- 1Department of Neurology, Tainan Sin-Lau Hospital, Tainan, Taiwan
- 2Department of Pediatrics, Chi-Mei Medical Center, Tainan, Taiwan
- 3Department of Pediatric Neurology, Chiayi Christian Hospital, Chiayi, Taiwan
- 4Department of Neurology, College of Medicine, National Cheng Kung University, Tainan, Taiwan
- 5Department of Physiology, College of Medicine, National Cheng Kung University, Tainan, Taiwan
Pioglitazone (PIO), a thiazolidinedone, was reported to stimulate peroxisome proliferator-activated receptor-γ (PPAR-γ) with anti-inflammatory, anti-proliferative, anti-diabetic, and antidepressive activities. However, whether this compound exerts any perturbations on Ca2+-activated K+ and M-type K+ currents in central neurons remains largely unresolved. In this study, we investigated the effects of PIO on these potassium currents in hippocampal neurons (mHippoE-14). In whole-cell current recordings, the presence of PIO (10 μM) increased the amplitude of Ca2+-activated K+ current [IK(Ca)] in mHippoE-14 cells. PIO-induced stimulation of IK(Ca) observed in these cells was reversed by subsequent addition of paxilline, yet not by TRAM-39 or apamin. In inside-out current recordings, PIO applied to the bath concentration-dependently increased the activity of large-conductance Ca2+-activated K+ (BKCa) channels with an EC50 value of 7.6 μM. Its activation of BKCa channels in mHippoE-14 cells was voltage-dependent and accompanied by both a lengthening in mean open time and a shortening in slow component of mean closed time. The activation curve of BKCa channels after addition of PIO was shifted to less depolarized potential without any change in the gating charge. PIO also suppressed the amplitude of M-type K+ currents inherently in mHippoE-14 neurons. Taken together, in addition to its agonistic action on PPAR-γ, PIO-induced perturbation of these potassium channels may be responsible for its widely pharmacological actions on hippocampal neurons.
Introduction
The family of PPARs is a type II nucleus receptor. They have been recognized to play essential roles in the regulation of cellular differentiation, development, and metabolism. There are three distinct isotypes in PPARs including α, γ, and β/δ (Kersten et al., 2000). Among them, PPAR-γγ plays an important role in fatty acid storage and glucose metabolism, expressing in endothelium, vascular muscle, and cells of the innate immune system (Tontonoz and Spiegelman, 2008). Two isoforms of PPAR-γ are detected in the human and in the mouse, i.e., PPAR-γ1 and PPAR-γ2. The two PPAR-γ isoforms show a distinct expression pattern; that is, PPAR-γ1 is abundantly expressed, while PPAR-γ2 was reported to be restricted to white and brown adipose tissue under physiological conditions.
PPAR-γ heterodimerizes with retinoid X receptor (RXR) and binds to specific PPAR response element. PPAR-γ is activated by natural and synthetic ligand. Natural ligands include fatty acids and prostanoids (e.g., 15-deoxy-δ12,14-prostaglandin J2). TZDs are synthetic PPAR-γ ligands, including rosiglitazone and pioglitazone. They are also type II diabetes drugs approved by U.S. Food and Drug Administration. There is growing evidence that the agonist of PPAR-γ might have neuroprotective potential in CNS diseases such as stroke, Parkinson’s disease and depression (Kapadia et al., 2008; Schlachetzki and Winkler, 2015; Li et al., 2018).
There were the experimental results showing the electrophysiological effects of troglitazone and PIO in African green monkey kidney cells. They found that ATP-sensitive K+ (KATP) channels, which closed when intracellular [ATP]/[ADP] ratio elevated and membrane depolarization, were inhibited by troglitazone but not by PIO (Sunaga et al., 1999). In a previous report, troglitazone has been demonstrated to suppress KATP activity in ventromedial hypothalamus neurons (Asano et al., 1999). However, it was noted that FDA-approved TZDs only included rosiglitazone and PIO but not troglitazone. Until now, investigations on TZDs’ electrophysiological effect in the brain is inconclusive.
The mHippoE-14 hippocampal cell line is known to possess the characteristics of embryonic hippocampal neurons and enables accurate in vitro assays for use in the discovery, development, and validation of new therapeutics targeted to central nervous system diseases and disorders, including obesity, stress, reproduction, and metabolic disorders (Gingerich et al., 2010; Lazniewska et al., 2013; Evans et al., 2016). Long-term treatment with PIO has been previously reported to influence hippocampal function (Blalock et al., 2010). However, to our knowledge, no studies concerning the biophysical and pharmacological properties of membrane ionic currents in these mHippoE-14 hippocampal cells have been thoroughly studied, although several previous reports have shown some effects of PIO on K+ and Ca2+ currents in muscle cells and Ca2+ currents in cultured hippocampal neurons (Zhang et al., 1994; Pancani et al., 2009; Gu et al., 2014).
The large-conductance Ca2+-activated K+ (BKCa) channels (maxi-K channels, KCa1.1, KCNMA1, Slo1) have the largest single-channel conductance of all K+ selective channels and can be synergistically activated by membrane depolarization and elevation of intracellular Ca2+. They have been described to be functionally expressed in hippocampal neurons (Li et al., 2002; Huang et al., 2007; Wu et al., 2017). Another interesting paper has previously shown the function of Ca2+-activated K+ channels in hippocampal pyramidal neurons (Storm, 1987). Specifically, a previous report has demonstrated the ability of PPAR-γ inhibitor to either increase or decrease the activity of BKCa channels (Wu et al., 2000). On the other hand, the KCNQ2, KCNQ3, and KCNQ5 genes are known to encode the core subunits of KV7.2, KV7.3, and KV7.5 channels, respectively. The increased activity of these K+ channels has been demonstrated to generate the M-type K+ current [IK(M)] which is a slowly activating and deactivating current suppressed by stimulation of muscarinic receptors (Brown and Yu, 2000; Hsu et al., 2014). Most of IK(M) in neurons are made by heterologously expressed KV7.2/7.3 channels. Targeting IK(M) is growingly recognized to be valuable as an adjunctive regimen for the treatment of many neurological disorders. However, whether PIO exerts any actions on these types of ionic currents remains largely unexplored.
In this study, we attempted to explore the effect of PIO on potassium currents, mainly Ca2+-activated K+ current [IK(Ca)], and M-type K+ current [IK(M)] observed in mHippoE-14 hippocampal neurons. Our findings revealed that PIO is able to stimulate the activity of BKCa channels and to suppress IK(M) with different potency. Apart from its agonism on PPAR-γ activation, these actions on potassium currents may significantly have an impact on electrical behaviors of hippocampal neurons.
Materials and Methods
Pioglitazone (PIO, C19H2ON2O3S, 5-[[4-[2-(5-ethylpyridin-2-yl)ethoxy]phenyl]methyl]-1,3-thiazolidine-2,4-dione) was obtained from Takeda Pharmaceutical, Co., Ltd. (Tokyo, Japan), 4-aminopyridine, flupirtine maleate, linopirdine, paxilline, tetraethylammonium chloride and tobutamide were from Sigma-Aldrich (St. Louis, MO, United States), TRAM-39 was from Tocris (Bristol, United Kingdom) and apamin was from Alomone Labs (Jerusalem, Israel). Chlorotoxin was kindly provided by Dr. Woei-Jer Chuang (Department of Biochemistry, National Cheng Kung University Medical College, Tainan, Taiwan). Pioglitazone was dissolved in dimethyl sulfoxide (less than 0.1%) and made immediately prior to experiments. Unless stated otherwise, tissue culture media, fetal bovine serum, L-glutamine, penicillin–streptomycin, fungizone, and trypsin/EDTA were obtained from Invitrogen, Corp. (Carlsbad, CA, United States). All other chemicals including CsCl and CsOH were of the best available quality, mostly at analytical grades. The twice-distilled water was de-ionized through a Milli-Q water purification system (APS Water Services, Inc., Van Nuys, CA, United States).
The composition of bath solution (i.e., normal Tyrode’s solution) was as follows (in mM): NaCl 136.5, KCl 5.4, CaCl2 1.8, MgCl2 0.53, glucose 5.5, and HEPES-NaOH buffer 5 (pH 7.4). To record IK(Ca) or IK(M), the recording pipettes were backfilled with a solution consisting of K-aspartate 130, KCl 20, KH2PO4 1, MgCl2 1, Na2ATP 3, Na2GTP 0.1, EGTA 0.1, and HEPES-KOH buffer 5 (pH 7.2). For the recordings of BKCa-channel activity or whole-cell IK(M), high K+-bathing solution was composed of the following (in mM): KCl 145, MgCl2 0.53, and HEPES-KOH 5 (pH 7.4), and the pipette was filled with a solution (in mM): KCl 145, MgCl2 2, and HEPES-KOH 5 (pH 7.2). The value of p/4Ca2+ concentration was calculated assuming a dissociation constant for EGTA and Ca2+ (at pH 7.2) of 0.1 μM1. For example, to provide 0.1 μM Ca2+ in the bath solution, 0.5 mM CaCl2 and 1 mM EGTA were added.
