Corrigendum: Regulatory Role of Hexokinase 2 in Modulating Head and Neck Tumorigenesis
- 1Institute of Oral Biology, School of Dentistry, National Yang-Ming University, Taipei, Taiwan
- 2Department of Dentistry, School of Dentistry, National Yang-Ming University, Taipei, Taiwan
- 3Cancer Progression Research Center, National Yang-Ming University, Taipei, Taiwan
- 4Department of Oral and Maxillofacial Surgery, MacKay Memorial Hospital, Taipei, Taiwan
- 5Department of Medical Research, MacKay Memorial Hospital, Taipei, Taiwan
- 6School of Dentistry and Department of Dentistry, National Taiwan University Medical College and National Taiwan University Hospital, Taipei, Taiwan
- 7Department of Health Technology and Informatics (HTI), The Hong Kong Polytechnic University (PolyU), Kowloon, Hong Kong
- 8Department of Stomatology, Taipei Veterans General Hospital, Taipei, Taiwan
To support great demand of cell growth, cancer cells preferentially obtain energy and biomacromolecules by glycolysis over mitochondrial oxidative phosphorylation (OxPhos). Among all glycolytic enzymes, hexokinase (HK), a rate-limiting enzyme at the first step of glycolysis to catalyze cellular glucose into glucose-6-phosphate, is herein emphasized. Four HK isoforms, HK1-HK4, were discovered in nature. It was shown that HK2 expression is enriched in many tumor cells and correlated with poorer survival rates in most neoplastic cells. HK2-mediated regulations for cell malignancy and mechanistic cues in regulating head and neck tumorigenesis, however, are not fully elucidated. Cellular malignancy index, such as cell growth, cellular motility, and treatment sensitivity, and molecular alterations were determined in HK2-deficient head and neck squamous cell carcinoma (HNSCC) cells. By using various cancer databases, HK2, but not HK1, positively correlates with HNSCC progression in a stage-dependent manner. A high HK2 expression was detected in head and neck cancerous tissues compared with their normal counterparts, both in mouse and human subjects. Loss of HK2 in HNSCC cells resulted in reduced cell (in vitro) and tumor (in vivo) growth, as well as decreased epithelial-mesenchymal transition–mediated cell movement; in contrast, HK2-deficient HNSCC cells exhibited greater sensitivity to chemotherapeutic drugs cisplatin and 5-fluorouracil but are more resistant to photodynamic therapy, indicating that HK2 expression could selectively define treatment sensitivity in HNSCC cells. At the molecular level, it was found that HK2 alteration drove metabolic reprogramming toward OxPhos and modulated oncogenic Akt and mutant TP53-mediated signals in HNSCC cells. In summary, the present study showed that HK2 suppression could lessen HNSCC oncogenicity and modulate therapeutic sensitivity, thereby being an ideal therapeutic target for HNSCCs.
Introduction
Most cancers have acquired the same set of functional capabilities during tumorigenesis including sustained proliferative signal, evaded growth suppressors, resisted cell death, sustained angiogenesis, and deregulated cellular energetics (1). In the aspect of energy production, glucose is a major source of bioenergetics and biomolecules used to maintain cellular homeostasis. Glucose molecules could be metabolized by glycolysis to generate pyruvate in cells; the pyruvate could be either oxidatively metabolized to generate up to 38 ATPs through the process of oxidative phosphorylation (OxPhos) or be reductively converted into organic acids or alcohols by fermentation producing only 2 ATP (2). In general, normal cells in non-malignant tissues developed an evolutionary choice of “Pasteur effect” to allow a system to fine tune cell metabolism in unfavorable environment (3, 4). On the contrary, cancer cells exhibited a unique metabolic system by taking advantage of aerobic glycolysis for demands of energy and biomolecules regardless of oxygen status; in this way, cancer cells preferentially drive their metabolism away from OxPhos toward glycolysis, so-called Warburg effect (5).
Previous studies have sought to target different cancer-specific metabolic pathways, in particular glycolysis, for development of antitumor treatments (6–9). There are three irreversible reactions in glycolysis. It is well-known that the glycolytic rate is limited by the phosphorylation state of glycolytic enzymes hexokinases (HKs), because at the first step of glycolysis, HKs catalyze the intake glucose into glucose-6-phosphate (G-6-P) (10). Hexokinase–mediated catalysis is ATP dependent and a key determinant for the direction and magnitude of glucose flux within cells; thus, it would more efficient to target glucose metabolism in cancer cells by cutting down this earliest step of glucose flux (11). Four HK isoforms including HK1, HK2, HK3, and HK4 encoded by different genes were found in nature. Among them, HK1 is ubiquitously expressed in most mammalian tissues, and HK2 is detected in adipose, skeletal, and cardiac muscles, whereas HK3 and HK4 show relatively low expression in mammalian tissues (12). In the aspect of protein structure, two catalytic domains, both in N- and C-terminal portions, are detected in HK2, resulting in greater glycolytic rate compared with HK1-mediated glycolysis, whereas only one catalytic domain was found at the carboxyl terminal part in HK1 protein (13). In clinic, HK2 is rarely expressed in normal tissues as a high level of HK2 expression was detected in many solid tumors (14) and is associated with elevated progression, poorer overall survival, and treatment resistance in breast cancer (both primary and metastatic kinds) (15–18), bladder cancer (19), cervical squamous cell carcinoma (20), colorectal cancer (21), epithelial ovarian tumors (22), glioblastoma multiforme (23), hepatocellular carcinoma (24), laryngeal squamous cell carcinoma (25), lung cancer (26), neuroblastoma (27), neuroendocrine tumor (28), pancreatic cancer (29), and prostate cancer (30), making it an excellent target for development of anticancer therapy. Moreover, given its selective expression in tumors, HK2 has drawn increasing attention on its clinical implications forming the basis of fluorodeoxyglucose F-18 positron emission tomography imaging, a widely used clinical procedure to detect tumors. Indeed, a recent meta-analysis provides evidence that HK2 could be a marker to predict the risk of all-cause mortality and cancer progression in patients with tumors of the digestive system (31).
Numbers of molecular cues including signal transducer and activator of transcription 3 (32–35), protein kinase B (PKB), also known as Akt (19, 36–38), mammalian target of rapamycin (38–40), and PH domain leucine-rich repeat protein phosphatase (41), as well as autophagy (42, 43), have been found to control HK2-mediated metabolic alterations, thereby regulating tumor identity. Moreover, HK2 contains an N-terminal hydrophobic domain allowing it to bind to outer mitochondrial membrane voltage-dependent anion channel (VDAC) protein, thereby resulting in Bax-mediated apoptosis. HK2–VDAC interaction could also provide kinetic benefits in HK2 exhibiting greater affinity to ATP over G-6-P, suggesting that HK2 could regulate oncogenicity via modulation of mitochondrial physiology (44–47). More recent studies further discovered that p53-inducible protein TIGAR (Tp53-induced Glycolysis and Apoptosis Regulator), Akt, and ER stress sensor kinase could regulate mitochondrial HK2 localization (48–51). Interestingly, mitochondrial TIGAR–HK2 complex upregulated HK2 and hypoxia-inducible factor 1 activity limiting reactive oxygen species production and protecting from tumor cell death under hypoxic condition implying that p53 could be an essential key regulator for HK2-mediated oncogenesis (26, 30, 48). In addition to signaling factors, HK2 regulated by epigenetic mediators including microRNAs (32, 52–55), long non-coding RNAs (56–58), and histone/DNA methylation (59) are also important to modulate HK2-mediated tumorigenicity. Taken together, HK2 seems to be a master promoting factor in controlling carcinogenesis in different cancers; to date, however, the role of HK2 in controlling head and neck squamous cell carcinoma (HNSCC) development was rarely focused. The present study was therefore conceived to determine cellular, molecular, and physiological alterations of HNSCC cells in response to HK2 silencing.
