- 1Graduate Program in Translational Biology, Medicine, and Health, Virginia Polytechnic Institute and State University, Roanoke, VA, United States
- 2Department of Biomedical Engineering and Mechanics, Virginia Polytechnic Institute and State University, Blacksburg, VA, United States
- 3Department of Electrical and Computer Engineering, Virginia Polytechnic Institute and State University, Blacksburg, VA, United States
- 4Department of Biomedical Sciences and Pathobiology, Virginia-Maryland College of Veterinary Science, Blacksburg, VA, United States
New therapeutic strategies and paradigms are direly needed for the treatment of cancer. While the surgical removal of tumors is favored in most cancer treatment plans, resection options are often limited based on tumor localization. Over the last two decades, multiple tumor ablation strategies have emerged as promising stand-alone or combination therapeutic options for patients. These strategies are often employed to treat tumors in areas where surgical resection is not possible or where chemotherapeutics have proven ineffective. The type of cell death induced by the ablation modality is a critical aspect of therapeutic success that can impact the efficacy of the treatment and systemic anti-tumor immune system responses. Electroporation-based ablation technologies include electrochemotherapy, irreversible electroporation, and other modalities that rely on pulsed electric fields to create pores in cell membranes. These pores can either be reversible or irreversible depending on the electric field parameters and can induce cell death either alone or in combination with a therapeutic agent. However, there have been many controversial findings among these technologies as to the cell death type initiated, from apoptosis to pyroptosis. As cell death mechanisms can impact treatment side effects and efficacy, we review the main types of cell death induced by electroporation-based treatments and summarize the impact of these mechanisms on treatment response. We also discuss potential reasons behind the variability of findings such as the similarities between cell death pathways, differences between cell-types, and the variation in electric field strength across the treatment area.
Introduction
Despite improvements in survival and quality of life provided by current therapeutic practices, cancer death rates remain unacceptably high (1). New treatment paradigms are direly needed. Minimally invasive tumor ablation treatments such as cryotherapy, laser irradiation, microwave irradiation, radiofrequency ablation, high-intensity focused ultrasound ablation, and irreversible electroporation (IRE), have shown significant promise (2, 3). Ablation modalities function through the direct or indirect induction of cell death, resulting in the destruction of tissue by thermal, mechanical, or electrical means. Thermal ablation modalities, such as microwave or radiofrequency, use intense temperatures to lyse cells and induce apoptosis in treatment margins (4). Unfortunately, tumor location near critical structures can lead to hemorrhaging, heat sink effects, and healthy tissue damages during treatment (5, 6). Non-thermal modalities such as those that utilize electroporation can overcome these treatment barriers to induce cell death through different mechanisms and may initiate systemic immune responses to target both the local tumor microenvironment and metastatic sites (7–10).
Extensive investigations have explored the mechanisms underlying cell death induced by pore formation and homeostasis loss following electroporation-based tumor ablation treatments (11, 12). However, it should be noted that thresholds for electroporation, both reversible and irreversible, and cell death induction can differ based on cell-type (13–16). This may be why studies utilizing electroporation-based treatments report different cell death mechanisms ranging from apoptosis to pyroptosis (8, 17). Cell death is a complex and nuanced process, especially in the context of cancer. The type of cell death induced can have significant biological and physiological consequences in terms of local and systemic treatment efficacy. Here, we review these four major types of cell death, offer insight into the biological impacts of each on tumor ablation, and summarize cell death findings from electroporation-based treatment strategies.
Cell Death Mechanisms in Electroporation-Based Treatments
Cell death can diverge into many pathways, eliciting a large range of responses (Figure 1). Each pathway can be further modified by genetic, epigenetic, and regulatory factors for different cell types and tissues. These pathways are not singular; many similar proteins and biochemical pathways have been shown to be involved with multiple cell death subroutines, making it difficult to ascertain a definitive type of cell death (18, 19). Inducing the optimum type of cell death is critical for effective tumor treatment as it can influence both local and systemic effects that significantly impact cancer recurrence, inflammation, and autoimmunity (20–24).
Apoptosis
Apoptosis is one of the most commonly mentioned forms of cell death in electroporation-based ablations (25–28). Generally considered non-inflammatory, apoptosis is a programmed form of cell death required for normal maintenance of tissues such as intestinal epithelium or epidermis where cells are regularly replaced to avoid the accumulation of cellular damage or mutations (29–31). This pathway is commonly dysregulated in cancers as the cells lose the ability to respond to internal signals due to mutations in apoptosis regulatory pathways or key genes such as Bcl2 or p53 (32, 33). The induction of apoptosis implies a “quiet cell death” with little immune involvement beyond dead tissue clearance. Hallmark features include the cleaving and activation of Caspase 3 and 7 along with the expression of phosphatidylserine on the cellular surface that binds Annexin V (34–36). In tumor ablation, apoptosis can be considered beneficial due to the reduced potential for inflammation-driven damage to nearby healthy tissues as it leads to the suppression of inflammatory signaling (37). While apoptosis can be highly effective in ablating primary tumors, the lack of innate and adaptive immune system activation is sub-optimal for inducing systemic anti-tumor immune responses. This can negatively impact the potential to induce an abscopal effect to target metastatic lesions and may create a permissive niche for tumor reoccurrence once the apoptotic pressure is removed (38, 39).
Necrosis
Necrosis lies opposite of apoptosis and involves rapid cell death. Necrosis is typically induced by sudden loss in cell homeostasis, such as rapid osmolarity or temperature changes, influx of calcium into the cell or mitochondrial spaces, or mechanical tissue damages that can lead to autolysis (40). It should be noted that this section refers to cellular necrosis, which differs from “tissue necrosis,” or irreversible tissue injury, the presence of dead tissue often based on acellularity or tissue morphology and does not specify specific cell death mechanisms.
Necrosis is often referred to as accidental or lytic cell death and is characterized by the breakdown of the cell membrane and the release of large amounts of damage-associated molecular patterns (DAMPs) (38, 41). Further investigation into necrosis's mechanisms show that contrary to the chaotic description, its mechanism may also be regulated by specific programs similar to apoptosis (41). For example, the serine/threonine kinase RIP1 appears to play a pivotal role as a central initiator of necrosis (41). Activation of RIP1 results in NF-κB and transient MAPK signaling, directly inducing the production of pro-inflammatory mediators (42–44). RIP1 may also play a role in the TNF signaling and the generation of ceramide during necrosis (45). Likewise, calcium and reactive oxygen species (ROS) signaling cascades lead to the propagation and execution phases of cell death. Ultimately, these result in damaged proteins, lipids, and DNA that drive necrosis and are released upon cell death (41, 46). The production of DAMPs stimulate neighboring cells to activate the innate immune system, resulting in inflammation (47). Unfortunately, inflammatory signaling recruits immune cells to the lesion that may be polarized to facilitate regeneration growth and repair which the tumor can reprogram to assist the tumor in grow, repair, and create a more favorable niche for progression (48, 49). Necrosis may also induce tumor lysis syndrome (TLS) as large numbers of DAMPs acutely released into the blood stream can lead to systemic inflammation (50–52). Any tumor ablation modality that induces high DAMP production should focus on optimizing targeting and minimizing the treatment area. It should be noted that TLS has not been reported in electroporation-based treatment clinical trials.
