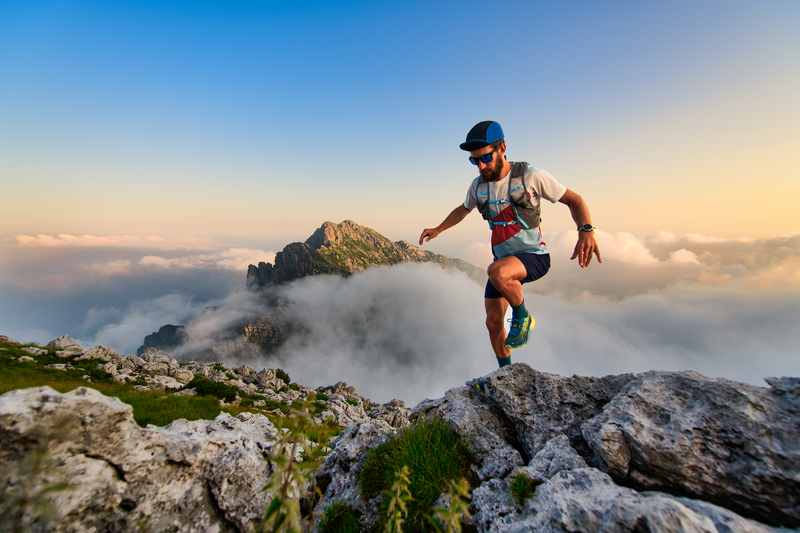
94% of researchers rate our articles as excellent or good
Learn more about the work of our research integrity team to safeguard the quality of each article we publish.
Find out more
REVIEW article
Front. Pharmacol. , 05 September 2017
Sec. Predictive Toxicology
Volume 8 - 2017 | https://doi.org/10.3389/fphar.2017.00606
In the last two decades, nanotechnologies demonstrated various applications in different fields, including detection, sensing, catalysis, electronics, and biomedical sciences. However, public concerns regarding the well-being of human may hinder the wide utilization of this promising innovation. Although, humans are exposed to airborne nanosized particles from an early age, exposure to such particles has risen dramatically within the last century due to anthropogenic sources of nanoparticles. The wide application of nanomaterials in industry, consumer products, and medicine has raised concerns regarding the potential toxicity of nanoparticles in humans. In this review, the effects of nanomaterials on the reproductive system in animal models are discussed. Females are particularly more vulnerable to nanoparticle toxicity, and toxicity in this population may affect reproductivity and fetal development. Moreover, various types of nanoparticles have negative impacts on male germ cells, fetal development, and the female reproductive system. These impacts are associated with nanoparticle modification, composition, concentration, route of administration, and the species of the animal. Therefore, understanding the impacts of nanoparticles on animal growth and reproduction is essential. Many studies have examined the effects of nanoparticles on primary and secondary target organs, with a concentration on the in vivo and in vitro effects of nanoparticles on the male and female reproductive systems at the clinical, cellular, and molecular levels. This review provides important information regarding organism safety and the potential hazards of nanoparticle use and supports the application of nanotechnologies by minimizing the adverse effects of nanoparticles in vulnerable populations.
The vast growth of nanotechnologies with all their far-reaching benefits has fostered concerns about the potential health risks of nanoparticles (NPs) (Ema et al., 2010). These products currently have extensive applications in nearly all manufacturing sectors. For example, advances in nano medicine may provide solutions for the early diagnosis of diseases and in personalized medicine with regard to treating complex diseases, such as cancer or metabolic disorders (Sanhai et al., 2008). Nanotechnologies also provide potential systems for helping to resolve societal difficulties, such as energy shortages (Le Goff et al., 2009; Li et al., 2012) and environmental pollution (Savage and Diallo, 2005). Due to their unique characteristics, NPs are widely used in biomedical and industrial applications (Lee et al., 2007; Zhang et al., 2008; Das et al., 2009; Vance et al., 2015). Currently, there are 1,814 marketed consumer products containing nanoparticles, including antibiotics, food items, textiles, sports tools, and electronic materials, and the number is increasing steadily (Chou et al., 2008; Vance et al., 2015).
Despite the benefits of NPs, various applications of nanotechnology have exposed humans and animals to their potential toxicities. As far as the exposure of humans to NPs is concerned, they can enter the body through inhalation, ingestion, skin uptake, injection, or implantation (Figure 1; Oberdörster et al., 2005). It is also interesting to note that NP uptake could be intentional or non-intentional (Yah et al., 2012). Thus, the wide use of nanomaterials has raised concerns about the negative impact of NPs on human health, mainly on the reproductive systems of both men and women and on fetal health, particularly in view of the small size of NPs, their ease of penetration and biocompatibility and their potential ability to breach the placental barrier. Early research studies on anthropogenic NPs, for example, diesel exhaust (DE), demonstrate that, due to with daily exposure, they aggregate in and bind to human cells, disturbing normal physiological systems. Additionally, NPs are associated with different disorders in animals, including pulmonary injury, heapatotoxicity, immuno-nanotoxicity neurotoxicity, renal toxicity, and irreversible testis damage (Derfus et al., 2004; Chou et al., 2008; Lin et al., 2008; Schipper et al., 2008; Wu et al., 2011; Bartneck et al., 2012; Vance et al., 2015).
Figure 1. Scheme of the different exposure routes of nanoparticles in the human body. (I) Skin, (II) inhalation, (III) fabric, (VI) intravenous injection, (V) food intake, (VI) water intake, (VII) gastrointestinal tract, (VIII) lymph, (IX) bone marrow, (X) breast milk, (XI) placenta, (XII) kidney, (XIII) muscles, (XIV) liver, and (XV) spleen.
Various reports describe the pharmacological applications of NPs and the effects of environmental exposure to NPs (Chen et al., 2003; Xue et al., 2005; Kim et al., 2006). The primary objective of all the above-mentioned studies was to determine the toxicity of surface modified NPs and to analyze whether these NPs exhibited particular biological characteristics, and they were also assessed to determine if they were effective as gene or drug transfection vectors. However, their utility, in this regard, introduces another opportunity for potentially toxic effects in various organs, including the reproductive system, if the NPs are distributed throughout the body.
Fertility, reproduction, and fetal development are essential to sustain a species, highlighting the importance of the growing public awareness of the toxicity of NPs on the reproductive system. Women have only about 400 follicles that reach maturity and undergo ovulation during their lifetime, meaning that there is a limited opportunity for reproduction (Hillier, 1994; Song et al., 2009). Moreover, reproductive female organs, including the uterus and ovaries, exhibit periodic growth, and regeneration that is regulated by hormones. The hormonal control system has dynamic functions and is susceptible to the physiological stress caused by foreign particles (Warren and Perlroth, 2001; Armenti et al., 2008), and any interruption in female reproduction potentially results in fetal anomalies.
Environmental pollutants have toxic effects on reproduction and embryonic development (Anway et al., 2005; Armenti et al., 2008). Similarly, NPs present a potential threat to the susceptible female population, and their toxicity has been studied in different models of female reproductive health (Tsuchiya et al., 1996; Wang et al., 2011). Both short- and long-term toxicities in animals and humans have been documented. Additionally, several reports demonstrate the biological effects of NPs on isolated physiological systems, such as organs, biomolecules, and primary cells. Overall, such studies have raised as many questions as they have answered, and it is clear that more studies are needed to determine the mechanisms by which NPs affect particular organ systems. Moreover, NPs can distribute in different organs, and signals may be transmitted between these organ systems, affecting the entire individual. This is not limited to females. NPs can also cross the biological barriers shielding various parts of the human body, such as the blood-testes barrier and enter the testes in animal models (Araujo et al., 1999).
Many studies on the toxicity of NPs have been conducted in mice and rats, which exhibit genetic similarities to humans. Although, these are common mammalian models, their use is limited by their long developmental cycle and ethical concerns. Moreover, it is difficult to study their development in utero. Therefore, zebrafish have been used as a model for molecular studies, embryonic development, and developmental biology. Herein, in vitro and in vivo toxicological evaluations of NPs in animal models, such as mice, rats, and zebrafish and the impact of NPs on the male and female reproductive systems are discussed, with an emphasis on the results of the exposure to man-made NPs.
The development of novel nanoparticle-based technologies and products for the delivery of nanoparticles to humans is constantly expanding. The current methods for delivering nanoparticles for the treatment of human disease include oral administration, transdermal delivery, intravenous injection, or implantation (Wennerberg et al., 2011). Exposure of the human body to nanoparticles can also accidentally occur via inhalation, dermal contact, or swallowing (Figure 1). Upon entering the human circulation, nanoparticles undergo metabolism, as well as excretion and/or retention in various body compartments. Different nanoparticles have distinctly different physiochemical properties and vary in their size, shape, and surface properties, which determine how they are absorbed, distributed, metabolized, and eliminated in the human body as well as inside the cells. These processes as referred to as the absorption, distribution, metabolism, and elimination (ADME) processes and will be discussed in detail next. The adaptive evolution of mammals has led to the employment of various physiological barriers for their protection against natural and environmental hazards. One of the most important barriers of this kind in humans is the skin, which prevents absorption by the contact of nanoparticles. Despite the effectiveness of such protective mechanisms, nanoparticles smaller in size can still penetrate the skin barrier and enter human circulation (Wu et al., 2009). Upon entrance into the blood circulation, nanoparticles use the bloodstream to reach body compartments and vital organs such as the liver and spleen. In the latter organ, nanoparticles are captured by the cells of the reticuloendothelial system (RES), which is a process that is essential for the deactivation and elimination of foreign bodies (Sadauskas et al., 2007; Liu et al., 2008b). Other vital organs of the human body that nanoparticles reach include the brain (Elder et al., 2006; Wang et al., 2008), and the testis (Bai et al., 2010), or even the fetus, which are protected by their own specialized barriers. Nevertheless, even these vital organs are not fully protected, since certain nanoparticles can effectively penetrate their barriers (De Jong et al., 2008). The ability of nanoparticles to bypass/penetrate these defensive, protective barriers of the human body depends on their physical (e.g., size, shape, aspect ratio; Meng et al., 2007; Qiu et al., 2010; Ma et al., 2011) and chemical properties (e.g., aggregation, surface chemical, charge status). For example, positively charged nanoparticles can more effectively enter the cell since the cellular membrane (which consists of a double layer of phospholipids) is negatively charged. This has been also confirmed in independent experiments studying the cellular uptake of nanoparticles (e.g., polyethyleneimine-coated mesoporous silica nanoparticles), which are positively charged, demonstrating an increased uptake by cells compared to negatively charged nanoparticles (Xia et al., 2009). Thus, the increased uptake of positively charged (cationic) nanoparticles may result in increased damage of membrane phospholipids as well as increased damage to cellular compartments (e.g., the lysosomes; Xia et al., 2006). Other factors affecting the cellular uptake of nanoparticles include ligand molecules that bind to specific cell membrane receptors (Nel et al., 2009). How nanoparticles travel between different organ systems remains poorly understood. This is a process regulated by complex mechanisms and may include the translocation into the systemic blood circulation via the lymphatic vessels, as in the case of dermal or gastrointestinal absorption (Jani et al., 1990; Kim et al., 2004). In the case of inhalation, the possible routes that the nanoparticles can be transferred to include the blood (systemic) circulation, the lymphatic vessels, the gastrointestinal tract, and the central and/or peripheral nervous system (Oberdörster et al., 2005). In particular cases, such as in the case of Caenorhabditis elegans, ingested quantum dots can also reach the reproductive system (Qu et al., 2011), which is a process that is mainly regulated by the properties of nanoparticles, such as their surface charge (Choi et al., 2010). Given that the ADME process of nanoparticles heavily depends on their physiochemical properties (Zhang et al., 2014), Table 1 summarizes the nanoparticle properties that affect their distribution in the human body as well as the possible routes of exposure, and the main findings of the published studies. Recently, accumulating evidence has elucidated the fate of nanoparticles upon entering the human body (Wang et al., 2012). For example, the metabolism of nanoparticles entering the stomach is now better understood, and the role of cellular lysosomes in their metabolism is now well-established. The entrance of nanoparticles into cells occurs mainly by endocytosis. Nevertheless, carriage by endosomes is done in an acidic environment, which can have a potent effect on certain metallic nanoparticles, e.g., silver (Arora et al., 2008), quantum dots (Gao et al., 2004; Hoshino et al., 2004), or iron oxide nanoparticles (Arbab et al., 2005; Zhu et al., 2011), and induce their dissolution. Similarly, non-metallic nanoparticles, e.g., SiO2 nanoparticles (Souris et al., 2010), can also be affected by the pH of the microenvironment they are carried into and undergo dissolution intracellularly. Therefore, the development of appropriate coatings to protect nanoparticles from their dissolution could increase their durability in vivo and minimize their toxic effects on human cells (Li et al., 2011). Experimental studies suggest that nanoparticles undergo enzymatic catalysis [e.g., single-walled carbon nanotubes (SWCNTs) and multi-walled carbon nanotubes (MWCNTs) can be degraded by horseradish peroxidase and human myeloperoxidase]. Whether this is applicable in-vivo is something that has to be explored (Kagan et al., 2010). The effective clearance of nanoparticles via their enzymatic degradation in the liver or lungs minimizes their deleterious toxic effects (Kagan et al., 2010). Liver cells contain several enzymatic systems that enzymatically degrade nanoparticles (Wang et al., 2012), while lung macrophages internalize large nanoparticles, such as those with a diameter above 100 nm (Zhu et al., 2009). The surface properties of nanoparticles are other important factors that determine their fate in the human body. For example, polyethylene glycol-coating of nanoparticles prevents their endocytosis by RES cells and extends their circulation in human blood (Liu et al., 2008b). Other chemical properties of nanoparticles, such as their stereoisomeric form, also determine their toxicity. For example, d-glutathione coated cadmium telluride (CdTe) quantum dots have a lower cytotoxicity compared to l-glutathione (Li et al., 2011). When entering the human body, nanoparticles can undergo passive modifications that might differentially affect their metabolism (Lundqvist et al., 2008; Walkey and Chan, 2012). One such passive modification is the adsorption of proteins on their surface, which forms a corona. This contributes to a more effective recognition by immune cells and improved clearance (Ruge et al., 2011) or conversely attenuates their aggregation and prevents them from undergoing phagocytosis (Geiser, 2010). Other modifications that the nanoparticles undergo in the human body relate to the cleavage of surface molecules in endosomes and the absorbance of other moieties. These physico-chemical properties of the nanoparticles and their ADME processes are taken advantage of during drug delivery approaches to maximize the efficiency of the therapeutic strategies (Nahire et al., 2013), while the metabolism of nanoparticles plays a main role in determining/ minimizing any toxic effects. However, in this area, our understanding is insufficient (Kagan et al., 2010). Nanoparticles can affect physiological and metabolic processes in vivo, due to the high electron density on their surface (Berry, 2013). For example, graphene oxide nanoparticles serve as hydrogen peroxide catalase systems (thanks to their high electron density on their surface) and transform H2O2 to OH in C. elegans (Zhang et al., 2012). These properties of nanoparticles have implications for the redox state of cellular systems. By interfering in redox-related reactions, nanoparticles can alter redox-sensitive pathways in cells and induce toxicity (Nel et al., 2006; Zhang et al., 2012). This means that nanoparticle use as a drug delivery system in the human body needs to be implemented with extreme caution due to potential side effects, which may be even more pronounced in disease states, such as diabetes or aging. What is important and still poorly explored is how the metabolism of nanoparticles potentiates their toxicity. For example, metallic nanoparticles do release metallic ions intracellularly upon metabolism, which may induce cellular toxicity (Derfus et al., 2004; Fukui et al., 2012). Thus, in the cases of metallic ions or quantum dots, it is difficult to assess the toxicity of nanoparticles. Improvements in their synthesis and possible chemical modifications can be useful ways to prevent ion release and the toxic effects of nanoparticles. The physicochemical properties of nanoparticles also affect their excretion from the human body. The excretion (partial or total) of nanoparticles from the human body occurs though various routes (Figure 1). For example, the hepatobiliary route is responsible for the excretion of liposomes (Alexis et al., 2008), and SWCNTs coated with polyethylene glycol are excreted primarily via feces and urine (Liu et al., 2008b). Nanoparticle size also determines their route of excretion, such that larger nanoparticles (e.g., >80 nm in diameter) that enter the liver and spleen are excreted in feces slowly (Alexis et al., 2008), while smaller ones (diameter < 10 nm) are cleared by the renal pathway (Longmire et al., 2008). Nanoparticles that are trapped by RES cells are degraded intracellularly in macrophages, while non-degradable nanoparticles stay in human tissue systems for an extended period of time (Chen et al., 2008; Zhao et al., 2011). In addition to their excretion in feces and urine, nanoparticles can be also be excreted in saliva, sweat, and breast milk (Li et al., 2010). in vitro studies in lower organisms, like C. elegans, are useful for the better understanding the ADME processes of nanoparticles. For example, the metabolic pathway that CsSeZnS and CdTe quantum dots nanoparticles undergo in C. elegans is significantly different from E. coli and involves the oxidation of selenium and its distribution to the intestines and reproductive system (Qu et al., 2011).
