconazole, antifungal agent, as well as dopamine-loaded PEGylated immunoliposomes; Kang et al., 2016; Dzieciuch-Rojek et al., 2017). Although effective, such a strategy is unsuitable for EV stabilization. Coating the particles in polymer can have a similar effect and would be a much more suitable strategy for EV preservation, allowing EVs to be stabilized by the simple addition of polymer to the isolated preparation. Other polymers that have been used include the synthetic polymer PVA (polyvinylalcohol), and the naturally derived polysaccharides OPP (O-palmitoylpullulan), chitosan, and hyaluronic acid (Sehgal and Rogers, 1995; Takeuchi et al., 2000; Manconi et al., 2017). Specific stabilization of EVs has thus far been limited to the use of trehalose. Addition of trehalose to solutions of EVs was proven to enhance colloidal stability during electroporation, for the modification of EV cargo (Hood et al., 2014). Addition of 25 mM trehalose to EVs derived from pancreatic beta cells was observed to narrow the particle size distribution (i.e., increase the stability) and improve the particle yield (Bosch et al., 2016), presumably by also reducing loss of EVs through interactions with the walls of the storage vessel.
The matrix of tissues in the body hosts a population of vesicles, often termed matrix bound vesicles (MBVs) (Shapiro et al., 2015; Huleihel et al., 2016). In a similar manner to the protection of growth factors by sequestration and release from the extracellular matrix (ECM), the binding of these vesicles has a vital role in enhancing their stability and biological availability. Although, there is still debate as to whether MBVs possess all of the characteristics required to be defined as an EV, there is also evidence that EVs can bind to ECM components; for example, a study by Narayanan et al. showed binding of MSC-derived EVs to bind to both fibronectin and collagen type I in the ECM (Narayanan et al., 2016). Such interactions between EVs and the ECM are likely mediated by adhesion receptors, known to be present on the exosomal membrane, including integrins, tetraspanins, and ICAM-1 (Escola et al., 1998; Thery et al., 1999, 2001; Rana and Zoller, 2013).
In the case of MBVs, interaction with the matrix has proven to enhance their stability. MBVs can survive chemical, enzymatic and detergent-based treatments and subsequently induce changes in cellular behavior (Huleihel et al., 2016). These intriguing findings indicate that incorporation of EVs with ECM or biomaterial components may be a powerful tool to both enhance EV stability and provide a controlled spatiotemporal release within the body. This premise is supported by a few early studies in which EVs have been incorporated into biomaterial constructs for delivery. For example, Zhang et al. (2016) stabilized MSC-derived EVs by incorporation into porous tricalcium phosphate (β-TCP) scaffolds. In doing so they demonstrated that EVs could be released over several days and further that the function of these EVs in promoting bone repair was retained. In another study, Shi et al. combined MSC-EVs with a hydrogel synthesized from chitosan and silk, showing that EVs incorporated into the biomaterial could be released over time and retained their function to improve wound healing (Shi et al., 2017). Although, in its infancy, these studies uniting EV biology and bioengineering provide an exciting glimpse into future applications of biomaterials to preserve and deliver EVs for therapeutic application.
Given that EVs largely retain the properties of their cells of origin, it is unsurprising that cell therapy companies have jumped on this particular bandwagon in order to maximize the proprietary cell lines. For example, Capricor Therapeutics (Beverly Hills, CA, United States) are investigating the clinical potential of CAP-2003, which refer to the EVs produced by their proprietary cardiosphere-derived cells. Capricor has made efforts to evaluate the regenerative potential of these cardiosphere-derived EVs on diseases involving inflammation and fibrosis (Ibrahim et al., 2014). Similarly, cell therapy company specializing in neurological disease, ReNeuron, has sought to do the same with EVs from their proprietary CTX neural cell line, which are currently in Phase IIb clinical trials for US-based patients living with post-stroke disabilities. It is likely that we will need an emergence of EV-based therapeutics from other cell therapy companies as the proverbial penny drops – there is immense value in what was essentially considered a waste product of cell manufacturing.
Regardless of whether EVs will be used for the purposes of regenerative medicine, cancer vaccination, veterinary or agriculture, there is an obvious need to develop methods to reliably store, transport and apply the EVs. Of these considerations, storage of the EVs is perhaps the most critical aspect of the supply chain. The stability of the EVs in their storage medium necessarily dictates the rigidity of the cold chain and will have direct impact on the cost of goods. Investment into technologies that refine the stability of EVs will likely afford significant cost savings downstream. The storage medium will also impact the final formulation of the EV therapeutic as challenges around solubility of injectables and particle size of aerosols must be considered. These factors will have knock-on effects on biodistribution and therapeutic efficacy. As such, rigorous preclinical testing should be designed with this in mind, in order to expedite product development and facilitate regulatory approval.
The FDA approval of chimeric antigen receptor T cells (CAR-T), Kymriah (Novartis) for refractory B-cell precursor acute lymphoblastic leukemia in August 2017, heralded the dawn of a new age for cell therapies. There are, however, broader implications for these approved cell therapies. Chief amongst these is the growing acceptance of cellular therapies and regenerative medicine in mainstream clinical care. However, the relative high cost of goods remains prohibitive for cellular therapies. Challenges in scalable manufacturing, maintenance of a master cell bank, complex cold chain logistics and ambiguity around product release criteria, have led to lengthy delays in realizing the potential of cellular therapies. While regulatory hurdles for this new class of biologics remain a challenge to be met, it is likely that the relative stability of EVs will see a significantly expedited path to regulatory approval. Furthermore, as critical questions around scalable manufacturing and long-term preservation are answered, EV-based therapeutics may offer a more affordable form of regenerative medicine, thereby increasing market penetration and patient access. In essence, the development of novel preservation protocols tailored for EVs are likely to fast forward the manufacturing process to establish EVs as commercially viable therapeutics.
GK, MB, JT, DM, JF, and RL contributed to the writing and editing of this manuscript. GK and JT prepared the figures. GK and MB prepared the tables.
The work was supported by Interdisciplinary Research Seed Funding Scheme from Monash University (awarded to JF and RL). This work is supported by the Victorian Government’s Operational Infrastructure Support Program.
The authors declare that the research was conducted in the absence of any commercial or financial relationships that could be construed as a potential conflict of interest.
Abdelwahed, W., Degobert, G., and Fessi, H. (2006a). A pilot study of freeze drying of poly(epsilon-caprolactone) nanocapsules stabilized by poly(vinyl alcohol): formulation and process optimization. Int. J. Pharm. 309, 178–188. doi: 10.1016/j.ijpharm.2005.10.003
Abdelwahed, W., Degobert, G., and Fessi, H. (2006b). Investigation of nanocapsules stabilization by amorphous excipients during freeze-drying and storage. Eur. J. Pharm. Biopharm. 63, 87–94. doi: 10.1016/j.ejpb.2006.01.015
Admyre, C., Bohle, B., Johansson, S. M., Focke-Tejkl, M., Valenta, R., Scheynius, A., et al. (2007). B cell-derived exosomes can present allergen peptides and activate allergen-specific T cells to proliferate and produce TH2-like cytokines. J. Allergy Clin. Immunol. 120, 1418–1424. doi: 10.1016/j.jaci.2007.06.040
Alihosseini, F., Ghaffari, S., Dabirsiaghi, A. R., and Haghighat, S. (2015). Freeze-drying of ampicillin solid lipid nanoparticles using mannitol as cryoprotectant. Braz. J. Pharm. Sci. 51, 797–802. doi: 10.1590/S1984-82502015000400005
Almalik, A., Alradwan, I., Kalam, M. A., and Alshamsan, A. (2017). Effect of cryoprotection on particle size stability and preservation of chitosan nanoparticles with and without hyaluronate or alginate coating. Saudi Pharm. J. 25, 861–867. doi: 10.1016/j.jsps.2016.12.008
Amarnath, S., Foley, J. E., Farthing, D. E., Gress, R. E., Laurence, A., Eckhaus, M. A., et al. (2015). Bone marrow-derived mesenchymal stromal cells harness purinergenic signaling to tolerize human th1 cells in vivo. Stem Cells 33, 1200–1212. doi: 10.1002/stem.1934
Anderson, J. D., Johansson, H. J., Graham, C. S., Vesterlund, M., Pham, M. T., Bramlett, C. S., et al. (2016). Comprehensive proteomic analysis of mesenchymal stem cell exosomes reveals modulation of angiogenesis via nuclear factor-kappaB signaling. Stem Cells 34, 601–613. doi: 10.1002/stem.2298
Arslan, F., Lai, R. C., Smeets, M. B., Akeroyd, L., Choo, A., Aguor, E. N. E., et al. (2013). Mesenchymal stem cell-derived exosomes increase ATP levels, decrease oxidative stress and activate PI3K/Akt pathway to enhance myocardial viability and prevent adverse remodeling after myocardial ischemia/reperfusion injury. Stem Cell Res. 10, 301–312. doi: 10.1016/j.scr.2013.01.002
Badowski, M., Muise, A., and Harris, D. T. (2014). Mixed effects of long-term frozen storage on cord tissue stem cells. Cytotherapy 16, 1313–1321. doi: 10.1016/j.jcyt.2014.05.020
Batista, B. S., Eng, W. S., Pilobello, K. T., Hendricks-Munoz, K. D., and Mahal, L. K. (2011). Identification of a conserved glycan signature for microvesicles. J. Proteome Res. 10, 4624–4633. doi: 10.1021/pr200434y
Beattie, G. M., Crowe, J. H., Lopez, A. D., Cirulli, V., Ricordi, C., and Hayek, A. (1997). Trehalose: a cryoprotectant that enhances recovery and preserves function of human pancreatic islets after long-term storage. Diabetes Metab. Res. Rev. 46, 519–523. doi: 10.2337/diab.46.3.519
Best, B. P. (2015). Cryoprotectant toxicity: facts, issues, and questions. Rejuvenation Res. 18, 422–436. doi: 10.1089/rej.2014.1656
Bissoyi, A., and Pramanik, K. (2013) Effects of non-toxic cryoprotective agents on the viability of cord blood derived MNCs. Cryo. Letters 34, 453–465.