Pyroptosis
Pyroptosis is one of twelve identified subclasses of regulated cell death and displays high local inflammatory responses distinct from necrosis (36). Traditionally, pyroptosis is associated with the host innate immune response to viral and bacterial pathogens (53–56). Pyroptosis is an extremely specific form of inflammatory programmed cell death and is characterized by the cleavage and activation of Caspase-1 and Caspase-11 (55, 57). Caspase-1 activation results in the cleavage and processing of IL-1β and IL-18, potent proinflammatory cytokines. These caspases also cleave gasdermin D, generating an N-terminal cleavage product that drives pyroptosis (58). In addition to IL-1β and IL-18, pyroptosis produces a significant amount of DAMPs, including HMGB1, ATP, and ROS to further stimulate the innate immune system (56, 59, 60). This high signaling state leads to rapid responses from the body with recruitment of immune cells to the local area as well as increased systemic signaling to enhance immunosurveillance and heighten antigen presentation potential that could be beneficial for cancer treatment (61). While this can be ideal for allowing the immune system to recognize tumor cells in the body and create immune memory, the heightened inflammatory state can lead to severe side effects such as fever and autoimmunity (21, 51).
Necroptosis
In addition to pyroptosis, a fourth major regulated cell death routine termed necroptosis has also been described following irreversible electroporation. Necroptosis is also termed programmed necrosis or alternative necrosis and has features characteristic to both apoptosis and necrosis. Necroptosis is triggered by perturbations of extracellular or intracellular homeostasis and does not induce a quick, automatic lysis of the cell (62). Rather, the cell produces low levels of DAMPs and proinflammatory cytokines that drive moderate levels of inflammation compared to much more inflammatory mechanisms associated with pyroptosis (19). Necroptosis critically depends on the pseudo-kinase mixed lineage kinase domain-like (MLKL) protein, which is phosphorylated by the kinase RIPK3 (63). While mechanistically less clear, RIPK1 has also been suggested to mediate necroptosis under some conditions and the activation of an inflammasome has been suggested to underlie inflammatory cytokine production (36, 64). This can make it somewhat challenging to discern from necrosis. However, due to the temporal nature of necroptosis and the attenuated production of local cytokines, necroptosis is less likely to induce TLS but still retains the potential of inducing inflammation that can promote an anti-tumor microenvironment and improve antigen presentation.
Electroporation-Based Ablation Modalities
Electrochemotherapy (ECT) and Calcium-Based Electroporation
ECT was one of the first electroporation-based modalities to progress to clinical trials and is routinely used in Europe for cutaneous malignancies at over 150 centers (65–67). ECT utilizes pulsed electric fields (PEFs) of micro- to nanosecond pulse durations for short periods that lead to reversible electroporation and allow for the internal delivery of chemotherapeutic reagents to the site of treatment (68, 69). Many chemotherapeutic agents such as bleomycin or cisplatin can have off-target effects, difficulty penetrating beyond the surface of the tumor, and the potential for chemoresistance (70, 71). Thus, ECT facilitates using local or acute systemic application of smaller doses of the chemotherapeutic, which can reduce side effects, and increases cellular uptake of the chemotherapeutics in the treatment zone (72–74). Cell death via ECT varies based on the chemotherapy's mechanism of action, but reversible electroporation alone does not lead to significant cell death or tumor ablation (75). ECT has been shown to induce apoptosis-like cell death with bleomycin in head and neck carcinoma, necroptosis with bleomycin, cisplatin, and oxaliplatin in pancreatic cancer, and evidence of pyroptosis-like immunogenic cell death when applied with bleomycin in colon cancer (76–78). This is an interesting observation in the variability of cell death mechanisms in different cell types utilizing the same chemotherapeutic. Adjusting chemotherapeutic choice or even dosage for specific malignancies may alter cell death mechanisms and enhance patient outcomes.
Expanding on ECT strategies, the use of calcium represents another option to this treatment strategy. Calcium electroporation enhances necrosis in the treatment zone and its application can forgo or reduce the use of chemotherapeutics and avoid some chemotherapy side effects (79, 80). The induction of necrosis by calcium influx may overcome the chemoresistance mechanisms involving DNA repair and altered survival-linked proteins such as Bcl-2 in some cancers (79, 81–83). Furthermore, there is potential selectivity in calcium electroporation as malignant cells are more likely to die than healthy or benign cells (84, 85). This may be due to the modification many cancers develop to avoid cell death that make them susceptible to calcium imbalances (86, 87).
Irreversible Electroporation (IRE)
IRE utilizes microsecond pulse electric fields, similar to ECT, but for a higher pulse count. This higher pulse count results in pores and tears forming in the cell membrane that stabilize, leading to loss of cell homeostasis and initiating cell death processes (12, 88). The use of IRE in difficult-to-treat malignancies, such as pancreatic and hepatocellular tumors, is currently becoming more widespread and has led to numerous clinical trials showing inspiringly high success rates (89–95).
IRE-induced cell death was originally considered to be apoptotic (25, 27, 28, 96). However, a majority of these studies did not fully consider alternative cell death types. While apoptosis is certainly occurring in some cells within the treatment zone, our data suggest that treatment could initiate multiple types of cell death mechanisms, though the size and shape of the regions in which each type is experienced may vary between clinical treatments due to differences in pulsing parameters, tissue type, and treatment time [Figure 2; (97, 98)]. This is mediated, in part, by the proximity of the cells to the electrodes, which impacts the voltage each cell experiences during treatment (28, 99–101). While directly near the electrodes may be temperature dependent, cell death becomes temperature independent in other regions based on minimum heating effects seen in vitro at comparable electric field magnitudes (7). Multiple studies argued that there may be more than one type of cell death mechanism at play, from necrotic cell death to apoptotic-like non-apoptotic cell death (17, 102–104). These responses could come from differing tissues being predisposed to specific types of cell death depending on the electric field strength applied (105). Likewise, for cells at the margins of the treatment areas, the response may actually be survival signaling to reversible electroporation. In theory, this could be taken advantage of and combined with chemotherapy treatments to increase drug delivery, tumor penetration, and treatment of remnant cancer cells (106–108).