Table 1. Some of the main physicochemical properties of nanoparticles, as well as the exposure routes, and main findings.
In addition, it is important to understand how nanoparticles differ from their bulk counterparts. For example, the dissolution processes, which involve the release of metallic cations, are greater in NPs than in bulk materials and are influenced by a number of factors, such as the reduced size, the high surface-to-mass ratio, the high radii of curvature, and the corresponding low coordinated atoms at the surface of the NPs (Casals et al., 2012). This ability of NPs to release ions in an aqueous environment can have detrimental health effects, and specifically, silver ions released by NPs can even damage the membranes of sperm (Auffan et al., 2009). Moreover, studies demonstrate a correlation between NP toxicity and the ions released from Ag-NPs and ZnO-NPs compared to their bulk counter parts (Dibrov et al., 2002; Morones et al., 2005; Franklin et al., 2007). Additional novel properties that differentiate nanomaterials from bulk materials are typically related to size. Although, the size at which the material displays the different properties compared to its bulk material depends on the material in question (Kedziora et al., 2013). When looking from a biological point of view, matching the size of NPs with the naturally occurring functional units or components of living organisms would, thus, mostly likely make them more active in biological systems then their bulk counterparts, aiding in function or causing toxicity (Buzea et al., 2007).
In summary, nanoparticles exhibit a wide variety of physico-chemical properties and ADME properties too. This has implications on their absorption, and distribution to human body organs as well as their metabolism in vivo and their excretion. The latter process can be either complete or partial, implying that an amount of nanoparticles can be trapped in the human body for an extended period of time, which, thus, increases their potential harmful effects on human cells. Similar to small moieties used as drugs, nanoparticles enter the human body via various routes and undergo an ADME process, which is determined by their chemical properties and their size, but may be unique and radically different to that of small molecules (Zhang et al., 2014). Further studies on the ADME processes of nanoparticles are expected to enhance our understanding about their in vivo effects and their potential use as drug delivery systems. Table 2 summarizes the differences in ADME between nanoparticles and small molecules.
Table 2. The property differences in the adsorption, distribution, metabolism, and excretion between nanoparticles and small molecules.
NP-mediated toxicity is a major focus of many studies regarding the utilization of NPs but it is still not well understood. in vivo and in vitro studies reveal that NPs induce toxicity by increasing intracellular reactive oxygen species (ROS) levels and/or the levels of pro-inflammatory mediators. NP-induced ROS alters the homeostatic redox state of the host. NPs activate nuclear factor-kappa B (NF-κB) signaling by up-regulating the transcription of various pro-inflammatory genes, including tumor necrosis factor-α and interleukins (IL)-1, IL-6, and IL-8, which leads to oxidative stress, followed by severe DNA damage and apoptosis (Figure 2; Khanna et al., 2015).
Figure 2. This figure illustrates that nanotoxicity produced by overproduction of free radicals which induced oxidative stress. Oxidative stress causes lipid peroxidation, protein oxidation and DNA damages, these all together potentiate inflammatory response by implying variety of inflammatory pathways. On the other hand, antioxidant defense encounter the production of oxidative stress and ameliorate reproductive nanotoxicity of animal models. Modified from Khanna et al. (2015).
One example of this type of toxicity occurs with nanosilver particles, which enter the cell via diffusion or endocytosis, leading to mitochondrial dysfunction and the generation of ROS, which damages proteins and nucleic acids inside the cell and, finally, inhibits cell proliferation (McShan et al., 2014). However, the molecular mechanisms underlying nanotoxicity are not entirely understood. Although, because it is well established that oxidative stress is a key determinant of NP-induced injury, the characterization the ROS response resulting from NPs is necessary. A better physico-chemical characterization and understanding of the multiple signaling cascades activated by NP-induced ROS, will contribute to NP-induced injury studies (Manke et al., 2013). Indeed, as we described below, there is evidence that NP-induced toxicity via ROS is a major factor related to how NPs affect the reproduction system in animal models.
Reproductive toxicity refers to detrimental effects on any stage of reproduction and pregnancy in the human reproductive cycle, such as an interruption in the development of healthy embryos among child bearing age females (Adler et al., 2010). The side effects that impact the offspring at any stage of life as a consequence of parental exposure are characterized as developmental toxicity (Rogers and Kavlock, 1998). The negative effects of numerous natural contaminants, such as pollutants, on the development and reproduction of humans and animals (Slama et al., 2008), are shown in Figure 3. NPs also have negative effects on reproductive organ function, physiological structure, germ cells and fertility, as discussed below.
Figure 3. Environmental pollutants and nanoparticles have adverse effects on human reproduction. IUGR, intrauterine growth retardation; LBW, low birth weight; PTD, preterm delivery.
To study the effect of toxic NPs on biological systems, researchers have utilized numerous in vitro toxicity models. However, the problem with these assays is that NPs interfere with either the detection system or the assay materials, leading to conflicting or inconsistent results and thus, toxicological models by focusing more on in vivo studies (Bahadar et al., 2016). For the purpose of this review, we focused on the animal models that have been used to study various aspects of NP-induced toxicity and its affect on reproduction. Past research reveals the most frequently utilized animal models include rats and mice, but rabbit and C. elegans have also been a focus of these studies (Bahadar et al., 2016; Kong et al., 2016). While animal models reduce the assay interference problems experienced with in vitro models and provide a better study model, as described later on, there is still a need to improve the manner in which animal studies are conducted, as the precise mechanism of NP-induced reproductive toxicity is still lacking.
The reproductive structure of female animals involves the reproductive organs, including the ovary, oviducts, uterus, vagina, external genitalia, and the hypothalamic–pituitary–gonadal axis. Multiple feedback mechanisms between different components of this axis are required to carry out the normal functions of reproduction (Apter, 1997; Figure 4). The accumulation of NPs is observed in female reproductive organs in animal studies, but information on whether this results in toxicity is lacking. Studies differ in terms of the type, size, dosage and length of exposure of the NP, technique of administration, and animal model used. These studies tend to focus the impact on the uterus and ovaries.
Figure 4. The female reproductive normal operation, showing positive and negative response within the hypothalamic-pituitary ovarian axis. Stands for positive response.
Stands for negative response.
There is some evidence to suggest that smaller sized NPs are more likely to accumulate in the uterus than larger NPs. Similarly, the solubility factor also plays a major role in nanotoxicity. One recent study in rats, indicated that 1.4 nm gold NPs (AuNPs), administered intravenously at 5 μg per rat, accumulated at the uterine wall at concentrations that were two levels of magnitude higher than for 18 nm (3 μg/rat) or 80 nm (27 μg/rat) NPs (Semmler-Behnke et al., 2014). While the detected levels of AuNPs were higher in pregnant versus non-pregnant uteri, and this was proportional to the increased size and weight of the pregnant uterus. Similarly, iron oxide magnetic NPs (IOMNs) of 10 nm were recently shown to enter the mouse uterus more readily than larger NPs of 20, 30, and 40 nm (Yang et al., 2015). Information on whether these or other types of NPs cause toxicity in the uterus is lacking, However one recent study demonstrated sex-differences in the effects of gold NPs on mice livers and kidneys, having no toxicity on the reproductive system induced by 4.4 and 22.5, 29.3, or 36.1 nm particles (4,000 μg/kg), when administered by an intraperitoneal injection to either male or female mice (Chen et al., 2013).
The nature of the NP is undoubtedly of significance in terms of its toxicity to female reproductive organs. For example, cadmium, which is also known as metalloestrogen, has an impact at several levels of female reproduction. Inhalation of cadmium oxide (CdO) NPs of either 11 or 15.3 nm diameter, in one study, resulted in the dose-independent accumulation of Cd in the uterus (Blum et al., 2012). However, a daily exposure to a higher dose of CdO NPs (230 μg/m3) resulted in an increasing uterine weight in pregnant mice when compared to a lower dose (100 μg/m3) or no CdO. This higher dose of CdO NPs was delivered at ~1 μg CdO per mouse per day, whereas at the current highest allowed levels for occupational exposure, a worker in the cadmium industry might inhale 14 μg/day. This would be a considerably lower concentration, given the relative weights of humans and mice. Nevertheless, the readiness with which NPs can be distributed to secondary organs, including the uterus makes the results of studies like these worth considering when addressing safe practice in industry and any potential medical applications (Blum et al., 2012). In particular, great caution should be exercised in exposing vulnerable populations such as pregnant women to potentially toxic NPs, including CdO NPs.
In terms of other female reproductive organs, there is evidence of the accumulation of NPs in these areas. However, there is a lack of overall clarity on their potential toxicity. In the ovaries, for example, a short-term oral administration of titanium dioxide (TiO2) nanoparticles at 0, 1, or 2 mg/kg body weight per day in rats, results in increases in the total Ti tissue levels without general toxicity (Tassinari et al., 2014). Similarly, the acute treatment of adult mice via a single oral gavage of a high concentration (5 g/kg body weight) of 25 and 80 nm TiO2 NPs, results in no evidence of abnormal pathological changes in the ovaries over a 2 week period (Wang et al., 2007). In contrast a, long-term intragastric treatment, over 90 days, with a TiO2 nanoparticle at a lower concentration of 10 mg/kg results in ovarian damage in adult mice and is accompanied by alterations in the expression of genes associated with estrogen and progesterone synthesis and metabolism (Gao et al., 2012). An in vitro study on prenatal follicles dissected from rat ovaries also suggests potential ovarian toxicity with high TiO2 nanoparticle doses (Juan et al., 2009). In this study, 25 nm TiO2 nanoparticles dose-dependently reduced follicle development and oocyte maturation, at rather high concentrations >25 μg/ml (Juan et al., 2009). The in vitro and in vivo effects of exposure to TiO2 nanoparticles in the ovaries of rats and mice is documented in three different studies (Wang et al., 2007; Juan et al., 2009; Gao et al., 2012). It is clear that studies are needed to definitively distinguish between low and high TiO2 dosage and acute and long-term exposure, and to apply the information obtained for assessing safer levels of exposure for humans and potential unintended effects on the ovaries, for example, the use of TiO2 in sunscreens, cosmetics and prosthetic implants, and its potential use in the treatment of acne and other dermatological conditions should be assessed (Wiesenthal et al., 2011; Shi et al., 2013).
AuNPs can also target the ovaries, resulting in their development as potential anticancer drug carriers in ovarian cancer (Kafshdooz et al., 2016). Nickel NPs, however, when administered to adult rats, decrease ovarian weight coefficients, increase apoptosis and the infiltration of eosinophils and inflammatory cells into ovaries, and induce vascular dilation and congestion (Kong et al., 2014). The NP size in this study was relatively large, with an average diameter of 90 nm, while the dosing at 15 or 45 mg/kg body weight was also relatively high (Kong et al., 2014). Again, therefore, there is an urgent need to address this question of likely exposure and the relative impact of different types and sizes of nanoparticles, particularly in the context of more vulnerable populations, such as pregnant women and their fetuses.
NPs are also implicated in other aspects of female reproduction beyond the physical structures, with potential implications for fertility and development of healthy pregnancies. These include alterations of ovarian gene expression, such as for genes associated with many pathways, including steroidogenesis, apoptosis, and the acute inflammatory response.
In females, the main female sex hormones are estrogen and progesterone, which, in humans, are mainly synthesized in the ovaries or in the placenta during pregnancy. There is some evidence to suggest that different NPs can alter the expression of genes encoding proteins involved in steroidogenesis, including ovarian genes crucial to the synthesis of estrogen and/or progesterone. For example, as mentioned above, long-term intragastric treatment of adult mice with TiO2 nanoparticles (5–6 nm) at 10 mg/kg, as well as causing ovarian damage, also resulted in alterations in the expression of genes in the pathways of estrogen and progesterone synthesis and metabolism, including cytochrome P450 17A1 (Cyp17a1) and aldo-keto reductase family 1, member C18 (Akr1c18) (Gao et al., 2012). Cyp17a1 is an enzyme that converts both pregnenolone and progesterone to their 17-hydroxy forms and further converts these forms to DHEA and androstenedione, respectively, thus placing it at the center of the progesterone metabolism and estrogen biosynthesis pathways. The expression of the Cyp17a1 gene, which encodes cytochrome P450 17A1, is increased upon long-term TiO2 NP exposure, feeding into an increase in estradiol (Gao et al., 2012). The expression of the Akrc18 gene, which encodes aldo-keto reductase family 1 member C18 (20-alpha-hydroxysteroid dehydrogenase; 20-alpha-HSD), is also increased. This is consistent with observed reductions in progesterone levels in TiO2 NP-treated mice, as 20-alpha-HSD is associated with progesterone metabolism (Gao et al., 2012). In this study, it was also demonstrated that long-term, high dosage TiO2 nanoparticle treatment resulted in an alteration of the expression of apoptosis-related genes, as well as genes associated with inflammatory and immune responses, cell proliferation, ion transport, and oxidative stress (Gao et al., 2012). Thus, changes in sex steroid levels, ovarian oxidative stress and inflammation, and increased apoptosis in the ovaries may all contribute to the ovarian damage, decreased fertility and decreased pregnancy rate observed in this study, in response to TiO2 nanoparticles over the long-term (Gao et al., 2012).
The impact of excessive TiO2 on inflammation and apoptosis-associated genes is reminiscent of a recent in vitro and in vivo mouse study using high levels of silver NPs (AgNPs; Kim et al., 2004). An in vitro treatment of mouse ovarian follicle granulosa cells resulted in increased mitochondrial-mediated apoptosis, while the in vivo expression of pro-inflammatory cytokines was increased, along with the loss of germ cells (Han et al., 2016). Thus, it appears that excessive exposure to either TiO2 or Ag NPs has potentially catastrophic consequences for the induction of apoptosis and/or acute inflammatory responses in the ovaries. What remains unclear is, how likely it is that human females would be exposed to such high levels at average day-to-day exposure levels. These findings are perhaps more likely to be relevant in the context of workers within industries or research projects associated with TiO2 or Ag NPs, or to those subject to potential therapeutic uses of these nanoparticles.