Bosch, S., De Beaurepaire, L., Allard, M., Mosser, M., Heichette, C., Chrétien, D., et al. (2016). Trehalose prevents aggregation of exosomes and cryodamage. Sci. Rep. 6:36162. doi: 10.1038/srep36162
Bozzo, T. (1999). Blood component recalls. Transfusion 39, 439–441. doi: 10.1046/j.1537-2995.1999.39050439.x
Broadhead, J., Edmond Rouan, S. K., and Rhodes, C. T. (1992). The spray drying of pharmaceuticals. Drug Dev. Ind. Pharm. 18, 1169–1206. doi: 10.3109/03639049209046327
Bruder, S. P., Jaiswal, N., and Haynesworth, S. E. (1997). Growth kinetics, self-renewal, and the osteogenic potential of purified human mesenchymal stem cells during extensive subcultivation and following cryopreservation. J. Cell. Biochem. 64, 278–294. doi: 10.1002/(SICI)1097-4644(199702)64:2<278::AID-JCB11>3.0.CO;2-F
Bruno, S., Grange, C., Collino, F., Deregibus, M. C., Cantaluppi, V., Biancone, L., et al. (2012). Microvesicles derived from mesenchymal stem cells enhance survival in a lethal model of acute kidney injury. PLoS One 7:e33115. doi: 10.1371/journal.pone.0033115
Bruno, S., Grange, C., Deregibus, M. C., Calogero, R. A., Saviozzi, S., Collino, F., et al. (2009). Mesenchymal stem cell-derived microvesicles protect against acute tubular injury. J. Am. Soc. Nephrol. 20, 1053–1067. doi: 10.1681/ASN.2008070798
Bu, N., Wu, H.-Q., Zhang, G.-L., Zhan, S.-Q., Zhang, R., Fan, Q.-Y., et al. (2015). Immature dendritic cell exosomes suppress experimental autoimmune myasthenia gravis. J. Neuroimmunol. 285, 71–75. doi: 10.1016/j.jneuroim.2015.04.009
Buchanan, S. S., Gross, S. A., Acker, J. P., Toner, M., Carpenter, J. F., and Pyatt, D. W. (2004). Cryopreservation of stem cells using trehalose: evaluation of the method using a human hematopoietic cell line. Stem Cells Dev. 13, 295–305. doi: 10.1089/154732804323099226
Buchanan, S. S., Menze, M. A., Hand, S. C., Pyatt, D. W., and Carpenter, J. F. (2005). Cryopreservation of human hematopoietic stem and progenitor cells loaded with trehalose: transient permeabilization via the adenosine triphosphate-dependent P2Z receptor channel. Cell Preserv. Technol. 3, 212–222. doi: 10.1089/cpt.2005.3.212
Cantaluppi, V., Biancone, L., Figliolini, F., Beltramo, S., Medica, D., Deregibus, M. C., et al. (2012). Microvesicles derived from endothelial progenitor cells enhance neoangiogenesis of human pancreatic islets. Cell Transplant. 21, 1305–1320. doi: 10.3727/096368911X627534
Cardoso, L. M. D. F., Pinto, M. A., Henriques Pons, A., and Alves, L. A. (2017). Cryopreservation of rat hepatocytes with disaccharides for cell therapy. Cryobiology 78, 15–21. doi: 10.1016/j.cryobiol.2017.07.010
Carrasco-Ramírez, P., Greening, D. W., Andrés, G., Gopal, S. K., Martín-Villar, E., Renart, J., et al. (2016). Podoplanin is a component of extracellular vesicles that reprograms cell-derived exosomal proteins and modulates lymphatic vessel formation. Oncotarget 7, 16070–16089. doi: 10.18632/oncotarget.7445
Casado-Díaz, A., Santiago-Mora, R., Jiménez, R., Caballero-Villarraso, J., Herrera, C., Torres, A., et al. (2008). Cryopreserved human bone marrow mononuclear cells as a source of mesenchymal stromal cells: application in osteoporosis research. Cytotherapy 10, 460–468. doi: 10.1080/14653240802192644
Chacón, M., Molpeceres, J., Berges, L., Guzmán, M., and Aberturas, M. R. (1999). Stability and freeze-drying of cyclosporine loaded poly(D,L-lactide-glycolide) carriers. Eur. J. Pharm. Sci. 8, 99–107. doi: 10.1016/S0928-0987(98)00066-9
Chan, H. K., Clark, A., Gonda, I., Mumenthaler, M., and Hsu, C. (1997). Spray dried powders and powder blends of recombinant human deoxyribonuclease (rhDNase) for aerosol delivery. Pharm. Res. 14, 431–437. doi: 10.1023/A:1012035113276
Chang, Y.-P., Hong, H.-P., Lee, Y.-H., and Liu, I.-H. (2015). The canine epiphyseal-derived mesenchymal stem cells are comparable to bone marrow derived-mesenchymal stem cells. J. Vet. Med. Sci. 77, 273–280. doi: 10.1292/jvms.14-0265
Chase, L. C. G., Rao, M. S. G., and Vemuri, M. S. (2011). Mesenchymal stem cell assays and applications. Methods Mol. Biol. 698, 3–8. doi: 10.1007/978-1-60761-999-4
Chatzistamatiou, T. K., Papassavas, A. C., Michalopoulos, E., Gamaloutsos, C., Mallis, P., Gontika, I., et al. (2014). Optimizing isolation culture and freezing methods to preserve Wharton’s jelly’s mesenchymal stem cell (MSC) properties: an MSC banking protocol validation for the Hellenic Cord Blood Bank. Transfusion 54, 3108–3120. doi: 10.1111/trf.12743
Chen, C., Han, D., Cai, C., and Tang, X. (2010). An overview of liposome lyophilization and its future potential. J. Control. Release 142, 299–311. doi: 10.1016/j.jconrel.2009.10.024
Chen, G., Yue, A., Ruan, Z., Yin, Y., Wang, R., Ren, Y., et al. (2016). Comparison of the effects of different cryoprotectants on stem cells from umbilical cord blood. Stem Cells Int. 2016:1396783. doi: 10.1155/2016/1396783
Cheng, Y., Zeng, Q., Han, Q., and Xia, W. (2018). Effect of pH, temperature and freezing-thawing on quantity changes and cellular uptake of exosomes. Protein Cell 7, 1–5. doi: 10.1007/s13238-018-0529-4
Choudhery, M. S., Badowski, M., Muise, A., Pierce, J., and Harris, D. T. (2014). Cryopreservation of whole adipose tissue for future use in regenerative medicine. J. Surg. Res. 187, 24–35. doi: 10.1016/j.jss.2013.09.027
Colino, J., and Snapper, C. M. (2006). Exosomes from bone marrow dendritic cells pulsed with diphtheria toxoid preferentially induce type 1 antigen-specific IgG responses in naive recipients in the absence of free antigen. J. Immunol. 177, 3757–3762. doi: 10.4049/jimmunol.177.6.3757
Costantino, H. R., Firouzabadian, L., Hogeland, K., Wu, C., Beganski, C., Carrasquillo, K. G., et al. (2000). Protein spray-freeze drying. Effect of atomization conditions on particle size and stability. Pharm. Res. 17, 1374–1383. doi: 10.1023/A:1007570030368
Crowe, J. H., Crowe, L. M., Carpenter, J. F., and Aurell Wistrom, C. (1987). Stabilization of dry phospholipid bilayers and proteins by sugars. Biochem. J. 242, 1–10. doi: 10.1042/bj2420001
Cruz, F. F., Borg, Z. D., Goodwin, M., Sokocevic, D., Wagner, D. E., Coffey, A., et al. (2015). Systemic administration of human bone marrow-derived mesenchymal stromal cell extracellular vesicles ameliorates aspergillus hyphal extract-induced allergic airway inflammation in immunocompetent mice. Stem Cells Transl. Med. 4, 1302–1316. doi: 10.5966/sctm.2014-0280
Cui, Z., Hsu, C. H., and Mumper, R. J. (2003). Physical characterization and macrophage cell uptake of mannan-coated nanoparticles. Drug Dev. Ind. Pharm. 29, 689–700. doi: 10.1081/DDC-120021318
Dai, S., Wei, D., Wu, Z., Zhou, X., Wei, X., Huang, H., et al. (2008). Phase I clinical trial of autologous ascites-derived exosomes combined with GM-CSF for colorectal cancer. Mol. Ther. 16, 782–790. doi: 10.1038/mt.2008.1
Damjanovic, V., and Thomas, D. (1974). The use of polyvinylpyrrolidone as a cryoprotectant in the freezing of human lymphocytes. Cryobiology 11, 312–316. doi: 10.1016/0011-2240(74)90007-8