Materials and Methods
Basic Reagents, Cells, and Animal and Human Protocols
All experimental reagents including 4-nitroquinoline 1-oxide (4-NQO), 3-(4,5-Dimethylthiazol-2-yl)-2,5-diphenyltetrazolium bromide (MTT), cisplatin (CDDP), and 5-fluorouracil (5-FU) were purchased from Sigma. The HNSCC cell lines, clinical human HNSCC tissues, and animal procedure were obtained and described elsewhere (60, 61).
Establishment of HK2 Silencing HNSCC Cells
Three plasmids encoding small hairpin RNAs (shRNAs) targeting HK2 gene were purchased from the National RNAi Core Facility (NRCF), Academia Sinica, Taipei, Taiwan. The targeting sequences were as listed: 5-GCCTGGCTAACTTCATGGATA-3 (A), 5-CCGTAACATTCTCATCGATTT-3 (B), and 5-GCTTGAAGATTAGGTACTATC-3 (C) (NRCF). The control nucleotide sequence of shRNA was 5-GCGGTTGCCAAGAGGTTCCAT-3, which was the random sequence that targets luciferase (shLuc) mRNA. Lentiviral vectors containing shHK2/shLuc were generated in 293T cells.
Cellular and Molecular Assays
Cell growth (MTT and trypan blue exclusion assays), cell cycle, annexin V–fluorescein isothiocyanate–based cell apoptosis, Transwell-based cell migration/invasion, drug resistance (IC50 determination), quantitative real-time polymerase chain reaction (qRT-PCR), Western blot, aldehyde dehydrogenase (ALDH) activity, immunostaining analysis, human phospho-antibody array, and XF Metabolic Assay analyses were performed as previously described at Instrumentation Resource Center, National Yang Ming University (61, 62). Primers for qRT-PCR analysis (Supplementary Table 1) and antibodies used for protein assays (Supplementary Table 2) are listed. ImageJ (National Institute of Health, Bethesda, Maryland, United States) was used for quantification.
Aminolevulinic Acid–Photodynamic Therapy Treatment
5-Aminolevulinic acid (ALA) 1 mM was made by diluting 1 M ALA stock with serum-free medium and neutralized to pH 7.2 with NaOH immediately before use. The total number of 5 × 105 cells were incubated with 1 mM ALA for 3 h, and the medium was replaced with 100 μL phosphate-buffered saline to prevent thermal injury by the light. The cells were then exposed to various doses of light source (30.33 mW/cm2) with a 635-nm wavelength emission of red light. After exposure, cells are replaced with culture medium and cultured for 24 h. Relative viable cells were determined by the MTT method.
Measurement of Lactate, ATP, and PDH Activity
Extracellular lactate, intracellular ATP level, and pyruvate dehydrogenase (PDH) activity were determined previously (62). Cell number or genomic DNA content was used to normalize detections.
Xenografic Model
The HNSCC cells 1 × 106 to 4 × 106 and 1 μL of each medium were mixed and subcutaneously injected into the back of nude mice. Tumor volume and size were recorded.
Statistical Analysis
All analyses were statistically determined using Microsoft Excel 2013 (Microsoft, Redmond, WA, United States)/Prism 5 (GraphPad, San Diego, CA, United States). All quantifications are presented as mean ± SEM. A significant difference was defined as p < 0.05.
Results
HK2 Expression Defines HNSCC Progression in Clinic
By taking advantage of The Cancer Genomic Atlas–based UALCAN, Firebrowse, and The Human Protein Atlas (www.proteinatlas.org) databases (63–65), it was found that HK2 transcript, in comparison with HK1, is enriched in most primary tumor tissues compared with their normal counterparts (Figures 1A,B). Further analysis for HK1 and HK2 expression in HNSCCs indicated that (i) HK2 expression, but not HK1, is positively correlated with tumor stages and grades (Figures 1C,D); (ii) HK2 level, but not HK1, is upregulated in HPV− HNSCC tissues (Figure 1E); and (iii) HK2 expression, but not HK1, is increased in early metastatic HNSCCs [with one to three axillary lymph nodes (N1)] (Figure 1F). Furthermore, immunohistochemical analysis showed that HK2 is strongly detected in 4-NQO–induced mouse tongue neoplastic lesions compared with normal oral epithelium (Figure 1G), as well as human oral squamous cell carcinoma (Figure 1H). Surprisingly, differential HK1 level could better stratify cancer-specific survival rates than HK2 in HNSCC patients (Supplementary Figure 1), suggesting that HK1 and HK2 might play differential roles during HNSCC tumorigenesis. Taken together, these analyses suggest that HK2 serves as a rather early prognostic indicator in the majority of HNSCC population.
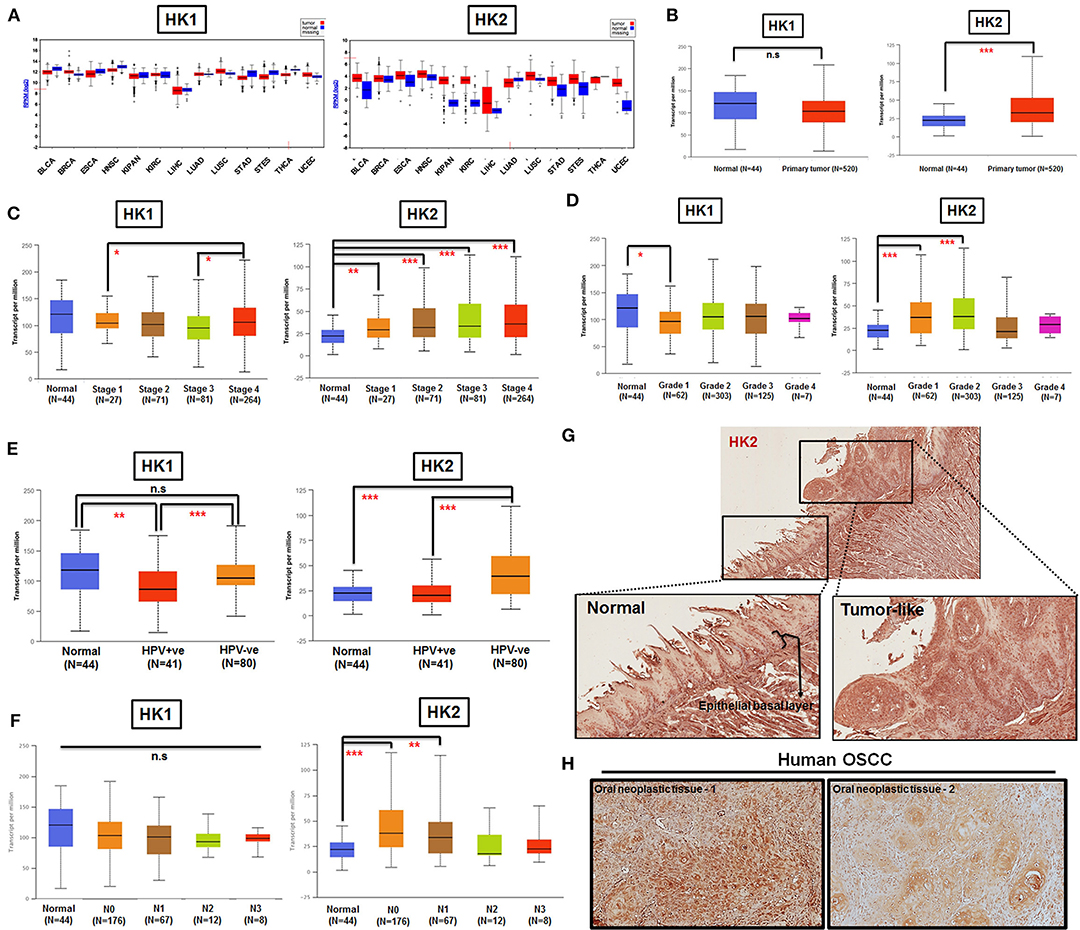
Figure 1. HK2 level positively correlates with HNSCC progression in clinic. (A) HK1, but not HK2, mRNA expression is enriched in most human tumors by using Firebrowser database. BCLA, bladder urothelial carcinoma; BRCA, breast invasive carcinoma; ESCA, esophageal carcinoma; HNSCC, head and neck squamous cell carcinoma; KIPAN, Pan-kidney cohort (KICH+KIRC+KIRP); KIRC, kidney renal clear cell carcinoma; LIHC, liver hepatocellular carcinoma; LUAD, lung adenocarcinoma; LUSC, lung squamous cell carcinoma; STAD, stomach adenocarcinoma; STES, stomach and esophageal carcinoma; THCA, Thyroid carcinoma; UCEC, uterine corpus endometrial carcinoma. (B) Statistical analysis for HK1 and HK2 mRNA levels in normal and primary HNSCC tissues as well as tumor tissues, classified by clinical (C) stages, (D) grade, (E) HPV infection status, and (F) nodal metastasis status from UALCAN database. Immunohistochemical analysis for HK2 in (G) in 12-week 4-NQO–treated mouse tongue and (H) human oral squamous cell carcinoma (hOSCC) tissues. Data are shown as mean ± SEM. ***p < 0.001, **p < 0.01, *p < 0.05, and n.s., non-significant.