In contrast to long-term intragastric TiO2-induced increases in estradiol, long-term daily exposure of mice to inhaled CdO NPs (230 μg/m3) resulted in an ~50% reduced level of 17β-estradiol and increased mRNA for the estrogen receptors Erα and ERβ in the uterus but not in the ovaries and was associated with increased uterine weight in pregnant mice. The capacity of Cd to act as an estrogen mimetic may be of relevance in this context. Cd may act as an ER agonist and interfere with implantation. The reduced levels of implantation observed among mice treated with 230 μg/m3 CdO NPs is consistent with this (Blum et al., 2012).
Other studies have also addressed the potential contributions of different types of NPs to dysfunctions in female steroidogenesis. Diesel exhaust (DE) particles, for example, contain NPs, which may be implicated in reproductive toxicity. One study showed that NP-rich diesel exhaust (NP-DE) administered to pregnant rats from days 1–19 of gestation reduced the mRNA expression of the enzymes cytochrome P450 side-chain cleavage enzyme and 3β-hydroxysteroid dehydrogenase, which are key to the generation of progesterone from cholesterol during luteal steroidogenesis in rats, and also the luteinizing hormone (LH) receptor (Chun-Mei et al., 2013). However, filtered DE (F-DE) had comparable effects on these parameters, as well as inducing increased levels of estradiol-17β in serum, suggesting that NPs may not be major contributors to DE-induced steroidogenesis dysfunction in the rat (Chun-Mei et al., 2013).
Thus, the nature of the NP, the dose received, and, potentially, the route of entry can result in contrasting long-term effects. Although, the overall consequences may still be reproductive toxicity. The dose and duration of the exposure also impacts the eventual distribution of the NPs to the different organs and how far-reaching their effects might be. Further consequences can arise in pregnant females if the NPs make their way across the placenta, with potentially toxic consequences for the developing fetus.
The placenta produces hormones that regulate the maternal/fetal exchange and maintain gestation and embryonic growth, as well as protect the fetus from potentially harmful agents. However, the placental barrier is, out of necessity, permissive to the passage of, for example, nutrients, antibodies, and hormones, and is not completely impervious to all toxic agents, including environmental pollutants, secondary cigarette smoke, drugs, or disease-causing pathogens, with potentially deleterious consequences for the fetus (Ananth et al., 1996; Olivero et al., 1997; Perera et al., 2003; Menon et al., 2011; Robbins and Bakardjiev, 2012; Tong et al., 2013; Whidbey et al., 2013; Adebambo et al., 2015). Thus, developmental toxicity may be caused by transplacental transmission from the mother to the offspring. The small size of nanoparticles and their ready distribution to reproductive organs makes them prime candidates to breach the placental barrier. To explore this issue, different placental models have been used, including rodent and zebrafish (Danio rerio) embryogenesis models and perfusion models of the human placenta. Such studies confirm that NPs, such as Au, TiO2, SiO2, carbon (C), and QD NPs can readily pass through the placental barrier (Figure 5; Semmler-Behnke et al., 2007; Takeda et al., 2009; Chu et al., 2010; Sumner et al., 2010; Refuerzo et al., 2011; Yamashita et al., 2011). The effects of these NPs are size-dependent, and NPs smaller than 240 nm diameter have transplacental activity in an ex vivo human placental perfusion model (Wick et al., 2010). Therefore, the placental penetration ability of NPs may rely on their type, composition, and size. However, as with studies on reproductive organs and gene expression, there is wide variability in the dose and duration of NP administration. For example, different routes of administration make it difficult to directly compare between studies.
Figure 5. Physiological barriers and transplacental penetration of nanoparticles. a, 198Gold-nanoparticles; b, black carbons; c, titanium oxide; d, single-walled carbon nanotubes; e, platinum, f, multi-walled carbon nanotubes; g, cadmium telluride/cadmium sulfide quantum dots; h, diesel exhaust; i, sodium chloride-modified silica nanoparticles; j, silicon dioxide; k, silica-coated magnetite nanoparticles (rhodamine B isothiocyanate); l, metal-free polymethyl methacrylate; m, carbon; I, intravenous; II, intranasal; III, inhalation; IV, subcutaneous; V, oral exposure; VI, by gavage; VII, intraperitoneal; VIII, intragastric. Enter fetus (Semmler-Behnke et al., 2007).
Collagen in offspring (Takahashi and Matsuoka, 1981), interrupt male reproductive system and decrease DSP (Kubo-Irie et al., 2011).
Toxic effects in embryo (Wang et al., 2007), irregular expression of genes in offspring livers (Takahashi et al., 2010), inhibition in female offspring (Fujimoto et al., 2005).
Skeletal defects and phenotypic imperfections (Snyder et al., 2015), different morphological abnormalities (Sugamata et al., 2006).
Increased mortality during lactation period and decrease growth of pups (Meng et al., 2010).
Crossed placental barrier (Jackson et al., 2011).
Toxic effects in embryo (Mattison et al., 1990).
Oligospermia (Hougaard et al., 2010). Hypersensitivity in neonates and inflammation in offspring (Jackson et al., 2013), interrupt development of offspring (Kyjovska et al., 2013), disruption of sertoli and spermatozoa cells, decreased daily sperm production in offspring (Pietroiusti et al., 2011), reduces size of vesicular and prostate gland, decreased concentrations of different hormones and loss of sperm (Hamada et al., 2003).
Crossed blood-testis barrier (Philbrook et al., 2011).
Crossed blood-testis barrier (Yoshida et al., 2009).
Crossed blood-testis barrier (Bai et al., 2010).
Crossed blood-testis barrier (Kashiwada, 2006).
Oligospermia (Kubo-Irie et al., 2011).
A study by Yamashita et al. (2011) was designed to assess the transplacental transfer of silica (Si) (70 nm) and TiO2 NPs (35 nm) following an intravenous injection into the tail vein of pregnant mice for 2 consecutive days. The results of the study indicated that there was transfer and attachment of the particles to the placental trophoblasts. (Yamashita et al., 2011) This resulted in a 20–30% reduction in uterine weight, increased fetal resorption rate, and smaller fetuses at gestational days 16 and 17, all resulting from placental dysfunction. Notably, fullerenes (0.7 nm) and Si (300 and 1,000 nm) NPs did not enter the placental trophoblast or cause adverse outcomes. Additionally, such complications were abolished by surface modification of the Si NPs with amine or carboxyl groups. However, the NP doses used in this study were very high, at 800 μg/mouse, which may represent an effective “overload.” Such doses are representative of those used in preclinical studies in animal models to test silica particle drug delivery effectiveness, but would far exceed what would be likely to result, for example, from the topical application of TiO2 NP-containing sunscreens or cosmetics (Yamashita et al., 2011). The lower doses used in this study (200 or 400 μg/mouse) did not exert the fetal consequences observed at the 800 μg/mouse dose. Another more recent study aimed to determin the mechanisms used by the NPs to cross the placenta, employing the AuNPs at much lower concentrations (Semmler-Behnke et al., 2014). The NPs, at sizes of 1.4 nm (5 μg/rat), 18 nm (3 μg/rat), and 80 nm (27 μg/rat), were intravenously injected into pregnant and non-pregnant rats. While all three sizes of NPs entered the placenta and amniotic fluid, the smallest NPs were present at orders of magnitude higher concentrations than the larger particles, which was also the case for the uterine wall. The translocation appeared to occur via the transtrophoblastic channels and/or by transcellular processes. However, no NPs were observed in the fetuses. Another study using 20 and 50 nm AuNPs in mice similarly reported the presence of Au in the placenta but not in fetal organs, with no evidence of toxicity to the placenta or fetus (Rattanapinyopituk et al., 2014). This study did report increased endocytic vesicles in syncytiotrophoblasts and fetal endothelial cells in the maternal-fetal barrier, and proposed a role for clathrin- and caveolin-mediated endocytosis in the AuNPs crossing of the placenta (Rattanapinyopituk et al., 2014). While a different type of NP was used in the studies by Semmler-Behnke et al. (2014) and Rattanapinyopituk et al. (2014) compared to that of Yamashita et al. (2011) the difference in the distribution of NPs to the fetus may also have been due to the very high concentrations of NPs used in the Yamashita study. This might result in open pathways that would not normally be functional, a phenomenon that was previously demonstrated in lung tissue when NP overload occurs (Yamashita et al., 2011; Semmler-Behnke et al., 2014).
The preferential tendency of smaller versus larger NPs to cross the placenta to the fetus has, in fact, been recognized in animal models for some time. In one study, 20 μg of radioactively labeled Au-colloid particles were injected into a Wistar rat tail vein, and 5 nm NPs were reported in fetuses at 19 gestational days at a higher transfer rate than the 30 nm NPs (Semmler-Behnke et al., 2014). NPs of both size were found at 100- to 300-fold higher levels in the placenta and fetal membrane as opposed to the fetus itself, suggesting that the NPs may have crossed the yolk sac and chorioallantoic placenta to penetrate the fetus (Takahashi and Matsuoka, 1981). However, in another similar study in mice, no transfer was observed 1, 4, and 24 h after intraperitoneal or intravenous injections of AuNPs (2 and 40 nm) in pregnant C57BL/6 mice (Sadauskas et al., 2007). Inconsistencies in the findings between studies add to the overall lack of consensus in the field. The utilization of different NP preparation protocols in various laboratories, for example, may contribute to these inconsistencies.
Other types of NPs also follow the pattern of more transfer of smaller particles. For example, fluorescent QDs have potential for use in diagnostic and biomedical imaging applications. However, in a study in pregnant mice, Cd telluride QDs (CdTe/CdS QDs) were dose-dependently transferred across the placenta to fetuses, with smaller QDs being more easily transferred than larger QDs (Chu et al., 2010). The transfer was reduced by coating with an inorganic silica shell or with polyethylene glycol (PEG), but some transfer still occurred. Such studies are important, as they indicate how the clinical utility of some NPs would be potentially limited in pregnancy.
A confounding issue with studies in rat or mouse placenta is the difficulty of extrapolating the results to humans, as the human placental physiology and anatomy are unique (Wick et al., 2010). For example, the rat and mouse placenta have three trophoblastic layers on the maternal side compared to the one layer in the human placenta (Wick et al., 2010; Rattanapinyopituk et al., 2014). This suggests that higher concentrations of NPs may be likely to penetrate the human compared to rodent placentas, with, potentially, a greater exposure to the fetus at lower NP doses. In one study, an ex vivo human placental perfusion model was used to attempt to assess the transfer of NPs across the human placenta specifically (Wick et al., 2010). Fluorescent polystyrene beads of diameters 50, 80, 240, and 500 nm were perfused at a concentration of 25 μg/ml, which is a rather high concentration that might be expected to resemble concentrations used in clinical applications, such as magnetic fluid hyperthermia, as opposed to regular environmental exposure (Thiesen and Jordan, 2008; Wick et al., 2010). A size-dependent translocation of the NPs across the placenta was observed, with NPs larger than 240 nm barely passed the placental barrier. The NP translocation had no observable impact on placental viability or structure. The critical nature of the NP composition is highlighted by comparing the results of this study to those of a similar study carried out using PEG-coated AuNPs in an ex vivo human placental perfusion model (Myllynen et al., 2008). In this case, 10 or 30 nm NPs did not cross the placenta into the fetal circulation. The limitations of ex vivo human placental perfusion models should be noted, i.e., that they are limited to a time period of a few hours due to subsequent tissue degradation, and therefore, they cannot be used to determine what happens over a period of chronic NP exposure. Furthermore, they give a picture of the placenta only at the final pregnancy stage, when the barrier between the maternal and fetal compartments has thinned considerably. Earlier in the pregnancy, the perfusion rates may be lower (Wick et al., 2010). Nevertheless, the results of these types of studies highlight the necessity of individually considering different types of NPs and their capacity to cross the placenta at different gestational stages. Indeed, returning to animal studies, the findings from one study in which AuNPs were injected into the tail vein of mice at concentrations between 0.9 and 7.2 mg/kg body weight suggest that the extent of fetal exposure is dependent on the gestational stage, including the stage of placental maturation (Yang et al., 2012). In fact, it was during early gestational stages, prior to 11.5 days, that the NPs primarily accumulated in the fetal tissues, whereas the extra-embryonic tissue accumulation increased after this point. The surface composition of the NP is also revealed as crucial in this study, as both ferritin- and PEG-modified nanoparticles accumulated in both fetal and extra-embryonic tissues at a greater rate than citrate-capped nanoparticles (Yang et al., 2012).
The placenta grows after uterine implantation of the blastocyst into the maternal endometrium, and its cellular composition and its structure changes throughout pregnancy (Wick et al., 2010; Yang et al., 2012). Thus, as studies suggest, NPs may affect the placenta and fetus in an exposure time-dependent manner and the defensive capacity of the embryo against exogenous toxicants may shift. A study on murine pregnancy (Yang et al., 2012) suggests extra fetal vulnerability at the early stages of pregnancy. However, the thinning of the stromal cell layer between the syncytiotrophoblast layers facing the maternal compartment and the endothelial layer facing the fetal compartment later in human pregnancy should not be overlooked, as it may facilitate a later vulnerability of the fetus to any potentially toxic NP effects (Wick et al., 2010).
There is considerable evidence to suggest the potentially toxic effects of various types of NPs on the fetus, which were described in the preceding section, with deleterious consequences for fetal development. Yamashita et al. (2011), for example, observed the accumulation of TiO2 and SiO2 nanoparticles in rat fetuses, with an associated toxicity as evidenced by an increased fetal resorption rate, and smaller fetuses at gestational days 16 and 17, at a high IV dosage of 800 μg per mouse but not at lower dosages (Yamashita et al., 2011). Other studies on similarly sized AuNPs, by contrast, did not lead to the accumulation of gold in rat fetuses or any evidence of fetal toxicity, despite evidence of nanoparticle transport across the placenta into the uterus (Rattanapinyopituk et al., 2014; Semmler-Behnke et al., 2014). In these studies, the dosages of the NPs were 100- to 1,000-fold less than the TiO2 and SiO2 dosages employed by Yamashita et al. (2011). These types of anomalies highlight the difficulties in determining how much of a threat to reproductive and fetal health different types of NPs really represent, given the inherent uncertainties in determining what levels individuals are likely to be exposed to, including the relativity vulnerable people, for example, pregnant women and their fetuses.
Nevertheless, the preponderance of NPs and their biocompatibility mean that studies suggesting the potential fetal toxicity of certain NPs cannot be ignored, especially in the context of the therapeutic use of NPs in pregnant women or potentially high occupational exposure levels. Increased fetal absorption rates observed not only with high dose TiO2 or SiO2 (Yamashita et al., 2011), but also, for example, with DE elements (Fujimoto et al., 2005). Carbon-14-labeled C60 (((14) C(U))C60), for example, when administered via tail vein injection at 200–300 mg/kg to pregnant or lactating rats, crosses the placenta or is transferred via the dam's milk, resulting in increased oxidative stress in the female pups of the exposed dams (Sumner et al., 2010; Snyder et al., 2015). Meanwhile, the embryonic undeveloped cell line ES-D3 is used to investigate the developmental toxicity of AuNPs and cobalt ferrite (CoFe2O4) in vitro (Meng et al., 2010). AuNPs covered with hyaluronic acid and CoFe2O4 NPs covered with silanes exhibit toxic properties.