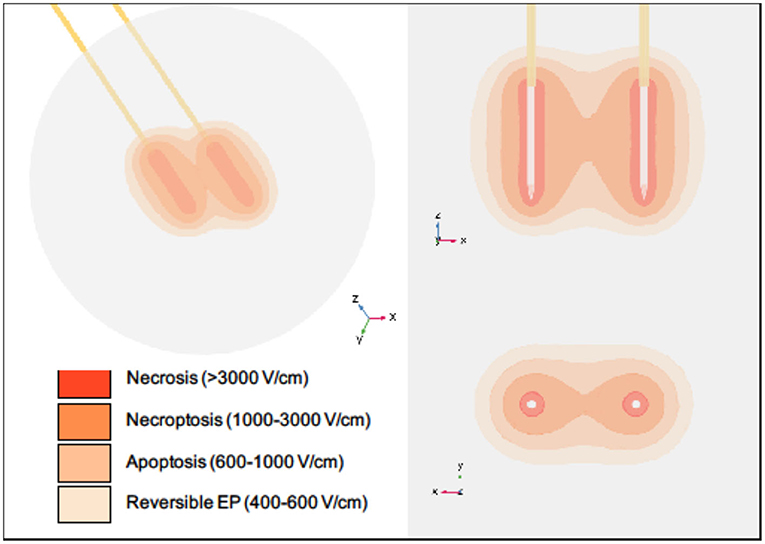
Figure 2. Regions of cell death within a typical IRE treatment zone vary spatially. Necrosis is thought to occur in close proximity to the electrodes during pulse delivery, while necroptosis, apoptosis, and reversible electroporation are thought to occur with increasing distances from the electrodes. It should be noted that this diagram is intended for illustrative purposes only. Specific volumes and thresholds will vary with many parameters, including electrode spacing and exposure, voltage applied, tissue type, number of pulses applied, and time after treatment. The electric field distribution for a single IRE pulse was simulated using COMSOL Multiphysics software (version 5.5, Burlington, MA). Two stainless steel electrodes with insulating holders were inserted into a spherical tissue domain, which was assigned a dynamic electrical conductivity corresponding with normal human pancreatic tissue (97). Electrodes were placed 1.5 cm apart with 1.5 cm exposure, and a voltage of 3,000 V was applied.
High-Frequency Irreversible Electroporation (H-FIRE)
Building upon the advantages of IRE, a novel technology termed high-frequency irreversible electroporation (H-FIRE) utilizes 1–10 μs bipolar bursts applied in a pattern with interpulse delay when switching poles. These parameters are highly effective in non-thermally ablating tissues without causing the muscle contractions and heart dysrhythmia associated with the long-duration monophasic pulses of IRE (8, 109–112). Its use in pre-clinical models has progressed without the need for cardiac synchronization as well (112). Cell death following H-FIRE is considered to be highly similar to IRE. However, pre-clinical studies show that H-FIRE may be eliciting immunologic cell death and pyroptosis in addition to apoptosis and necrosis (8, 104). Intriguingly, the use of H-FIRE may allow tuning of cell death with calcium similar to calcium ECT. In in vitro hydrogel studies, H-FIRE applied in calcium-rich media showed a significant shift toward necrotic cell death with higher lesion areas and fewer survival signals (113). These data suggest that H-FIRE effects could be modified by injecting calcium or sucrose near the treatment site to allow for controlled applications in difficult-to-treat malignancies or tissue locations.
Nanosecond Pulsed Electric Fields (nsPEFs)
Nanosecond pulse electric fields (nsPEFs) are characterized by PEFs with short nanosecond pulse durations and high electric fields. Originally, nsPEFs were thought not to permeabilize the cell membrane but rather induce cell death by interfering with molecular patterns inside the cell, disrupting processes in the mitochondria, and other intracellular membrane-bound organelles (114). However, later studies have found that cell membranes and the membranes of internal cellular structures are permeabilized, albeit with smaller tears than those of microsecond PEFs (26, 115–117). nsPEFs have been noted to induce both apoptotic and potentially caspase-independent apoptotic-like cell death similar to necroptosis (116, 117). Recent in vivo findings provide greater mechanistic insight into the types of cell death induced by nsPEFs (118–120). Based on the inflammatory and immunomodulatory conditions, these studies reveal that nsPEFs may induce programmed necrosis or necroptotic cell death in breast, melanoma, and pancreatic cancers (118–120).
Discussion
As electroporation ablation modalities become more mainstream and progress from preclinical studies to clinical applications, characterization of specific cell death mechanisms associated with treatment will become more relevant. The type of cell death has significant consequences for the patient's treatment outcomes, immune system activation, regeneration and repair processes, and co-therapy applications. Seemingly conflicting reports on cell death mechanisms ranging from apoptosis to necrosis and pyroptosis has led to confusion in the field. However, these data suggest that cell death following electroporation may be dependent on electric field and cell type, and that multiple cell death mechanisms could occur within the treatment zone. While studies investigating the effects of related cell lines with varying morphology suggest that cells with larger nuclei are more likely to be affected by H-FIRE, whether these findings are consistent among different electroporation modalities remains to be seen (14, 15). It would be reasonable to hypothesize that different tissues and cell types, both healthy and malignant, have different responses to electroporation-based technologies and may require altering dosing, treatment parameters, or co-therapies to obtain optimal effects. In fact, a recent study on pancreatic cancer shows differences in cell death mechanisms initiated by IRE and HFIRE with similar parameters (104). Further investigation into these cell death mechanisms among electroporation-based technologies may help tailor these treatments for personalized medicine.
Author Contributions
RB, NB-W, RD, and IA contributed to the writing and editing of the manuscript. All authors contributed to the article and approved the submitted version.
Funding
This work was supported by the Virginia-Maryland College of Veterinary Medicine (IA), the Virginia Tech Institute for Critical Technology and Applied Science Center for Engineered Health (IA and RD), the Virginia Biosciences Health Research Corporation Catalyst (RD), and the National Institutes of Health R01CA213423 (RD), P01CA207206 (RD), and R21EB028429 (IA). The content is solely the responsibility of the authors and does not necessarily represent the official views of the NIH or any other funding agency.
Conflict of Interest
IA, NB-W, and RD are inventors on pending and issued patents related to the work.
The remaining author declares that the research was conducted in the absence of any commercial or financial relationships that could be construed as a potential conflict of interest.
Acknowledgments
We would like to thank Alissa Hendricks-Wenger and Nastaran Alinezhadbalalami for their critical reviews of the manuscript. We would also like to thank Sheryl Coutermarsh-Ott for her professional consultation for this manuscript.