Cell Preparation
Embryonic mouse hippocampal cell line (mHippoE-14; CLU198) was obtained from Cedarlane CELLutions Biosystems, Inc. (Burlington, ON, Canada) (Gingerich et al., 2010). Cells were grown as a monolayer culture in 50-ml plastic culture flasks in a humidifier environment of 5% CO2/95% air at 37°C. They were maintained at a density of 106/ml in 5 ml of Dulbecco’s modified Eagle’s medium supplemented with 10% heat-inactivated fetal bovine serum (v/v) and 2 mM L-glutamine. The medium was refreshed every 2 days to maintain a healthy cell population. Under our experimental conditions, the presence of neuritis and varicosities during cell preparations was often observed. The patch clamp experiments were performed 5 or 6 days after cells were subcultured (60–80% confluence).
Electrophysiological Measurements
Mouse hippocampal neurons (mHippoE-14) were harvested with 1% trypsin/EDTA solution prior to each experiment and a portion of detached cells was thereafter transferred to a recording chamber mounted on the stage of a CKX-41 inverted fluorescent microscope (Olympus, Tokyo, Japan) coupled to a digital video system (DCR-TRV30; Sony, Japan) with a magnification of up to 1500×. They were immersed at room temperature (20–25°C) in normal Tyrode’s solution containing 1.8 mM CaCl2. Patch pipettes were made from Kimax-51 glass capillaries (#34500; Kimble, Vineland, NJ, United States) using a PP-830 (Narishige, Tokyo, Japan) or P-97 micropipette puller (Sutter, Novato, CA, United States), and their tips were then fire-polished with an MF-83 microforge (Narishige). The recording pipettes used had a resistance of 3–5 MΩ as they were immersed in different solutions described above. Patch-clamp recordings were made in whole-cell, cell-attached, or inside-out configuration by means of an RK-400 (Bio-Logic, Claix, France) or Axopatch 200B patch amplifier (Molecular Devices, Sunnyvale, CA, United States) (Huang et al., 2018; Lai et al., 2018). Liquid junctional potential was commonly nulled immediately before establishment of the seal.
Data Recordings
The signals consisting of voltage and current tracings were stored online in an ASUSPRO-BU401LG computer (ASUS, Taipei, Taiwan) at 10 kHz connected through a Digidata 1440 digitizer (Molecular Devices) which was driven by pCLAMP 10.2 software (Molecular Devices). Current signals were low-pass filtered at 3 kHz. The data achieved during each experiment were analyzed off-line using different kinds of analytical tools including LabChart 7.0 program (AD Instruments; Gerin, Tainan, Taiwan), OriginPro 2016 (OriginLab, Northampton, MA, United States) and custom-made macro procedures built under Microsoft Excel 2013 (Redmond, WA, United States). Through digital-to-analog conversion, the gapped voltage-step protocols with either rectangular or ramp pulses created from pCLAMP 10.2 were employed to evaluate the steady-state activation or inactivation curve for different types of ion currents.
Single-Channel Analyses
Single amplitudes of BKCa-channel currents were analyzed with pCLAMP 10.2 (Molecular Devices). Multi-gaussian adjustments of the amplitude distributions among channels or mean-variance histograms were implemented to determine single-channel events accurately. The number of functional active channels seen in each patch was taken as the maximum number of channels simultaneously open under conditions of maximum open probability. The channel open probabilities were evaluated using an iterative process to minimize χ2 values, as calculated with an adequately large number of independent observations. Single-channel conductance of the channel taken in the absence or presence of PIO was determined by linear regression using mean values of current amplitudes collected at different levels of holding potentials. The lifetime distributions of open or closed states were satisfactorily fitted with single or two exponentials, and the distributions are illustrated in logarithmically scaled bin width. The mean-variance analysis obtained with or without addition of PIO was also made for determination of single-channel opening events (Wu et al., 2003).
To calculate percentage increase of PIO on the probability of BKCa-channel openings, inside-out current recordings were made, bath medium contained 0.1 μM Ca2+, and the potential was set at +60 mV. BKCa-channel activity in the presence of 100 μM PIO was taken to be 100% and those in different concentration (0.1–30 μM) of PIO were then compared. The PIO concentration needed to increase 50% of channel open probability was determined using the following multi-parameter logistic equation:
where [C] is the PIO concentration, EC50 and nH are the concentration required for a 50% increase of channel activity and the Hill coefficient, respectively; and Emax is the PIO-induced maximal increase in the probability of BKCa channels that would be open.
To determine effects of PIO on the activation curve of BKCa channels, the ramp pulse from 0 to +80 mV with a duration of 1 s were repetitively delivered from pCLAMP 10.2 program through digital-to-analog conversion. The activation curves evoked in response to ramp pulses were calculated by averaging current traces in responses to 20 voltage ramps and dividing each point of mean current by single-channel amplitude at each potential when the leakage component was corrected. The linear passive leak currents were subtracted using a P/4 regimen. The activation curve of BKCa channels (i.e., the relative open probability versus membrane potential) taken with or without addition of PIO (10 μM) was fitted by the Boltzmann function:
where PO(max) is the maximal open probability of BKCa-channel openings taken during the exposure to 10 μM PIO at the level of +80 mV, V1/2 the voltage at which half-maximal activation occurs, q the apparent gating charge, F Faraday’s constant, R the universal gas constant and T the absolute temperature.
Statistical Analyses
The values are expressed as the means ± SEM with sample sizes (n) indicating the number of cells from which the data were obtained, and error bars are plotted as SEM. The linear or non-linear curve-fitting to the data sets was performed with the aid of either Excel 2013 (i.e., Excel’s Solver add-in) or OriginPro 2016. The paired or unpaired Student’s t-test, or one-way analysis of variance (ANOVA) followed by post hoc Fisher’s least-significance difference test for multiple-group comparisons, were used for the statistical evaluation of differences among means. Non-parametric Kruskal–Wallis test was used, as the assumption of normality underlying ANOVA was violated. Statistical analyses were made using SPSS version 22.0 (IBM, Corp., Armonk, NY, United States). Statistical significance was determined at a P-value of <0.05.
Results
Effect of PIO on Whole-Cell Ca2+-Activated K+ Current (IK(Ca)) in mHippoE-14 Hippocampal Neurons
Whole-cell current recordings were initially made to evaluate whether PIO can exert any effects on ionic currents in these cells. In these experiments, cells were immersed in normal Tyrode’s solution containing 1.8 mM CaCl2, and the recording pipette was filled with K+-containing solution. To inactivate most of other K+ currents, the examined cells were held at 0 mV and the depolarizing voltages ranging between 0 and +60 mV were applied. Of interest, as cells were exposed to PIO at a concentration of 10 μM, current amplitudes were readily elevated throughout the entire depolarizing voltages examined (Figures 1A,B). For example, as the examined cell was depolarized from 0 to +50 mV, current amplitude was progressively increased to 203 ± 26 pA from a control value of 111 ± 14 pA (n = 11, P < 0.05). After washout of the compound, current amplitude was returned to 121 ± 16 pA (n = 11). Likewise, whole-cell ionic conductance measured at the voltages ranging between +40 and +60 mV was significantly increased from 2.8 ± 0.2 to 6.1.2 ± 0.4 nS (n = 11, P < 0.05).