In animal models, the brain and nervous system are directly affected by prenatal exposure to NPs. A subcutaneous injection of TiO2 NPs of a diameter <300 nm administered at 100 μg/animal at gestational days 3, 7, 10, and 14 (400 μg total), resulted in the entry of NPs into the brain of the offspring of the exposed pregnant female mice, resulting in blood vessel stenosis in the hippocampus and cerebral cortex (Takeda et al., 2009). A similar experimental set-up in which 100 μg of TiO2 (25–70 nm) was given to pregnant ICR mice at 6, 9, 12, 15, and 18 gestational days, giving a relatively high 500 μg total dose, resulted in increased levels of dopamine (DA) and its metabolites in the prefrontal cortex and neostriatum metabolites (Takahashi et al., 2010). Moreover, the use of the same type and size of TiO2 NPs on days 6, 9, 12, and 15 (400 μg total) in pregnant mice revealed that in the brain tissue of the male offspring, the striatum at embryonic day (ED) 16 and post-natal day (PND) 7 and 14, the olfactory bulb at PND 2 and 14 and the cerebral cortex at PND 7, 14, and 21, were enriched in the brain gene expression changes in the regions closely associated with the DA system (Umezawa et al., 2012).
DE similarly impacts the central nervous system of the offspring of the pregnant mice, with a reduced locomotion and a decreased turnover of DA in the striatum and nucleus accumbens (Yokota et al., 2009), while apoptosis in the brain tissues is reported after 2–16 days post-coitus with a daily inhalation of DE at doses of 0.3, 1, and 3.0 particles/m3 in ICR mice (Sugamata et al., 2006). Meanwhile, an intratracheal administration to pregnant C57BL/6BomTac mice at gestational days 7, 10, 15, and 18, at 268 μg/animal but not at lower concentrations, induces changes in innate behavioral patterns of female offspring (Jackson et al., 2011). These studies highlight the potential vulnerability of the fetal brain to the toxicity of a variety of different types of NPs, in advance of the formation of a robust blood-brain barrier.
NP exposure by inhalation is one of the most likely environmental exposure routes causing damage to fetal organs. The overall pathological aspects associated with these deleterious effects on the offspring still need to be deciphered. However, it is presumed that nanoparticle inhalation is associated with molecular changes in various molecular moieties involved in the developmental processes. There are several reports describing the deleterious effects of nanoparticles on offspring in animal models (Hougaard et al., 2008, 2010, 2013; Jackson et al., 2011, 2012a,b, 2013). An elaborate review on the developmental toxicity of inhaled nanoparticles warns about the toxicity of nanoparticles and suggests that a significant amount of groundwork is warranted for testing a strategy to be established on a sound scientific basis (Hougaard et al., 2015). Intriguingly, in an open field test, female offspring that were prenatally exposed to 268 ug Printex 90/animal displayed an altered habituation pattern (Jackson et al., 2011). Similarly, an intranasal instillation of pregnant ICR mice by carbon black (CB), an element of DE that can be absorbed through the airways, at a total dose of 2 mg/kg at gestational days 5 and 9, results in the increased expression of renal VIII collagen and decreased type I collagen mRNA expression in the kidneys of 12-week old offspring (Umezawa et al., 2011). This increase in nonfibrillar short-chain collagen was similar to what is observed in tubulointerstitial fibrosis in diabetic nephropathy. Although, intranasal instillation with a single bolus is not directly reflective of exposure by inhalation, it does suggest the potentially toxic consequences for offspring if there is exposure to carbon black at sufficiently high levels during pregnancy (Umezawa et al., 2011).
CB and TiO2 are actually considered immunologically “inert” components of DE in many immunotoxicity studies (Fedulov et al., 2008). However, a study on the intranasal exposure of pregnant versus non-pregnant mice to suspensions (50 μg/mouse) of DE particles or “inert” TiO2 nanoparticles showed that pregnancy increased inflammatory responses, including the levels of pro-inflammatory cytokines, to such NPs (Komatsu et al., 2008). Exposure to either NP suspension increased the chances of allergic responsiveness in the offspring of non-allergic mothers, with increased airway hyperresponsiveness and increased pulmonary inflammation, as indicated by eosinophilic pulmonary infiltration and increased bronchoalveolar lavage (BAL) levels of eosinophils (Komatsu et al., 2008). Meanwhile, exposure to TiO2 NP (1 h/day, 42 mg/m3) through inhalation pregnant C57BL/6 mice on gestational days 8-18 resulted in irregular hepatic gene expression of components in the retinoic acid signaling pathway in female offspring (Jackson et al., 2013). Other sex-specific changes induced by a daily inhalation of TiO2 nanoparticles (21 nm) coated with polyalcohol at a dose of 42 mg/m3 from gestational days 8–18 for 1 h/day included an enhancement of the prepulse inhibition in female offspring in pregnant C57BL/6BomTac mice (Hougaard et al., 2010).
It was already mentioned that inhaled CdTe/CdS quantum dots, at a dose of 230 μg/m3, released Cd ions in pregnant mice, resulting in altered endocrine functions, including reduced 17β-estradiol levels and increased uterus estrogen receptor mRNA (Blum et al., 2012). This has a deleterious effect on fetal attachment, disrupts the attached blastocysts and reduces fetal length and neonatal growth (Blum et al., 2012). However, the mediating effects of this mechanism of action are not yet demonstrated. The potentially toxic effects of NPs extend further to the reproductive function of the offspring, thereby providing a risk to generations following those directly exposed in utero.
Nanoparticle administration is associated with toxic effects on fetal development and a compromised fertility (Tsuchiya et al., 1996). Additionally, NPs may cause changes in embryogenesis and anomalies in the fetal reproductive system. Subcutaneous TiO2 NPs (<300 nm diameter at 100 μg/animal at gestational days 3, 7, 10, and 14; 400 μg total), results in the entry of NPs to testicular Leydig cells, sertoli cells, and spermatids in male offspring aged 4 days and 6 weeks old, as detected by electron microscopy (Takeda et al., 2009). It was also reported that nanoparticles, like DE particulates and TiO2, transiently suppress the proliferation of Leydig cells (Hong et al., 2016). In the male reproductive system, sertoli cells contribute to spermatogenesis. Interestingly, damage to these cells ultimately impacts sperm production. Exposing primary isolated sertoli cells from the mice to TiO2 results in apoptosis through lactate dehydrogenase release (Ritz et al., 2011). In a 6-week old mice, testicular morphology is altered and daily sperm production (DSP) is reduced (Takeda et al., 2009). In another study, along similar lines, maternal exposure to diesel exhaust particles enhances mutations in male germlines during development (Boisen et al., 2013). As far as the female murine germ cells are concerned, nanosized carbon black (Printex90) NanoTIO2 does not induce mutations in female murine germ cells (Boisen et al., 2012; Zhao et al., 2013). However, although this is good news, it still needs to be confirmed by evaluating the parallel effects on both males and females, as another study showed that injury to the ovaries associated with nano-TiO2 exposure (Di Bona et al., 2015). High doses (100 mg/kg) of intra-peritoneal positively-or negatively charged Fe2O3-NPs to pregnant mice also has consequences for the reproductive health of both female and male offspring (Tsukue et al., 2002). Both positively and negatively charged NPs induce morphological alterations of the uteri, while positively charged NPs also altered the morphology of the testes in the surviving offspring. This has far-reaching consequences, as positively-charged NPs when given after gestational day 10, cause both short-term fetal loss and also increase long-term fetal loss in second generation mating (Tsukue et al., 2002).
The reproductive development of offspring is also interrupted by DE, which contains fine and ultrafine particles (Ono et al., 2007; Kubo-Irie et al., 2011). Pre- and post-natal exposure of ICR (imprinting control region) mice to DE, at a concentration of 0.17 mg/m3, results in disruption of the sertoli and spermatozoa cells and decreases daily the DSP in male offspring (Li et al., 2009). However, the contribution of NPs to the overall reproductive toxicity of DE is more questionable. The administration of NP-rich DE compared to filtered DE (F-DE) to pregnant F344 rats, for example, between gestational days 1–19 results in comparable reductions of the relative weights of the seminal vesicle and prostate to body weight, and similar decreases in concentrations of different hormones, including serum levels of testosterone, progesterone, corticosterone, and follicle stimulating hormone and testicular levels of steroidogenic acute regulatory protein and 17β-hydroxysteroid dehydrogenase (Watanabe, 2005). Similarly, prenatal DE exposure from gestational day 7 to delivery, results in reduced levels of spermatids and sertoli cells, and, hence, reduces the daily sperm levels in the offspring, and the similarity of the results in low- and high-dose particulate exposures suggests that the toxicity is due to the DE gaseous element (Philbrook et al., 2011). Follicle stimulating hormone (FSH), receptors and mRNA expression, in contrast, increases in response to NP-rich DE only (Watanabe, 2005). Thus, it is important to exercise caution when distinguishing between the effects caused by NPs and those that are caused by other elements of DE.
Thus, the results obtained animal models with NPs, such as TiO2 and positively versus negatively-charged Fe2O3, nevertheless suggest that long-term effects on fertility and reproductive function in offspring of NP-exposed pregnant females cannot be ruled out. This adds another reason for the urgent clarification of the relative effects of different types of NPs, the importance of surface modification, and the definition of safe doses.
Nanoparticle administration is also associated with toxic effects on fetal morphological development and organogenesis at different gestational periods (Tsuchiya et al., 1996). An oral administration of TiO2 NPs in a high single dose of 100 or 1,000 mg/kg to pregnant dams causes a significant increase in fetal deformities and mortality (Park et al., 2010). It would be of interest to determine what the effects would be over a range of doses in order to more accurately reflect the likely range of TiO2 doses arising from environmental, occupational and therapeutic exposures. In other studies, the mortality of pups increased during the period of lactation and decreased growth without deformities, are detected after an oral administration of platinum (Pt) NPs at concentrations of 0.25, 0.5, and 1 mg/kg, 14 days before and 4 days after mating in ICR mice(Pietroiusti et al., 2011).
Meanwhile, the administration of SWCNTs (1–2 nm in diameter, 5–30 μm in length) at doses of 10 ng to 30 μg/mouse to pregnant CD-1 mice, 5.5 days after implantation, also results in skeletal defects, including divided cervical vertebra and a reduced formation of new bones in the sternum, fingers, and toes, and phenotypic imperfections (Lim et al., 2011). The lowest effective dose was determined at 100 ng. Fetal malformations are associated with increased ROS in both the placenta and fetus, and oxidized SWCNTs cause more fetal anomalies and are rated more embryotoxic than pristine SWCNTs (Lim et al., 2011). In contrast, the administration of MWCNTs by gavage, at doses up to 1,000 mg/kg/day into pregnant rats did not induce fetotoxic effects, in fetuses delivered by Cesarean section at gestational day 20 (Kyjovska et al., 2013). Thus, it appears that MWCNTs may have a less fetotoxic impact than SWCNTs, and particularly oxidized SWCNTs (Lim et al., 2011; Kyjovska et al., 2013). Clarity on this issue requires studies in which the effects of the different carbon nanotubes (CNTs) are compared directly within a single model.
Exposure to nanoparticles also affects the male reproductive system, including an impact on spermatogenesis from the point where it begins in the seminiferous tubules of the testes. Furthermore, NP exposure as reduces sperm production (Boisen et al., 2013). However, the impact varies from species to species (Gao et al., 2013). Decreased sperm production is associated with several molecular changes by altering the overall expression of genes involved in spermatogenesis (Lan and Yang, 2012; Hong et al., 2015a,b). A review on nanoparticles on spermatogenesis suggests precautionary measures in nanomedicines and understanding the passage of nanoparticles through the blood-testes barrier (Yoshida et al., 2010). Issues can begin from the fetal stage if the NPs are transmitted in utero. For example, as previously mentioned, a subcutaneous administration of 400 μg total of TiO2 NPs (<300 nm diameter) to pregnant mice facilitates NP entry to Sertoli cells and spermatids into the seminiferous tubules and the adjacent Leydig cells, in male offspring aged 4 days and 6 weeks old (Takeda et al., 2009). This is accompanied by a reduced sertoli cell number, altered testicular morphology, with scattered and interrupted seminiferous tubules, and a reduced DSP (Takeda et al., 2009). Epididymal sperm motility is also altered in 6-week old male offspring. Thus, exposure of the fetus to TiO2 NPs impairs the development and function of the male regenerative system at the basic level of spermatogenesis. Similarly, an intratracheal administration of 200 μg of carbon NPs (14 nm) to pregnant ICR mice at gestational days 7 and 14 (400 μg total) results in histological changes in the seminiferous tubule and reduces DSP in male offspring at 5, 10, and 15 weeks old (Yoshida et al., 2009).
Changes in the seminiferous tubules and spermatogenesis can also be directly induced by exposing of adult male mice to NPs. An intratracheal administration of CB NPs (14, 56, or 95 nm) at a rather high dosage of 0.1 mg/mouse for 10 times every week, again results in histological changes in the seminiferous tubules and also elevates serum testosterone levels in response to 14 or 56 nm NPs (Bai et al., 2010). A smaller 14 nm CB has lesser antagonistic effects than the 56 nm NPs, despite the similar particle numbers, suggesting that NPs size may be a critical determinant on the impact on spermatogenesis (Bai et al., 2010). In another trial model, an intravenous injection of MWCNTs into male mice induced reversible damage in testes without influencing fertility (Kashiwada, 2006). Seminiferous epithelium thickness was reduced at day 15, and oxidative stress increased. However, ROS levels and seminiferous epithelium thickness were restored within 60–90 days. There was no alteration in the amount or quality of sperm or in hormone levels in the MWCNT-treated mice in the 90-day period, and mating of the treated animals resulted in normal pregnancy and delivery success rate, suggesting no influence on fertility. These results highlight the differential impact of different types on NPs and, in this case, the difference between different carbon-based NPs, namely CB NPs and MWCNT.
The accumulation of NPs in the testes is demonstrated in some animal models, but an understanding of the bio-distribution of NPs in the testes remains limited and nanotoxic effects are difficult to categorically define based on the evidence from the available studies. An intravenous and/or intra-abdominal administration of Si NPs (coated with either sodium chloride or sodium iodide) at a dose of 0–225 mg/kg of body weight to Kunming white mice (Chen et al., 2003) results in NP accumulation in the testicles, among other organs, including in the glands and interstitial cells, 96 h after administration. However, there was no indication of pathological cell changes or increased animal mortality (Chen et al., 2003). Similarly, an intraperitoneal administration of silica-coated magnetic NPs containing rhodamine B isothiocyanate (MNPs@SiO2 RITC; water-soluble, 50-nm thickness) at 10, 25, 50, or 100 mg/kg in male IRC mice (6 weeks of age) for 4 weeks results in testes accumulation with no apparent toxic effect (Kim et al., 2006). Moreover, the administration of fluorescent NPs of 39.4 nm in diameter at 1 mg/L to Medaka fish eggs showed that the NPs were detected in the testes (Leclerc et al., 2015). However, again there were no pathological changes in the cell nuclei, and there were no acute NP associated toxicities or an increased mortality even at higher doses, including in the ensuing generations (Xue et al., 2005; Leclerc et al., 2015). By contrast, a recent study on an intramuscular injection of silica-gold NPs into mice showed no evidence of the 70-nm NPs presence in the testes even 45 days after administration (Ong et al., 2016).