References
2. Wells SA, Hinshaw JL, Lubner MG, Ziemlewicz TJ, Brace CL, Lee FT Jr. Liver ablation: best practice. Radiol Clin North Am. (2015) 53:933–71. doi: 10.1016/j.rcl.2015.05.012
3. Paiella S, De Pastena M, D'Onofrio M, Crinò SF, Pan TL, De Robertis R, et al. Palliative therapy in pancreatic cancer-interventional treatment with radiofrequency ablation/irreversible electroporation. Transl Gastroenterol Hepatol. (2018) 3:80. doi: 10.21037/tgh.2018.10.05
4. Saccomandi P, Lapergola A, Longo F, Schena E, Quero G. Thermal ablation of pancreatic cancer: a systematic literature review of clinical practice and pre-clinical studies. Int J Hyperthermia. (2018) 35:398–418. doi: 10.1080/02656736.2018.1506165
5. Chen M-H, Yang W, Yan K, Gao W, Dai Y, Wang Y-B, et al. Treatment efficacy of radiofrequency ablation of 338 patients with hepatic malignant tumor and the relevant complications. World J Gastroenterol. (2005) 11:6395–401. doi: 10.3748/wjg.v11.i40.6395
6. Liang P, Wang Y, Yu X, Dong B. Malignant liver tumors: treatment with percutaneous microwave ablation—complications among cohort of 1136 patients. Radiology. (2009) 251:933–40. doi: 10.1148/radiol.2513081740
7. Davalos RV, Bhonsle S, Neal Ii RE. Implications and considerations of thermal effects when applying irreversible electroporation tissue ablation therapy. Prostate. (2015) 75:1114–8. doi: 10.1002/pros.22986
8. Ringel-Scaia VM, Beitel-White N, Lorenzo MF, Brock RM, Huie KE, Coutermarsh-Ott S, et al. High-frequency irreversible electroporation is an effective tumor ablation strategy that induces immunologic cell death and promotes systemic anti-tumor immunity. EBioMedicine. (2019) 44:112–25. doi: 10.1016/j.ebiom.2019.05.036
9. Shao Q, O'Flanagan S, Lam T, Roy P, Pelaez F, Burbach BJ, et al. Engineering T cell response to cancer antigens by choice of focal therapeutic conditions. Int J Hyperthermia. (2019) 36:130–8. doi: 10.1080/02656736.2018.1539253
10. Geboers B, Scheffer HJ, Graybill PM, Ruarus AH, Nieuwenhuizen S, Puijk RS, et al. High-voltage electrical pulses in oncology: irreversible electroporation, electrochemotherapy, gene electrotransfer, electrofusion, and electroimmunotherapy. Radiology. (2020) 295:254–72. doi: 10.1148/radiol.2020192190
11. Weaver JC, Chizmadzhev YA. Theory of electroporation: a review. Bioelectrochem Bioenerget. (1996) 41:135–60.
12. Davalos RV, Mir LM, Rubinsky B. Tissue ablation with irreversible electroporation. Ann Biomed Eng. (2005) 33:223–31. doi: 10.1007/s10439-005-8981-8
13. Methods N. Transfection of mammalian cells by electroporation. Nat Methods. (2006) 3:67–8. doi: 10.1038/nmeth0106-67
14. Ivey JW, Latouche EL, Sano MB, Rossmeisl JH, Davalos RV, Verbridge SS. Targeted cellular ablation based on the morphology of malignant cells. Nat Publish Group. (2015). doi: 10.1038/srep17157
15. Rolong A, Schmelz EM, Davalos RV. High-frequency irreversible electroporation targets resilient tumor-initiating cells in ovarian cancer. Integr Biol. (2017) 9:979–87. doi: 10.1039/c7ib00116a
16. Shao Q, Liu F, Chung C, Elahi-Gedwillo K, Provenzano PP, Forsyth B, et al. Physical and chemical enhancement of and adaptive resistance to irreversible electroporation of pancreatic cancer. Ann Biomed Eng. (2018) 46:25–36. doi: 10.1007/s10439-017-1932-3
17. Piñero J, López-Baena M, Ortiz T, Cortés F. Apoptotic and necrotic cell death are both induced by electroporation in HL60 human promyeloid leukaemia cells. Apoptosis. (1997) 2:330–6. doi: 10.1023/A:1026497306006
18. Vanden Berghe T, Kaiser WJ, Bertrand MJ, Vandenabeele P. Molecular crosstalk between apoptosis, necroptosis, and survival signaling. Mol Cell Oncol. (2015) 2:e975093. doi: 10.4161/23723556.2014.975093
19. Frank D, Vince JE. Pyroptosis versus necroptosis: similarities, differences, and crosstalk. Cell Death Differ. (2019) 26:99–114. doi: 10.1038/s41418-018-0212-6
20. Hanahan D, Weinberg RA. Hallmarks of cancer: the next generation. Cell. (2011) 144:646–74. doi: 10.1016/j.cell.2011.02.013
21. Baroja-Mazo A, Martín-Sánchez F, Gomez AI, Martínez CM, Amores-Iniesta J, Compan V, et al. The NLRP3 inflammasome is released as a particulate danger signal that amplifies the inflammatory response. Nat Immunol. (2014) 15:738–48. doi: 10.1038/ni.2919
22. Zhang J, Wolfgang CL, Zheng L. Precision immuno-oncology: prospects of individualized immunotherapy for pancreatic cancer. Cancers. (2018) 10:39. doi: 10.3390/cancers10020039
23. Aizawa S, Brar G, Tsukamoto H. Cell death and liver disease. Gut Liver. (2020) 14:20–9. doi: 10.5009/gnl18486
24. Khoury MK, Gupta K, Franco SR, Liu B. Necroptosis in the pathophysiology of disease. Am J Pathol. (2020) 190:272–85. doi: 10.1016/j.ajpath.2019.10.012
25. Hofmann F, Ohnimus H, Scheller C, Strupp W, Zimmermann U, Jassoy C. Electric field pulses can induce apoptosis. J Memb Biol. (1999) 169:103–9. doi: 10.1007/s002329900522
26. Vernier PT, Aimin L, Marcu L, Craft CM, Gundersen MA. Ultrashort pulsed electric fields induce membrane phospholipid translocation and caspase activation: differential sensitivities of Jurkat T lymphoblasts and rat glioma C6 cells. IEEE Trans Dielectr Electr Insulat. (2003) 10:795–809. doi: 10.1109/TDEI.2003.1237329
27. Lee EW, Chen C, Prieto VE, Dry SM, Loh CT, Kee ST. Advanced hepatic ablation technique for creating complete cell death: irreversible electroporation. Radiology. (2010) 255:426–33. doi: 10.1148/radiol.10090337
28. Faroja M, Ahmed M, Appelbaum L, Ben-David E, Moussa M, Sosna J, et al. Irreversible electroporation ablation: Is all the damage nonthermal? Radiology. (2013) 266:462–70. doi: 10.1148/radiol.12120609