HK2 Loss Modulates HNSCC Cell Growth and Motility
In consistent with former findings in other cancer tissues, HK2, both in mRNA and protein level, is highly expressed in tested HNSCC cell lines (Supplementary Table 3) compared to normal human oral keratinocytes (NHOKs) (Figure 2A and Supplementary Figure 2). A wide spectrum of cellular alterations and their potential underlying regulatory cues in response to HK2 loss were next focused on. As shRNA-mediated HK2-deficient HNSCC cells were successfully established without apparent morphological change (Figures 2B,C and Supplementary Figure 3), it was demonstrated that, by using both trypan blue exclusion (Figure 2D) and MTT (Figure 2E) assays, HK2-silencing resulted in decreased cell growth in HNSCC cells. Further analysis found no significant cell cycling change and cell apoptosis (data now shown) in HK2-silencing HNSCC cells compared with control cells, suggesting that HK2 could possibly control cell growth via self-protective machinery, such as autophagy (42, 43). In vivo growth for HK2-silencing tumors was also analyzed, and it was shown that HK2 loss resulted in significantly smaller xenografic tumors compared with control tumors (Figure 2F).
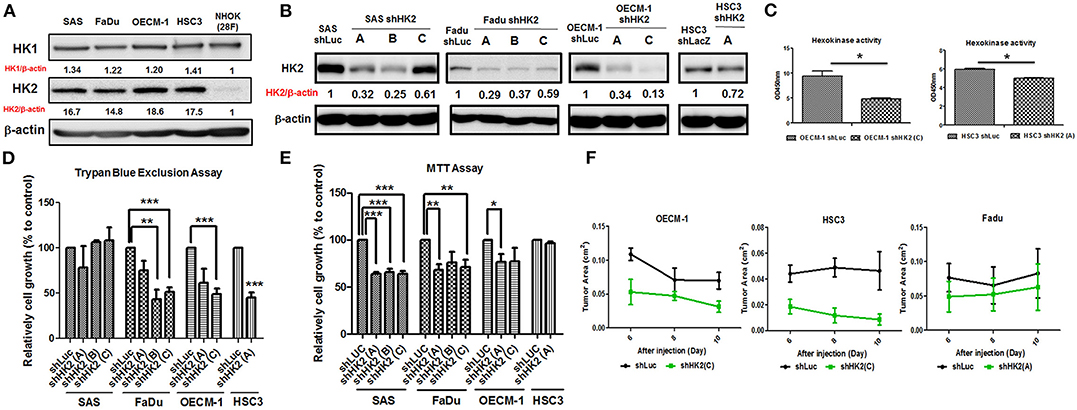
Figure 2. Differential HNSCC cell growth and HNSCC-bearing xenografic tumor growth in response to HK2 loss. Respective Western blot analysis for HK1 and HK2 protein expression in (A) HNSCC and NHOK cells and (B) shRNA-mediated HK2-silencing HNSCCC cells. (C) Colorimetric hexokinase activity assay showed functional deficient in HK2-silencing OECM-1 and HSC3 cells. Cell growth was examined in HK2-silencing HNSCC cells using (D) trypan exclusion and (E) MTT assays. (F) HK2 loss downregulated HNSCC-bearing xenografic tumor growth. shLuc vector is used as a control plasmid. Data are shown as mean ± SEM (N ≥ 3). ***p < 0.001, **p < 0.01, and *p < 0.05.
Potential impact of HK2 loss in regulating cell motility was also analyzed by using Transwell-based assays (62). The results indicated that HK2 deficiency led to significant decrease of cell migration and invasion in OECM-1 and HSC3 clones (Figures 3A,B). At cellular and molecular levels, lactate level, cytoskeletal organization and epithelial–mesenchymal transition (EMT) could modulate cell motility (66–68). While a slight, but not significant, increase in extracellular lactate production was detected in HK2-silencing HNSCC cells (Supplementary Figure 4), immunofluorescence staining analysis implied that lamellipodia-like F-actin (dot-lined in Figure 3C) was predominantly found in HK2-deficient HNSCC cells in comparison with control cells those contain more evident filopodia-like protrusions. Interestingly, as shRNA-mediated HK2 manipulation seems to heterogeneously silence HK2 protein, HNSCC cells with greater HK2 loss (white arrows in Figure 3C) showed more and compacted F-actin staining signals compared with cells with less HK2 expression (yellow arrows in Figure 3C), revealing that HK2 loss potentially reduces F-actin level in a single-cell basis. Moreover, increased epithelial marker E-cadherin accompanied with decreased mesenchymal markers N-cadherin, Snail, and Twist was detected in mRNA level (Figure 3D), further supporting the important role of EMT in HK2-mediated cell motility alteration. The role of HK2 in regulating tumor metastasis in vivo was further defined in a previously established primary and metastatic xenografic tumor model (60). By using microarray analysis, it was found that HK2, but not HK1 and HK3, is enriched in highly migrating cells in this model (Figure 3E). In brief, multiple molecular factors play key roles in regulating cell movement upon HK2 loss.