Other types of NPs, however, may induce toxic effects upon accumulation in the testes, with consequences for male fecundity. Recent studies focused on AgNPs, which are associated with deleterious effects on male reproduction in mammalian models (Lafuente et al., 2016). Studies on AgNP ingestion by fruit flies suggest that AgNP accumulation in the testes impacts on the number of germline stem cells, which is discussed in more detail below (Lafuente et al., 2016). A sub-chronic oral exposure of polyvinyl propylene (PVP)-coated AgNPs to rats also leads to altered testicular histology and sperm morphology abnormalities (Hong et al., 2016). Exposure to TiO2 NPs is also associated with reproductive toxicity in male animal models (Ritz et al., 2011). Importantly, the relative solubility and penetration through tissue barriers might be an important factor in inducing toxicity. Soluble nanoparticles, like Ag-NP, might be excreted through normal routes and, thus, have relatively lesser toxicity, whereas insoluble particles and the ones with a long half-life will have issues, as far as the toxicity effects are concerned. Recent studies suggest that, in male mice, reduced fertility and apoptosis/necrosis of spermatogenic cells and Sertoli cells in response to TiO2 NP administration is related to increased inflammatory responses and compromised immunity in the testes, including impaired tumor associated macrophages/toll-like receptor 3 (TAM/TLR3) signaling (Ritz et al., 2011). Meanwhile, oligospermia was observed in ICR mice after an intratracheal injection of carbon nanoparticles (14 nm) at a high dose of 1 mg/mouse for 10 times every week (Bai et al., 2010).
There is, therefore, a pressing need to carry out comparative studies to definitively assess the accumulation of different NP types at different doses and with different delivery modes in the testes and whether this causes toxicity, especially in the context of occupational exposure for male workers and the potential consequences on fertility.
The potential toxicity of NPs in the reproductive system is also directly examined by analyzing germ cells. The outcome seems to depend on the type of NPs used. In one study, AuNPs (9 nm) were directly added to fresh semen from a normal healthy male to investigate the effects on human sperm (Wiwanitkit et al., 2009). When added at a dose of 44 μg/mL, the NPs accumulate in the sperm tails and head, causing immotility in 25% of the sperm. It is unclear whether it is likely that direct exposure to these levels of NPs would be likely, although the use of AuNPs in, for example, imaging applications results in rather high doses, which could in turn have an impact on sperm upon the distribution of the NPs throughout the body. The effects of directly mixing polyvinyl alcohol (PVA)-coated Fe3O4 NPs with bovine sperm were also examined (Ben-David Makhluf et al., 2006). While NPs enter sperm and attach to mitochondria in the tail and in the acrosome region in the head, there is no apparent impact on the sperm acrosome reaction and motility.
Spermatogonial stem cells from mice are a suitable in vitro model for comparing the nanotoxicity of different NPs in male reproductive studies (Braydich-Stolle et al., 2005). The C4 and 18 cells, established from type A spermatogonia and isolated from 6-day old male mouse and bovine sperm cells, were used to test the ability of magnetite- PVA-coated NPs to enter the sperm/primary cells without disturbing the ability of the sperm to undergo the acrosome reaction or remain motile (Braydich-Stolle et al., 2005). Mitochondrial function was assessed to give a read-out of NP cytotoxicity, along with cell morphology, membrane leakage, and apoptosis after treatment with digitonin to permeabilize the cell membrane and release the bound particles. Importantly, the NP toxicity depends on the particle dosage in the testes, whereas the solubility of salts had no significant positive effect. AgNPs were the most toxic. For example, the EC50 in the MTS mitochondrial function assay is 8.75 μg/ml, whereas molybdenum trioxide (MoO3) NPs are less toxic (EC50 90 μg/ml). Such studies, which allow for a direct comparison of the effects of different types of NPs under the same experimental conditions, would help to clear up the confusion surrounding the relative effects of different NP types and dosages on the male reproductive system, as well as other areas of reproduction.
There are several industrial setups also releasing small particulate matter resembling nanoparticles. Air pollution with nanoparticles and their impact on human health is an emerging area to be explored in future. In a study to evaluate the impact of air particulate matters on living, laboratory mice exposed with ambient air in a polluted industrial area near steel mills manifested germ line mutations (Somers et al., 2002; Yauk et al., 2008). These findings are very alarming and warrant future studies aimed at evaluating the health of people living in the peripheries of industries.
NPs that are inhaled may be deposited in the airways and lungs, and due to their high surface reactivity, they have an inherent potential to induce inflammation and the generation of ROS at the site of deposition (Hougaard et al., 2010; Møller et al., 2010). In general, ROS generation is thought to be a major causative factor in the toxicity of NPs. ROS molecules are unstable and typically do not travel far beyond their site of formation (Wells et al., 2005). However, if there is an imbalance between oxidant and antioxidant mechanism, oxidative stress increases and induces or exacerbates NP-induced inflammation. In this case, neither the particles nor the inflammatory condition need is restricted to the lungs. The NPs can translocate, and inflammatory mediators might be released into the systemic circulation (Erdely et al., 2011). The NPs can be transported to organs related to pregnancy and fetal development and may be taken up by placental cells and interfere indirectly with fetal development by inducing oxidative stress and inflammation at that site. In particular, the placental cells contain toll like receptors (TLRs; especially, TLR-2 and TLR-4), which are involved in NP-induced inflammatory responses in the airway (Zhao et al., 2012; Koga et al., 2014). Clearly, there is evidence that inflammation and ROS generation might contribute to NP-induced toxicity in reproduction and development, but further studies are needed to better elucidate these mechanisms.
One of the main gaps in our understanding of how NPs are related to reproduction comes from the fact that studies are not designed consistently, and thus, it is hard to make comparisons across methods. To fill this gap, nano-toxicologists and reproductive scientists should work more closely together to design and interpret studies. By achieving optimal designs for the investigation of specific hypotheses and the understanding of certain aspects of the study, researchers can actually begin to fully answer important questions, such as whether there is a NP dose-effect. In addition, one of the most questions involves the mechanism of NP-induced lung and systemic inflammation. It is necessary to understand if inflammation is the driving force for developmental effects and whether the inflammatory response is different between the pregnant and the non-pregnant state. Furthermore, because of the fact that inflammation enhances particle transfer across the placenta (Tian et al., 2013), it might be important to know how development is affected if a NP exposure occurs in someone with an existing state of low-grade chronic inflammation, such as asthma and obesity, compared to NP exposure in a non-inflammatory state. Another gap to fill is the ability to compare studies with respect to exposure regimens and outcome assessments. This would require grouping of different NPs based on risk assessment and looking for patterns among different NPs. Finally, the dosing should be examined more thoroughly. There are many inconsistencies in the importance of the dose rate and the potential underlying differences for the induction of inflammation and the translocation of NPs across the lung and placenta.
The volume of studies on the toxicity of NPs in the reproductive system of animals is increasing, but the field is effectively still in its preliminary stages. While there is evidence to suggest the entry of some NPs into both male and female reproductive organs, both directly in adult animals and in utero, the studies were carried out with widely varying doses and administration routes, making direct comparisons and definitive conclusions difficult. In female animals, targeting of the uterus and ovaries is shown for a variety of NPs, including, for example TiO2, Cd, and Au, but there is wide variability in the results of the studies in terms of the evidence of morphological effects. In males, again there is evidence to suggest that NPs accumulate in the testes. For example, while Si-based NPs appear to have few, if any, toxic effects, Ag and TiO2-based NPs may be more dangerous, with an impact on cells in the seminiferous tubules, immune and inflammatory reactions, and sperm motility and morphology. All of these present a potential risk to male fertility if safe exposure levels are not defined and applied, such as among men working in the NP industry. There is a need to clarify the likely exposures of humans and animals to different types of NPs and tailor animal studies to mirror doses and entry routes for environmental, occupational, therapeutic, diagnostic, and cosmetic uses. Transplacental transfer of many types of NPs, including Au, TiO2, SiO2, C, and QDs, is established in animal models, and there is evidence to suggest that, in many cases, this results in the transfer of NPs to the vulnerable fetus, with varying toxic effects on, for example, the fetal brain and nerve development and future fertility. Preclinical studies with clearly defined parameters are urgently needed to clarify the situation with respect to fetal vulnerability, as well as reproductive health in general, to different NP types, sizes, surface charges, treatments, doses, and routes of delivery and the potentially toxic effects thereof. For example, the inhalation of NPs is a likely route of exposure in both environmental and occupational conditions, and CB-, TiO2-, and Cd-based NPs, among others, have been shown to have fetotoxic effects when inhaled by pregnant animals. The definition of “safe” levels of NPs in terms of reproductive and fetal health is imperative as the use of these products widen. The challenge is to carry out better coordinated and defined animal pre-clinical studies and human clinical trials to allow these goals to be achieved.
RB and LH were involved in the writing manuscript. RB, LH, LW, HT, ZR, and DB reviewed, summarized, and analyzed the literature to develop the conceptional outline to present the analysis, formulated the key summary point of review and drafted the manuscript and figures. DW, FK, and FF critically revised manuscript and assisted final review of figures, tables, and helped to the final preparation of manuscript.
The authors declare that the research was conducted in the absence of any commercial or financial relationships that could be construed as a potential conflict of interest.
This study was supported by National Natural Science Foundation of China (Grant No. 31171378) and the Fundamental Research Funds for the Central Universities (Program No. 2014PY045). The author grateful to Jing Cao, Chun-Jie Huang, and Li-Lin Huang for their help in improving the manuscript.
Adebambo, O. A., Ray, P. D., Shea, D., and Fry, R. C. (2015). Toxicological responses of environmental mixtures: environmental metal mixtures display synergistic induction of metal-responsive and oxidative stress genes in placental cells. Toxicol. Appl. Pharmacol. 289, 534–541. doi: 10.1016/j.taap.2015.10.005
Adler, S., Basketter, D., Creton, S., Pelkonen, O., Van Benthem, J., Zuang, V., et al. (2010). Alternative (non-animal) methods for cosmetics testing: current status and future prospects—2010. Arch. Toxicol. 85, 367–485. doi: 10.1007/s00204-011-0693-2
Alexis, F., Pridgen, E., Molnar, L. K., and Farokhzad, O. C. (2008). Factors affecting the clearance and biodistribution of polymeric nanoparticles. Mol. Pharm. 5, 505–515. doi: 10.1021/mp800051m
Ananth, C. V., Savitz, D. A., and Luther, E. R. (1996). Maternal cigarette smoking as a risk factor for placental abruption, placenta previa, and uterine bleeding in pregnancy. Am. J. Epidemiol. 144, 881–889. doi: 10.1093/oxfordjournals.aje.a009022
Anway, M. D., Cupp, A. S., Uzumcu, M., and Skinner, M. K. (2005). Epigenetic transgenerational actions of endocrine disruptors and male fertility. Science 308, 1466–1469. doi: 10.1126/science.1108190
Apter, D. (1997). Development of the hypothalamic-pituitary-ovarian axis. Ann. N.Y. Acad. Sci. 816, 9–21. doi: 10.1111/j.1749-6632.1997.tb52125.x