Damo, M., Wilson, D. S., Simeoni, E., and Hubbell, J. A. (2015). TLR-3 stimulation improves anti-tumor immunity elicited by dendritic cell exosome-based vaccines in a murine model of melanoma. Sci. Rep. 5:17622. doi: 10.1038/srep17622
Dariolli, R., Bassaneze, V., Nakamuta, J. S., Omae, S. V., Campos, L. C. G., and Krieger, J. E. (2013). Porcine adipose tissue-derived mesenchymal stem cells retain their proliferative characteristics, senescence, karyotype and plasticity after long-term cryopreservation. PLoS One 8:e67939. doi: 10.1371/journal.pone.0067939
Date, P. V., Samad, A., and Devarajan, P. V. (2010). Freeze thaw: a simple approach for prediction of optimal cryoprotectant for freeze drying. AAPS PharmSciTech 11, 304–313. doi: 10.1208/s12249-010-9382-3
De Lara Janz, F., De Aguiar Debes, A., De Cássia Cavaglieri, R., Duarte, S. A., Romão, C. M., Morón, A. F., et al. (2012). Evaluation of distinct freezing methods and cryoprotectants for human amniotic fluid stem cells cryopreservation. J. Biomed. Biotechnol. 2012:649353. doi: 10.1155/2012/649353
Del Fattore, A., Luciano, R., Pascucci, L., Goffredo, B. M., Giorda, E., Scapaticci, M., et al. (2015). Immunoregulatory effects of mesenchymal stem cell-derived extracellular vesicles on T lymphocytes. Cell Transplant. 24, 2615–2627. doi: 10.3727/096368915X687543
Deregibus, M. C., Cantaluppi, V., Calogero, R., Lo Iacono, M. Tetta, C., Bruno, S., et al. (2012). Endothelial progenitor cell derived microvesicles activate an angiogenic program in endothelial cells by a horizontal transfer of mRNA. Blood 110, 2440–2448. doi: 10.1182/blood-2007-03-078709
Ding, G., Wang, W., Liu, Y., An, Y., Zhang, C., Shi, S., et al. (2010). Effect of cryopreservation on biological and immunological properties of stem cells from apical papilla. J. Cell. Physiol. 223, 415–422. doi: 10.1002/jcp.22050
Dulugiac, M., Moldovan, L., and Zarnescu, O. (2015). Comparative studies of mesenchymal stem cells derived from different cord tissue compartments - The influence of cryopreservation and growth media. Placenta 36, 1192–1203. doi: 10.1016/j.placenta.2015.08.011
Dzieciuch-Rojek, M., Poojari, C., Bednar, J., Bunker, A., Kozik, B., Nowakowska, M., et al. (2017). Effects of membrane PEGylation on entry and location of antifungal drug itraconazole and their pharmacological implications. Mol. Pharm. 14, 1057–1070. doi: 10.1021/acs.molpharmaceut.6b00969
Edamura, K., Nakano, R., Fujimoto, K., Teshima, K., Asano, K., and Tanaka, S. (2014). Effects of cryopreservation on the cell viability, proliferative capacity and neuronal differentiation potential of canine bone marrow stromal cells. J. Vet. Med. Sci. 76, 573–577. doi: 10.1292/jvms.13-0296
Eirin, A., Zhu, X.-Y., Puranik, A. S., Tang, H., McGurren, K. A., van Wijnen, A. J., et al. (2017). Mesenchymal stem cell-derived extracellular vesicles attenuate kidney inflammation. Kidney Int. 92, 114–124. doi: 10.1016/j.kint.2016.12.023
El Assal, R., Guven, S., Gurkan, U. A., Gozen, I., Shafiee, H., Dalbeyler, S., et al. (2014). Bio-inspired cryo-ink preserves red blood cell phenotype and function during nanoliter vitrification. Adv. Mater. 26, 5815–5822. doi: 10.1002/adma.201400941
Elliott, G. D., Wang, S., and Fuller, B. J. (2017). Cryobiology Cryoprotectants : a review of the actions and applications of cryoprotective solutes that modulate cell recovery from ultra-low temperatures. Cryobiology 76, 74–91. doi: 10.1016/j.cryobiol.2017.04.004
El-Nesr, O. H., Yahiya, S. A., and El-Gazayerly, O. N. (2010). Effect of formulation design and freeze-drying on properties of fluconazole multilamellar liposomes. Saudi Pharm. J. 18, 217–224. doi: 10.1016/j.jsps.2010.07.003
Eroglu, A., Russo, M. J., Bieganski, R., Fowler, A., Cheley, S., Bayley, H., et al. (2000). Intracellular trehalose improves the survival of cryopreserved mammalian cells. Nat. Biotechnol. 18, 163–167. doi: 10.1038/72608
Eroglu, A., Toner, M., and Toth, T. L. (2002). Beneficial effect of microinjected trehalose on the cryosurvival of human oocytes. Fertil. Steril. 77, 152–158. doi: 10.1016/S0015-0282(01)02959-4
Escola, J. M., Kleijmeer, M. J., Stoorvogel, W., Griffith, J. M., Yoshie, O., and Geuze, H. J. (1998). Selective enrichment of tetraspan proteins on the internal vesicles of multivesicular endosomes and on exosomes secreted by human B-lymphocytes. J. Biol. Chem. 273, 20121–20127. doi: 10.1074/jbc.273.32.20121
Fais, S., O’Driscoll, L., Borras, F. E., Buzas, E., Camussi, G., Cappello, F., et al. (2016). Evidence-based clinical use of nanoscale extracellular vesicles in nanomedicine. ACS Nano 10, 3886–3899. doi: 10.1021/acsnano.5b08015
Favaro, E., Carpanetto, A., Caorsi, C., Giovarelli, M., Angelini, C., Cavallo-Perin, P., et al. (2016). Human mesenchymal stem cells and derived extracellular vesicles induce regulatory dendritic cells in type 1 diabetic patients. Diabetologia 59, 325–333. doi: 10.1007/s00125-015-3808-0
Favaro, E., Carpanetto, A., Lamorte, S., Fusco, A., Caorsi, C., Deregibus, M. C., et al. (2014). Human mesenchymal stem cell-derived microvesicles modulate T cell response to islet antigen glutamic acid decarboxylase in patients with type 1 diabetes. Diabetologia 57, 1664–1673. doi: 10.1007/s00125-014-3262-4
Feng, Y., Huang, W., Wani, M., Yu, X., and Ashraf, M. (2014). Ischemic preconditioning potentiates the protective effect of stem cells through secretion of exosomes by targeting Mecp2 via miR-22. PLoS One 9:e88685. doi: 10.1371/journal.pone.0088685
Fonte, P., Soares, S., Costa, A., Andrade, J. C., Seabra, V., Reis, S., et al. (2012). Effect of cryoprotectants on the porosity and stability of insulin-loaded PLGA nanoparticles after freeze-drying. Biomatter 2, 329–339. doi: 10.4161/biom.23246
Freimark, D., Sehl, C., Weber, C., Hudel, K., Czermak, P., Hofmann, N., et al. (2011). Systematic parameter optimization of a Me2SO- and serum-free cryopreservation protocol for human mesenchymal stem cells. Cryobiology 63, 67–75. doi: 10.1016/j.cryobiol.2011.05.002
Gala, R. P., Khan, I., Elhissi, A. M. A., and Alhnan, M. A. (2015). A comprehensive production method of self-cryoprotected nano-liposome powders. Int. J. Pharm. 486, 153–158. doi: 10.1016/j.ijpharm.2015.03.038
Ge, Q., Zhou, Y., Lu, J., Bai, Y., Xie, X., and Lu, Z. (2014). MiRNA in plasma exosome is stable under different storage conditions. Molecules 19, 1568–1575. doi: 10.3390/molecules19021568
Gnecchi, M., Danieli, P., Malpasso, G., and Ciuffreda, M. C. (2016). “Paracrine mechanisms of mesenchymal stem cells in tissue repair,” in Mesenchymal Stem Cells: Methods and Protocols, ed. M. Gnecchi (New York, NY: Springer), 123–146. doi: 10.1007/978-1-4939-3584-0_7
Ha, S. Y., Jee, B. C., Suh, C. S., Kim, H. S., Oh, S. K., Kim, S. H., et al. (2005). Cryopreservation of human embryonic stem cells without the use of a programmable freezer. Hum. Reprod. 20, 1779–1785. doi: 10.1093/humrep/deh854
Hansen, L. J. J., Daoussi, R., Vervaet, C., Remon, J. -P., and De Beer, T. R. M. (2015). Freeze-drying of live virus vaccines: a review. Vaccine 33, 5507–5519. doi: 10.1016/j.vaccine.2015.08.085