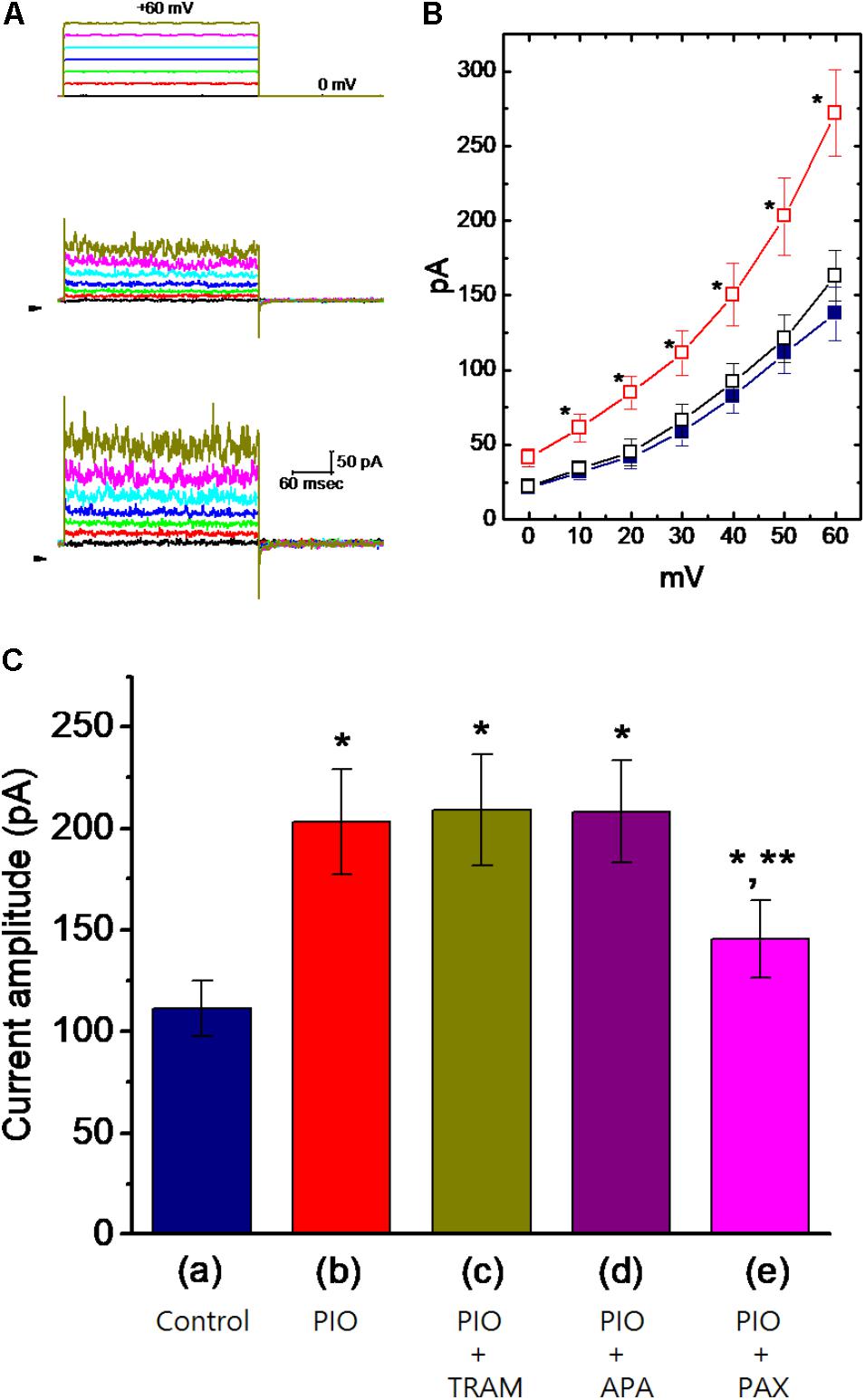
FIGURE 1. Effect of PIO on whole-cell Ca2+-activated K+ current (IK(Ca)) in mHippoE-14 hippocampal neurons. In these experiments, cells were bathed in normal Tyrode’s solution, the composition of which was described under Section “Materials and Methods.” The recording pipette was filled with K+-containing solution. (A) Superimposed IK(Ca) traces obtained in the control (middle part) and during cell exposure to 10 μM PIO (bottom part). The upper part indicates the voltage protocol applied, and arrowheads are zero current level. (B) Averaged I–V relationships of IK(Ca) obtained in the control (), during the exposure (
) to 10 μM PIO and after washout (
) of PIO (mean ± SEM; n = 11 for each point). ∗Significantly different from control groups taken at the same level of voltage pulse. (C) Bar graph showing summary of the effect of PIO, PIO plus TRAM-39, PIO plus apamin, and PIO plus paxilline, and PIO plus tolbutamide on IK(Ca) amplitude (mean ± SEM; n = 10–12 for each bar). Current amplitude was measured at +50 mV. (a) Control; (b) 10 μM PIO; (c) 10 μM PIO plus 3 μM TRAM-39; (d) 10 μM PIO plus 200 nM apamin; (e) 10 μM PIO plus 1 μM paxilline; (f) 10 μM PIO plus 30 μM tolbutamide. ∗Significantly different from control (P < 0.05) and ∗∗significantly different from PIO alone group (P < 0.05) (n = 9–10 for each bar).
The nature of PIO-induced simulation of whole-cell IK(Ca) in mHippoE-14 hippocampal neurons was further evaluated. As illustrated in Figure 1C, in continued presence of 10 μM PIO, further addition of TRAM-39 (3 μM) or apamin (200 nM) was not found to have any measurable effects on PIO-mediated increase of IK(Ca) in these cells. TRAM-39 and apamin are inhibitors of intermediate- (IKCa) and small-conductance Ca2+-activated K+ (SKCa) channels, respectively. Similarly, chlorotoxin (1 μM), an inhibitor of Cl− channels, failed to counteract PIO-stimulated increase of IK(Ca). However, in continued presence of PIO, subsequent application of paxilline (1 μM) known to suppress the activity of BKCa channels was effective at counteracting the inhibition of IK(Ca) caused by PIO. The PIO-mediated increase of IK(Ca) was little affected by further addition of 30 μM tolbutamide, an inhibitor of KATP channels. The data thus prompted us to suggest that PIO-induced stimulation of whole-cell IK(Ca) could predominantly arise from its effects on the activity of BKCa channels, not on those of IKCa or SKCa channels.
PIO Enhances BKCa-Channel Activity Measured From mHippoE-14 Hippocampal Neurons
In order to further assess whether and how PIO can alter the activity and kinetics of BKCa channels in these cells, single-channel current recordings were further performed. In these experiments, cells were bathed in symmetrical K+ concentration (145 mM) and bath medium contained 0.1 μM Ca2+. Under inside-out configuration, the examined cell was held at the level of +60 mV. As PIO at a concentration of 10 μM was applied to the bath, channel activity was drastically raised (Figure 2A), as evidenced from the results showing that addition of PIO (10 μM) increased the probability of channel openings significantly from 0.072 ± 0.004 to 0.132 ± 0.007 (n = 11, P < 0.05). After washout of the compound, channel activity returned to 0.081 ± 0.004 (n = 8) (Figure 2B).
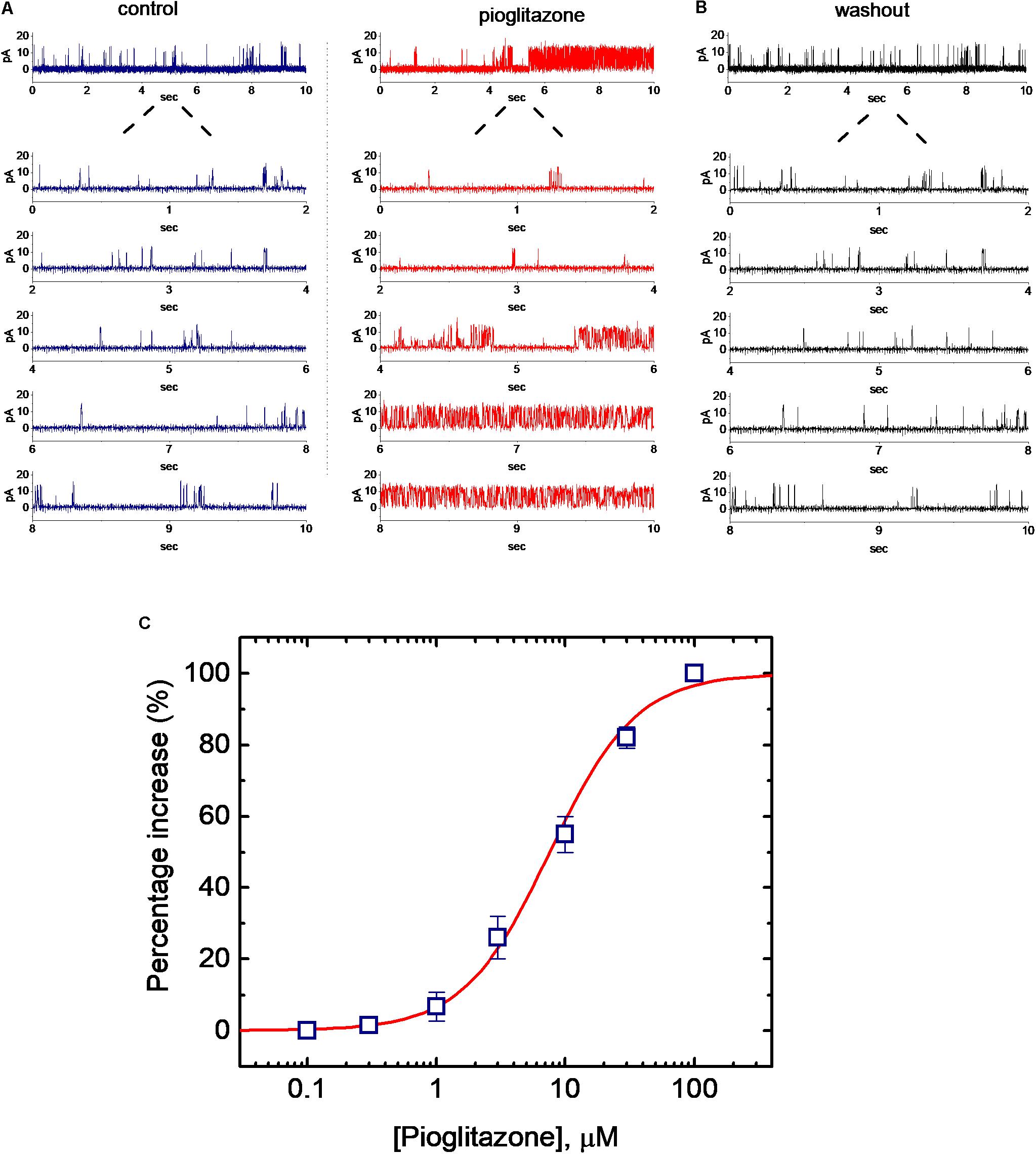
FIGURE 2. Effect of PIO on BKCa channel activity in mHippoE-14 hippocampal neurons. (A) Original current traces of BKCa channels obtained in the absence (left) and presence (right) of 10 μM pioglitazone (PIO). The examined cells were bathed in symmetrical K+ solution (145 mM). Under inside-out current recordings, the potential was held at +60 mV and bath medium contained 0.1 μM Ca2+. The upward deflection represents the opening event of the channel. The lower part indicates the expanded trace recorded from the uppermost part in the control and during exposure to PIO. (B) BKCa-channel trace obtained after washout of PIO. (C) Concentration-dependent increase in channel open probability (mean ± SEM; n = 9–11 for each point). Channel activity measured at +60 mV during the exposure to 100 μM PIO was taken to be 100%. The values for EC50, Hill coefficient and maximal percentage increase of BKCa channels in the presence of PIO were 7.6 μM 1.3 and 100%, respectively.