Araujo, L., Sheppard, M., Löbenberg, R., and Kreuter, J. (1999). Uptake of PMMA nanoparticles from the gastrointestinal tract after oral administration to rats: modification of the body distribution after suspension in surfactant solutions and in oil vehicles. Int. J. Pharm. 176, 209–224. doi: 10.1016/S0378-5173(98)00314-7
Arbab, A. S., Wilson, L. B., Ashari, P., Jordan, E. K., Lewis, B. K., and Frank, J. A. (2005). A model of lysosomal metabolism of dextran coated superparamagnetic iron oxide (SPIO) nanoparticles: implications for cellular magnetic resonance imaging. NMR Biomed. 18, 383–389. doi: 10.1002/nbm.970
Armenti, A. E., Zama, A. M., Passantino, L., and Uzumcu, M. (2008). Developmental methoxychlor exposure affects multiple reproductive parameters and ovarian folliculogenesis and gene expression in adult rats. Toxicol. Appl. Pharmacol. 233, 286–296. doi: 10.1016/j.taap.2008.09.010
Arora, S., Jain, J., Rajwade, J. M., and Paknikar, K. M. (2008). Cellular responses induced by silver nanoparticles: in vitro studies. Toxicol. Lett. 179, 93–100. doi: 10.1016/j.toxlet.2008.04.009
Auffan, M., Rose, J., Wiesner, M. R., and Bottero, J. Y. (2009). Chemical stability of metallic nanoparticles: a parameter controlling their potential cellular toxicity in vitro. Environ. Pollut. 157, 1127–1133. doi: 10.1016/j.envpol.2008.10.002
Bahadar, H., Maqbool, F., Niaz, K., and Abdollahi, M. (2016). Toxicity of nanoparticles and an overview of current experimental models. Iran. Biomed. J. 20, 1–11. doi: 10.7508/ibj.2016.01.001
Bai, Y., Zhang, Y., Zhang, J., Mu, Q., Zhang, W., Butch, E. R., et al. (2010). Repeated administrations of carbon nanotubes in male mice cause reversible testis damage without affecting fertility. Nat. Nanotechnol. 5, 683–689. doi: 10.1038/nnano.2010.153
Bartneck, M., Ritz, T., Keul, H. A., Wambach, M., Bornemann, J., Gbureck, U., et al. (2012). Peptide-functionalized gold nanorods increase liver injury in hepatitis. ACS Nano 6, 8767–8777. doi: 10.1021/nn302502u
Ben-David Makhluf, S., Qasem, R., Rubinstein, S., Gedanken, A., and Breitbart, H. (2006). Loading magnetic nanoparticles into sperm cells does not affect their functionality. Langmuir 22, 9480–9482. doi: 10.1021/la061988z
Berry, V. (2013). Impermeability of graphene and its applications. Carbon 62, 1–10. doi: 10.1016/j.carbon.2013.05.052
Blum, J. L., Xiong, J. Q., Hoffman, C., and Zelikoff, J. T. (2012). Cadmium associated with inhaled cadmium oxide nanoparticles impacts fetal and neonatal development and growth. Toxicol. Sci. 126, 478–486. doi: 10.1093/toxsci/kfs008
Boisen, A. M. Z., Shipley, T., Jackson, P., Hougaard, K. S., Wallin, H., Yauk, C. L., et al. (2012). NanoTIO 2 (UV-Titan) does not induce ESTR mutations in the germline of prenatally exposed female mice. Part. Fibre Toxicol. 9:19. doi: 10.1186/1743-8977-9-19
Boisen, A. M. Z., Shipley, T., Jackson, P., Wallin, H., Nellemann, C., Vogel, U., et al. (2013). In utero exposure to nanosized carbon black (Printex90) does not induce tandem repeat mutations in female murine germ cells. Reprod. Toxicol. 41, 45–48. doi: 10.1016/j.reprotox.2013.06.068
Braydich-Stolle, L., Hussain, S., Schlager, J. J., and Hofmann, M. C. (2005). in vitro cytotoxicity of nanoparticles in mammalian germline stem cells. Toxicol. Sci. 88, 412–419. doi: 10.1093/toxsci/kfi256
Buzea, C., Pacheco, I. I., and Robbie, K. (2007). Nanomaterials and nanoparticles: sources and toxicity. Biointerphases 2, MR17–MR71. doi: 10.1116/1.2815690
Casals, E., Gonzalez, E., and Puntes, V. F. (2012). Reactivity of inorganic nanoparticles in biological environments: insights into nanotoxicity mechanisms. J. Phys. D Appl. Phys. 45:443001. doi: 10.1088/0022-3727/45/44/443001
Chen, J., Wang, H., Long, W., Shen, X., Wu, D., Song, S. S., et al. (2013). Sex differences in the toxicity of polyethylene glycol-coated gold nanoparticles in mice. Int. J. Nanomedicine 8, 2409–2419. doi: 10.2147/IJN.S46376
Chen, Y., Xue, Z., Zheng, D., Xia, K., Zhao, Y., Liu, T., et al. (2003). Sodium chloride modified silica nanoparticles as a non-viral vector with a high efficiency of DNA transfer into cells. Curr. Gene Ther. 3, 273–279. doi: 10.2174/1566523034578339
Chen, Z., Chen, H., Meng, H., Xing, G., Gao, X., Sun, B., et al. (2008). Bio-distribution and metabolic paths of silica coated CdSeS quantum dots. Toxicol. Appl. Pharmacol. 230, 364–371. doi: 10.1016/j.taap.2008.03.022
Choi, H. S., Ashitate, Y., Lee, J. H., Kim, S. H., Matsui, A., Insin, N., et al. (2010). Rapid translocation of nanoparticles from the lung airspaces to the body. Nat. Biotechnol. 28, 1300–1303. doi: 10.1038/nbt.1696
Chou, C. C., Hsiao, H. Y., Hong, Q. S., Chen, C. H., Peng, Y. W., Chen, H. W., et al. (2008). Single-walled carbon nanotubes can induce pulmonary injury in mouse model. Nano Lett. 8, 437–445. doi: 10.1021/nl0723634
Chu, M., Wu, Q., Yang, H., Yuan, R., Hou, S., Yang, Y., et al. (2010). Transfer of quantum dots from pregnant mice to pups across the placental barrier. Small 6, 670–678. doi: 10.1002/smll.200902049
Chun-Mei, L. I., Xuezheng, L. I., Suzuki, A. K., Zhang, Y., Fujitani, Y., Nagaoka, K., et al. (2013). Effects of exposure to nanoparticle-rich diesel exhaust on pregnancy in rats. J. Reprod. Dev. 59, 145–150. doi: 10.1262/jrd.2012-145
Das, S. K., Das, A. R., and Guha, A. K. (2009). Gold nanoparticles: microbial synthesis and application in water hygiene management. Langmuir 25, 8192–8199. doi: 10.1021/la900585p
De Jong, W. H., Hagens, W. I., Krystek, P., Burger, M. C., Sips, A. J., and Geertsma, R. E. (2008). Particle size-dependent organ distribution of gold nanoparticles after intravenous administration. Biomaterials 29, 1912–1919. doi: 10.1016/j.biomaterials.2007.12.037
Derfus, A. M., Chan, W. C., and Bhatia, S. N. (2004). Probing the cytotoxicity of semiconductor quantum dots. Nano Lett. 4, 11–18. doi: 10.1021/nl0347334
Di Bona, K. R., Xu, Y., Gray, M., Fair, D., Hayles, H., Milad, L., et al. (2015). Short-and long-term effects of prenatal exposure to iron oxide nanoparticles: influence of surface charge and dose on developmental and reproductive toxicity. Int. J. Mol. Sci. 16, 30251–30268. doi: 10.3390/ijms161226231
Dibrov, P., Dzioba, J., Gosink, K. K., and Häse, C. C. (2002). Chemiosmotic mechanism of antimicrobial activity of Ag+ in Vibrio cholerae. Antimicrob. Agents Chemother. 46, 2668–2670. doi: 10.1128/AAC.46.8.2668-2670.2002
Elder, A., Gelein, R., Silva, V., Feikert, T., Opanashuk, L., Carter, J., et al. (2006). Translocation of inhaled ultrafine manganese oxide particles to the central nervous system. Environ. Health Perspect. 1172–1178. doi: 10.1289/ehp.9030
Ema, M., Kobayashi, N., Naya, M., Hanai, S., and Nakanishi, J. (2010). Reproductive and developmental toxicity studies of manufactured nanomaterials. Reprod. Toxicol. 30, 343–352. doi: 10.1016/j.reprotox.2010.06.002
Erdely, A., Liston, A., Salmen-Muniz, R., Hulderman, T., Young, S. H., Zeidler-Erdely, P. C., et al. (2011). Identification of systemic markers from a pulmonary carbon nanotube exposure. J. Occup. Environ. Med. 53, S80–S86. doi: 10.1097/JOM.0b013e31821ad724
Fedulov, A. V., Leme, A., Yang, Z., Dahl, M., Lim, R., Mariani, T. J., et al. (2008). Pulmonary exposure to particles during pregnancy causes increased neonatal asthma susceptibility. Am. J. Respir. Cell Mol. Biol. 38, 57–67. doi: 10.1165/rcmb.2007-0124OC
Franklin, N. M., Rogers, N. J., Apte, S. C., Batley, G. E., Gadd, G. E., and Casey, P. S. (2007). Comparative toxicity of nanoparticulate ZnO, bulk ZnO, and ZnCl2 to a freshwater microalga (Pseudokirchneriella subcapitata): the importance of particle solubility. Environ. Sci. Technol. 41, 8484–8490. doi: 10.1021/es071445r
Fujimoto, A., Tsukue, N., Watanabe, M., Sugawara, I., Yanagisawa, R., Takano, H., et al. (2005). Diesel exhaust affects immunological action in the placentas of mice. Environ. Toxicol. 20, 431–440. doi: 10.1002/tox.20129
Fukui, H., Horie, M., Endoh, S., Kato, H., Fujita, K., Nishio, K., et al. (2012). Association of zinc ion release and oxidative stress induced by intratracheal instillation of ZnO nanoparticles to rat lung. Chem. Biol. Interact. 198, 29–37. doi: 10.1016/j.cbi.2012.04.007
Gao, G., Ze, Y., Li, B., Zhao, X., Zhang, T., Sheng, L., et al. (2012). Ovarian dysfunction and gene-expressed characteristics of female mice caused by long-term exposure to titanium dioxide nanoparticles. J. Hazard. Mater. 243, 19–27. doi: 10.1016/j.jhazmat.2012.08.049
Gao, G., Ze, Y., Zhao, X., Sang, X., Zheng, L., Ze, X., et al. (2013). Titanium dioxide nanoparticle-induced testicular damage, spermatogenesis suppression, and gene expression alterations in male mice. J. Hazard. Mater. 258, 133–143. doi: 10.1016/j.jhazmat.2013.04.046
Gao, X., Cui, Y., Levenson, R. M., Chung, L. W., and Nie, S. (2004). in vivo cancer targeting and imaging with semiconductor quantum dots. Nat. Biotechnol. 22, 969–976. doi: 10.1038/nbt994
Geiser, M. (2010). Update on macrophage clearance of inhaled micro-and nanoparticles. J. Aerosol Med. Pulm. Drug Deliv. 23, 207–217. doi: 10.1089/jamp.2009.0797
Hamada, K., Suzaki, Y., Goldman, A., Ning, Y. Y., Goldsmith, C., Palecanda, A., et al. (2003). Allergen-independent maternal transmission of asthma susceptibility. J. Immunol. 170, 1683–1689. doi: 10.4049/jimmunol.170.4.1683
Han, J. W., Jeong, J. K., Gurunathan, S., Choi, Y. J., Das, J., Kwon, D. N., et al. (2016). Male-and female-derived somatic and germ cell-specific toxicity of silver nanoparticles in mouse. Nanotoxicology 10, 361–373. doi: 10.3109/17435390.2015.1073396
Hillier, S. G. (1994). Current concepts of the roles of follicle stimulating hormone and luteinizing hormone in folliculogenesis. Hum. Reprod. 9, 188–191. doi: 10.1093/oxfordjournals.humrep.a138480
Hong, F., Wang, Y., Zhou, Y., Zhang, Q., Ge, Y., Chen, M., et al. (2015a). Exposure to TiO2 nanoparticles induces immunological dysfunction in mouse testitis. J. Agric. Food Chem. 64, 346–355. doi: 10.1021/acs.jafc.5b05262
Hong, F., Zhao, X., Chen, M., Zhou, Y., Ze, Y., Wang, L., et al. (2016). TiO2 nanoparticles-induced apoptosis of primary cultured Sertoli cells of mice. J. Biomed. Mater. Res. Part A 104, 124–135. doi: 10.1002/jbm.a.35548
Hong, F., Zhao, X., Si, W., Ze, Y., Wang, L., Zhou, Y., et al. (2015b). Decreased spermatogenesis led to alterations of testis-specific gene expression in male mice following nano-TiO 2 exposure. J. Hazard. Mater. 300, 718–728. doi: 10.1016/j.jhazmat.2015.08.010
Hoshino, A., Hanaki, K. I., Suzuki, K., and Yamamoto, K. (2004). Applications of T-lymphoma labeled with fluorescent quantum dots to cell tracing markers in mouse body. Biochem. Biophys. Res. Commun. 314, 46–53. doi: 10.1016/j.bbrc.2003.11.185
Hougaard, K. S., Campagnolo, L., Chavatte-Palmer, P., Tarrade, A., Rousseau-Ralliard, D., Valentino, S., et al. (2015). A perspective on the developmental toxicity of inhaled nanoparticles. Reprod. Toxicol. 56, 118–140. doi: 10.1016/j.reprotox.2015.05.015
Hougaard, K. S., Jackson, P., Jensen, K. A., Sloth, J. J., Löschner, K., Larsen, E. H., et al. (2010). Effects of prenatal exposure to surface-coated nanosized titanium dioxide (UV-Titan). A study in mice. Part. Fibre Toxicol. 7:16. doi: 10.1186/1743-8977-7-16
Hougaard, K. S., Jackson, P., Kyjovska, Z. O., Birkedal, R. K., De Temmerman, P. J., Brunelli, A., et al. (2013). Effects of lung exposure to carbon nanotubes on female fertility and pregnancy. A study in mice. Reprod. Toxicol. 41, 86–97. doi: 10.1016/j.reprotox.2013.05.006
Hougaard, K. S., Jensen, K. A., Nordly, P., Taxvig, C., Vogel, U., Saber, A. T., et al. (2008). Effects of prenatal exposure to diesel exhaust particles on postnatal development, behavior, genotoxicity and inflammation in mice. Part. Fibre Toxicol. 5:3. doi: 10.1186/1743-8977-5-3
Jackson, P., Halappanavar, S., Hougaard, K. S., Williams, A., Madsen, A. M., Lamson, J. S., et al. (2013). Maternal inhalation of surface-coated nanosized titanium dioxide (UV-Titan) in C57BL/6 mice: effects in prenatally exposed offspring on hepatic DNA damage and gene expression. Nanotoxicology 7, 85–96. doi: 10.3109/17435390.2011.633715
Jackson, P., Hougaard, K. S., Boisen, A. M. Z., Jacobsen, N. R., Jensen, K. A., Møller, P., et al. (2012a). Pulmonary exposure to carbon black by inhalation or instillation in pregnant mice: effects on liver DNA strand breaks in dams and offspring. Nanotoxicology 6, 486–500. doi: 10.3109/17435390.2011.587902
Jackson, P., Hougaard, K. S., Vogel, U., Wu, D., Casavant, L., Williams, A., et al. (2012b). Exposure of pregnant mice to carbon black by intratracheal instillation: toxicogenomic effects in dams and offspring. Mutat. Res. Genet. Toxicol. Environ. Mutagen. 745, 73–83. doi: 10.1016/j.mrgentox.2011.09.018
Jackson, P., Vogel, U., Wallin, H., and Hougaard, K. S. (2011). Prenatal exposure to carbon black (Printex 90): effects on sexual development and neurofunction. Basic Clin. Pharmacol. Toxicol. 109, 434–437. doi: 10.1111/j.1742-7843.2011.00745.x
Jani, P., Halbert, G. W., Langridge, J., and Florence, A. T. (1990). Nanoparticle uptake by the rat gastrointestinal mucosa: quantitation and particle size dependency. J. Pharm. Pharmacol. 42, 821–826. doi: 10.1111/j.2042-7158.1990.tb07033.x