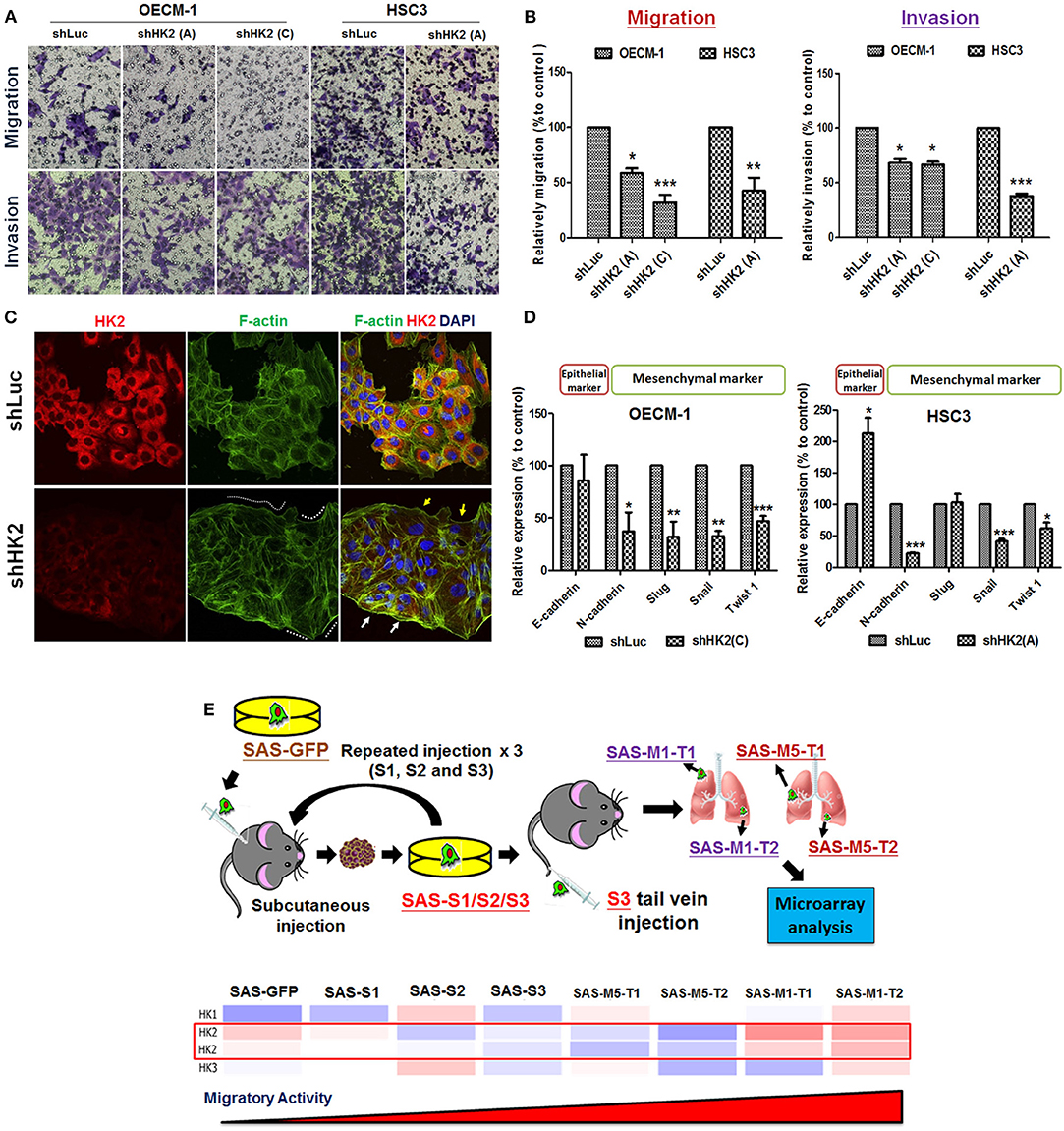
Figure 3. Cell motility changes in HK2-silencing HNSCC Cells. (A,B) Transwell cell migration and invasion assays were performed in HK2-silencing HNSCC cells. HK2 knockdown led to significantly less migration and invasion in HNSCC cells. The changes of cell motility could result from (C) rearrangement of lamellipodia-like (dot-lined) and filopodia-like F-actin structures. HK2-silencing OECM-1 cells showed heterogeneous population, whereas cells with higher HK2 expression exhibited more condense F-actin structure (white arrows), while cells with less HK2 expression displayed little F-actin staining; and (D) altered EMT-associated mRNA expression. (E) Microarray analysis showed an increasing HK2, but not HK1 and HK3, mRNA expression in in situ injected (S1/2/3) and distant metastatic (M1/M5) HNSCC derived tumors. Data are presented as mean ± SEM (N ≥ 3). ***p < 0.001, **p < 0.01, and *p < 0.05.
HK2 Expression Determines Treatment Resistance in HNSCCs Through Regulations of Stemness and Differentiation
The sensitivity for chemotherapeutic drugs, CDDP and 5-FU, as well as for photodynamic therapy (PDT), in HK2-silencing HNSCC cells was next examined. The results showed a common pattern of decreased half maximal inhibitory concentration (IC50) level for CDDP and 5-FU in HK2-deficient HNSCC cells compared with control cells (Figure 4A). Photodynamic therapy is an approved adjuvant treatment to lessen the disease mass of various premalignant and malignant lesions, including HNSCCs (69, 70). Photodynamic therapy–mediated cell apoptosis could be induced under numbers of physiological and pathological conditions, and one of the main advantages of PDT treatment is the higher selectivity to target transformed cells over normal cells (71). As HK2 loss could potentially reduce cell malignancy in HNSCC cells, it is expected that HK2-silencing HNSCC cells are less sensitive to PDT treatment (Figure 4B). At molecular level, previous study has shown that HK2 is essential for tumor initiation and survival in Kras-driven mouse lung cancer and ErbB2-driven mouse breast cancer, indicating that HK2 could be important to regulate cancer stemness (24). Moreover, it was also shown that elevated stemness could be a key factor underlying chemoresistance in HNSCCs (72). Consistent with this notion, stemness indicators Nanog and Oct4 mRNA (Figure 4C) and ALDH activity (Figure 4D) were downregulated in HK2-silencing HNSCC cells. Further analysis showed that HK2 protein is enriched in stem-like/high-grade sphere cells (Figure 4E), and strikingly, HK2 loss resulted in significantly less sphere number in selective medium used to culture head and neck cancer–initiating cells (Figure 4F). On the other hand, epithelial differentiation marker involucrin in OECM-1 cells with greater HK2 loss (clone C) was highly detected (Figure 4G), further supporting that HK2 change could regulate stemness and differentiation status, which modulate treatment sensitivity in HNSCC cells.
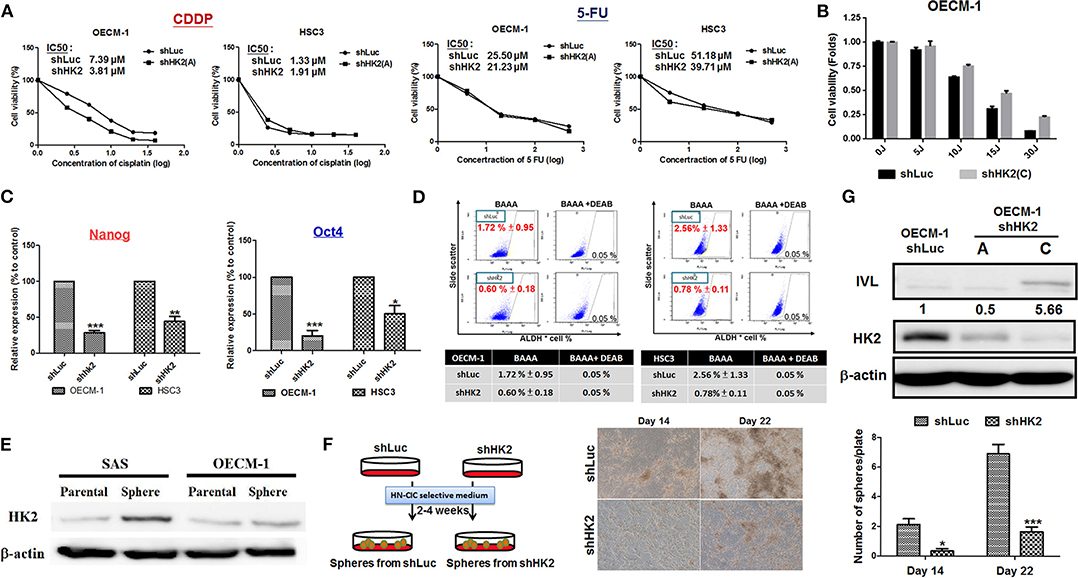
Figure 4. HK2 loss modulated therapeutic sensitivity in HNSCC Cells. (A) Decreased IC50 for clinical chemotherapeutic agent CDDP and 5-FU, whereas (B) greater resistance in response to photodynamic therapy was detected in HK2-deficient HNSCC cells. This effect is probably regulated via decreased (C) Nanog/Oct4 mRNA expression and/or (D) ALDH activity. While (E) HK2 is highly expressed in SAS and OECM-1 derived sphere cells compared with parental cells, (F) HK2 loss resulted in decease of sphere formation. (G) Upregulation for cellular differentiation marker involucrin (IVL) protein was detected by Western blot analysis in HK2-silencing HNSCC cells. Data are presented as mean ± SEM (N ≥ 3). ***p < 0.001, **p < 0.01, and *p < 0.05.