Concentration-dependent stimulation of BKCa-channel activity in mHippoE-14 hippocampal neurons was further evaluated. The relationship between the PIO concentration and the percentage increase of BKCa channels was derived and is hence illustrated in Figure 2C. To measure channel activity taken with or without addition of different PIO concentrations (0.1–100 μM), each detached patch was held at +60 mV. As the intracellular surface of excised patch was exposed to PIO, the probability of BKCa-channel openings was progressively raised in a concentration-dependent manner. Based on a modified form of Hill equation, the half-maximal concentration (EC50) required for stimulatory effect of PIO on channel open probability was calculated to be 7.6 ± 0.3 μM, and it at a concentration of 100 μM increased almost all of channel activity in these cells.
Mean-variance analysis for single BKCa-channel currents was further performed to evaluate whether PIO has any effect on single-channel amplitude once the open level was detected. As shown in Figure 3, the single-channel amplitude was not noted to differ significantly between the absence and presence of PIO (10 μM), despite its ability to elevate channel open probability.
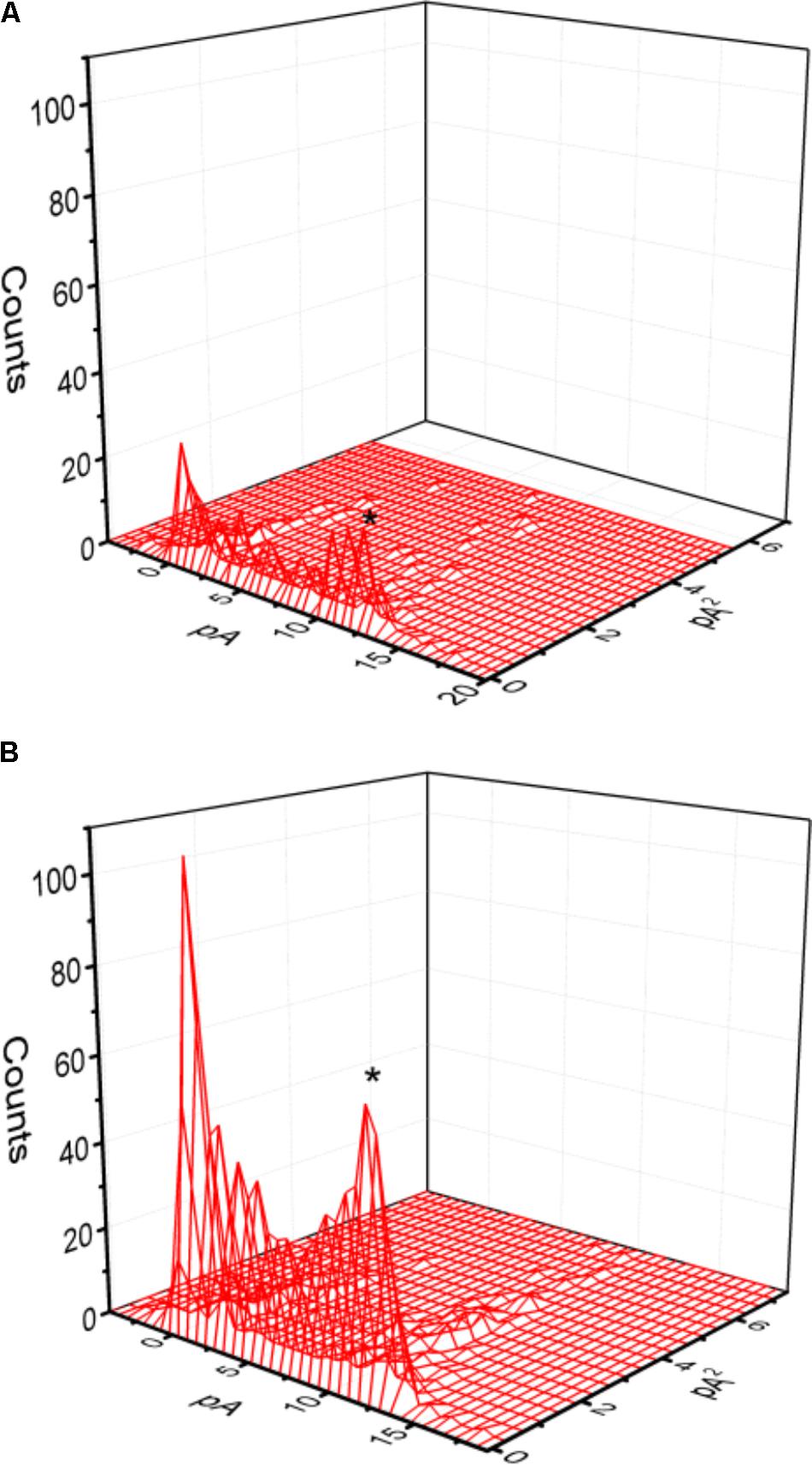
FIGURE 3. Mean-variance histogram of BKCa channels taken from the absence (A) and presence (B) of 10 μM PIO. The examined cells were bathed in symmetrical K+ solution, bath medium contained 0.1 μM Ca2+ and the holding potential was set at +60 mV. The closed state corresponds to the peak at 0 pA. The mean currents (indicated by asterisk) in (A,B) were 10.9 and 10.7 pA, respectively.
Effect of PIO on the Kinetic Behavior of BKCa Channels
Pioglitazone-induced increase of BKCa-channel activity may result from different types of single-channel kinetic behavior. As such, its effects on mean open and closed times of these channels recorded from mHippoE-14 hippocampal neurons were further examined and analyzed. As depicted in Figure 4, in an excised patch, the open-time histogram of BKCa channels at the level of +60 mV was fitted by a single exponential with mean open time of 1.9 ± 0.2 ms (n = 9). Addition of 10 μM PIO prolonged the lifetime of the open state to 2.7 ± 0.3 ms (n = 9, P < 0.05). On the other hand, the closed-time histogram of BKCa channels measured at the same level was necessarily fitted by a two-exponential curve with mean closed time of 3.5 ± 0.2 and 47.5 ± 1.3 ms (n = 9). Addition of 10 μM PIO decreased the slow component of mean closed time to 28.7 ± 1.1 ms (n = 9, P < 0.05), while it had minimal change in the fast component of mean closed time (3.4 ± 0.2 ms, n = 9, P > 0.05). Therefore, it becomes clear that stimulatory effect of PIO on BKCa-channel activity in these cells is predominantly attributable to a lengthening in channel open time and a decrease in the slow component of channel closed time, although no change in single-channel amplitude was clearly seen.
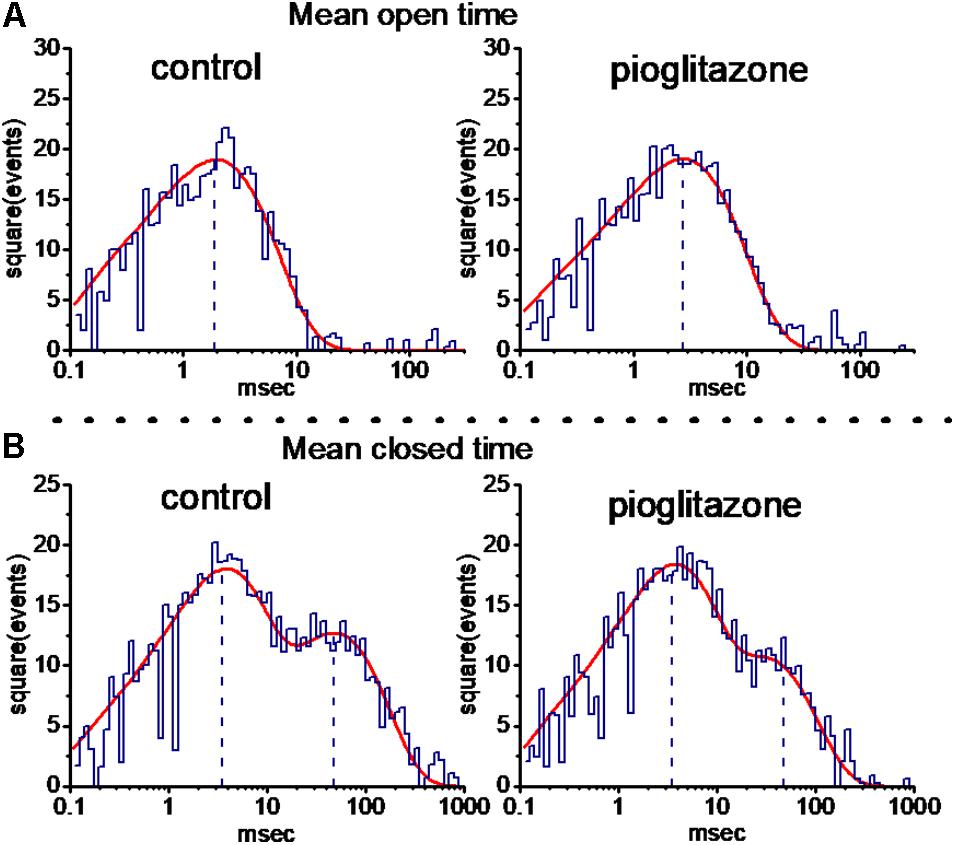
FIGURE 4. Effect of PIO on mean open- (A) and closed-time (B) histograms of BKCa channels recorded from mHippoE-14 hippocampal neurons. The holding potential was set at +60 mV, and inside-out configuration was performed. In control (left side), the open-time histogram of the channel was fitted by a single exponential function (indicated by red smooth line) with a mean open time of 1.9 ms, while the closed-time histogram was by a sum of a two-exponential function with a mean closed time of 3.5 and 47.5 ms. After addition of 10 μM PIO (right side), the mean open time was increased to 2.7 ms, and the slow component of closed time was shortened to 28.7 ms; however, minimal change in the fast component of closed time (i.e., 3.4 ms) in the presence of this compound. Of note, the abscissa and ordinate in each histogram indicate the logarithm of open or closed time (ms) and the square root of even number, respectively. Data were taken from a measurement of 100 channel openings. The vertical black dashed lines are placed at the values of mean open or closed time for BKCa channels.