29. Kerr JFR, Wyllie AH, Currie AR. Apoptosis: a basic biological phenomenon with wideranging implications in tissue kinetics. Br J Cancer. (1972) 26:239–57. doi: 10.1038/bjc.1972.33
30. Johnstone RW, Ruefli AA, Lowe SW. Apoptosis. Cell. (2002) 108:153–64. doi: 10.1016/s0092-8674(02)00625-6
31. Berghe TV, Kalai M, Denecker G, Meeus A, Saelens X, Vandenabeele P. Necrosis is associated with IL-6 production but apoptosis is not. Cell Signal. (2006) 18:328–35. doi: 10.1016/j.cellsig.2005.05.003
32. Hoffman WH, Biade S, Zilfou JT, Chen J, Murphy M. Transcriptional repression of the anti-apoptoticsurvivingene by wild type p53. J Biol Chem. (2002) 277:3247–57. doi: 10.1074/jbc.m106643200
33. Koff J, Ramachandiran S, Bernal-Mizrachi L. A time to kill: targeting apoptosis in cancer. Int J Mol Sci. (2015) 16:2942–55. doi: 10.3390/ijms16022942
34. van Engeland M, Ramaekers FCS, Schutte B, Reutelingsperger CPM. A novel assay to measure loss of plasma membrane asymmetry during apoptosis of adherent cells in culture. Cytometry. (1996) 24:131–9. doi: 10.1002/(sici)1097-0320(19960601)24:2<131::Aid-cyto5>3.0.Co;2-m
35. Julien O, Wells JA. Caspases and their substrates. Cell Death Differ. (2017) 24:1380–9. doi: 10.1038/cdd.2017.44
36. Galluzzi L, Vitale I, Aaronson SA, Abrams JM, Adam D, Agostinis P, et al. Molecular mechanisms of cell death: recommendations of the Nomenclature Committee on Cell Death 2018. Cell Death Differ. (2018) 25:486–541. doi: 10.1038/s41418-017-0012-4
37. Poon IKH, Lucas CD, Rossi AG, Ravichandran KS. Apoptotic cell clearance: basic biology and therapeutic potential. Nat Rev Immunol. (2014) 14:166–80. doi: 10.1038/nri3607
38. Scaffidi P, Misteli T, Bianchi ME. Release of chromatin protein HMGB1 by necrotic cells triggers inflammation. Nature. (2002) 418:191–5. doi: 10.1038/nature00858
39. Labi V, Erlacher M. How cell death shapes cancer. Cell Death Dis. (2015) 6:e1675. doi: 10.1038/cddis.2015.20
40. Golstein P, Kroemer G. Cell death by necrosis: towards a molecular definition. Trends Biochem Sci. (2007) 32:37–43. doi: 10.1016/j.tibs.2006.11.001
41. Festjens N, Vanden Berghe T, Vandenabeele P. Necrosis, a well-orchestrated form of cell demise: signalling cascades, important mediators and concomitant immune response. Biochim Biophys Acta. (2006) 1757:1371–87. doi: 10.1016/j.bbabio.2006.06.014
42. Ting AT, Pimentel-Muinios FX, Seed B. RIP mediates tumor necrosis factor receptor 1 activation of NF-kB but not Fas/APO-1-initiated apoptosis. EMBO J. (1996) 15:6189–96. doi: 10.1002/j.1460-2075.1996.tb01007.x
43. Devin A, Cook A, Lin Y, Rodriguez Y, Kelliher M, Liu ZG. The distinct roles of TRAF2 and RIP in IKK activation by TNF-R1: TRAF2 recruits IKK to TNF-R1 while RIP mediates IKK activation. Immunity. (2000) 12:419–29. doi: 10.1016/S1074-7613(00)80194-6
44. Devin A, Lin Y, Liu ZG. The role of the death-domain kinase RIP in tumour-necrosis-factor-induced activation of mitogen-activated protein kinases. EMBO Rep. (2003) 4:623–7. doi: 10.1038/sj.embor.embor854
45. Thon L, Möhlig H, Mathieu S, Lange A, Bulanova E, Winoto-Morbach S, et al. Ceramide mediates caspase-independent programmed cell death. FASEB J. (2005) 19:1945–56. doi: 10.1096/fj.05-3726com
46. England K, Cotter TG. Direct oxidative modifications of signalling proteins in mammalian cells and their effects on apoptosis. Redox Rep. (2005) 10:237–45. doi: 10.1179/135100005X70224
47. Cocco RE, Ucker DS. Distinct modes of macrophage recognition for apoptotic and necrotic cells are not specified exclusively by phosphatidylserine exposure. Mol Biol Cell. (2001) 12:919–30. doi: 10.1091/mbc.12.4.919
48. Vakkila J, Lotze MT. Inflammation and necrosis promote tumour growth. Nat Rev Immunol. (2004) 4:641–8. doi: 10.1038/nri1415
49. Jacobson LS, Lima H, Goldberg MF, Gocheva V, Tsiperson V, Sutterwala FS, et al. Cathepsin-mediated necrosis controls the adaptive immune response by Th2 (T helper type 2)-associated adjuvants. J Biol Chem. (2013) 288:7481–91. doi: 10.1074/jbc.m112.400655
50. Matsukawa T, Yamashita Y, Arakawa A, Nishiharu T, Urata J, Murakami R, et al. Percutaneous microwave coagulation therapy in liver tumors. Acta Radiol. (1997) 38:410–5. doi: 10.1080/02841859709172092
51. Zhang Q, Raoof M, Chen Y, Sumi Y, Sursal T, Junger W, et al. Circulating mitochondrial DAMPs cause inflammatory responses to injury. Nature. (2010) 464:104–7. doi: 10.1038/nature08780
52. Sahay A, Sahay N, Kapoor A, Kapoor J, Chatterjee A. Percutaneous image-guided radiofrequency ablation of tumors in inoperable patients - immediate complications and overall safety. Indian J Palliat Care. (2016) 22:67–73. doi: 10.4103/0973-1075.173951
53. Willingham SB, Allen IC, Bergstralh DT, Brickey WJ, Huang MTH, Taxman DJ, et al. NLRP3 (NALP3, cryopyrin) facilitates in vivo caspase-1 activation, necrosis, and HMGB1 release via inflammasome-dependent and -independent pathways. J Immunol. (2009) 183:2008–15. doi: 10.4049/jimmunol.0900138
54. Jorgensen I, Miao EA. Pyroptotic cell death defends against intracellular pathogens. Immunol Rev. (2015) 265:130–42. doi: 10.1111/imr.12287
55. Shi J, Zhao Y, Wang K, Shi X, Wang Y, Huang H, et al. Cleavage of GSDMD by inflammatory caspases determines pyroptotic cell death. Nature. (2015) 526:660–5. doi: 10.1038/nature15514
56. Ma Y, Jiang J, Gao Y, Shi T, Zhu X, Zhang K, et al. Research progress of the relationship between pyroptosis and disease. Am J Transl Res. (2018) 10:2213–9.