Molecular Alterations and Metabolic Shift in HK2-Silencing HNSCC Cells
HK2-mediated metabolic and molecular regulators for HNSCC malignancy were next examined. Metabolic reprogramming was recently proposed, suggesting that cancer cells could adapt to unfavorable metabolic stresses (73). Numbers of investigations have been reported showing metabolic shift upon HK2 changes in non-HNSCC cells (32, 40, 50, 54); in agreement with these findings, Seahorse analysis showed that HK2 loss led to increased basal and maximal mitochondrial respiration (Figures 5A,B), implying HK2-silencing HNSCC cells were metabolically programmed, being more dependent on mitochondrial metabolism. This statement could be further supported by the results showing a greater extracellular acidification rate (ECAR) upon addition of ATPase inhibitor oligomycin (Figure 5C) and increased PDH activity (Figure 5D) in HK2-silencing HNSCC cells. Unexpectedly, HK2 deficiency–mediated decreased cell growth did not result from reduction of cellular ATP level (Figure 5E), suggesting that bioenergetics could be dispensable for metabolism-mediated cell growth. Using a high-throughput human protein array, it was discovered that Akt (S473), TP53 (S15, S46, and S392), and p27 (T198) proteins were downregulated in HK2-silencing OECM-1 cells (Figure 5F). Western blot analysis further confirmed that Akt activity, but not another common oncogenic signaling factor Erk1/2, was deceased in HK2-deficient OECM-1 and HSC3 cells (Figure 5G).
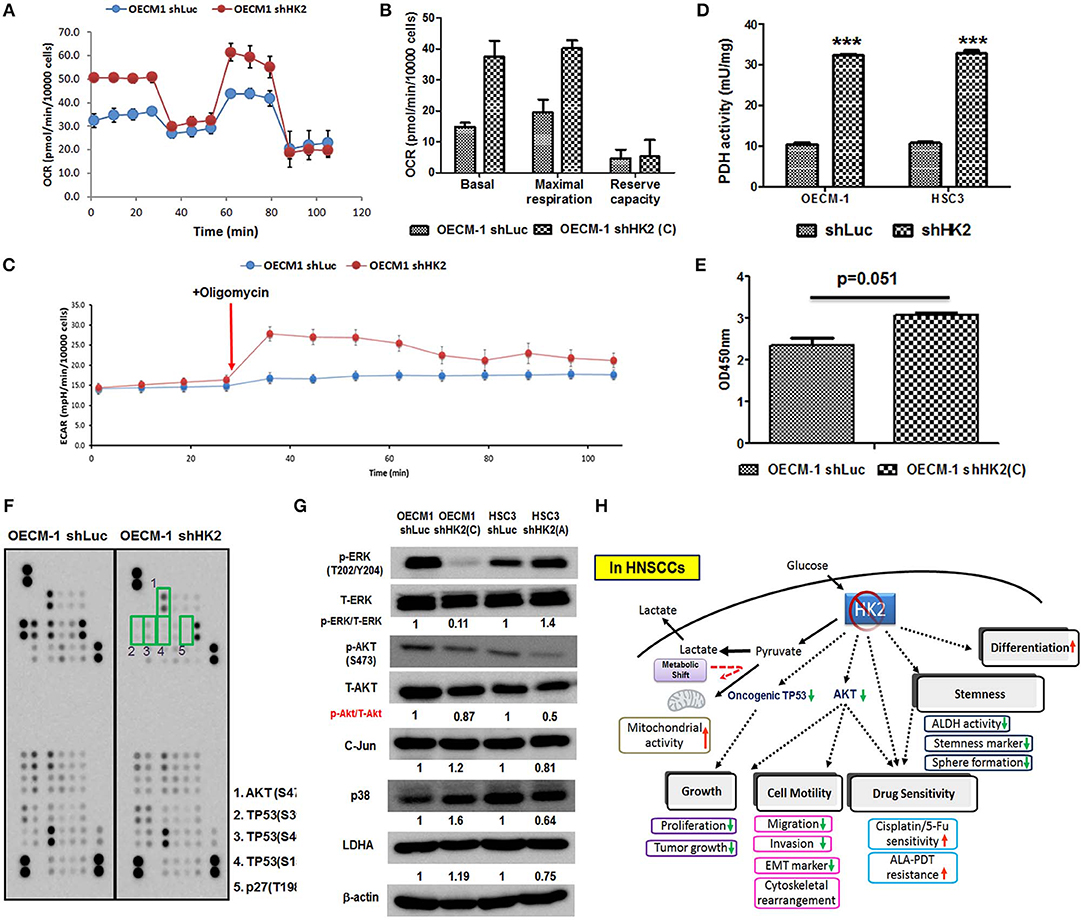
Figure 5. Metabolic and molecular changes in HK2-silencing HNSCC cells. (A,B) Seahorse metabolic analysis showed an elevated basal respiration and maximal respiration in HK2 deficient OECM-1 cells. (C) Sharp increase of ECAR upon addition of ATPase inhibitor oligomycin and (D) increased pyruvate dehydrogenase activity reflected that HK2 loss triggers HNSCC cells toward mitochondrial metabolism. (E) ATP level was slightly increased in HK2-silencing OECM-1 cells. (F) Human protein array analysis for phosphorylated proteins differentially expressed in HK2-silencing OECM-1 cells was performed. Green boxes indicated oncogenic phosphorylated molecules: (1) Akt (S473), (2) TP53 (S392), (3) TP53 (S46), (4) TP53 (S15), and (5) p27 (T198). (G) Western blot analysis confirmed that phosphorylated PKB/Akt proteins is downregulated in HK2-silencing HNSCC cells. p38, P38 mitogen-activated protein kinase; LDHA, lactate dehydrogenase A. (H) Graphic summary of HK2-mediated regulations in HNSCC cells. Data are presented as mean ± SEM (N > 3). ***P < 0.001.
Discussion
In the present study, it was shown that HK2 loss downregulated cell growth, EMT-mediated cell movement, and stemness/differentiation-related treatment sensitivity; in addition, altered bioenergetics and modulation of oncogenic signaling molecules including Akt and phosphorylated TP53 could underlie HK2-mediated regulations in HNSCC cells (Figure 5H). Overall findings concluded that targeting HK2 could be an ideal therapeutic strategy in treating HNSCCs. One interesting discovery from the current study is that in HNSCC cells HK2-mediated malignant suppression is likely through glycolytic manipulation based on the following data: (i) HK2 is highly expressed in HNSCC cells implying that HNSCCs are “glycolytic” tumor; (ii) HK2 inhibition triggers metabolic shift away from glycolysis toward mitochondrial metabolism and (iii) it is found that mitochondria are swelling and lack cristae in HNSCC cells compared with human oral fibroblasts (Supplementary Figure 5). This finding agreed with previous investigations showing that HK2-mediated metabolic modulation is a key machinery to control cancerous characteristics (41, 54, 74, 75), even though it is required to further define the potential impact of mitochondrial HK2 (49, 51) in controlling tumorigenesis in HNSCC cells in the current experimental setting.
Another interesting finding is the potential involvement of phosphorylated TP53 and p27 proteins in HK2-mediated regulations for HNSCC oncogenicity. Based on protein array analysis, HK2 loss resulted in decreased phosphorylated TP53 at transactivation domain (S15 and S46) and C-terminal domain (S392) in OECM-1 cells. While previous sequencing analysis for TP53 gene in OECM-1 cells showed that OECM-1 cells carry mutant TP53 (P139R/V239L) (62), phosphorylation on S15, S46, and S392 of TP53 could be oncogenic in OECM-1 cells. This statement could be supported by previous studies showing that S15 phosphorylation of TP53 contributed mutant TP53 stability, thereby prolonging cell viability in ovarian cancer cells (76), and S392 phosphorylation in mutant TP53 could lead to tumor progression in esophageal squamous cell carcinoma (77). While it was reported that T198 phosphorylation of cell cycle regulator p27 promotes cyclin D1–CDK4–p27 complex assembly (78), as well as increases p27-RhoA–mediated cell motility (79), HK2 silencing–mediated T198 p27 loss could be a potential underlying cue in controlling HNSCC cell growth and migration. Further elucidation for roles of different TP53 phosphorylation sites in both wild-type and mutant HNSCC cells in regulating tumorigenicity is expected.