Harrigan, P. R., Madden, T. D., and Cullis, P. R. (1990). Protection of liposomes during dehydration or freezing. Chem. Phys. Lipids 52, 139–149. doi: 10.1016/0009-3084(90)90157-M
Hasan, A. (2017). Tissue Engineering for Artificial Organs. Available at: https://onlinelibrary.wiley.com/doi/book/10.1002/9783527689934
Hau, Z. Z., Li, B. G., Liu, Z. J., and Sun, D. W. (2003). Freeze-drying of liposomes with cryoprotectants and its effect on retention rate of encapsulated ftorafur and vitamin A. Dry. Technol. 21, 1491–1505. doi: 10.1081/DRT-120024489
Heinrich, U., Garbe, B., and Tronnier, H. (2007). In vivo assessment of ectoin: a randomized, vehicle-controlled clinical trial. Skin Pharmacol. Physiol. 20, 211–218. doi: 10.1159/000103204
Hendriks, J., Riesle, J., and van Blitterswijk, C. A. (2010). Co-culture in cartilage tissue engineering. J. Tissue Eng. Regen. Med. 4, 524–531. doi: 10.1002/term
Hergenreider, E., Heydt, S., Treguer, K., Boettger, T., Horrevoets, A. J. G., Zeiher, A. M., et al. (2012). Atheroprotective communication between endothelial cells and smooth muscle cells through miRNAs. Nat. Cell Biol. 14, 249–256. doi: 10.1038/ncb2441
Hewson, C., Capraro, D., Burdach, J., Whitaker, N., and Morris, K. V. (2016). Extracellular vesicle associated long non-coding RNAs functionally enhance cell viability. Noncoding RNA Res. 1, 3–11. doi: 10.1016/j.ncrna.2016.06.001
Hood, J. L., Scott, M. J., and Wickline, S. A. (2014). Maximizing exosome colloidal stability following electroporation. Anal. Biochem. 448, 41–49. doi: 10.1016/j.ab.2013.12.001
Hu, G. W., Li, Q., Niu, X., Hu, B., Liu, J., Zhou, S. M., et al. (2015). Exosomes secreted by human-induced pluripotent stem cell-derived mesenchymal stem cells attenuate limb ischemia by promoting angiogenesis in mice. Stem Cell Res. Ther. 6, 1–15. doi: 10.1186/scrt546
Hu, L., Wang, J., Zhou, X., Xiong, Z., Zhao, J., Yu, R., et al. (2016). Exosomes derived from human adipose mensenchymal stem cells accelerates cutaneous wound healing via optimizing the characteristics of fibroblasts. Sci. Rep. 6:32993. doi: 10.1038/srep32993
Hu, Y., Rao, S.-S., Wang, Z.-X., Cao, J., Tan, Y.-J., Luo, J., et al. (2018). Exosomes from human umbilical cord blood accelerate cutaneous wound healing through miR-21-3p-mediated promotion of angiogenesis and fibroblast function. Theranostics 8, 169–184. doi: 10.7150/thno.21234
Huang, H., Choi, J. K., Rao, W., Zhao, S., Agarwal, P., Zhao, G., et al. (2015). Alginate hydrogel microencapsulation inhibits devitrification and enables large-volume low-CPA cell vitrification. Adv. Funct. Mater. 25, 6839–6850. doi: 10.1002/adfm.201503047
Huleihel, L., Hussey, G. S., Naranjo, J. D., Zhang, L., Dziki, J. L., Turner, N. J., et al. (2016). Matrix-bound nanovesicles within ECM bioscaffolds. Sci. Adv. 2:e1600502. doi: 10.1126/sciadv.1600502
Ibrahim, A. G., Cheng, K., and Marban, E. (2014). Exosomes as critical agents of cardiac regeneration triggered by cell therapy. Stem Cell Rep. 2, 606–619. doi: 10.1016/j.stemcr.2014.04.006
Iglesias, D. M., El-Kares, R., Taranta, A., Bellomo, F., Emma, F., Besouw, M., et al. (2012). Stem cell microvesicles transfer cystinosin to human cystinotic cells and reduce cystine accumulation in vitro. PLoS One 7:e42840. doi: 10.1371/journal.pone.0042840
Jain, N. K., and Roy, I. (2009). Effect of trehalose on protein structure. Protein Sci. 18, 24–36. doi: 10.1002/pro.3
Jan, D. B., Van Blitterswijk, C., Thomsen, P., Hubbell, J., Cancedda, R., de Bruijn, J. D., Lindahl, A., et al. (2008). Tissue Engineering. Amsterdam: Elsevier, 369.
Jong, A. Y., Wu, C. H., Li, J., Sun, J., Fabbri, M., Wayne, A. S., et al. (2017). Large-scale isolation and cytotoxicity of extracellular vesicles derived from activated human natural killer cells. J. Extracell. Vesicles 6:1294368. doi: 10.1080/20013078.2017.1294368
Kalra, H., Drummen, G. P. C., and Mathivanan, S. (2016). Focus on extracellular vesicles: introducing the next small big thing. Int. J. Mol. Sci. 17:170. doi: 10.3390/ijms17020170
Kang, Y.-S., Jung, H.-J., Oh, J.-S., and Song, D.-Y. (2016). Use of PEGylated immunoliposomes to deliver dopamine across the blood-brain barrier in a rat model of parkinson’s disease. CNS Neurosci. Ther. 22, 817–823. doi: 10.1111/cns.12580
Katenz, E., Vondran, F. W., Schwartlander, R., Pless, G., Gong, X., Cheng, X., Neuhaus, P., et al. (2007). Cryopreservation of primary human hepatocytes: the benefit of trehalose as an additional cryoprotective agent. Liver Transplant. 13, 465–466. doi: 10.1002/lt
Katsuda, T., Tsuchiya, R., Kosaka, N., Yoshioka, Y., Takagaki, K., Oki, K., et al. (2013). Human adipose tissue-derived mesenchymal stem cells secrete functional neprilysin-bound exosomes. Sci. Rep. 3:1197. doi: 10.1038/srep01197
Kaviani, M., Ezzatabadipour, M., Nematollahi-Mahani, S. N., Salehinejad, P., Mohammadi, M., Kalantar, S. M., et al. (2014). Evaluation of gametogenic potential of vitrified human umbilical cord Wharton’s jelly-derived mesenchymal cells. Cytotherapy 16, 203–212. doi: 10.1016/j.jcyt.2013.10.015
Ke, X., Yang, D., Liang, J., Wang, X., Wu, S., Wang, X., et al. (2017). Human endothelial progenitor cell-derived exosomes increase proliferation and angiogenesis in cardiac fibroblasts by promoting the mesenchymal–endothelial transition and reducing high mobility group box 1 protein B1 expression. DNA Cell Biol. 36, 1018–1028. doi: 10.1089/dna.2017.3836
Keerthikumar, S., Chisanga, D., Ariyaratne, D., Al Saffar, H., Anand, S., Zhao, K., et al. (2016). ExoCarta: a web-based compendium of exosomal cargo. J. Mol. Biol. 428, 688–692. doi: 10.1016/j.jmb.2015.09.019
Kesenci, K., Motta, A., and Fambri, L. (2001). Poly(ε-caprolactone-co-D, L-lactide)/silk fibroin composite materials: preparation and characterization. J. Biomater. Sci. Polym. Ed. 12, 337–351. doi: 10.1016/S0939
Khairnar, S., Kini, R., Harwalker, M., Slaunkhe, K., and Chaudhari, S. (2013). A review on freeze drying process and pharmaceuticals. Int. J. Res. Pharm. Sci. 4, 76–94.
Khatua, A. K., Taylor, H. E., Hildreth, J. E. K., and Popik, W. (2009). Exosomes packaging APOBEC3G confer human immunodeficiency virus resistance to recipient cells. J. Virol. 83, 512–521. doi: 10.1128/JVI.01658-08
Kim, C.-G., Yong, H., Lee, G., and Cho, J. (2008). Effect of the polyvinylpyrrolidone concentration of cryoprotectant on mouse embryo development and production of pups: 7.5% of PVP is beneficial for in vitro and in vivo development of frozen-thawed mouse embryos. J. Reprod. Dev. 54, 250–253. doi: 10.1262/jrd.19185
Kordelas, L., Rebmann, V., Ludwig, A.-K., Radtke, S., Ruesing, J., Doeppner, T. R., et al. (2014). MSC-derived exosomes: a novel tool to treat therapy-refractory graft-versus-host disease. Leukemia 28, 970–973. doi: 10.1038/leu.2014.41
Kotobuki, N., Hirose, M., Machida, H., Katou, Y., Muraki, K., Takakura, Y., et al. (2005). Viability and osteogenic potential of cryopreserved human bone marrow-derived mesenchymal cells. Tissue Eng. 11, 663–673. doi: 10.1089/ten.2005.11.663
Kreke, M., Smith, R., Hanscome, P., Peck, K., and Ibrahim, A. (2016). Processes for producing stable exosome formulations. U.S. Patent No 14/958,804.