Lack of Pioglitazone Effect on Single-Channel Conductance of BKCa Channels
We also measured the amplitude of single BKCa channels at a series of voltages ranging between +30 and +80 mV. Throughout the voltage range examined, the I–V relationships of BKCa channels taken with or without addition of PIO (10 μM) were analyzed and then compared (Figures 5A,B). In the control, fitting these single-channel amplitudes with a linear regression revealed BKCa channels of 165 ± 6 pS (n = 12). The value for these channels obtained in the absence of PIO did not differ significantly from that (166 ± 7 pS, n = 13, P > 0.05) during exposure to this agent. It is clear from the results that this compound applied intracellularly was unable to modify single-channel conductance, although it significantly raised the probability of BKCa channels that would be open.
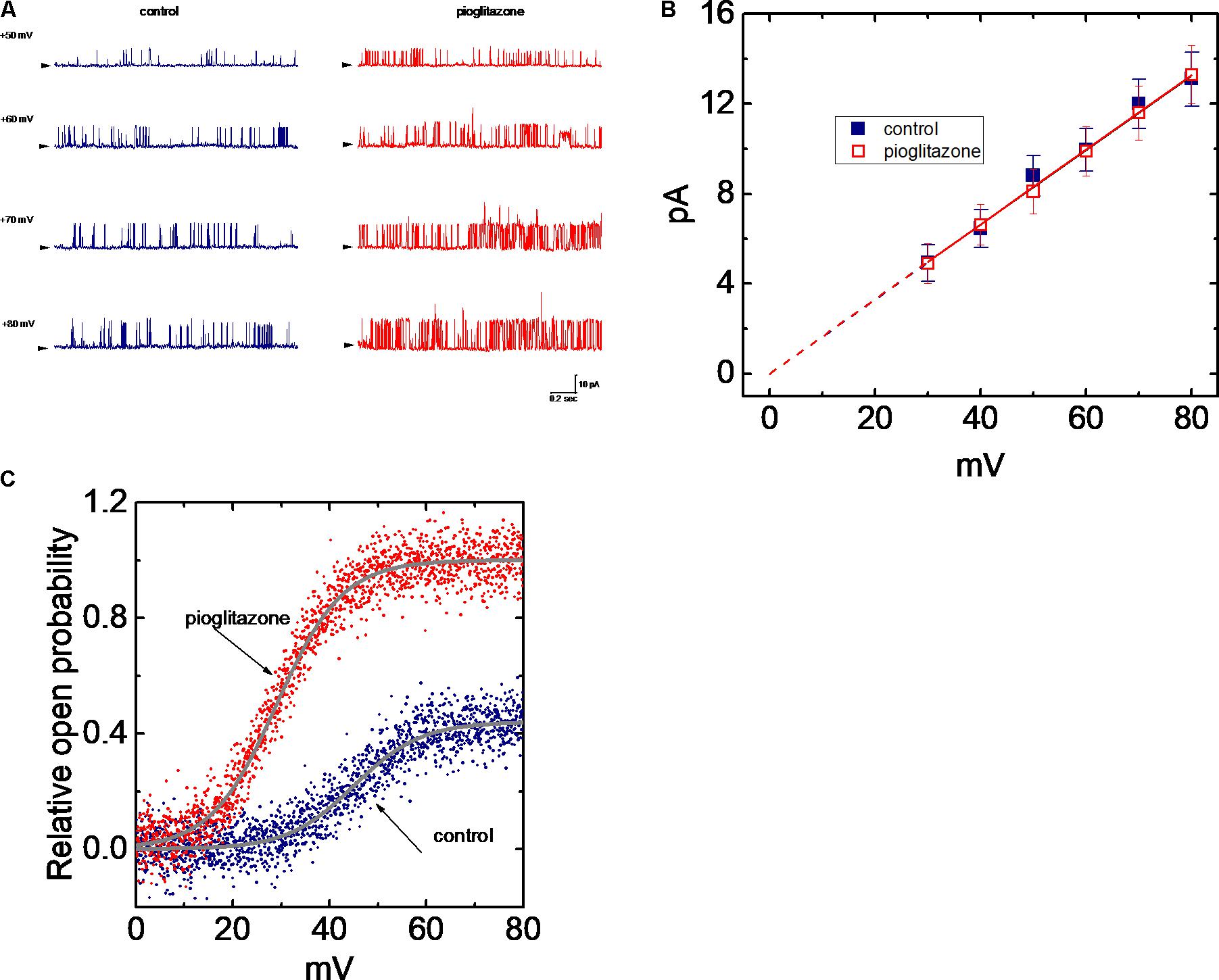
FIGURE 5. Effect of PIO on the I–V relation of BKCa channels in mHippoE-14 hippocampal neurons. The experiments on BKCa channels were conducted with symmetrical K+-rich concentration (145 mM). Under inside-out configuration, the potential was held at +60 mV and bath medium medium contained 0.1 μM Ca2+. (A) Original current traces obtained in the control and during exposure to 10 μM PIO. The labels in the rightmost side indicate the holding potential applied. Arrowhead in each trace corresponds to zero current level, and the upper deflection indicates the opening event of the channel. In (B), the single-channel conductance in the absence () and presence (
) of 10 μM PIO is nearly identical. Each point represents mean ± SEM (n = 9–10). The dashed red lines obtained with or without addition of PIO are pointed toward the values of the reversal potential (i.e., 0.0 ± 0.1 mV, n = 8). (C) The relationship between relative open probability of BKCa channels and membrane potential obtained with or without addition of 10 μM PIO. The ramp pulses were applied from 0 to +80 mV with a duration of 1 s. Under inside-out current recordings, PIO (10 μM) was applied to the intracellular surface of the excised patch. The smooth lines represent the best fit to the Boltzmann equation as detailed in Section “Materials and Methods.”
Effect of PIO on the Activation Curve of BKCa Channels
Figure 5C shows the activation curve of BKCa channels taken with or without addition of PIO (10 μM). In this set of experiments, the curves were achieved by use of digitized voltage-ramp protocols together with digital-to-analog conversion. The long-lasting ramp pulses were repetitively delivered from 0 to +80 mV with a duration of 1 s. The plots of relative open probability of BKCa channels as a function of membrane potential was derived and then fitted with the Boltzmann function as described in Section “Materials and Methods.” In control (i.e., in the absence of PIO), PO(max) = 0.44 ± 0.03, V1/2 = 45.1 ± 0.8 mV and q = 3.6 ± 0.3 e (n = 13), whereas in the presence of PIO, PO(max) = 0.99 ± 0.01, V1/2 = 28.9 ± 0.8 mV and q = 3.7 ± 0.2 e (n = 11). Therefore, the experimental results showed that PIO not only produced a 2.3-fold increase in the maximal open probability of the channel, but it also significantly shifted the activation curve to a lower membrane potential by approximately 16 mV; however, minimal change in the q value (i.e., gating charge) of the curve was seen in its presence. Therefore, the presence of PIO is capable of enhancing the activity of BKCa channels in a voltage-dependent fashion, despite no effect on single-channel conductance.