With extensive studies, including the present investigation, showing that HK2 is a master promoting factor in different stages of carcinogenesis (Supplementary Table 4), much efforts were put in exploring HK-mediated tumor inhibitors, despite that no potent drugs targeting HK2 are yet available in clinic (80–84). Taking the most promising HK2-targeting drug, 3-bromopyruvate (3-BP), as an example, although single treatment of 3-BP, at the appropriate dose and formulation, could effectively destroy glycolytic tumors and seems to be non-toxic to all sorts of vertebrates, certain failure cases were still reported in clinical test (83). It is noteworthy that 3-BP administration also resulted in great pain and inflammation in an HNSCC patient enrolled in a 3-BP trial at the Dayspring Cancer Clinic (http://www.dayspringcancerclinic.com). In addition to a demand of a larger clinical cohort, to achieve better outcomes by using 3-BP or other HK2 inhibitors, as stated in Supplementary Table 4, to cure cancer in clinic, a very recent article suggested that simultaneous or sequential treatment of 3-BP and other conventional therapeutic agents needs to be tested for optimal tumor-killing ability, and/or localized delivery of 3-BP directly into tumor tissues should be considered without affecting normal cells (85). Based on current findings, a more systemic study to test synergic antiproliferative effects of different HK2 inhibitors, not only for 3-BP but also for other known small molecules (listed in Supplementary Table 4), and their underlying molecular mechanisms in combination of treatments of chemotherapeutic drugs, radiotherapy, and targeted therapy (such as EGFR antagonist cetuximab), needs to be conducted, both in vitro and in vivo. In addition to BP-3, it is hoped that other inexpensive and safe anticancer HK2 inhibitors could also be discovered, successfully pass clinical trials, and soon be available in global market.
Data Availability Statement
The datasets generated for this study are available on request to the corresponding author.
Ethics Statement
The studies involving human participants were reviewed and approved by Institutional Review Boards (IRB) of MacKay Memorial Hospital and National Taiwan University Hospital, Taiwan. The patients/participants provided their written informed consent to participate in this study. This animal study was reviewed and approved by Institutional Animal Care and Use Committee (IACUC), National Yang-Ming University.
Author Contributions
W-CL devised and coordinated the study. C-HH, Y-TH, T-YC, L-HC, and C-YC performed experiments. C-HH conducted animal experiments. C-HH and Y-TH performed statistical analysis. C-JL, H-MC, C-LH, J-FL, and K-WC provided samples and critical discussion. W-CL wrote the manuscript. All authors read and approved the final manuscript.
Funding
This work was supported by grants from Ministry of Science and Technology, Taiwan (Grant Nos. NSC-102-2314-B-010-010-, Most-103-2314-B-010-024-MY3, and MOST-106-2314-B-010-005-MY3), Yen Tjing Ling Medical Foundation (Grant No. CI-106-13) and a grant from Ministry of Education, Aiming for the Top University Plan as well as the Higher Education Sprout Project by the Ministry of Education (MOE) in Taiwan.
Conflict of Interest
The authors declare that the research was conducted in the absence of any commercial or financial relationships that could be construed as a potential conflict of interest.
Acknowledgments
We thank the National RNAi Core Facility at Academia Sinica in Taiwan for providing shRNA reagents. We thank Dr. Jude Clapper for review and English revision for the manuscript.
Supplementary Material
The Supplementary Material for this article can be found online at: https://www.frontiersin.org/articles/10.3389/fonc.2020.00176/full#supplementary-material
References
1. Hanahan D, Weinberg RA. The hallmarks of cancer. Cell. (2000) 100:57–70. doi: 10.1016/S0092-8674(00)81683-9
2. Nijsten MW, van Dam GM. Hypothesis: using the Warburg effect against cancer by reducing glucose and providing lactate. Med Hypotheses. (2009) 73:48–51. doi: 10.1016/j.mehy.2009.01.041
3. Dang CV. Links between metabolism and cancer. Genes Dev. (2012) 26:877–90. doi: 10.1101/gad.189365.112
4. Racker E. History of the Pasteur effect and its pathobiology. Mol Cell Biochem. (1974) 5:17–23. doi: 10.1007/BF01874168
5. Warburg O. On the origin of cancer cells. Science. (1956) 123:309–14. doi: 10.1126/science.123.3191.309
6. Hsieh YT, Chen YF, Lin SC, Chang KW, Li WC. Targeting Cellular Metabolism Modulates Head and Neck Oncogenesis. Int J Mol Sci. (2019) 20:E3960. doi: 10.3390/ijms20163960
7. Vander Heiden MG Targeting cancer metabolism: a therapeutic window opens. Nat Rev Drug Discov. (2011) 10:671–84. doi: 10.1038/nrd3504
8. Vander Heiden MG, De Berardinis RJ. Understanding the Intersections between Metabolism and Cancer Biology. Cell. (2017) 168:657–669. doi: 10.1016/j.cell.2016.12.039
9. Hamanaka RB, Chandel NS. Targeting glucose metabolism for cancer therapy. J Exp Med. (2012) 209:211–5. doi: 10.1084/jem.20120162
10. Gatenby RA, Gillies RJ. Why do cancers have high aerobic glycolysis? Nat Rev Cancer. (2004) 4:891–9. doi: 10.1038/nrc1478
11. Lunt SY, Vander Heiden MG. Aerobic glycolysis: meeting the metabolic requirements of cell proliferation. Annu Rev Cell Dev Biol. (2011) 27:441–64. doi: 10.1146/annurev-cellbio-092910-154237
12. Wilson JE. Isozymes of mammalian hexokinase: structure, subcellular localization and metabolic function. J Exp Biol. (2003) 206, 2049–57. doi: 10.1242/jeb.00241
13. Roberts DJ, Miyamoto S. Hexokinase II integrates energy metabolism and cellular protection: akting on mitochondria and TORCing to autophagy. Cell Death Differ. (2015) 22:248–57. doi: 10.1038/cdd.2014.173
14. Liu Y, Wu K, Shi L, Xiang F, Tao K, Wang G. Prognostic significance of the metabolic marker hexokinase-2 in various solid tumors: a meta-analysis. PLoS ONE. (2016) 11:e0166230. doi: 10.1371/journal.pone.0166230
15. Bacci M, Giannoni E, Fearns A, Ribas R, Gao Q, Taddei ML, et al. Morandi: miR-155 drives metabolic reprogramming of ER+ breast cancer cells following long-term estrogen deprivation and predicts clinical response to aromatase inhibitors. Cancer Res. (2016) 76:1615–26. doi: 10.1158/0008-5472.CAN-15-2038
16. van't LJ, Veer Dai H, van de Vijver MJ, He YD, Hart AA, Mao M, et al. Gene expression profiling predicts clinical outcome of breast cancer. Nature. (2002) 415:530–6. doi: 10.1038/415530a
17. Liu X, Miao W, Huang M, Li L, Dai X, Wang Y. Elevated hexokinase II expression confers acquired resistance to 4-hydroxytamoxifen in breast cancer cells. Mol Cell Proteomics. (2019) 18:2273–2284. doi: 10.1074/mcp.RA119.001576
18. Palmieri D, Fitzgerald D, Shreeve SM, Hua E, Bronder JL, Weil RJ, et al. Analyses of resected human brain metastases of breast cancer reveal the association between up-regulation of hexokinase 2 and poor prognosis. Mol Cancer Res. (2009) 7:1438–45. doi: 10.1158/1541-7786.MCR-09-0234
19. Yang X, Cheng Y, Li P, Tao J, Deng X, Zhang X, et al. A lentiviral sponge for miRNA-21 diminishes aerobic glycolysis in bladder cancer T24 cells via the PTEN/PI3K/AKT/mTOR axis. Tumour Biol. (2015) 36:383–91. doi: 10.1007/s13277-014-2617-2
20. Huang X, Liu M, Sun H, Wang F, Xie X, Chen X, et al. HK2 is a radiation resistant and independent negative prognostic factor for patients with locally advanced cervical squamous cell carcinoma. Int J Clin Exp Pathol. (2015) 8:4054–63.