Kumar, V., Qin, J., Jiang, Y., Duncan, R. G., Brigham, B., Fishman, S., et al. (2014). Shielding of lipid nanoparticles for siRNA delivery: impact on physicochemical properties, cytokine induction, and efficacy. Mol. Ther. Nucleic Acids 3:e210. doi: 10.1038/mtna.2014.61
Kusuma, G. D., Carthew, J., Lim, R., and Frith, J. E. (2017). Effect of the microenvironment on mesenchymal stem cells paracrine signalling: opportunities to engineer the therapeutic effect. Stem Cells Dev. 26, 617–631. doi: 10.1089/scd.2016.0349
Laulagnier, K., Motta, C., Hamdi, S., Roy, S., Fauvelle, F., Pageaux, J.-F., et al. (2004). Mast cell- and dendritic cell-derived exosomes display a specific lipid composition and an unusual membrane organization. Biochem. J. 380, 161–171. doi: 10.1042/bj20031594
Lee, G. (2002). Spray-drying of proteins. Pharm. Biotechnol. 13, 135–158. doi: 10.1007/978-1-4615-0557-0_6
Lee, J. (2014). Trehalose Glycopolymers and Hydrogels for Enhancing Protein Stability. Doctoral dissertation, Los Angeles, CA, Regents of the University of California.
Lee, M., Ban, J. J., Im, W., and Kim, M. (2016). Influence of storage condition on exosome recovery. Biotechnol. Bioprocess Eng. 21, 299–304. doi: 10.1007/s12257-015-0781-x
Lener, T., Gioma, M., Aigner, L., Börger, V., Buzas, E., Camussi, G., et al. (2015). Applying extracellular vesicles based therapeutics in clinical trials - an ISEV position paper. J. Extracell. Vesicles 4, 1–31. doi: 10.3402/jev.v4.30087
Li, J., Zhang, Y., Liu, Y., Dai, X., Li, W., Cai, X., et al. (2013). Microvesicle-mediated transfer of microRNA-150 from monocytes to endothelial cells promotes angiogenesis. J. Biol. Chem. 288, 23586–23596. doi: 10.1074/jbc.M113.489302
Li, X., Jiang, C., and Zhao, J. (2016). Human endothelial progenitor cells-derived exosomes accelerate cutaneous wound healing in diabetic rats by promoting endothelial function. J. Diabetes Complications 30, 986–992. doi: 10.1016/j.jdiacomp.2016.05.009
Liang, X., Zhang, L., Wang, S., Han, Q., and Zhao, R. C. (2016). Exosomes secreted by mesenchymal stem cells promote endothelial cell angiogenesis by transferring miR-125a. J. Cell Sci. 129, 2182–2189. doi: 10.1242/jcs.170373
Lindemann, D., Werle, S. B., Steffens, D., Garcia-Godoy, F., Pranke, P., and Casagrande, L. (2014). Effects of cryopreservation on the characteristics of dental pulp stem cells of intact deciduous teeth. Arch. Oral Biol. 59, 970–976. doi: 10.1016/j.archoralbio.2014.04.008
Lindoso, R. S., Collino, F., Bruno, S., Araujo, D. S., Sant’Anna, J. F., Tetta, C., et al. (2014). Extracellular vesicles released from mesenchymal stromal cells modulate miRNA in renal tubular cells and inhibit ATP depletion injury. Stem Cells Dev. 23, 1809–1819. doi: 10.1089/scd.2013.0618
Liu, S., Liu, D., Chen, C., Hamamura, K., Moshaverinia, A., Yang, R., et al. (2015). MSC transplantation improves osteopenia via epigenetic regulation of notch signaling in lupus. Cell Metab. 22, 606–618. doi: 10.1016/j.cmet.2015.08.018
Lörincz,Á. M., Timár, C. I., Marosvári, K. A., Veres, D. S., Otrokocsi, L., Kittel, Á., et al. (2014). Effect of storage on physical and functional properties of extracellular vesicles derived from neutrophilic granulocytes. J. Extracell. Vesicles 3:25465. doi: 10.3402/jev.v3.25465
Lötvall, J., Hill, A. F., Hochberg, F., Buzás, E. I., Di Vizio, D., Gardiner, C., et al. (2014). Minimal experimental requirements for definition of extracellular vesicles and their functions: a position statement from the International Society for Extracellular Vesicles. J. Extracell. Vesicles 3:26913. doi: 10.3402/jev.v3.26913
Lou, G., Song, X., Yang, F., Wu, S., Wang, J., Chen, Z., et al. (2015). Exosomes derived from MIR-122-modified adipose tissue-derived MSCs increase chemosensitivity of hepatocellular carcinoma. J. Hematol. Oncol. 8, 1–11. doi: 10.1186/s13045-015-0220-7
Lv, L. L., Cao, Y., Liu, D., Xu, M., Liu, H., Tang, R. N., et al. (2013). Isolation and quantification of MicroRNAs from urinary exosomes/microvesicles for biomarker discovery. Int. J. Biol. Sci. 9, 1021–1031. doi: 10.7150/ijbs.6100
Lydic, T. A., Townsend, S., Adda, C. G., Collins, C., Mathivanan, S., and Reid, G. E. (2015). Rapid and comprehensive “shotgun” lipidome profiling of colorectal cancer cell derived exosomes. Methods 87, 83–95. doi: 10.1016/j.ymeth.2015.04.014
Manconi, M., Manca, M. L., Valenti, D., Escribano, E., Hillaireau, H., Fadda, A. M., et al. (2017). Chitosan and hyaluronan coated liposomes for pulmonary administration of curcumin. Int. J. Pharm. 525, 203–210. doi: 10.1016/j.ijpharm.2017.04.044
Maroto, R., Zhao, Y., Jamaluddin, M., Popov, V. L., Wang, H., Kalubowilage, M., et al. (2017). Effects of storage temperature on airway exosome integrity for diagnostic and functional analyses. J. Extracell. Vesicles 6:1359478. doi: 10.1080/20013078.2017.1359478
Marquez-Curtis, L. A., Janowska-Wieczorek, A., McGann, L. E., and Elliott, J. A. W. (2015). Mesenchymal stromal cells derived from various tissues: biological, clinical and cryopreservation aspects. Cryobiology 71, 181–197. doi: 10.1016/j.cryobiol.2015.07.003
Martinello, T., Bronzini, I., Maccatrozzo, L., Iacopetti, I., Sampaolesi, M., Mascarello, F., et al. (2010). Cryopreservation does not affect the stem characteristics of multipotent cells isolated from equine peripheral blood. Tissue Eng. Part C Methods 16, 771–781. doi: 10.1089/ten.tec.2009.0512
Martinetti, D., Colarossi, C., Buccheri, S., Denti, G., Memeo, L., and Vicari, L. (2017). Effect of trehalose on cryopreservation of pure peripheral blood stem cells. Biomed. Rep. 6, 314–318. doi: 10.3892/br.2017.859
Masters, K. (1972). Spray Drying : an Introduction to Principles, Operational Practice, and Applications /K. Masters. London: G. Godwin.
Mayourian, J., Ceholski, D. K., Gorski, P., Mathiyalagan, P., Murphy, J. F., Salazar, S. I., et al. (2018). Exosomal microRNA-21-5p mediates mesenchymal stem cell paracrine effects on human cardiac tissue contractility. Circ. Res. 122, 933–944. doi: 10.1161/CIRCRESAHA.118.312420
Monsel, A., Zhu, Y., Gennai, S., Hao, Q., Hu, S., Rouby, J.-J., et al. (2015). Therapeutic effects of human mesenchymal stem cell-derived microvesicles in severe pneumonia in mice. Am. J. Respir. Crit. Care Med. 192, 324–336. doi: 10.1164/rccm.201410-1765OC
Moon, J. H., Lee, J. R., Jee, B. C., Suh, C. S., Kim, S. H., Lim, H. J., et al. (2008). Successful vitrification of human amnion-derived mesenchymal stem cells. Hum. Reprod. 23, 1760–1770. doi: 10.1093/humrep/den202
Morse, M. A., Garst, J., Osada, T., Khan, S., Hobeika, A., Clay, T. M., et al. (2005). A phase I study of dexosome immunotherapy in patients with advanced non-small cell lung cancer. J. Transl. Med. 3, 1–8. doi: 10.1186/1479-5876-3-9
Motta, J. P. R., Paraguassú-Braga, F. H., Bouzas, L. F., and Porto, L. C. (2014). Evaluation of intracellular and extracellular trehalose as a cryoprotectant of stem cells obtained from umbilical cord blood. Cryobiology 68, 343–348. doi: 10.1016/j.cryobiol.2014.04.007
Naaldijk, Y., Staude, M., Fedorova, V., and Stolzing, A. (2012). Effect of different freezing rates during cryopreservation of rat mesenchymal stem cells using combinations of hydroxyethyl starch and dimethylsulfoxide. BMC Biotechnol. 12:49. doi: 10.1186/1472-6750-12-49
Nam, B. M., Kim, B. Y., Jo, Y. H., Lee, S., Nemeno, J. G., Yang, W., et al. (2014). Effect of cryopreservation and cell passage number on cell preparations destined for autologous chondrocyte transplantation. Transplant. Proc. 46, 1145–1149. doi: 10.1016/j.transproceed.2013.11.117
Narayanan, R., Huang, C., and Ravindran, S. (2016). Hijacking the cellular mail: exosome mediated differentiation of mesenchymal stem cells. Stem Cells Int. 2016:3808674. doi: 10.1155/2016/3808674
Naslund, T. I., Gehrmann, U., Qazi, K. R., Karlsson, M. C. I., and Gabrielsson, S. (2013). Dendritic cell-derived exosomes need to activate both T and B cells to induce antitumor immunity. J. Immunol. 190, 2712–2719. doi: 10.4049/jimmunol.1203082
Nidhi, K., Indrajeet, S., Khushboo, M., Gauri, K., and Sen, D. J. (2011). Hydrotropy: a promising tool for solubility enhancement: a review. Int. J. Drug Dev. Res. 3, 26–33. doi: 10.1002/jps
Nounou, M., and El-Khordagui, L. (2005). Influence of different sugar cryoprotectants on the stability and physico-chemical characteristics of freeze-dried 5-fluorouracil plurilamellar vesicles. DARU J. Pharm. Sci. 13, 133–142.