Effect of PIO on M-Type K+ Current (IK(M)) in mHippoE-14 Hippocampal Neurons
In another set of experiments, we explored the possible effect of PIO on IK(M) observed in these cells. The examined cells were bathed in high-K+, Ca2+-free solution and the recording pipette was filled with K+-containing solution. As shown in Figure 6, when the cell was depolarized from −50 to −10 mV with a duration of 1 s, K+ inward current with the slowly activating and deactivating properties was readily evoked. This K+ current elicited by long-lasting membrane depolarization was sensitive to inhibition by linopirdine (10 μM), yet not by either 4-aminopyridine (1 mM) or tetraethylammonium (10 mM), and it was hence identified as an IK(M) (Sankaranarayanan and Simasko, 1996; Selyanko et al., 1999; Huang et al., 2008). Linopirdine is recognized as a selective blocker of IK(M) (Huang et al., 2008). Notably, as cells were exposed to PIO, the IK(M) amplitude evoked in response to depolarizing pulse from −50 to −10 mV was progressively diminished, as evidenced by the data showing that addition of 10 μM PIO caused a significant reduction in current amplitude from 48.1 ± 5.1 to 17.8 ± 1.9 pA (n = 11, P < 0.05). As IK(M) elicited in response to membrane depolarization was noted to be suppressed by addition of 10 μM linopirdine. In continued presence linopirdine, further application of 10 μM flupirtine significantly reversed linopirdine-induced inhibition of IK(M) in mHippoE-14 neurons (Figure 6). Moreover, in continued presence of 10 μM PIO, subsequent addition of 10 μM flupirtine, an activator of IK(M) (Wu et al., 2012), was found to reverse its inhibition of IK(M) (Figure 7). Therefore, it is possible that the presence of PIO suppressed the amplitude of IK(M) effectively in mHippoE-14 hippocampal neurons.
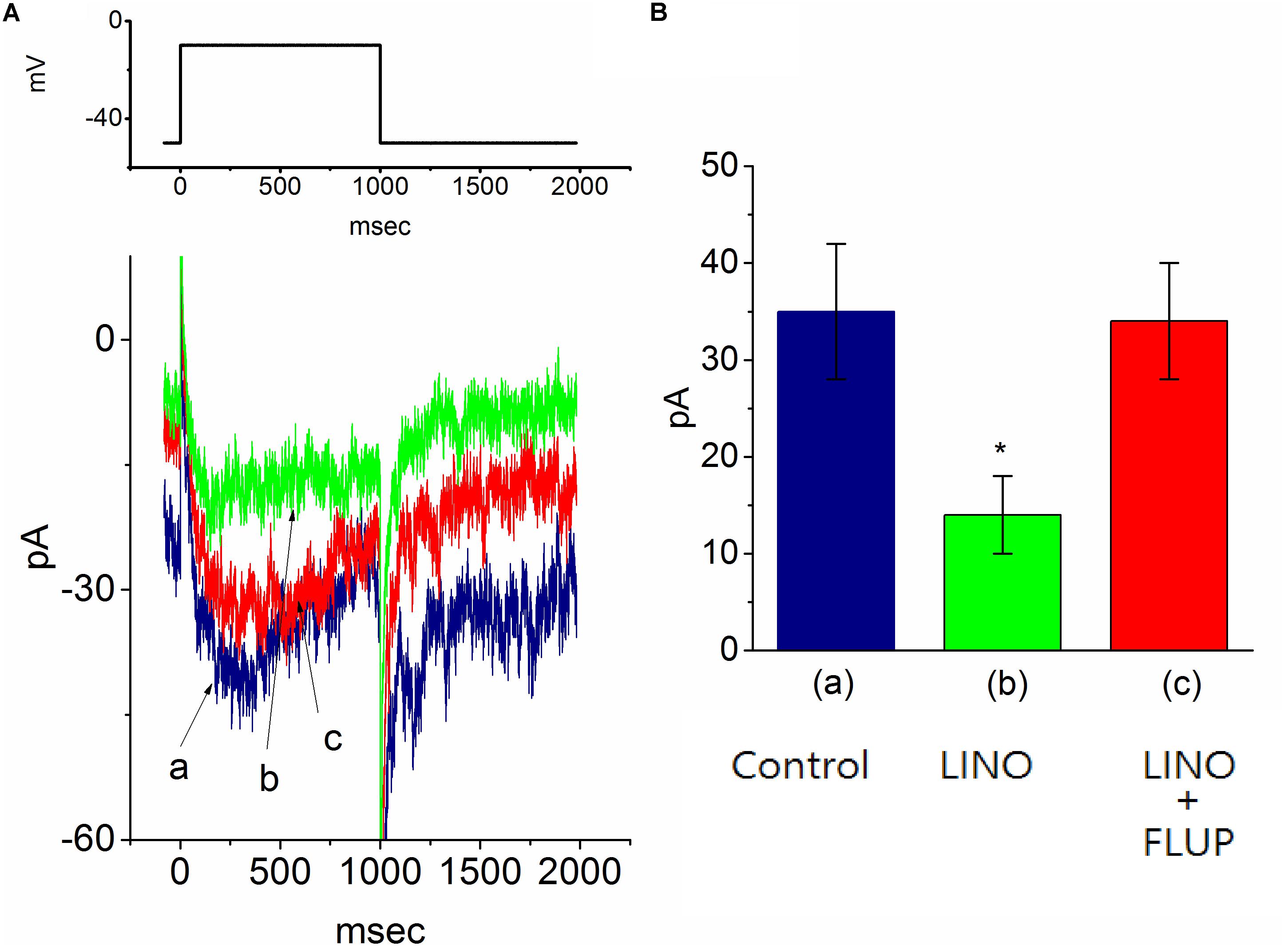
FIGURE 6. Effect of linopirdine and linopirdine plus flupirtine on the amplitude of M-type K+ current [IK(M)] in mHippoE-14 hippocampal neurons. In this set of experiments, cells were bathed in high K+, Ca2+-free solution and the recording pipette was filled with K+-containing solution. (A) Superimposed IK(M) traces obtained in the control (a) and during the exposure to 10 μM linopirdine (b), and 10 μM linopirdine plus 10 μM flupirtine (c). The upper part indicates the voltage protocol used. (B) Bar graph showing the effect of linopirdine and linopirdine plus flupirtine on IK(M) amplitude (mean ± SEM; n = 9 for each bar). ∗Significantly different from control (P < 0.05). LINO, linopirdine; FLUP, flupirtine.
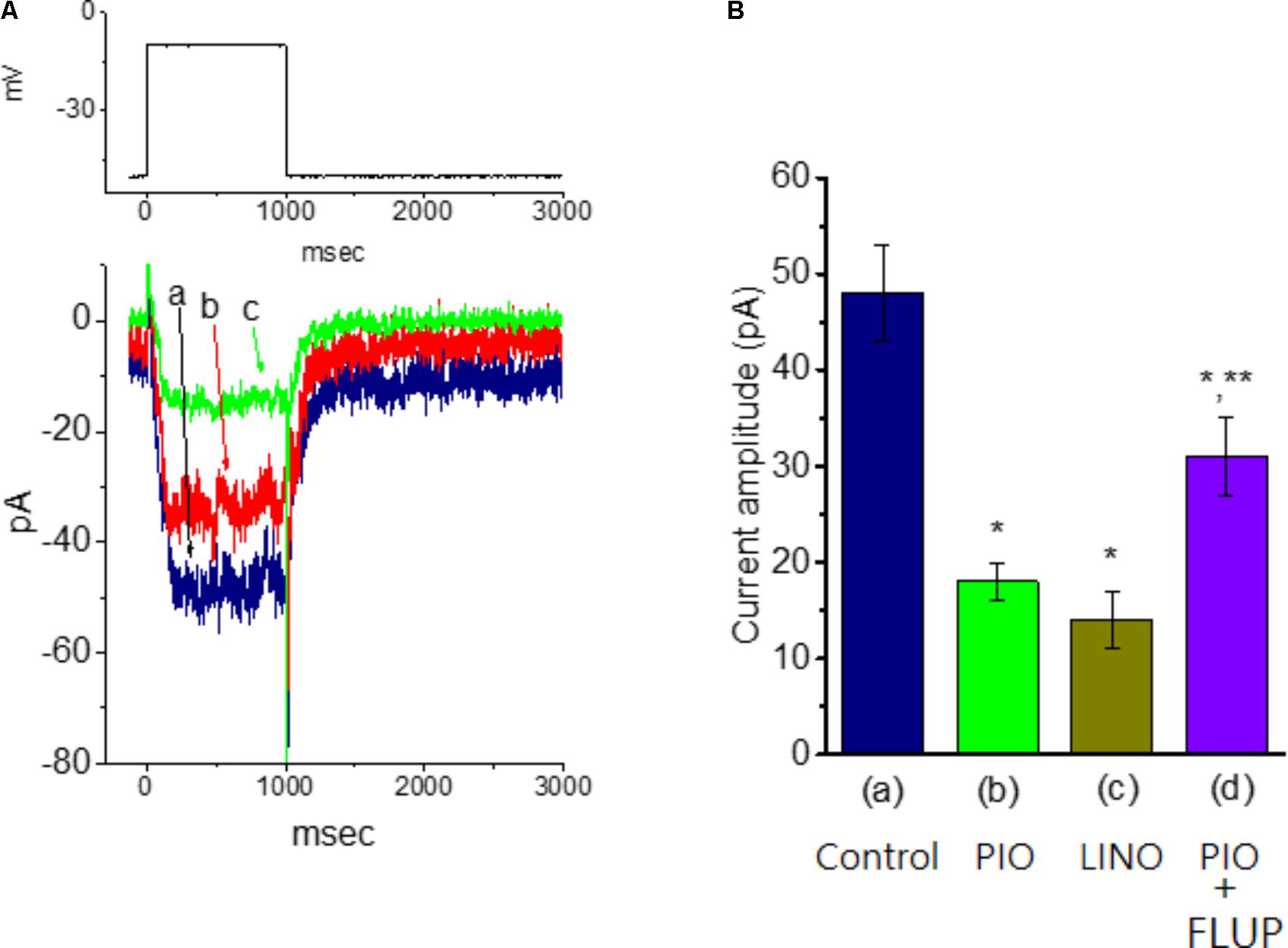
FIGURE 7. Effect of PIO on IK(M) amplitude in mHippoE-14 hippocampal neurons. These experiments were conducted in cells bathed in high K+, Ca2+-free solution and the recording pipette was filled with K+-containing solution. (A) Superimposed IK(M) traces obtained in the absence (a) and presence of 3 μM (b), and 10 μM PIO (c). The upper part indicates the voltage protocol used. (B) Bar graph showing the effect of PIO, linopirdine, and PIO plus flupirtine on IK(M) amplitude (mean ± SEM; n = 9–11 for each bar). The IK(M) amplitude elicited by membrane depolarization from –50 to –10 mV was measured. (a) Control; (b) 10 μM PIO; (c) 10 μM linopirdine; (d) 10 μM PIO plus 10 μM flupirtine. ∗Significantly different from control (P < 0.05) and ∗∗significantly different from PIO (10 μM) alone group (P < 0.05). LINO, linopirdine; FLUP, flupirtine.