57. Vande Walle L, Lamkanfi M. Pyroptosis. Curr Biol. (2016) 26:R568–72. doi: 10.1016/j.cub.2016.02.019
58. McKenzie BA, Mamik MK, Saito LB, Boghozian R, Monaco MC, Major EO, et al. Caspase-1 inhibition prevents glial inflammasome activation and pyroptosis in models of multiple sclerosis. Proc Natl Acad Sci USA. (2018) 115:E6065–74. doi: 10.1073/pnas.1722041115
59. He WT, Wan H, Hu L, Chen P, Wang X, Huang Z, et al. Gasdermin D is an executor of pyroptosis and required for interleukin-1β secretion. Cell Res. (2015) 25:1285–98. doi: 10.1038/cr.2015.139
60. Bortolotti P, Faure E, Kipnis E. Inflammasomes in tissue damages and immune disorders after trauma. Front Immunol. (2018) 9:1900. doi: 10.3389/fimmu.2018.01900
61. Walsh MP, Duncan B, Larabee S, Krauss A, Davis JPE, Cui Y, et al. Val-boroPro accelerates T cell priming via modulation of dendritic cell trafficking resulting in complete regression of established murine tumors. PLoS ONE. (2013) 8:e58860. doi: 10.1371/journal.pone.0058860
62. Dhuriya YK, Sharma D. Necroptosis: a regulated inflammatory mode of cell death. J Neuroinflammation. (2018) 15:199. doi: 10.1186/s12974-018-1235-0
63. Yoon S, Kovalenko A, Bogdanov K, Wallach D. MLKL, the protein that mediates necroptosis, also regulates endosomal trafficking and extracellular vesicle generation. Immunity. (2017) 47:51–65.e57. doi: 10.1016/j.immuni.2017.06.001
64. Lawlor KE, Khan N, Mildenhall A, Gerlic M, Croker BA, D'Cruz AA, et al. RIPK3 promotes cell death and NLRP3 inflammasome activation in the absence of MLKL. Nat Commun. (2015) 6:6282. doi: 10.1038/ncomms7282
65. Belehradek M, Domenge C, Luboinski B, Orlowski S, Belehradek J, Mir LM. Electrochemotherapy, a new antitumor treatment. First clinical phase I-II trial. Cancer. (1993) 72:3694–700. doi: 10.1002/1097-0142(19931215)72:12<3694::aid-cncr2820721222>3.0.co;2-2
66. Plaschke CC, Bertino G, McCaul JA, Grau JJ, de Bree R, Sersa G, et al. European Research on Electrochemotherapy in Head and Neck Cancer (EURECA) project: results from the treatment of mucosal cancers. Eur J Cancer. (2017) 87:172–81. doi: 10.1016/j.ejca.2017.10.008
67. Esmaeili N, Friebe M. Electrochemotherapy: a review of current status, alternative IGP approaches, and future perspectives. J Healthcare Eng. (2019) 2019:2784516. doi: 10.1155/2019/2784516
68. Mir LM, Banoun H, Paoletti C. Introduction of definite amounts of nonpermeant molecules into living cells after electropermeabilization: direct access to the cytosol. Exp Cell Res. (1988) 175:15–25. doi: 10.1016/0014-4827(88)90251-0
69. Heller R, Jaroszeski MJ, Glass LF, Messina JL, Rapaport DP, DeConti RC, et al. Phase I/II trial for the treatment of cutaneous and subcutaneous tumors using electrochemotherapy. Cancer. (1996) 77:964–71. doi: 10.1002/(sici)1097-0142(19960301)77:5<964::Aid-cncr24>3.0.Co;2-0
70. Florea AM, Büsselberg D. Cisplatin as an anti-tumor drug: cellular mechanisms of activity, drug resistance and induced side effects. Cancers. (2011) 3:1351–71. doi: 10.3390/cancers3011351
71. Kalal BS, Upadhya D, Pai VR. Chemotherapy resistance mechanisms in advanced skin cancer. Oncol Rev. (2017) 11:326–326. doi: 10.4081/oncol.2017.326
72. Belehradek JJr, Orlowski S, Ramirez LH, Pron G, Poddevin B, Mir LM. Electropermeabilization of cells in tissues assessed by the qualitative and quantitative electroloading of bleomycin. Biochim Biophys Acta. (1994) 1190:155–63. doi: 10.1016/0005-2736(94)90045-0
73. Marty M, Sersa G, Garbay JR, Gehl J, Collins CG, Snoj M, et al. Electrochemotherapy – An easy, highly effective and safe treatment of cutaneous and subcutaneous metastases: Results of ESOPE (European Standard Operating Procedures of Electrochemotherapy) study. Eur J Cancer Suppl. (2006) 4:3–13. doi: 10.1016/j.ejcsup.2006.08.002
74. Longo F, Perri F, Pavone E, Aversa C, Maglione MG, Guida A, et al. Electrochemotherapy as palliative treatment in patients with advanced head and neck tumours: outcome analysis in 93 patients treated in a single institution. Oral Oncol. (2019) 92:77–84. doi: 10.1016/j.oraloncology.2019.03.016
75. Mir LM, Orlowski S. Mechanisms of electrochemotherapy. Adv Drug Deliv Rev. (1999) 35:107–18. doi: 10.1016/S0169-409X(98)00066-0
76. Tounekti O, Pron G, Belehradek J Jr, Mir LM. Bleomycin, an apoptosis-mimetic drug that induces two types of cell death depending on the number of molecules internalized. Cancer Res. (1993) 53:5462–69.