Ono, M., Kosaka, N., Tominaga, N., Yoshioka, Y., Takeshita, F., Takahashi, R. U., et al. (2014). Exosomes from bone marrow mesenchymal stem cells contain a microRNA that promotes dormancy in metastatic breast cancer cells. Sci. Signal. 7:ra63. doi: 10.1126/scisignal.2005231
Ophelders, D. R. M. G., Wolfs, T. G. A. M., Jellema, R. K., Zwanenburg, A., Andriessen, P., Delhaas, T., et al. (2016). Mesenchymal stromal cell-derived extracellular vesicles protect the fetal brain after hypoxia-ischemia. Stem Cells Transl. Med. 5, 754–763. doi: 10.5966/sctm.2015-0197
Pachler, K., Lener, T., Streif, D., Dunai, Z. A., Desgeorges, A., Feichtner, M., et al. (2017). A Good Manufacturing Practice–grade standard protocol for exclusively human mesenchymal stromal cell–derived extracellular vesicles. Cytotherapy 19, 458–472. doi: 10.1016/j.jcyt.2017.01.001
Pakravan, K., Babashah, S., Sadeghizadeh, M., Mowla, S. J., Mossahebi-Mohammadi, M., Ataei, F., et al. (2017). MicroRNA-100 shuttled by mesenchymal stem cell-derived exosomes suppresses in vitro angiogenesis through modulating the mTOR/HIF-1α/VEGF signaling axis in breast cancer cells. Cell. Oncol. 40, 457–470. doi: 10.1007/s13402-017-0335-7
Panyam, J., and Labhasetwar, V. (2012). Biodegradable nanoparticles for drug and gene delivery to cells and tissue. Adv. Drug Deliv. Rev. 64, 61–71. doi: 10.1016/j.addr.2012.09.023
Patel, A. R., Patel, D. A., and Chaudhry, S. V. (2011). Mucoadhesive buccal drug delivery system. Int. J. Pharm. Life Sci. 2, 848–856.
Pemberton, T. A., Still, B. R., Christensen, E. M., Singh, H., Srivastava, D., and Tanner, J. J. (2012). Proline: mother Natures cryoprotectant applied to protein crystallography. Acta Crystallogr. Sect. D Biol. Crystallogr. 68, 1010–1018. doi: 10.1107/S0907444912019580
Phinney, D. G., Di Giuseppe, M., Njah, J., Sala, E., Shiva, S., St Croix, C. M., et al. (2015). Mesenchymal stem cells use extracellular vesicles to outsource mitophagy and shuttle microRNAs. Nat. Commun. 6:8472. doi: 10.1038/ncomms9472
Pironti, G., Strachan, R. T., Abraham, D., Mon-Wei Yu, S., Chen, M., Chen, W., et al. (2015). Circulating exosomes induced by cardiac pressure overload contain functional angiotensin II type 1 receptors. Circulation 131, 2120–2130. doi: 10.1161/CIRCULATIONAHA.115.015687
Pusic, A. D., Pusic, K. M., Clayton, B. L. L., and Kraig, R. P. (2014). IFNgamma-stimulated dendritic cell exosomes as a potential therapeutic for remyelination. J. Neuroimmunol. 266, 12–23. doi: 10.1016/j.jneuroim.2013.10.014
Qin, Y., Wang, L., Gao, Z., Chen, G., and Zhang, C. (2016). Bone marrow stromal/stem cell-derived extracellular vesicles regulate osteoblast activity and differentiation in vitro and promote bone regeneration in vivo. Sci. Rep. 6:21961. doi: 10.1038/srep21961
Quintanar-Guerrero, D., Ganem-Quintanar, A., Allémann, E., Fessi, H., and Doelker, E. (1998). Influence of the stabilizer coating layer on the purification and freeze-drying of poly(D,L-lactic acid) nanoparticles prepared by an emulsion-diffusion technique. J. Microencapsul. 15, 107–119. doi: 10.3109/02652049809006840
Ragni, E., Banfi, F., Barilani, M., Cherubini, A., Parazzi, V., Larghi, P., et al. (2016). Extracellular vesicle-shuttled mRNA in mesenchymal stem cell communication. Stem Cells 35, 1093–1105. doi: 10.1002/stem.2557
Rana, S., and Zoller, M. (2013). “The functional importance of tetraspanins in exosomes,” in Emerging Concepts of Tumor Exosome-Mediated Cell-Cell Communication, ed. H.-G. Zhang (Berlin: Springer Science+Business Media), 69–106. doi: 10.1007/978-1-4614-3697-3
Rao, W., Huang, H., Wang, H., Zhao, S., Dumbleton, J., Zhao, G., et al. (2015). Nanoparticle-mediated intracellular delivery enables cryopreservation of human adipose-derived stem cells using trehalose as the sole cryoprotectant. ACS Appl. Mater. Interfaces 7, 5017–5028. doi: 10.1021/acsami.5b00655
Raposo, G., and Stoorvogel, W. (2013). Extracellular vesicles: exosomes, microvesicles, and friends. J. Cell Biol. 200, 373–383. doi: 10.1083/jcb.201211138
Ray, S. S., Pramanik, K., Sarangi, S. K., and Jain, N. (2016). Serum-free non-toxic freezing solution for cryopreservation of human adipose tissue-derived mesenchymal stem cells. Biotechnol. Lett. 38, 1397–1404. doi: 10.1007/s10529-016-2111-6
Roy, D., Guillon, X., Lescure, F., Couvreur, P., Bru, N., and Breton, P. (1997). On shelf stability of freeze-dried: poly(methylidene malonate 2.1.2) nanoparticles. Int. J. Pharm. 148, 165–175. doi: 10.1016/S0378-5173(96)04842-9
Roy, S., Arora, S., Kumari, P., and Ta, M. (2014). A simple and serum-free protocol for cryopreservation of human umbilical cord as source of Wharton’s jelly mesenchymal stem cells. Cryobiology 68, 467–472. doi: 10.1016/j.cryobiol.2014.03.010
Rubenfeld, G. D., Caldwell, E., Peabody, E., Weaver, J., Martin, D. P., Neff, M., et al. (2005). Incidence and outcomes of acute lung injury. N. Engl. J. Med. 353, 1685–1693. doi: 10.1056/NEJMoa050333
Rust, P. A., Tingerides, C., Cannon, S. R., Briggs, T. W. R., and Blunn, G. W. (2006). Characterisation of cryopreserved cells freshly isolated from human bone marrow. Cryo Lett. 27, 17–28.