21. Iwamoto M, Kawada K, Nakamoto Y, Itatani Y, Inamoto S, Toda K, et al. Regulation of 18F-FDG accumulation in colorectal cancer cells with mutated KRAS. J Nucl Med. (2014) 55:2038–44. doi: 10.2967/jnumed.114.142927
22. Jin Z, Gu J, Xin X, Li Y, Wang H. Expression of hexokinase 2 in epithelial ovarian tumors and its clinical significance in serous ovarian cancer. Eur J Gynaecol Oncol. (2014) 35:519–24.
23. Wolf A, Agnihotri S, Micallef J, Mukherjee J, Sabha N, Cairns R, et al. Hexokinase 2 is a key mediator of aerobic glycolysis and promotes tumor growth in human glioblastoma multiforme. J Exp Med. (2011) 208:313–26. doi: 10.1084/jem.20101470
24. Kwee SA, Hernandez B, Chan O, Wong L. Choline kinase alpha and hexokinase-2 protein expression in hepatocellular carcinoma: association with survival. PLoS ONE. (2012) 7:e46591. doi: 10.1371/journal.pone.0046591
25. Chen J, Zhang S, Li Y, Tang Z, Kong W. Hexokinase 2 overexpression promotes the proliferation and survival of laryngeal squamous cell carcinoma. Tumour Biol. (2014) 35:3743–53. doi: 10.1007/s13277-013-1496-2
26. Wang H, Wang L, Zhang Y, Wang J, Deng Y, Lin D. Inhibition of glycolytic enzyme hexokinase II (HK2) suppresses lung tumor growth. Cancer Cell Int. (2016) 16: 38. doi: 10.1186/s12935-016-0313-6
27. Botzer LE, Maman S, Sagi-Assif O, Meshel T, Nevo I, Yron I. Hexokinase 2 is a determinant of neuroblastoma metastasis. Br J Cancer. (2016) 114:759–66. doi: 10.1038/bjc.2016.26
28. Binderup T, Knigge UP, Federspiel B, Sommer P, Hasselby JP, Loft A. Gene expression of glucose transporter 1 (GLUT1), hexokinase 1 and hexokinase 2 in gastroenteropancreatic neuroendocrine tumors: correlation with F-18-fluorodeoxyglucose positron emission tomography and cellular proliferation. Diagnostics. (2013) 3:372–84. doi: 10.3390/diagnostics3040372
29. Ogawa H, Nagano H, Konno M, Eguchi H, Koseki J, Kawamoto K, et al. The combination of the expression of hexokinase 2 and pyruvate kinase M2 is a prognostic marker in patients with pancreatic cancer. Mol Clin Oncol. (2015) 3:563–571. doi: 10.3892/mco.2015.490
30. Wang L, Xiong H, Wu F, Zhang Y, Wang J, Zhao L, et al. Hexokinase 2-mediated Warburg effect is required for PTEN- and p53-deficiency-driven prostate cancer growth. Cell Rep. (2014) 8:1461–74. doi: 10.1016/j.celrep.2014.07.053
31. Wu J, Hu L, Wu F, Zou L, He T. Poor prognosis of hexokinase 2 overexpression in solid tumors of digestive system: a meta-analysis. Oncotarget. (2017) 8:32332–44. doi: 10.18632/oncotarget.15974
32. Jiang S, Zhang LF, Zhang HW, Hu S, Lu MH, Liang S, et al. A novel miR-155/miR-143 cascade controls glycolysis by regulating hexokinase 2 in breast cancer cells. EMBO J. (2012) 31:1985–98. doi: 10.1038/emboj.2012.45
33. Li M, Jin R, Wang W, Zhang T, Sang J, Li N, et al. STAT3 regulates glycolysis via targeting hexokinase 2 in hepatocellular carcinoma cells. Oncotarget. (2017) 8:24777–84. doi: 10.18632/oncotarget.15801
34. Li J, Liu T, Zhao L, Chen W, Hou H, Ye Z, et al. Ginsenoside 20(S)Rg3 inhibits the Warburg effect through STAT3 pathways in ovarian cancer cells. Int J Oncol. (2015) 46:775–81. doi: 10.3892/ijo.2014.2767
35. Li Z, Li X, Wu S, Xue M, Chen W. Long non-coding RNA UCA1 promotes glycolysis by upregulating hexokinase 2 through the mTOR-STAT3/microRNA143 pathway. Cancer Sci. (2014) 105:951–5. doi: 10.1111/cas.12461
36. Li W, Ma X, Li N, Liu H, Dong Q, Zhang J, et al. Resveratrol inhibits hexokinases II mediated glycolysis in non-small cell lung cancer via targeting Akt signaling pathway. Exp Cell Res. (2016) 349:320–327. doi: 10.1016/j.yexcr.2016.11.002
37. Liu CC, Chou KT, Hsu JW, Lin JH, Hsu TW, Yen DH, et al. High metabolic rate and stem cell characteristics of esophageal cancer stem-like cells depend on the Hsp27-AKT-HK2 pathway. Int J Cancer. (2019) 145:2144–2156. doi: 10.1002/ijc.32301
38. Gao X, Han H. Jolkinolide B inhibits glycolysis by downregulating hexokinase 2 expression through inactivating the Akt/mTOR pathway in non-small cell lung cancer cells. J Cell Biochem. (2018) 119:4967–4974. doi: 10.1002/jcb.26742
39. Kim JE, He Q, Chen Y, Shi C, Yu K. mTOR-targeted therapy: differential perturbation to mitochondrial membrane potential and permeability transition pore plays a role in therapeutic response. Biochem Biophys Res Commun. (2014) 447:184–91. doi: 10.1016/j.bbrc.2014.03.124
40. deWaal D, Nogueira V, Terry AR, Patra KC, Jeon SM, Guzman G, et al. Hexokinase-2 depletion inhibits glycolysis and induces oxidative phosphorylation in hepatocellular carcinoma and sensitizes to metformin. Nat Commun. (2018) 9:446. doi: 10.1038/s41467-017-02733-4
41. Xiong X, Wen YA, Mitov MI, CO M, Miyamoto S, Gao T. PHLPP regulates hexokinase 2-dependent glucose metabolism in colon cancer cells. Cell Death Discov. (2017) 3:16103. doi: 10.1038/cddiscovery.2016.103
42. Jiao L, Zhang HL, Li DD, Yang KL, Tang J, Li X, et al. Regulation of glycolytic metabolism by autophagy in liver cancer involves selective autophagic degradation of HK2 (hexokinase 2). Autophagy. (2018) 14:671–684. doi: 10.1080/15548627.2017.1381804
43. Zhang XY, Zhang M, Cong Q, Zhang MX, Zhang MY, Lu YY, et al. Hexokinase 2 confers resistance to cisplatin in ovarian cancer cells by enhancing cisplatin-induced autophagy. Int J Biochem Cell Biol. (2018) 95:9–16. doi: 10.1016/j.biocel.2017.12.010
44. Arora KK, Pedersen PL. Functional significance of mitochondrial bound hexokinase in tumor cell metabolism. Evidence for preferential phosphorylation of glucose by intramitochondrially generated ATP. J Biol Chem. (1988) 263:17422–8.