77. Calvet CY, Famin D, André FM, Mir LM. Electrochemotherapy with bleomycin induces hallmarks of immunogenic cell death in murine colon cancer cells. Oncoimmunology. (2014) 3:e28131. doi: 10.4161/onci.28131
78. Fernandes P, O'Donovan TR, McKenna SL, Forde PF. Electrochemotherapy causes caspase-independent necrotic-like death in pancreatic cancer cells. Cancers. (2019) 11:1177. doi: 10.3390/cancers11081177
79. Frandsen SK, Gissel H, Hojman P, Tramm T, Eriksen J, Gehl J. Direct therapeutic applications of calcium electroporation to effectively induce tumor Necrosis. (2012) 72:1336–41. doi: 10.1158/0008-5472.can-11-3782
80. Falk H, Matthiessen LW, Wooler G, Gehl J. Calcium electroporation for treatment of cutaneous metastases; a randomized double-blinded phase II study, comparing the effect of calcium electroporation with electrochemotherapy. Acta Ocol. (2017) 57:311–9. doi: 10.1080/0284186X.2017.1355109
81. Rimessi A, Giorgi C, Pinton P, Rizzuto R. The versatility of mitochondrial calcium signals: from stimulation of cell metabolism to induction of cell death. Biochim Biophys Acta. (2008) 1777:808–16. doi: 10.1016/j.bbabio.2008.05.449
82. Maji S, Panda S, Samal SK, Shriwas O, Rath R, Pellecchia M, et al. Bcl-2 antiapoptotic family proteins and chemoresistance in cancer. Adv Cancer Res. (2018) 137:37–75. doi: 10.1016/bs.acr.2017.11.001
83. Gibot L, Montigny A, Baaziz H, Fourquaux I, Audebert M, Rols M-P. Calcium delivery by electroporation induces in vitro cell death through mitochondrial dysfunction without DNA damages. Cancers. (2020) 12:425. doi: 10.3390/cancers12020425
84. Hansen EL, Sozer EB, Romeo S, Frandsen SK, Vernier PT, Gehl J. Dose-dependent ATP depletion and cancer cell death following calcium electroporation, relative effect of calcium concentration and electric field strength. PLoS ONE. (2015). 10:e0122973. doi: 10.1371/journal.pone.0122973
85. Frandsen SK, Krüger MB, Mangalanathan UM, Tramm T, Mahmood F, Novak I, et al. Normal and malignant cells exhibit differential responses to calcium electroporation. Cancer Res. (2017) 77:4389–401. doi: 10.1158/0008-5472.can-16-1611
86. Danese A, Patergnani S, Bonora M, Wieckowski MR, Previati M, Giorgi C, et al. Calcium regulates cell death in cancer: roles of the mitochondria and mitochondria-associated membranes (MAMs). Biochim Biophys Acta. (2017) 1858:615–27. doi: 10.1016/j.bbabio.2017.01.003
87. Ji S, Lee J-Y, Schrör J, Mazumder A, Jang DM, Chateauvieux S, et al. The dialkyl resorcinol stemphol disrupts calcium homeostasis to trigger programmed immunogenic necrosis in cancer. Cancer Lett. (2018) 416:109–23. doi: 10.1016/j.canlet.2017.12.011
88. Miller L, Leor J, Rubinsky B. Cancer cells ablation with irreversible electroporation. Technol Cancer Res Treat. (2005) 4:699–705. doi: 10.1177/153303460500400615
89. Belfiore MP, Ronza FM, Romano F, Ianniello GP, De Lucia G, Gallo C, et al. Percutaneous CT-guided irreversible electroporation followed by chemotherapy as a novel neoadjuvant protocol in locally advanced pancreatic cancer: our preliminary experience. Int J Surg. (2015) 21:S34–9. doi: 10.1016/j.ijsu.2015.06.049
90. Martin RCG, Kwon D, Chalikonda S, Sellers M, Kotz E, Scoggins C, et al. Treatment of 200 locally advanced (stage III) pancreatic adenocarcinoma patients with irreversible electroporation. Ann Surg. (2015) 262:486–94. doi: 10.1097/SLA.0000000000001441
91. Sugimoto K, Moriyasu F, Kobayashi Y, Saito K, Takeuchi H, Ogawa S, et al. Irreversible electroporation for nonthermal tumor ablation in patients with hepatocellular carcinoma: initial clinical experience in Japan. Jap J Radiol. (2015) 33:424–32. doi: 10.1007/s11604-015-0442-1
92. Scheffer HJ, Vroomen LGPH, De Jong MC, Melenhorst MCAM, Zonderhuis BM, Daams F, et al. Ablation of locally advanced pancreatic cancer with percutaneous irreversible electroporation: results of the phase I/II PANFIRE study. Radiol. (2016) 282:585–97. doi: 10.1148/radiol.2016152835
93. Sutter O, Calvo J, N'Kontchou G, Nault JC, Ourabia R, Nahon P, et al. Safety and efficacy of irreversible electroporation for the treatment of hepatocellular carcinoma not amenable to thermal ablation techniques: a retrospective single-center case series. Radiology. (2017) 284:877–86. doi: 10.1148/radiol.2017161413
94. Leen E, Picard J, Stebbing J, Abel M, Dhillon T, Wasan H. Percutaneous irreversible electroporation with systemic treatment for locally advanced pancreatic adenocarcinoma. J Gastrointest Oncol. (2018) 9:275–81. doi: 10.21037/jgo.2018.01.14
95. Kalra N, Gupta P, Gorsi U, Bhujade H, Chaluvashetty SB, Duseja A, et al. Irreversible electroporation for unresectable hepatocellular carcinoma: initial experience. Cardiovasc Intervent Radiol. (2019) 42:584–90. doi: 10.1007/s00270-019-02164-2
96. Lee EW, Loh CT, Kee ST. Imaging guided percutaneous irreversible electroporation: ultrasound and immunohistological correlation. Technol Cancer Res Treat. (2007) 6:287–93. doi: 10.1177/153303460700600404
97. Beitel-White N, Bhonsle S, Martin RCG, Davalos RV. Electrical characterization of human biological tissue for irreversible electroporation treatments. Conf Proc IEEE Eng Med Biol Soc. (2018) 2018:4170–3. doi: 10.1109/EMBC.2018.8513341
98. Brock RM, Beitel-White N, Coutermarsh-Ott S, Grider DJ, Lorenzo MF, Ringel-Scaia VM, et al. Patient derived xenografts expand human primary pancreatic tumor tissue availability for ex vivo irreversible electroporation testing. Front Oncol. (2020) 10:843. doi: 10.3389/fonc.2020.00843
99. Neal RE, Garcia PA, Robertson JL, Davalos RV. Experimental characterization of intrapulse tissue conductivity changes for electroporation. Conf Proc IEEE Eng Med Biol Soc. (2011) 2011:5581–4. doi: 10.1109/IEMBS.2011.6091350
100. Bhonsle S, Lorenzo MF, Safaai-Jazi A, Davalos RV. Characterization of nonlinearity and dispersion in tissue impedance during high-frequency electroporation. IEEE Trans Biomed Eng. (2018) 65:2190–201. doi: 10.1109/TBME.2017.2787038