Salama, R. O., Traini, D., Chan, H.-K., Sung, A., Ammit, A. J., and Young, P. M. (2009). Preparation and evaluation of controlled release microparticles for respiratory protein therapy. J. Pharm. Sci. 98, 2709–2717. doi: 10.1002/jps.21653
Sameti, M., Bohr, G., Ravi Kumar, M. N. V., Kneuer, C., Bakowsky, U., Nacken, M., et al. (2003). Stabilisation by freeze-drying of cationically modified silica nanoparticles for gene delivery. Int. J. Pharm. 266, 51–60. doi: 10.1016/S0378-5173(03)00380-6
Schaffazick, S. R., Pohlmann, A. R., Dalla-Costa, T., and Guterres, S. S. (2003). Freeze-drying polymeric colloidal suspensions: nanocapsules, nanospheres and nanodispersion. A comparative study. Eur. J. Pharm. Biopharm. 56, 501–505. doi: 10.1016/S0939-6411(03)00139-5
Schwarz, C., and Mehnert, W. (1997). Freeze-drying of drug-free and drug-loaded solid lipid nanoparticles ( SLN ). Int. J. Pharm. 157, 171–179. doi: 10.1016/S0378-5173(97)00222-6
Sehgal, S., and Rogers, J. A. (1995). Polymer-coated liposomes: improved liposome stability and release of cytosine arabinoside (Ara-C). J. Microencapsul. 12, 37–47. doi: 10.3109/02652049509051125
Shapiro, I. M., Landis, W. J., and Risbud, M. V. (2015). Matrix vesicles: are they anchored exosomes? Bone 79, 29–36. doi: 10.1016/j.bone.2015.05.013
Sheldon, H., Heikamp, E., Turley, H., Dragovic, R., Thomas, P., Oon, C. E., et al. (2010). New mechanism for Notch signaling to endothelium at a distance by delta-like 4 incorporation into exosomes. Blood 116, 2385–2394. doi: 10.1182/blood-2009-08-239228
Shen, J. L., Huang, Y. Z., Xu, S. X., Zheng, P. H., Yin, W. J., Cen, J., et al. (2012). Effectiveness of human mesenchymal stem cells derived from bone marrow cryopreserved for 23-25 years. Cryobiology 64, 167–175. doi: 10.1016/j.cryobiol.2012.01.004
Shi, Q., Qian, Z., Liu, D., Sun, J., Wang, X., Liu, H., et al. (2017). GMSC-derived exosomes combined with a chitosan/silk hydrogel sponge accelerates wound healing in a diabetic rat skin defect model. Front. Physiol. 8:904. doi: 10.3389/fphys.2017.00904
Shigemoto-Kuroda, T., Oh, J. Y., Kim, D.-K., Jeong, H. J., Park, S. Y., Lee, H. J., et al. (2017). MSC-derived extracellular vesicles attenuate immune responses in two autoimmune murine models: type 1 diabetes and uveoretinitis. Stem Cell Rep. 8, 1214–1225. doi: 10.1016/j.stemcr.2017.04.008
Shivakumar, S. B., Bharti, D., Jang, S. J., Hwang, S. C., Park, J. K., Shin, J. K., et al. (2015). Cryopreservation of human wharton’s jelly-derived mesenchymal stem cells following controlled rate freezing protocol using different cryoprotectants; a comparative study. Int. J. Stem Cells 8, 155–169. doi: 10.15283/ijsc.2015.8.2.155
Skotland, T., Sandvig, K., and Llorente, A. (2017). Lipids in exosomes: current knowledge and the way forward. Prog. Lipid Res. 66, 30–41. doi: 10.1016/j.plipres.2017.03.001
Sokolova, V., Ludwig, A., Hornung, S., Rotan, O., Horn, P. A., Epple, M., et al. (2011). Colloids and surfaces B : biointerfaces Characterisation of exosomes derived from human cells by nanoparticle tracking analysis and scanning electron microscopy. Colloids Surf. B Biointerfaces 87, 146–150. doi: 10.1016/j.colsurfb.2011.05.013
Song, Y., Dou, H., Li, X., Zhao, X., Li, Y., Liu, D., et al. (2017). Exosomal miR-146a contributes to the enhanced therapeutic efficacy of IL-1β-primed mesenchymal stem cells against sepsis. Stem Cells 35, 1208–1221. doi: 10.1002/stem.2564
Stamer, W. D., Hoffman, E. A., Luther, J. M., Hachey, D. L., and Schey, K. L. (2011). Protein profile of exosomes from trabecular meshwork cells. J. Proteomics 74, 796–804. doi: 10.1016/j.jprot.2011.02.024
Stark, B., Pabst, G., and Prassl, R. (2010). Long-term stability of sterically stabilized liposomes by freezing and freeze-drying: effects of cryoprotectants on structure. Eur. J. Pharm. Sci. 41, 546–555. doi: 10.1016/j.ejps.2010.08.010
Stolzenburg, L. R., and Harris, A. (2017). Microvesicle-mediated delivery of miR-1343: impact on markers of fibrosis. Cell Tissue Res. 371, 325–338. doi: 10.1007/s00441-017-2697-6
Storm, G., Belliot, S. O., Daemen, T., and Lasic, D. D. (1995). Surface modification of nanoparticles to oppose uptake by the mononuclear phagocyte system. Adv. Drug Deliv. Rev. 17, 31–48. doi: 10.1016/0169-409X(95)00039-A
Subedi, R. K., Kang, K. W., and Choi, H. K. (2009). Preparation and characterization of solid lipid nanoparticles loaded with doxorubicin. Eur. J. Pharm. Sci. 37, 508–513. doi: 10.1016/j.ejps.2009.04.008
Subra, C., Laulagnier, K., Perret, B., and Record, M. (2007). Exosome lipidomics unravels lipid sorting at the level of multivesicular bodies. Biochimie 89, 205–212. doi: 10.1016/j.biochi.2006.10.014
Sudres, M., Maurer, M., Robinet, M., Bismuth, J., Truffault, F., Girard, D., et al. (2017). Preconditioned mesenchymal stem cells treat myasthenia gravis in a humanized preclinical model. JCI Insight 2:e89665. doi: 10.1172/jci.insight.89665
Sun, H., Glasmacher, B., and Hofmann, N. (2012). Compatible solutes improve cryopreservation of human endothelial cells. Cryo Lett. 33, 485–493.
Takeuchi, H., Kojima, H., Yamamoto, H., and Kawashima, Y. (2000). Polymer coating of liposomes with a modified polyvinyl alcohol and their systemic circulation and RES uptake in rats. J. Control. Release 68, 195–205. doi: 10.1016/S0168-3659(00)00260-1
Tan, C. Y., Lai, R. C., Wong, W., Dan, Y. Y., Lim, S. -K., and Ho, H. K. (2014). Mesenchymal stem cell-derived exosomes promote hepatic regeneration in drug-induced liver injury models. Stem Cell Res. Ther. 5:76. doi: 10.1186/scrt465
Tan, J. L., Lau, S. N., Leaw, B., Nguyen, H. P. T., Salamonsen, L. A., Saad, M. I., et al. (2018). Amnion epithelial cell-derived exosomes restrict lung injury and enhance endogenous lung repair. Stem Cells Transl. Med. 7, 180–196. doi: 10.1002/sctm.17-0185
Tanaka, K., Kawamura, M., Otake, K., Toiyama, Y., Okugawa, Y., Inoue, Y., et al. (2014). Trehalose does not affect the functions of human neutrophils in vitro. Surg. Today 44, 332–339. doi: 10.1007/s00595-013-0625-2
Thery, C., Boussac, M., Veron, P., Ricciardi-Castagnoli, P., Raposo, G., Garin, J., et al. (2001). Proteomic analysis of dendritic cell-derived exosomes: a secreted subcellular compartment distinct from apoptotic vesicles. J. Immunol. 166, 7309–7318. doi: 10.4049/jimmunol.166.12.7309
Thery, C., Regnault, A., Garin, J., Wolfers, J., Zitvogel, L., Ricciardi-Castagnoli, P., et al. (1999). Molecular characterization of dendritic cell-derived exosomes. Selective accumulation of the heat shock protein hsc73. J. Cell Biol. 147, 599–610. doi: 10.1083/jcb.147.3.599
Thirumala, S., Gimble, J. M., and Devireddy, R. V. (2010). Evaluation of methylcellulose and dimethyl sulfoxide as the cryoprotectants in a serum-free freezing media for cryopreservation of adipose-derived adult stem cells. Stem Cells Dev. 19, 513–522. doi: 10.1089/scd.2009.0173
Tian, Y., Li, S., Song, J., Ji, T., Zhu, M., Anderson, G. J., et al. (2014). A doxorubicin delivery platform using engineered natural membrane vesicle exosomes for targeted tumor therapy. Biomaterials 35, 2383–2390. doi: 10.1016/j.biomaterials.2013.11.083
Tkach, M., and Théry, C. (2016). Communication by extracellular vesicles: where we are and where we need to go. Cell 164, 1226–1232. doi: 10.1016/j.cell.2016.01.043
Tomasoni, S., Longaretti, L., Rota, C., Morigi, M., Conti, S., Gotti, E., et al. (2013). Transfer of growth factor receptor mRNA via exosomes unravels the regenerative effect of mesenchymal stem cells. Stem Cells Dev. 22, 772–780. doi: 10.1089/scd.2012.0266
Trad, F. S., Toner, M., and Biggers, J. D. (1999). Effects of cryoprotectants and ice-seeding temperature on intracellular freezing and survival of human oocytes. Hum. Reprod. 14, 1569–1577. doi: 10.1093/humrep/14.6.1569
Valadi, H., Ekström, K., Bossios, A., Sjöstrand, M., Lee, J. J., and Lötvall, J. O. (2007). Exosome-mediated transfer of mRNAs and microRNAs is a novel mechanism of genetic exchange between cells. Nat. Cell Biol. 9, 654–659. doi: 10.1038/ncb1596
Vallabhaneni, K. C., Penfornis, P., Dhule, S., Guillonneau, F., Adams, K. V., Yuan Mo, Y., et al. (2015). Extracellular vesicles from bone marrow mesenchymal stem/stromal cells transport tumor regulatory microRNA, proteins, and metabolites. Oncotarget 6, 4953–4967. doi: 10.18632/oncotarget.3211
Van Backstal, P.-J., De Beer, T., and Corver, J. (2017). A continuous and controlled pharmaceutical freeze-drying technology for unit doses. Eur. Pharm. Rev. 22, 51–53. doi: 10.1016/j.ijpharm.2018.02.003
van Niel, G., D’Angelo, G., and Raposo, G. (2018). Shedding light on the cell biology of extracellular vesicles. Nat. Rev. Mol. Cell Biol. 19, 213–228. doi: 10.1038/nrm.2017.125
van Niel, G., Porto-Carreiro, I., Simoes, S., and Raposo, G. (2006). Exosomes: a common pathway for a specialized function. J. Biochem. 140, 13–21. doi: 10.1093/jb/mvj128
Viaud, S., Terme, M., Flament, C., Taieb, J., André, F., Novault, S., et al. (2009). Dendritic cell-derived exosomes promote natural killer cell activation and proliferation: a role for NKG2D ligands and IL-15Rα. PLoS One 4:e4942. doi: 10.1371/journal.pone.0004942
Vrijsen, K. R., Maring, J. A., Chamuleau, S. A. J., Verhage, V., Mol, E. A., Deddens, J. C., et al. (2016). Exosomes from cardiomyocyte progenitor cells and mesenchymal stem cells stimulate angiogenesis via EMMPRIN. Adv. Healthc. Mater. 5, 2555–2565. doi: 10.1002/adhm.201600308
Wang, B., Yao, K., Huuskes, B. M., Shen, H. -H., Zhuang, J., Godson, C., et al. (2016). Mesenchymal stem cells deliver exogenous MicroRNA-let7c via exosomes to attenuate renal fibrosis. Mol. Ther. 24, 1290–1301. doi: 10.1038/mt.2016.90
Wang, H.-Y., Lun, Z.-R., and Lu, S.-S. (2011). Cryopreservation of umbilical cord blood-derived mesenchymal stem cells without dimethyl sulfoxide. Cryo Lett. 32, 81–88.