Discussion
The pharmacological effects of PIO on ionic currents (i.e., IK(Ca) and IK(M)) seen in mHippoE-14 hippocampal neurons were described in this study. Application of PIO did not simply raise the amplitude of whole-cell IK(Ca). It produced a significant increase in the probability of BKCa channels that would be open with no change in single-channel amplitude. Our findings reflect that PIO is capable of enhancing BKCa-channel activity in a concentration-, voltage-, and state-dependent manner. Moreover, a leftward shift in the midpoint of the activation curve for the probability of channel openings was detected during the exposure to PIO.
In our study, PIO-mediated increase in BKCa-channel activity in mHippoE-14 hippocampal neurons is not due to an increase in single-channel amplitude because of the absence of notable difference in single-channel conductance taken with or without addition of PIO. However, a notable increase in mean open time accompanied by the shortening in slow component of mean closed time may predominantly contribute to PIO-mediated activation of BKCa channels. Moreover, the present results showed that the presence of PIO was capable of shifting the activation curve of BKCa to less depolarized potential, although no change in the gating charge for this activation was seen. This compound interacted with BKCa channels in a voltage-dependent fashion and its action would depend on the pre-existing level of membrane potential, the concentration of PIO used, or both.
Previous studies have demonstrates the ability of different TZDs to modulate the activity of KATP channels (Sunaga et al., 1999). The intracellular solution used in our whole-cell recordings contained 3 mM ATP, a value thought to suppress the activity of functional KATP channels adequately. The PIO-mediated increase of IK(Ca) was little affected by further addition of 30 μM tolbutamide, an inhibitor of KATP channels. Neither TRAM-39 nor apamin countered PIO-mediated increase of IK(Ca), while further addition of paxilline was effective at reversing it. Therefore, PIO-induced increase of K+ outward currents described in this study is unlikely to be predominantly linked to the stimulation of KATP, IKCa or SKCa channels, although these channels might be functionally active in hippocampal neurons.
Finding from the present results tends to be consistent with previous observations showing that different TZDs can regulate the activity of BKCa channels (Wu et al., 2000; Li et al., 2002). Several studies have previously demonstrated that troglitazone and PIO might differentially perturb ionic currents in isolated cardiac myocytes and in pancreatic β cells (Ikeda and Watanabe, 1998; Sunaga et al., 1999). Therefore, the ability of PIO to stimulate BKCa channels as well as to suppress IK(M) amplitude observed in the present study appears to be necessarily noted with caution in relation to its use as an agonist of PPAR-γ activation.
In continued presence of PIO, neither TRAM-39 nor apamin produced any effects on its stimulation of IK(Ca). TRAM-39 and apamin are known to block the intermediate- and small-conductance Ca2+-activated K+ channels, respectively. The observed effect of PIO on whole-cell IK(Ca) inherently in mHippoE-14 hippocampal neurons clearly did not involve the stimulation of small- and intermediate-conductance Ca2+-activated K+ channels. The activation of BKCa channels by PIO could be predominantly responsible for the increase of macroscopic IK(Ca) amplitude.
As far as the resting membrane potential is concerned, membrane hyperpolarization due to PIO-induced increase of BKCa channels could be potentially offset by its suppression IK(M). On the other hand, increased activity of BKCa channels would be expected to hasten the repolarization of neuronal action potential and to facilitate INa recovery. As a result, apart from stimulation of the nuclear receptor PPAR-γ, to what extent fluctuations in the resting membrane potential accompanied by shortening of neuronal action potential caused by PIO contributes to its pharmacological actions (e.g., insulin-sensitizing effects) remain to be further delineated.
One previous study reported that a peak plasma level of 3.4 μM for total PIO is present following a maximal PIO dose of 45 mg (Budde et al., 2003) and PIO was considered as “harmless at therapeutic concentrations” and it’s not likely that normally dosed PIO can strongly alter cardiac electrogenesis in healthy individuals (Kistamás et al., 2013). Although, the EC50 value required for PIO to stimulate BKCa-channel activity is relatively higher in our study. However, the present results showed that the presence of PIO was capable of shifting the activation curve of BKCa to less depolarized potential. This compound was capable of interacting with BKCa channels in a voltage-dependent fashion and its action would depend on the pre-existing level of membrane potential, the concentration of PIO used, or both. The effects reported herein are likely to be the relevant at therapeutic doses. Such discrepancy remains to be further delineated in the near future.
In summary, both stimulation of BKCa channels and inhibition of IK(M) caused by PIO may synergistically act to influence the electrical behavior of hippocampal neurons.
Author Contributions
T-SC, M-CL, C-WH, and S-NW conducted the experiments and data interpretation. T-SC, M-CL, T-YH, and C-WH analyzed the data and assisted in data interpretation. K-ML, C-WH, and S-NW interpreted study data and assisted in statistical analyses. T-SC, M-CL, C-WH, and S-NW conceptualized and designed the study, interpreted study data, and revised the manuscript. C-WH and S-NW contributed to qualify as the senior authors.
Funding
This study was partly funded by the National Cheng Kung University (Grant No. D104-35A16), Tainan, Taiwan and the Taiwan National Science Council (Grant No. NSC-102-2314-B-006-051-MY3), the Ministry of Science and Technology, ROC (Grant Nos. 105-2314-B-006-013-, 106-2314-B-006-034-, 106-2320-B-006-055-, 107-2314-B-006-018-, and 107-2320-B-006-019-), the National Cheng Kung University Hospital (Grant No. 20180254), and the Chi-Mei Medical Center (Grant No. CMNCKU10303).
Conflict of Interest Statement
The authors declare that the research was conducted in the absence of any commercial or financial relationships that could be construed as a potential conflict of interest.
Acknowledgments
Part of this abstract has been presented in Taiwan Neurological Society Annual Meeting, 2013. The authors acknowledge Huei-Ting Su for parts of the earlier experiments.
Abbreviations
BKCa channel, large-conductance Ca2+-activated K+ channel; EC50, the concentration required for half-maximal stimulation; FDA, Food and Drug Administration; I–V, current versus voltage; IKCa channel, intermediate-conductance Ca2+-activated K+ channel; IK(Ca), Ca2+-activated K+ current; KATP channel; ATP-sensitive K+ channel; PIO, pioglitazone; PPAR-γ, peroxisome proliferator-activated receptor-γ; SEM, standard error of mean; SKCa channel, small-conductance Ca2+-activated K+ channel; TZD, thiazolidinedione.