Juan, H., XuYing, W., Fei, W., GuiFeng, X., Zhen, L., and TianBao, Z. (2009). Effects of titanium dioxide nanoparticles on development and maturation of rat preantral follicle in vitro. Acad. J. Second Mil. Med. Univ. 30, 869–873. doi: 10.3724/sp.j.1008.2009.00869
Kafshdooz, L., Kafshdooz, T., Razban, Z., and Akbarzadeh, A. (2016). The application of gold nanoparticles as a promising therapeutic approach in breast and ovarian cancer. Artif. Cells Nanomed. Biotechnol. 44, 1222–1227. doi: 10.3109/21691401.2015.1029625
Kagan, V. E., Konduru, N. V., Feng, W., Allen, B. L., Conroy, J., Volkov, Y., et al. (2010). Carbon nanotubes degraded by neutrophil myeloperoxidase induce less pulmonary inflammation. Nat. Nanotechnol. 5, 354–359. doi: 10.1038/nnano.2010.44
Kashiwada, S. (2006). Distribution of nanoparticles in the see-through medaka (Oryzias latipes). Environ. Health Perspect. 114, 1697–1702. doi: 10.1289/ehp.9209
Kedziora, A., Gorzelanczyk, K., and Bugla Ploskonska, G. (2013). Positive and negative aspects of silver nanoparticles usage. Biol. Int. 53, 67–76. Available online at: https://www.scienceopen.com/document?vid=11a3c606-e197-4c4f-abca-29d7b8f72e17
Khanna, P., Ong, C., Bay, B. H., and Baeg, G. H. (2015). Nanotoxicity: an interplay of oxidative stress, inflammation and cell death. Nanomaterials 5, 1163–1180. doi: 10.3390/nano5031163
Kim, J. S., Yoon, T. J., Yu, K. N., Kim, B. G., Park, S. J., Kim, H. W., et al. (2006). Toxicity and tissue distribution of magnetic nanoparticles in mice. Toxicol. Sci. 89, 338–347. doi: 10.1093/toxsci/kfj027
Kim, S., Lim, Y. T., Soltesz, E. G., De Grand, A. M., Lee, J., Nakayama, A., et al. (2004). Near-infrared fluorescent type II quantum dots for sentinel lymph node mapping. Nat. Biotechnol. 22, 93–97. doi: 10.1038/nbt920
Koga, K., Izumi, G., Mor, G., Fujii, T., and Osuga, Y. (2014). Toll-like receptors at the maternal-fetal interface in normal pregnancy and pregnancy complications. Am. J. Reprod. Immunol. 72, 192–205. doi: 10.1111/aji.12258
Komatsu, T., Tabata, M., Kubo-Irie, M., Shimizu, T., Suzuki, K. I., Nihei, Y., et al. (2008). The effects of nanoparticles on mouse testis Leydig cells in vitro. Toxicol. In vitro 22, 1825–1831. doi: 10.1016/j.tiv.2008.08.009
Kong, L., Gao, X., Zhu, J., Cheng, K., and Tang, M. (2016). Mechanisms involved in reproductive toxicity caused by nickel nanoparticle in female rats. Environ. Toxicol. 31, 1674–1683. doi: 10.1002/tox.22288
Kong, L., Tang, M., Zhang, T., Wang, D., Hu, K., Lu, W., et al. (2014). Nickel nanoparticles exposure and reproductive toxicity in healthy adult rats. Int. J. Mol. Sci. 15, 21253–21269. doi: 10.3390/ijms151121253
Kubo-Irie, M., Oshio, S., Niwata, Y., Ishihara, A., Sugawara, I., and Takeda, K. (2011). Pre-and postnatal exposure to low-dose diesel exhaust impairs murine spermatogenesis. Inhal. Toxicol. 23, 805–813. doi: 10.3109/08958378.2011.610834
Kyjovska, Z. O., Boisen, A. M. Z., Jackson, P., Wallin, H., Vogel, U., and Hougaard, K. S. (2013). Daily sperm production: application in studies of prenatal exposure to nanoparticles in mice. Reprod. Toxicol. 36, 88–97. doi: 10.1016/j.reprotox.2012.12.005
Lafuente, D., Garcia, T., Blanco, J., Sánchez, D. J., Sirvent, J. J., Domingo, J. L., et al. (2016). Effects of oral exposure to silver nanoparticles on the sperm of rats. Reprod. Toxicol. 60, 133–139. doi: 10.1016/j.reprotox.2016.02.007
Lan, Z., and Yang, W. X. (2012). Nanoparticles and spermatogenesis: how do nanoparticles affect spermatogenesis and penetrate the blood–testis barrier. Nanomedicine 7, 579–596. doi: 10.2217/nnm.12.20
Leclerc, L., Klein, J. P., Forest, V., Boudard, D., Martini, M., Pourchez, J., et al. (2015). Testicular biodistribution of silica-gold nanoparticles after intramuscular injection in mice. Biomed. Microdevices 17:66. doi: 10.1007/s10544-015-9968-3
Lee, J. H., Huh, Y. M., Jun, Y. W., Seo, J. W., Jang, J. T., Song, H. T., et al. (2007). Artificially engineered magnetic nanoparticles for ultra-sensitive molecular imaging. Nat. Med. 13, 95–99. doi: 10.1038/nm1467
Le Goff, A., Artero, V., Jousselme, B., Tran, P. D., Guillet, N., Métayé, R., et al. (2009). From hydrogenases to noble metal–free catalytic nanomaterials for H2 production and uptake. Science 326, 1384–1387. doi: 10.1126/science.1179773
Li, C., Taneda, S., Taya, K., Watanabe, G., Li, X., Fujitani, Y., et al. (2009). Effects of in utero exposure to nanoparticle-rich diesel exhaust on testicular function in immature male rats. Toxicol. Lett. 185, 1–8. doi: 10.1016/j.toxlet.2008.11.012
Li, M., Al-Jamal, K. T., Kostarelos, K., and Reineke, J. (2010). Physiologically based pharmacokinetic modeling of nanoparticles. ACS Nano 4, 6303–6317. doi: 10.1021/nn1018818
Li, Y., Liu, Y., Fu, Y., Wei, T., Le Guyader, L., Gao, G., et al. (2012). The triggering of apoptosis in macrophages by pristine graphene through the MAPK and TGF-beta signaling pathways. Biomaterials 33, 402–411. doi: 10.1016/j.biomaterials.2011.09.091
Li, Y., Zhou, Y., Wang, H. Y., Perrett, S., Zhao, Y., Tang, Z., et al. (2011). Chirality of glutathione surface coating affects the cytotoxicity of quantum dots. Angewandte Chem. Int. Edn. 50, 5860–5864. doi: 10.1002/anie.201008206
Lim, J. H., Kim, S. H., Shin, I. S., Park, N. H., Moon, C., Kang, S. S., et al. (2011). Maternal exposure to multi-wall carbon nanotubes does not induce embryo–fetal developmental toxicity in rats. Birth Defects Res. B Dev. Reprod. Toxicol. 92, 69–76. doi: 10.1002/bdrb.20283
Lin, P., Chen, J. W., Chang, L. W., Wu, J. P., Redding, L., Chang, H., et al. (2008). Computational and ultrastructural toxicology of a nanoparticle, Quantum Dot 705, in mice. Environ. Sci. Technol. 42, 6264–6270. doi: 10.1021/es800254a
Liu, Z., Chen, K., Davis, C., Sherlock, S., Cao, Q., Chen, X., et al. (2008a). Drug delivery with carbon nanotubes for in vivo cancer treatment. Cancer Res. 68, 6652–6660. doi: 10.1158/0008-5472.CAN-08-1468
Liu, Z., Davis, C., Cai, W., He, L., Chen, X., and Dai, H. (2008b). Circulation and long-term fate of functionalized, biocompatible single-walled carbon nanotubes in mice probed by Raman spectroscopy. Proc. Natl. Acad. Sci. U.S.A. 105, 1410–1415. doi: 10.1073/pnas.0707654105
Longmire, M., Choyke, P. L., and Kobayashi, H. (2008). Clearance properties of nano-sized particles and molecules as imaging agents: considerations and caveats. Nanomedicine 3, 703–717. doi: 10.2217/17435889.3.5.703
Lundqvist, M., Stigler, J., Elia, G., Lynch, I., Cedervall, T., and Dawson, K. A. (2008). Nanoparticle size and surface properties determine the protein corona with possible implications for biological impacts. Proc. Natl. Acad. Sci. U.S.A. 105, 14265–14270. doi: 10.1073/pnas.0805135105
Ma, X., Wu, Y., Jin, S., Tian, Y., Zhang, X., Zhao, Y., et al. (2011). Gold nanoparticles induce autophagosome accumulation through size-dependent nanoparticle uptake and lysosome impairment. ACS Nano 5, 8629–8639. doi: 10.1021/nn202155y
Manke, A., Wang, L., and Rojanasakul, Y. (2013). Mechanisms of nanoparticle-induced oxidative stress and toxicity. Biomed Res. Int. 2013:942916. doi: 10.1155/2013/942916
Mattison, D. R., Plowchalk, D. R., Meadows, M. J., Al-Juburi, A. Z., Gandy, J., and Malek, A. (1990). Reproductive toxicity: male and female reproductive systems as targets for chemical injury. Med. Clin. North Am. 74, 391–411. doi: 10.1016/S0025-7125(16)30569-7
McShan, D., Ray, P. C., and Yu, H. (2014). Molecular toxicity mechanism of nanosilver. J. Food Drug Analysis 22, 116–127. doi: 10.1016/j.jfda.2014.01.010
Meng, H., Chen, Z., Xing, G., Yuan, H., Chen, C., Zhao, F., et al. (2007). Ultrahigh reactivity provokes nanotoxicity: explanation of oral toxicity of nano-copper particles. Toxicol. Lett. 175, 102–110. doi: 10.1016/j.toxlet.2007.09.015
Meng, J., Yang, M., Jia, F., Kong, H., Zhang, W., Wang, C., et al. (2010). Subcutaneous injection of water-soluble multi-walled carbon nanotubes in tumor-bearing mice boosts the host immune activity. Nanotechnology 21:145104. doi: 10.1088/0957-4484/21/14/145104
Menon, R., Fortunato, S. J., Yu, J., Milne, G. L., Sanchez, S., Drobek, C. O., et al. (2011). Cigarette smoke induces oxidative stress and apoptosis in normal term fetal membranes. Placenta 32, 317–322. doi: 10.1016/j.placenta.2011.01.015
Møller, P., Jacobsen, N. R., Folkmann, J. K., Danielsen, P. H., Mikkelsen, L., Hemmingsen, J. G., et al. (2010). Role of oxidative damage in toxicity of particulates. Free Radic. Res. 44, 1–46. doi: 10.3109/10715760903300691
Morones, J. R., Elechiguerra, J. L., Camacho, A., Holt, K., Kouri, J. B., Ramírez, J. T., et al. (2005). The bactericidal effect of silver nanoparticles. Nanotechnology 16:2346. doi: 10.1088/0957-4484/16/10/059
Myllynen, P. K., Loughran, M. J., Howard, C. V., Sormunen, R., Walsh, A. A., and Vähäkangas, K. H. (2008). Kinetics of gold nanoparticles in the human placenta. Reprod. Toxicol. 26, 130–137. doi: 10.1016/j.reprotox.2008.06.008
Nahire, R., Haldar, M. K., Paul, S., Mergoum, A., Ambre, A. H., Katti, K. S., et al. (2013). Polymer-coated echogenic lipid nanoparticles with dual release triggers. Biomacromolecules 14, 841–853. doi: 10.1021/bm301894z
Nel, A., Xia, T., Mädler, L., and Li, N. (2006). Toxic potential of materials at the nanolevel. Science 311, 622–627. doi: 10.1126/science.1114397
Nel, A. E., Mädler, L., Velegol, D., Xia, T., Hoek, E. M., Somasundaran, P., et al. (2009). Understanding biophysicochemical interactions at the nano–bio interface. Nat. Mater. 8, 543–557. doi: 10.1038/nmat2442
Oberdörster, G., Oberdörster, E., and Oberdörster, J. (2005). Nanotoxicology: an emerging discipline evolving from studies of ultrafine particles. Environ. Health Perspect. 823–839. doi: 10.1289/ehp.7339
Olivero, O. A., Yuspa, S. H., Poirier, M. C., Anderson, L. M., Jones, A. B., Wang, C., et al. (1997). Transplacental effects of 3′-azido-2′, 3′-dideoxythymidine (AZT): tumorigenicity in mice and genotoxicity in mice and monkeys. J. Natl. Cancer Inst. 89, 1602–1608. doi: 10.1093/jnci/89.21.1602
Ong, C., Lee, Q. Y., Cai, Y., Liu, X., Ding, J., Yung, L. Y. L., et al. (2016). Silver nanoparticles disrupt germline stem cell maintenance in the Drosophila testis. Sci. Rep. 6:20632. doi: 10.1038/srep20632
Ono, N., Oshio, S., Niwata, Y., Yoshida, S., Tsukue, N., Sugawara, I., et al. (2007). Prenatal exposure to diesel exhaust impairs mouse spermatogenesis. Inhal. Toxicol. 19, 275–281. doi: 10.1080/08958370601069257
Park, E. J., Kim, H., Kim, Y., and Park, K. (2010). Effects of platinum nanoparticles on the postnatal development of mouse pups by maternal exposure. Environ. Health Toxicol. 25, 279–286. Available online at: https://www.e-eht.org/journal/view.php?number=699
Perera, F. P., Rauh, V., Tsai, W. Y., Kinney, P., Camann, D., Barr, D., et al. (2003). Effects of transplacental exposure to environmental pollutants on birth outcomes in a multiethnic population. Environ. Health Perspect. 111, 201. doi: 10.1289/ehp.5742
Philbrook, N. A., Walker, V. K., Afrooz, A. N., Saleh, N. B., and Winn, L. M. (2011). Investigating the effects of functionalized carbon nanotubes on reproduction and development in Drosophila melanogaster and CD-1 mice. Reprod. Toxicol. 32, 442–448. doi: 10.1016/j.reprotox.2011.09.002
Pietroiusti, A., Massimiani, M., Fenoglio, I., Colonna, M., Valentini, F., Palleschi, G., et al. (2011). Low doses of pristine and oxidized single-wall carbon nanotubes affect mammalian embryonic development. ACS Nano 5, 4624–4633. doi: 10.1021/nn200372g
Qiu, Y., Liu, Y., Wang, L., Xu, L., Bai, R., Ji, Y., et al. (2010). Surface chemistry and aspect ratio mediated cellular uptake of Au nanorods. Biomaterials 31, 7606–7619. doi: 10.1016/j.biomaterials.2010.06.051
Qu, Y., Li, W., Zhou, Y., Liu, X., Zhang, L., Wang, L., et al. (2011). Full assessment of fate and physiological behavior of quantum dots utilizing Caenorhabditis elegans as a model organism. Nano Lett. 11, 3174–3183. doi: 10.1021/nl201391e
Rattanapinyopituk, K., Shimada, A., Morita, T., Sakurai, M., Asano, A., Hasegawa, T., et al. (2014). Demonstration of the clathrin-and caveolin-mediated endocytosis at the maternal–fetal barrier in mouse placenta after intravenous administration of gold nanoparticles. J. Vet. Med. Sci. 76, 377–387. doi: 10.1292/jvms.13-0512
Refuerzo, J. S., Godin, B., Bishop, K., Srinivasan, S., Shah, S. K., Amra, S., et al. (2011). Size of the nanovectors determines the transplacental passage in pregnancy: study in rats. Am. J. Obstet. Gynecol. 204, 546–e5. doi: 10.1016/j.ajog.2011.02.033
Ritz, C., Ruminski, W., Hougaard, K. S., Wallin, H., Vogel, U., and Yauk, C. L. (2011). Germline mutation rates in mice following in utero exposure to diesel exhaust particles by maternal inhalation. Mutat. Res. Fund. Mol. Mech. Mut. 712, 55–58. doi: 10.1016/j.mrfmmm.2011.04.007
Robbins, J. R., and Bakardjiev, A. I. (2012). Pathogens and the placental fortress. Curr. Opin. Microbiol. 15, 36–43. doi: 10.1016/j.mib.2011.11.006
Rogers, J. M., and Kavlock, R. J. (1998). “Developmental toxicology,” in Reproductive and Developmental Toxicology, ed K. S. Korach (New York, NY: Marcel Dekker, Inc.), 47–71.