101. O'Brien TJ, Passeri M, Lorenzo MF, Sulzer JK, Lyman WB, Swet JH, et al. Experimental high-frequency irreversible electroporation using a single-needle delivery approach for nonthermal pancreatic ablation in vivo. J Vasc Intervent Radiol. (2019) 30:854–62. doi: 10.1016/j.jvir.2019.01.032
102. Tekle E, Wolfe MD, Oubrahim H, Chock PB. Phagocytic clearance of electric field induced 'apoptosis-mimetic' cells. Biochem Biophys Res Commun. (2008) 376:256–60. doi: 10.1016/j.bbrc.2008.08.060
103. Vogel JA van Veldhuisen E Alles LK Busch OR Dijk F van Gulik TM . Time-dependent impact of irreversible electroporation on pathology and ablation size in the porcine liver: A 24-hour experimental study. Technol Cancer Res Treat. (2019) 18:1–9. doi: 10.1177/1533033819876899
104. Mercadal B, Beitel-White N, Aycock K, Castellví Q, Davalos R, Ivorra A. Dynamics of cell death after conventional IRE and H-FIRE treatments. Ann Biomed Eng. (2020) 48:1451–62. doi: 10.1007/s10439-020-02462-8
105. Ben-David E, Ahmed M, Faroja M, Moussa M, Wandel A, Sosna J, et al. Irreversible electroporation: treatment effect is susceptible to local environment and tissue properties. Radiology. (2013) 269:738–47. doi: 10.1148/radiol.13122590
106. Bhutiani N, Agle S, Li Y, Li S, Martin RCG II. Irreversible electroporation enhances delivery of gemcitabine to pancreatic adenocarcinoma. J Surg Oncol. (2016) 114:181–6. doi: 10.1002/jso.24288
107. Belfiore G, Belfiore MP, Reginelli A, Capasso R, Romano F, Ianniello GP, et al. Concurrent chemotherapy alone versus irreversible electroporation followed by chemotherapy on survival in patients with locally advanced pancreatic cancer. Med Oncol. (2017) 34:38. doi: 10.1007/s12032-017-0887-4
108. Kodama H, Shamay Y, Kimura Y, Shah J, Solomon SB, Heller D, et al. Electroporation-induced changes in tumor vasculature and microenvironment can promote the delivery and increase the efficacy of sorafenib nanoparticles. Bioelectrochemistry. (2019) 130:107328. doi: 10.1016/j.bioelechem.2019.107328
109. Arena CB, Sano MB, Rossmeisl JH, Caldwell JL, Garcia PA, Rylander MN, et al. High-frequency irreversible electroporation (H-FIRE) for non-thermal ablation without muscle contraction. BioMed Eng OnLine. (2011) 10:102. doi: 10.1186/1475-925X-10-102
110. Sano MB, Fan RE, Cheng K, Saenz Y, Sonn GA, Hwang GL, et al. Reduction of muscle contractions during irreversible electroporation therapy using high-frequency bursts of alternating polarity pulses: a laboratory investigation in an ex vivo swine model. J Vasc Intervent Radiol. (2018) 29:893–8.e4. doi: 10.1016/j.jvir.2017.12.019
111. Lorenzo MF, Thomas SC, Kani Y, Hinckley J, Lee M, Adler J, et al. Temporal characterization of blood-brain barrier disruption with high-frequency electroporation. Cancers. (2019) 11:1850. doi: 10.3390/cancers11121850
112. Partridge BR, O'Brien TJ, Lorenzo MF, Coutermarsh-Ott SL, Barry SL, Stadler K, et al. High-frequency irreversible electroporation for treatment of primary liver cancer: a proof-of-principle study in canine hepatocellular carcinoma. J Vasc Intervent Radiol. (2020) 31:482–91.e4. doi: 10.1016/j.jvir.2019.10.015
113. Wasson EM, Alinezhadbalalami N, Brock RM, Allen IC, Verbridge SS, Davalos RV. Understanding the role of calcium-mediated cell death in high-frequency irreversible electroporation. Bioelectrochemistry. (2020) 131:107369. doi: 10.1016/j.bioelechem.2019.107369
114. Schoenbach KH, Beebe SJ, Buescher ES. Intracellular effect of ultrashort electrical pulses. Bio Electro Magnet. (2001) 22:440–8. doi: 10.1002/bem.71
115. Beebe SJ, Fox PM, Rec LJ, Somers K, Stark RH, Schoenbach KH. Nanosecond pulsed electric field (nsPEF) effects on cells and tissues: apoptosis induction and tumor growth inhibition. IEEE Transac Plasma Scie. (2002) 30:286–92. doi: 10.1109/TPS.2002.1003872
116. Ford WE, Ren W, Blackmore PF, Schoenbach KH, Beebe SJ. Nanosecond pulsed electric fields stimulate apoptosis without release of pro-apoptotic factors from mitochondria in B16f10 melanoma. Arch Biochem Biophys. (2010) 497:82–9. doi: 10.1016/j.abb.2010.03.008
117. Beebe S, Sain N, Ren W. Induction of cell death mechanisms and apoptosis by Nanosecond Pulsed Electric Fields (nsPEFs). Cells. (2013) 2:136–62. doi: 10.3390/cells2010136
118. Guo S, Burcus NI, Hornef J, Jing Y, Jiang C, Heller R, et al. Nano-pulse stimulation for the treatment of pancreatic cancer and the changes in immune profile. Cancers. (2018) 10:217. doi: 10.3390/cancers10070217
119. Guo S, Jing Y, Burcus NI, Lassiter BP, Tanaz R, Heller R, et al. Nano-pulse stimulation induces potent immune responses, eradicating local breast cancer while reducing distant metastases. Int J Cancer. (2018) 142:629–40. doi: 10.1002/ijc.31071
Keywords: cancer, apoptosis, necrosis, pyroptosis, calcium, electroporation, ablation
Citation: Brock RM, Beitel-White N, Davalos RV and Allen IC (2020) Starting a Fire Without Flame: The Induction of Cell Death and Inflammation in Electroporation-Based Tumor Ablation Strategies. Front. Oncol. 10:1235. doi: 10.3389/fonc.2020.01235
Received: 11 April 2020; Accepted: 16 June 2020;
Published: 28 July 2020.
Edited by:
Gabi U. Dachs, University of Otago, New ZealandReviewed by:
Maja Cemazar, Institute of Oncology Ljubljana, SloveniaSandeep Mittal, University of Texas MD Anderson Cancer Center, United States
Copyright © 2020 Brock, Beitel-White, Davalos and Allen. This is an open-access article distributed under the terms of the Creative Commons Attribution License (CC BY). The use, distribution or reproduction in other forums is permitted, provided the original author(s) and the copyright owner(s) are credited and that the original publication in this journal is cited, in accordance with accepted academic practice. No use, distribution or reproduction is permitted which does not comply with these terms.
*Correspondence: Irving C. Allen, aWNhbGxlbkB2dC5lZHU=