Wang, Y., Zhang, L., Li, Y., Chen, L., Wang, X., Guo, W., et al. (2015). Exosomes/microvesicles from induced pluripotent stem cells deliver cardioprotective miRNAs and prevent cardiomyocyte apoptosis in the ischemic myocardium. Int. J. Cardiol. 192, 61–69. doi: 10.1016/j.ijcard.2015.05.020
Willison, J. H. M., and Rowe, A. J. (1980). Replica, Shadowing and Freeze-Etching Techniques. Amsterdam: Elsevier.
Woods, E. J., Perry, B. C., Hockema, J. J., Larson, L., Zhou, D., and Goebel, W. S. (2010). Optimized cryopreservation method for human dental pulp-derived stem cells and their tissues of origin for banking and clinical use. Cryobiology 59, 150–157. doi: 10.1016/j.cryobiol.2009.06.005
Wu, Y., Deng, W., Babb, M., Cancer, R., Virginia, W., and Biology, C. (2015a). Exosomes: improved methods to characterize their morphology, RNA content, and surface protein biomarkers. Analyst 140, 6631–6642. doi: 10.1039/c5an00688k
Wu, Y., Deng, W., and Klinke, D. J. (2015b). Exosomes: improved methods to characterize their morphology, RNA content, and surface protein biomarkers. Analyst 140, 6631–6642. doi: 10.1039/c5an00688k
Xin, H., Li, Y., Buller, B., Katakowski, M., Zhang, Y., Wang, X., et al. (2012). Exosome-mediated transfer of miR-133b from multipotent mesenchymal stromal cells to neural cells contributes to neurite outgrowth. Stem Cells 30, 1556–1564. doi: 10.1002/stem.1129.Exosome-Mediated
Xin, H., Li, Y., Liu, Z., Wang, X., Shang, X., Cui, Y., et al. (2013). MiR-133b promotes neural plasticity and functional recovery after treatment of stroke with multipotent mesenchymal stromal cells in rats via transfer of exosome-enriched extracellular particles. Stem Cells 31, 2737–2746. doi: 10.1002/stem.1409
Xu, X., Liu, Y., Cui, Z., Wei, Y., and Zhang, L. (2012). Effects of osmotic and cold shock on adherent human mesenchymal stem cells during cryopreservation. J. Biotechnol. 162, 224–231. doi: 10.1016/j.jbiotec.2012.09.004
Yang, T., Martin, P., Fogarty, B., Brown, A., Schurman, K., Phipps, R., et al. (2015). Exosome delivered anticancer drugs across the blood-brain barrier for brain cancer therapy in Danio rerio. Pharm. Res. 32, 2003–2014. doi: 10.1007/s11095-014-1593-y
Yu, B., Kim, H. W., Gong, M., Wang, J., Millard, R. W., Wang, Y., et al. (2015). Exosomes secreted from GATA-4 overexpressing mesenchymal stem cells serve as a reservoir of anti-apoptotic microRNAs for cardioprotection. Int. J. Cardiol. 182, 349–360. doi: 10.1016/j.ijcard.2014.12.043
Zhang, B., Wang, M., Gong, A., Zhang, X., Wu, X., Zhu, Y., et al. (2015a). HucMSc-exosome mediated-Wnt4 signaling is required for cutaneous wound healing. Stem Cells 33, 2158–2168. doi: 10.1002/stem.1771
Zhang, B., Wu, X., Zhang, X., Sun, Y., Yan, Y., Shi, H., et al. (2015b). Human umbilical cord mesenchymal stem cell exosomes enhance angiogenesis through the Wnt4/beta-catenin pathway. Stem Cells Transl. Med. 4, 513–522. doi: 10.5966/sctm.2014-0267
Zhang, B., Yin, Y., Lai, R. C., and Lim, S. K. (2014a). Immunotherapeutic potential of extracellular vesicles. Front. Immunol. 5:518. doi: 10.3389/fimmu.2014.00518
Zhang, B., Yin, Y., Lai, R. C., Tan, S. S., Choo, A. B. H., and Lim, S. K. (2014b). Mesenchymal stem cells secrete immunologically active exosomes. Stem Cells Dev. 23, 1233–1244. doi: 10.1089/scd.2013.0479
Zhang, G., Zou, X., Miao, S., Chen, J., Du, T., Zhong, L., et al. (2014c). The anti-oxidative role of micro-vesicles derived from human Wharton-Jelly mesenchymal stromal cells through NOX2/gp91(phox) suppression in alleviating renal ischemia-reperfusion injury in rats. PLoS One 9:e92129. doi: 10.1371/journal.pone.0092129
Zhang, J., Liu, X., Li, H., Chen, C., Hu, B., Niu, X., et al. (2016). Exosomes/tricalcium phosphate combination scaffolds can enhance bone regeneration by activating the PI3K/Akt signaling pathway. Stem Cell Res. Ther. 7:136. doi: 10.1186/s13287-016-0391-3
Zhao, B., Zhang, Y., Han, S., Zhang, W., Zhou, Q., Guan, H., et al. (2017). Exosomes derived from human amniotic epithelial cells accelerate wound healing and inhibit scar formation. J. Mol. Histol. 48, 121–132. doi: 10.1007/s10735-017-9711-x
Zhu, Y. G., Feng, X. M., Abbott, J., Fang, X. H., Hao, Q., Monsel, A., et al. (2014). Human mesenchymal stem cell microvesicles for treatment of Escherichia coli endotoxin-induced acute lung injury in mice. Stem. Cells 32, 116–125. doi: 10.1002/stem.1504.
Keywords: nanomedicine, exosomes, extracellular vesicles, biomaterials, cryopreservation, regenerative medicine, biologics
Citation: Kusuma GD, Barabadi M, Tan JL, Morton DAV, Frith JE and Lim R (2018) To Protect and to Preserve: Novel Preservation Strategies for Extracellular Vesicles. Front. Pharmacol. 9:1199. doi: 10.3389/fphar.2018.01199
Received: 03 August 2018; Accepted: 28 September 2018;
Published: 29 October 2018.
Edited by:
Lei Xi, Virginia Commonwealth University, United StatesReviewed by:
Sudheer Kumar Ravuri, Steadman Philippon Research Institute, United StatesCopyright © 2018 Kusuma, Barabadi, Tan, Morton, Frith and Lim. This is an open-access article distributed under the terms of the Creative Commons Attribution License (CC BY). The use, distribution or reproduction in other forums is permitted, provided the original author(s) and the copyright owner(s) are credited and that the original publication in this journal is cited, in accordance with accepted academic practice. No use, distribution or reproduction is permitted which does not comply with these terms.
*Correspondence: Rebecca Lim, UmViZWNjYS5MaW1AaHVkc29uLm9yZy5hdQ==
†These authors have contributed equally to this work
Disclaimer: All claims expressed in this article are solely those of the authors and do not necessarily represent those of their affiliated organizations, or those of the publisher, the editors and the reviewers. Any product that may be evaluated in this article or claim that may be made by its manufacturer is not guaranteed or endorsed by the publisher.
Research integrity at Frontiers
Learn more about the work of our research integrity team to safeguard the quality of each article we publish.