Ruge, C. A., Kirch, J., Cañadas, O., Schneider, M., Perez-Gil, J., Schaefer, U. F., et al. (2011). Uptake of nanoparticles by alveolar macrophages is triggered by surfactant protein A. Nanomedicine 7, 690–693. doi: 10.1016/j.nano.2011.07.009
Sadauskas, E., Wallin, H., Stoltenberg, M., Vogel, U., Doering, P., Larsen, A., et al. (2007). Kupffer cells are central in the removal of nanoparticles from the organism. Part. Fibre Toxicol. 4:10. doi: 10.1186/1743-8977-4-10
Sanhai, W. R., Sakamoto, J. H., Canady, R., and Ferrari, M. (2008). Seven challenges for nanomedicine. Nat. Nanotechnol. 3, 242–244. doi: 10.1038/nnano.2008.114
Savage, N., and Diallo, M. S. (2005). Nanomaterials and water purification: opportunities and challenges. J. Nano. Res. 7, 331–342. doi: 10.1007/s11051-005-7523-5
Schipper, M. L., Nakayama-Ratchford, N., Davis, C. R., Kam, N. W. S., Chu, P., Liu, Z., et al. (2008). A pilot toxicology study of single-walled carbon nanotubes in a small sample of mice. Nat. Nanotechnol. 3, 216–221. doi: 10.1038/nnano.2008.68
Semmler-Behnke, M., Fertsch, S., Schmid, G., Wenk, A., and Kreyling, W. G. (2007). Uptake of 1.4 nm versus 18 nm gold nanoparticles in secondary target organs is size dependent in control and pregnant rats after intratracheal or intravenous application. Proc. EuroNanoForum 2007, 19–21. Available online at: https://ec.europa.eu/research/industrial_technologies/pdf/euronanoforum2007-proceedings_en.pdf
Semmler-Behnke, M., Lipka, J., Wenk, A., Hirn, S., Schäffler, M., Tian, F., et al. (2014). Size dependent translocation and fetal accumulation of gold nanoparticles from maternal blood in the rat. Part. Fibre Toxicol. 11:33. doi: 10.1186/s12989-014-0033-9
Shi, H., Magaye, R., Castranova, V., and Zhao, J. (2013). Titanium dioxide nanoparticles: a review of current toxicological data. Part. Fibre Toxicol. 10:15. doi: 10.1186/1743-8977-10-15
Slama, R., Darrow, L., Parker, J., Woodruff, T. J., Strickland, M., Nieuwenhuijsen, M., et al. (2008). Meeting report: atmospheric pollution and human reproduction. Environ. Health Perspect. 116:791. doi: 10.1289/ehp.11074
Snyder, R. W., Fennell, T. R., Wingard, C. J., Mortensen, N. P., Holland, N. A., Shannahan, J. H., et al. (2015). Distribution and biomarker of carbon-14 labeled fullerene C60 ([14C (U)] C60) in pregnant and lactating rats and their offspring after maternal intravenous exposure. J. Appl. Toxicol. 35, 1438–1451. doi: 10.1002/jat.3177
Somers, C. M., Yauk, C. L., White, P. A., Parfett, C. L., and Quinn, J. S. (2002). Air pollution induces heritable DNA mutations. Proc. Natl. Acad. Sci. U.S.A. 99, 15904–15907. doi: 10.1073/pnas.252499499
Song, Y., Li, X., and Du, X. (2009). Exposure to nanoparticles is related to pleural effusion, pulmonary fibrosis and granuloma. Eur. Respir. J. 34, 559–567. doi: 10.1183/09031936.00178308
Souris, J. S., Lee, C. H., Cheng, S. H., Chen, C. T., Yang, C. S., Ja-an, A. H., et al. (2010). Surface charge-mediated rapid hepatobiliary excretion of mesoporous silica nanoparticles. Biomaterials 31, 5564–5574. doi: 10.1016/j.biomaterials.2010.03.048
Sugamata, M., Ihara, T., Takano, H., Oshio, S., and Takeda, K. (2006). Maternal diesel exhaust exposure damages newborn murine brains. J. Health Sci. 52, 82–84. doi: 10.1248/jhs.52.82
Sumner, S. C., Fennell, T. R., Snyder, R. W., Taylor, G. F., and Lewin, A. H. (2010). Distribution of carbon-14 labeled C60 ([14C] C60) in the pregnant and in the lactating dam and the effect of C60 exposure on the biochemical profile of urine. J. Appl. Toxicol. 30, 354–360. doi: 10.1002/jat.1503
Takahashi, S., and Matsuoka, O. (1981). Cross placental transfer of 198Au-colloid in near term rats. J. Radiat. Res. 22, 242–249. doi: 10.1269/jrr.22.242
Takahashi, Y., Mizuo, K., Shinkai, Y., Oshio, S., and Takeda, K. (2010). Prenatal exposure to titanium dioxide nanoparticles increases dopamine levels in the prefrontal cortex and neostriatum of mice. J. Toxicol. Sci. 35, 749–756. doi: 10.2131/jts.35.749
Takeda, K., Suzuki, K. I., Ishihara, A., Kubo-Irie, M., Fujimoto, R., Tabata, M., et al. (2009). Nanoparticles transferred from pregnant mice to their offspring can damage the genital and cranial nerve systems. J. Health Sci. 55, 95–102. doi: 10.1248/jhs.55.95
Tassinari, R., Cubadda, F., Moracci, G., Aureli, F., D'Amato, M., Valeri, M., et al. (2014). Oral, short-term exposure to titanium dioxide nanoparticles in Sprague-Dawley rat: focus on reproductive and endocrine systems and spleen. Nanotoxicology 8, 654–662. doi: 10.3109/17435390.2013.822114
Thiesen, B., and Jordan, A. (2008). Clinical applications of magnetic nanoparticles for hyperthermia. Int. J. Hyperther. 24, 467–474. doi: 10.1080/02656730802104757
Tian, X., Zhu, M., Du, L., Wang, J., Fan, Z., Liu, J., et al. (2013). Intrauterine inflammation increases materno-fetal transfer of gold nanoparticles in a size-dependent manner in murine pregnancy. Small 9, 2432–2439. doi: 10.1002/smll.201300817
Tong, V. T., Dietz, P. M., Morrow, B., D'Angelo, D. V., Farr, S. L., Rockhill, K. M., et al. (2013). Trends in smoking before, during, and after pregnancy—Pregnancy Risk Assessment Monitoring System, United States, 40 sites, 2000–2010. MMWR Surveill. Summ. 62, 1–19. Available online at: http://www.jstor.org/stable/24806088
Tsuchiya, T., Oguri, I., Yamakoshi, Y. N., and Miyata, N. (1996). Novel harmful effects of [60] fullerene on mouse embryos in vitro and in vivo. FEBS Lett. 393, 139–145. doi: 10.1016/0014-5793(96)00812-5
Tsukue, N., Tsubone, H., and Suzuki, A. K. (2002). Diesel exhaust affects the abnormal delivery in pregnant mice and the growth of their young. Inhal. Toxicol. 14, 635–651. doi: 10.1080/08958370290084548
Umezawa, M., Kudo, S., Yanagita, S., Shinkai, Y., Niki, R., Oyabu, T., et al. (2011). Maternal exposure to carbon black nanoparticle increases collagen type VIII expression in the kidney of offspring. J. Toxicol. Sci. 36, 461–468. doi: 10.2131/jts.36.461
Umezawa, M., Tainaka, H., Kawashima, N., Shimizu, M., and Takeda, K. (2012). Effect of fetal exposure to titanium dioxide nanoparticle on brain development− brain region information. J. Toxicol. Sci. 37, 1247–1252. doi: 10.2131/jts.37.1247
Vance, M. E., Kuiken, T., Vejerano, E. P., McGinnis, S. P., Hochella, M. F. Jr., Rejeski, D., et al. (2015). Nanotechnology in the real world: redeveloping the nanomaterial consumer products inventory. Beilstein J. Nanotechnol. 6, 1769–1780. doi: 10.3762/bjnano.6.181
Walkey, C. D., and Chan, W. C. (2012). Understanding and controlling the interaction of nanomaterials with proteins in a physiological environment. Chem. Soc. Rev. 41, 2780–2799. doi: 10.1039/C1CS15233E
Wang, B., He, X., Zhang, Z., Zhao, Y., and Feng, W. (2012). Metabolism of nanomaterials in vivo: blood circulation and organ clearance. Acc. Chem. Res. 46, 761–769. doi: 10.1021/ar2003336
Wang, J., Liu, Y., Jiao, F., Lao, F., Li, W., Gu, Y., et al. (2008). Time-dependent translocation and potential impairment on central nervous system by intranasally instilled TiO 2 nanoparticles. Toxicology 254, 82–90. doi: 10.1016/j.tox.2008.09.014
Wang, J., Zhou, G., Chen, C., Yu, H., Wang, T., Ma, Y., et al. (2007). Acute toxicity and biodistribution of different sized titanium dioxide particles in mice after oral administration. Toxicol. Lett. 168, 176–185. doi: 10.1016/j.toxlet.2006.12.001
Wang, J., Zhu, X., Zhang, X., Zhao, Z., Liu, H., George, R., et al. (2011). Disruption of zebrafish (Danio rerio) reproduction upon chronic exposure to TiO 2 nanoparticles. Chemosphere 83, 461–467. doi: 10.1016/j.chemosphere.2010.12.069
Warren, M. P., and Perlroth, N. E. (2001). The effects of intense exercise on the female reproductive system. J. Endocrinol. 170, 3–11. doi: 10.1677/joe.0.1700003
Watanabe, N. (2005). Decreased number of sperms and Sertoli cells in mature rats exposed to diesel exhaust as fetuses. Toxicol. Lett. 155, 51–58. doi: 10.1016/j.toxlet.2004.08.010
Wells, P. G., Bhuller, Y., Chen, C. S., Jeng, W., Kasapinovic, S., Kennedy, J. C., et al. (2005). Molecular and biochemical mechanisms in teratogenesis involving reactive oxygen species. Toxicol. Appl. Pharmacol. 207, 354–366. doi: 10.1016/j.taap.2005.01.061
Wennerberg, A., Jimbo, R., Allard, S., Skarnemark, G., and Andersson, M. (2011). in vivo stability of hydroxyapatite nanoparticles coated on titanium implant surfaces. Int. J. Oral Maxillofac. Implants 26, 1161–1166.
Whidbey, C., Harrell, M. I., Burnside, K., Ngo, L., Becraft, A. K., Iyer, L. M., et al. (2013). A hemolytic pigment of Group B Streptococcus allows bacterial penetration of human placenta. J. Exp. Med. 210, 1265–1281. doi: 10.1084/jem.20122753
Wick, P., Malek, A., Manser, P., Meili, D., Maeder-Althaus, X., Diener, L., et al. (2010). Barrier capacity of human placenta for nanosized materials. Environ. Health Perspect. 118, 432–436. doi: 10.1289/ehp.0901200
Wiesenthal, A., Hunter, L., Wang, S., Wickliffe, J., and Wilkerson, M. (2011). Nanoparticles: small and mighty. Int. J. Dermatol. 50, 247–254. doi: 10.1111/j.1365-4632.2010.04815.x
Wiwanitkit, V., Sereemaspun, A., and Rojanathanes, R. (2009). Effect of gold nanoparticles on spermatozoa: the first world report. Fertil. Steril. 91, e7–e8. doi: 10.1016/j.fertnstert.2007.08.021
Wu, J., Liu, W., Xue, C., Zhou, S., Lan, F., Bi, L., et al. (2009). Toxicity and penetration of TiO 2 nanoparticles in hairless mice and porcine skin after subchronic dermal exposure. Toxicol. Lett. 191, 1–8. doi: 10.1016/j.toxlet.2009.05.020
Wu, J., Wang, C., Sun, J., and Xue, Y. (2011). Neurotoxicity of silica nanoparticles: brain localization and dopaminergic neurons damage pathways. ACS Nano 5, 4476–4489. doi: 10.1021/nn103530b
Xia, T., Kovochich, M., Brant, J., Hotze, M., Sempf, J., Oberley, T., et al. (2006). Comparison of the abilities of ambient and manufactured nanoparticles to induce cellular toxicity according to an oxidative stress paradigm. Nano Lett. 6, 1794–1807. doi: 10.1021/nl061025k
Xia, T., Kovochich, M., Liong, M., Meng, H., Kabehie, S., George, S., et al. (2009). Polyethyleneimine coating enhances the cellular uptake of mesoporous silica nanoparticles and allows safe delivery of siRNA and DNA constructs. ACS Nano 3, 3273–3286. doi: 10.1021/nn900918w
Xue, Z., Liang, D., Li, Y., Long, Z., Pan, Q., Liu, X., et al. (2005). Silica nanoparticle is a possible safe carrier for gene therapy. Chin. Sci. Bull. 50, 2323–2327. doi: 10.1007/BF03183743
Yah, C. S., Simate, G. S., and Iyuke, S. E. (2012). Nanoparticles toxicity and their routes of exposures. Pak. J. Pharm. Sci. 25, 477–491.
Yamashita, K., Yoshioka, Y., Higashisaka, K., Mimura, K., Morishita, Y., Nozaki, M., et al. (2011). Silica and titanium dioxide nanoparticles cause pregnancy complications in mice. Nat. Nanotechnol. 6, 321–328. doi: 10.1038/nnano.2011.41
Yang, H., Sun, C., Fan, Z., Tian, X., Yan, L., Du, L., et al. (2012). Effects of gestational age and surface modification on materno-fetal transfer of nanoparticles in murine pregnancy. Sci. Rep. 2:847. doi: 10.1038/srep00847
Yang, L., Kuang, H., Zhang, W., Aguilar, Z. P., Xiong, Y., Lai, W., et al. (2015). Size dependent biodistribution and toxicokinetics of iron oxide magnetic nanoparticles in mice. Nanoscale 7, 625–636. doi: 10.1039/C4NR05061D
Yauk, C., Polyzos, A., Rowan-Carroll, A., Somers, C. M., Godschalk, R. W., Van Schooten, F. J., et al. (2008). Germ-line mutations, DNA damage, and global hypermethylation in mice exposed to particulate air pollution in an urban/industrial location. Proc. Natl. Acad. Sci. U.S.A. 105, 605–610. doi: 10.1073/pnas.0705896105
Yokota, S., Mizuo, K., Moriya, N., Oshio, S., Sugawara, I., and Takeda, K. (2009). Effect of prenatal exposure to diesel exhaust on dopaminergic system in mice. Neurosci. Lett. 449, 38–41. doi: 10.1016/j.neulet.2008.09.085
Yoshida, S., Hiyoshi, K., Ichinose, T., Takano, H., Oshio, S., Sugawara, I., et al. (2009). Effect of nanoparticles on the male reproductive system of mice. Int. J. Androl. 32, 337–342. doi: 10.1111/j.1365-2605.2007.00865.x
Yoshida, S., Hiyoshi, K., Oshio, S., Takano, H., Takeda, K., and Ichinose, T. (2010). Effects of fetal exposure to carbon nanoparticles on reproductive function in male offspring. Fertil. Steril. 93, 1695–1699. doi: 10.1016/j.fertnstert.2009.03.094
Zhang, L., Gu, F. X., Chan, J. M., Wang, A. Z., Langer, R. S., and Farokhzad, O. C. (2008). Nanoparticles in medicine: therapeutic applications and developments. Clin. Pharmacol. Ther. 83, 761–769. doi: 10.1038/sj.clpt.6100400
Zhang, W., Wang, C., Li, Z., Lu, Z., Li, Y., Yin, J. J., et al. (2012). Unraveling stress-induced toxicity properties of graphene oxide and the underlying mechanism. Adv. Mater. 24, 5391–5397. doi: 10.1002/adma.201202678
Zhang, Y., Bai, Y., Jia, J., Gao, N., Li, Y., Zhang, R., et al. (2014). Perturbation of physiological systems by nanoparticles. Chem. Soc. Rev. 43, 3762–3809. doi: 10.1039/C3CS60338E
Zhao, C., Liao, J., Chu, W., Wang, S., Yang, T., Tao, Y., et al. (2012). Involvement of TLR2 and TLR4 and Th1/Th2 shift in inflammatory responses induced by fine ambient particulate matter in mice. Inhal. Toxicol. 24, 918–927. doi: 10.3109/08958378.2012.731093
Zhao, F., Zhao, Y., Liu, Y., Chang, X., Chen, C., and Zhao, Y. (2011). Cellular uptake, intracellular trafficking, and cytotoxicity of nanomaterials. Small 7, 1322–1337. doi: 10.1002/smll.201100001
Zhao, X., Ze, Y., Gao, G., Sang, X., Li, B., Gui, S., et al. (2013). Nanosized TiO 2-induced reproductive system dysfunction and its mechanism in female mice. PLoS ONE 8:e59378. doi: 10.1371/journal.pone.0059378
Zhu, M. T., Feng, W. Y., Wang, Y., Wang, B., Wang, M., Ouyang, H., et al. (2009). Particokinetics and extrapulmonary translocation of intratracheally instilled ferric oxide nanoparticles in rats and the potential health risk assessment. Toxicol. Sci. 107, 342–351. doi: 10.1093/toxsci/kfn245
Keywords: nanoparticles, reproduction, animal models, human health, toxicity
Citation: Brohi RD, Wang L, Talpur HS, Wu D, Khan FA, Bhattarai D, Rehman Z-U, Farmanullah F and Huo L-J (2017) Toxicity of Nanoparticles on the Reproductive System in Animal Models: A Review. Front. Pharmacol. 8:606. doi: 10.3389/fphar.2017.00606
Received: 11 February 2017; Accepted: 21 August 2017;
Published: 05 September 2017.
Edited by:
Eleonore Fröhlich, Medical University of Graz, AustriaReviewed by:
Alberto Mantovani, Istituto Superiore di Sanità, ItalyCopyright © 2017 Brohi, Wang, Talpur, Wu, Khan, Bhattarai, Rehman, Farmanullah and Huo. This is an open-access article distributed under the terms of the Creative Commons Attribution License (CC BY). The use, distribution or reproduction in other forums is permitted, provided the original author(s) or licensor are credited and that the original publication in this journal is cited, in accordance with accepted academic practice. No use, distribution or reproduction is permitted which does not comply with these terms.
*Correspondence: Li-Jun Huo, bGpodW9AbWFpbC5oemF1LmVkdS5jbg==; bGlqdW5odW9AeWFob28uY29t
Disclaimer: All claims expressed in this article are solely those of the authors and do not necessarily represent those of their affiliated organizations, or those of the publisher, the editors and the reviewers. Any product that may be evaluated in this article or claim that may be made by its manufacturer is not guaranteed or endorsed by the publisher.
Research integrity at Frontiers
Learn more about the work of our research integrity team to safeguard the quality of each article we publish.