Footnotes
References
Asano, M., Nakajima, T., Iwasawa, K., Morita, T., Nakamura, F., Imuta, H., et al. (1999). Troglitazone and pioglitazone attenuate agonist-dependent Ca2+ mobilization and cell proliferation in vascular smooth muscle cells. Br. J. Pharmacol. 128, 673-683. doi: 10.1038/sj.bjp.0702818
Blalock, E. M., Phelos, J. T., Pancani, T., Searcy, J. L., Anderson, K. L., Gant, J. C., et al. (2010). Effects of long-term pioglitazone treatment on peripheral and central markers of aging. PLoS One 5:e10405. doi: 10.1371/journal.pone.0010405
Brown, B. S., and Yu, S. P. (2000). Modulation and genetic identification of the M channel. Prog. Biophys. Mol. Biol. 73, 135-166. doi: 10.1016/S0079-6107(00)00004-3
Budde, K., Neumayer, H. H., Fritsche, L., Sulowicz, W., Stompôr, T., and Eckland, D. (2003). The pharmacokinetics of pioglitazone in patients with impaired renal function. Br. J. Clin. Pharmacol. 55, 368-374. doi: 10.1046/j.1365-2125.2003.01785.x
Evans, N. J., Bayliss, A. L., Reale, V., and Evans, P. D. (2016). Characterization of signalling by the endogenous GPER1 (GPR30) receptor in an embryonic mouse hippocampal cell line (mHippoE-18). PLoS One 11:e0152138. doi: 10.1371/journal.pone.0152138
Gingerich, S., Kim, G. L., Chalmers, J. A., Koletar, M. M., Wang, X., Wang, Y., et al. (2010). Estrogen receptor alpha and G-protein coupled receptor 30 mediate the neuroprotective effects of 17β-estradiol in novel murine hippocampal cell models. Neuroscience 170, 54-66. doi: 10.1016/j.neuroscience.2010.06.076
Gu, J., Hu, W., and Liu, X. (2014). Pioglitazone improves potassium channel remodeling induced by angiotensin II in atrial myocytes. Med. Sci. Monit. Basic Res. 20, 153-160. doi: 10.12659/MSMBR.892450
Hsu, H. T., Tseng, Y. T., Lo, Y. C., and Wu, S. N. (2014). Ability of naringenin, a bioflavonoid, to activate M-type potassium current in motor neuron-like cells and to increase BKCa-channel activity in HEK293T cells transfected with β-hSlo subunit. BMC Neurosci. 15:135. doi: 10.1186/s12868-014-0135-1
Huang, C. W., Huang, C. C., Lin, M. W., Tsai, J. J., and Wu, S. N. (2008). The synergistic inhibitory actions of oxcarbazepine on voltage-gated sodium and potassium currents in differentiated NG108-15 neuronal cells and model neurons. Int. J. Neuropsychopharmacol. 11, 597-610. doi: 10.1017/S1461145707008346
Huang, C. W., Huang, C. C., and Wu, S. N. (2007). Activation by zonisamide, a newer antiepileptic drug, of large-conductance calcium-activated potassium channel in differentiated hippocampal neuron-derived H18-7 cells. J. Pharmacol. Exp. Ther. 321, 98-106. doi: 10.1124/jpet.106.116954
Huang, C. W., Lin, K. M., Hung, T. Y., Chuang, Y. C., and Wu, S. N. (2018). Multiple actions of rotenone, an inhibitor of mitochondrial respiratory chain, on ionic currents and miniature end-plate potential in mouse hippocampal (mHippoE-14) neurons. Cell. Physiol. Biochem. 47, 330-343. doi: 10.1159/000489810
Ikeda, S., and Watanabe, T. (1998). Effects of troglitazone and pioglitazone on the actions and membrane currents of rabbit ventricular myocytes. Eur. J. Pharmacol. 357, 243-250. doi: 10.1016/S0014-2999(98)00557-3
Kapadia, R., Yi, J. H., and Vemuganti, R. (2008). Mechanisms of anti-inflammatory and neuroprotective actions of PPAR-gamma agonists. Front. Biosci. 13, 1813-1826. doi: 10.2741/2802
Kersten, S., Desvergne, B., and Wahli, W. (2000). Roles of PPARs in health and disease. Nature 405, 421-424. doi: 10.1038/35013000
Kistamás, K., Szentandrássy, N., Hegyi, B., Ruzsnavszky, F., Váczi, K., Bárándi, L., et al. (2013). Effects of pioglitazone on cardiac ion currents and action potential morphology in canine ventricular myocytes. Eur. J. Pharmacol. 710, 10-19. doi: 10.1016/j.ejphar.2013.03.047
Lai, M. C., Hung, T. Y., Lin, K. M., Sung, P. S., Wu, S. J., Yang, C. S., et al. (2018). Sodium metabisulfite: effects on ionic currents and excitotoxicity. Neurotox. Res. 34, 1-15. doi: 10.1007/s12640-017-9844-4
Lazniewska, J., Milowska, K., Zablocka, M., Mignani, S., Caminade, A. M., Majoral, J. P., et al. (2013). Mechanism of cationic phosphorus dendrimer toxicity against murine neural cell lines. Mol. Pharm. 10, 3484-3496. doi: 10.1021/mp4003255
Li, J., Xu, B., Chen, Z., Zhou, C., Liao, L., Qin, Y., et al. (2018). PI3K/AKT/JNK/p38 signalling pathway-mediated neural apoptosis in the prefrontal cortex of miceis involved in the antidepressant-like effect of pioglitazone. Clin. Exp. Pharmacol. Physiol. 45, 525-535. doi: 10.1111/1440-1681.12918
Li, P. C., Liang, J. T., Huang, H. T., Lin, P. H., and Wu, S. N. (2002). Enhanced activity of Ca2+-activated K+ channels by 1-[2-hydroxy-3-propyl-4-[(1H-tetrazol-5-yl)butoxyl]phenyl] ethanone (LY-171883) in neuroendocrine and neuroblastoma cell lines. J. Cell. Physiol. 192, 188-199. doi: 10.1002/jcp.10127
Pancani, T., Phelps, J. T., Searcy, J. L., Kilgore, M. W., Chen, K. C., Porter, N. M., et al. (2009). Distinct modulation of voltage-gated and ligand-gated Ca2+ currents by PPAR-gamma agonists in cultured hippocampal neurons. J. Neurochem. 109, 1800-1811. doi: 10.1111/j.1471-4159.2009.06107.x
Sankaranarayanan, S., and Simasko, S. M. (1996). Characterization of an M-like current modulated by thyrotropin-releasing hormone in normal rat lactotrophs. J. Neurosci. 16, 1668-1678. doi: 10.1523/JNEUROSCI.16-05-01668.1996
Schlachetzki, J. C., and Winkler, J. (2015). The innate immune system in Parkinson’s disease: a novel target promoting endogenous neuroregeneration. Neural Regen. Res. 10, 704-706. doi: 10.4103/1673-5374.156958
Selyanko, A. A., Hadley, J. K., Wood, I. C., Abogadie, F. C., Delmas, P., Buckley, N. J., et al. (1999). Two types of K+ channel subunit, erg1 and KCNQ23, contribute to the M-like current in a mammalian neuronal cell. J. Neurosci. 19, 7742-7756. doi: 10.1523/JNEUROSCI.19-18-07742.1999
Storm, J. F. (1987). Action potential repolarization and a fast after-hyperpolarization in rat hippocampal pyramidal cells. J. Physiol. 385, 733-759. doi: 10.1113/jphysiol.1987.sp016517
Sunaga, Y., Inagaki, N., Gonoi, T., Yamada, Y., Ishida, H., Seino, Y., et al. (1999). Troglitazone but not pioglitazone affects ATP-sensitive K+ channel activity. Eur. J. Pharmacol. 381, 71-76. doi: 10.1016/S0014-2999(99)00539-7
Tontonoz, P., and Spiegelman, B. M. (2008). Fat and beyond: the diverse biology of PPARg. Annu. Rev. Biochem. 77, 289-312. doi: 10.1146/annurev.biochem.77.061307.091829
Wu, S. N., Chern, J. H., Shen, S., Chen, H. H., Hsu, Y. T., Lee, C. C., et al. (2017). Stimulatory actions of a novel thiourea derivative on large-conductance, calcium-activated potassium channels. J. Cell. Physiol. 232, 3409–3421. doi: 10.1002/jcp.25788
Wu, S. N., Ho, L. L., Li, H. F., and Chiang, H. T. (2000). Regulation of Ca2+-activated K+ currents by ciglitazone in rat pituitary GH3 cells. J. Investig. Med. 48, 259–269.
Wu, S. N., Hsu, M. C., Liao, Y. K., Wu, F. T., Jong, Y. J., and Lo, Y. C. (2012). Evidence for inhibitory effects of flupirtine, a centrally acting analgesic, on delayed rectifier K+ currents in motor neuron-like cells. Evid. Based Complement. Alternat. Med. 2012:148403. doi: 10.1155/2012/148403
Wu, S. N., Lin, P. H., Hsieh, K. S., Liu, Y. C., and Chiang, H. T. (2003). Behavior of nonselective cation channels and large-conductance Ca2+-activated K+ channels induced by dynamic changes in membrane stretch in cultured smooth muscle cells of human coronary artery. J. Cardiovasc. Electrophysiol. 14, 44-51. doi: 10.1046/j.1540-8167.2003.02040.x
Keywords: pioglitazone, Ca2+-activated K+ current, large-conductance Ca2+-activated K+ channel, M-type K+ current, hippocampal neuron
Citation: Chen T-S, Lai M-C, Hung T-Y, Lin K-M, Huang C-W and Wu S-N (2018) Pioglitazone, a PPAR-γ Activator, Stimulates BKCa but Suppresses IKM in Hippocampal Neurons. Front. Pharmacol. 9:977. doi: 10.3389/fphar.2018.00977
Received: 14 April 2018; Accepted: 08 August 2018;
Published: 29 August 2018.
Edited by:
Ashok Kumar, University of Florida, United StatesReviewed by:
John J. Wagner, University of Georgia, United StatesKyle Blaine Kelly, Northwestern Medicine, United States
Copyright © 2018 Chen, Lai, Hung, Lin, Huang and Wu. This is an open-access article distributed under the terms of the Creative Commons Attribution License (CC BY). The use, distribution or reproduction in other forums is permitted, provided the original author(s) and the copyright owner(s) are credited and that the original publication in this journal is cited, in accordance with accepted academic practice. No use, distribution or reproduction is permitted which does not comply with these terms.
*Correspondence: Chin-Wei Huang, aHVhbmdjd0BtYWlsLm5ja3UuZWR1LnR3 Sheng-Nan Wu, c253dUBtYWlsLm5ja3UuZWR1LnR3
†These authors have contributed equally to this work