45. Mathupala SP, Ko YH, Pedersen PL. Hexokinase II: cancer's double-edged sword acting as both facilitator and gatekeeper of malignancy when bound to mitochondria. Oncogene. (2006) 25:4777–86. doi: 10.1038/sj.onc.1209603
46. Zhang D, Yip YM, Li L. In silico construction of HK2-VDAC1 complex and investigating the HK2 binding-induced molecular gating mechanism of VDAC1. Mitochondrion. (2016) 30:222–8. doi: 10.1016/j.mito.2016.08.009
47. Nagdas S, Kashatus JA, Nascimento A, Hussain SS, Trainor RE, Pollock SR, et al. Drp1 promotes KRas-driven metabolic changes to drive pancreatic tumor growth. Cell Rep. (2019) 28:45–59.e5. doi: 10.1016/j.celrep.2019.07.031
48. Cheung EC, Ludwig RL, Vousden KH. Mitochondrial localization of TIGAR under hypoxia stimulates HK2 and lowers ROS and cell death. Proc Natl Acad Sci USA. (2012) 109:20491–6. doi: 10.1073/pnas.1206530109
49. Neary CL, Pastorino JG. Akt inhibition promotes hexokinase 2 redistribution and glucose uptake in cancer cells. J Cell Physiol. (2013) 228:1943–8. doi: 10.1002/jcp.24361
50. Gao F, Li M, Liu WB, Zhou ZS, Zhang R, Li JL, et al. Epigallocatechin gallate inhibits human tongue carcinoma cells via HK2mediated glycolysis. Oncol Rep. (2015) 33:1533–9. doi: 10.3892/or.2015.3727
51. Hou X, Liu Y, Liu H, Chen X, Liu M, Che H, et al. PERK silence inhibits glioma cell growth under low glucose stress by blockage of p-AKT and subsequent HK2's mitochondria translocation. Sci Rep. (2015) 5:9065. doi: 10.1038/srep09065
52. Gregersen LH, Jacobsen A, Frankel LB, Wen J, Krogh A, Lund AH. MicroRNA-143 down-regulates Hexokinase 2 in colon cancer cells. BMC Cancer. (2012) 12:232. doi: 10.1186/1471-2407-12-232
53. Yoshino H, Enokida H, Itesako T, Kojima S, Kinoshita T, Tatarano S, et al. Tumor-suppressive microRNA-143/145 cluster targets hexokinase-2 in renal cell carcinoma. Cancer Sci. (2013) 104:1567–74. doi: 10.1111/cas.12280
54. Guo W, Qiu Z, Wang Z, Wang Q, Tan N, Chen T, et al. MiR-199a-5p is negatively associated with malignancies and regulates glycolysis and lactate production by targeting hexokinase 2 in liver cancer. Hepatology. (2015) 62:1132–44. doi: 10.1002/hep.27929
55. Qin Y, Cheng C, Lu H, Wang Y. MiR-4458 suppresses glycolysis and lactate production by directly targeting hexokinase2 in colon cancer cells. Biochem Biophys Res Commun. (2016) 469:37–43. doi: 10.1016/j.bbrc.2015.11.066
56. Song J, Wu X, Liu F, Li M, Sun Y, Wang Y, et al. Long non-coding RNA PVT1 promotes glycolysis and tumor progression by regulating miR-497/HK2 axis in osteosarcoma. Biochem Biophys Res Commun. (2017) 490:217–224. doi: 10.1016/j.bbrc.2017.06.024
57. Fan L, Huang C, Li J, Gao T, Lin Z, Yao T. Long noncoding RNA urothelial cancer associated 1 regulates radioresistance via the hexokinase 2/glycolytic pathway in cervical cancer. Int J Mol Med. (2018) 42:2247–59. doi: 10.3892/ijmm.2018.3778
58. Ma Y, Hu M, Zhou L, Ling S, Li Y, Kong B. Long non-coding RNA HOTAIR promotes cancer cell energy metabolism in pancreatic adenocarcinoma by upregulating hexokinase-2. Oncol Lett. (2019) 18:2212–9. doi: 10.3892/ol.2019.10551
59. Lee HG, Kim H, Son T, Jeong Y, Kim SU, Dong SM, et al. Regulation of HK2 expression through alterations in CpG methylation of the HK2 promoter during progression of hepatocellular carcinoma. Oncotarget. (2016) 7:41798–810. doi: 10.18632/oncotarget.9723
60. Chen YS, Huang WL, Chang SH, Chang KW, Kao SY, Lo JF, et al. Enhanced filopodium formation and stem-like phenotypes in a novel metastatic head and neck cancer cell model. Oncol Rep. (2013) 30:2829–37. doi: 10.3892/or.2013.2772
61. Liu CJ, Chang WJ, Chen CY, Sun FJ, Cheng HW, Chen TY, et al. Dynamic cellular and molecular modulations of diabetes mediated head and neck carcinogenesis. Oncotarget. (2015) 6:29268–84. doi: 10.18632/oncotarget.4922
62. Chen TY, Hsieh YT, Huang JM, Liu CJ, Chuang LT, Huang PC, et al. Determination of pyruvate metabolic fates modulates head and neck tumorigenesis. Neoplasia. (2019) 21:641–52. doi: 10.1016/j.neo.2019.04.007
63. Deng M, Bragelmann J, Kryukov I, Saraiva-Agostinho N, Perner S. FirebrowseR: an R client to the broad institute's firehose pipeline. Database. (2017) 2017:160. doi: 10.1093/database/baw160
64. Chandrashekar DS, Bashel B, Balasubramanya SAH, Creighton CJ, Ponce-Rodriguez I, Chakravarthi B, et al. UALCAN: a portal for facilitating tumor subgroup gene expression and survival analyses. Neoplasia. (2017) 19:649–58. doi: 10.1016/j.neo.2017.05.002
65. Uhlen M, Zhang C, Lee S, Sjostedt E, Fagerberg L, Bidkhori G, et al. A pathology atlas of the human cancer transcriptome. Science. (2017) 357:eaan2507. doi: 10.1126/science.aan2507
66. San-Millan Brooks GA. Reexamining cancer metabolism: lactate production for carcinogenesis could be the purpose and explanation of the Warburg Effect. Carcinogenesis. (2017) 38:119–33. doi: 10.1093/carcin/bgw127
67. Fife CM, McCarroll JA, Kavallaris M. Movers and shakers: cell cytoskeleton in cancer metastasis. Br J Pharmacol. (2014) 171:5507–23. doi: 10.1111/bph.12704
68. Brabletz T, Kalluri R, Nieto MA, Weinberg RA. EMT in cancer. Nat Rev Cancer. (2018) 18:128–134. doi: 10.1038/nrc.2017.118
69. Seshadri M, Spernyak JA, Mazurchuk R, Camacho SH, Oseroff AR, Cheney RT, et al. Tumor vascular response to photodynamic therapy and the antivascular agent 5,6-dimethylxanthenone-4-acetic acid: implications for combination therapy. Clin Cancer Res. (2005) 11:4241–50. doi: 10.1158/1078-0432.CCR-04-2703
70. Nokes B, Apel M, Jones C, Brown G, Lang JE. Aminolevulinic acid (ALA): photodynamic detection and potential therapeutic applications. J Surg Res. (2013) 181:262–71. doi: 10.1016/j.jss.2013.02.002
71. Fayter D, Corbett M, Heirs M, Fox D, Eastwood A. A systematic review of photodynamic therapy in the treatment of pre-cancerous skin conditions, barrett's oesophagus and cancers of the biliary tract, brain, head and neck, lung, oesophagus and skin. Health Technol Assess. (2010) 14:1–288. doi: 10.3310/hta14370
72. Kulsum S, Sudheendra HV, Pandian R, Ravindra DR, Siddappa G, R N, et al. Cancer stem cell mediated acquired chemoresistance in head and neck cancer can be abrogated by aldehyde dehydrogenase 1 A1 inhibition. Mol Carcinog. (2017) 56:694–711. doi: 10.1002/mc.22526
73. Keibler MA, Wasylenko TM, Kelleher JK, Iliopoulos O, Vander Heiden MG, Stephanopoulos G. Metabolic requirements for cancer cell proliferation. Cancer Metab. (2016) 4:16. doi: 10.1186/s40170-016-0156-6
74. Gershon TR, Crowther AJ, Liu H, Miller CR, Deshmukh M. Cerebellar granule neuron progenitors are the source of Hk2 in the postnatal cerebellum. Cancer Metab. (2013) 1:15. doi: 10.1186/2049-3002-1-15
75. Li LQ, Yang Y, Chen H, Zhang L, Pan D, Xie WJ. MicroRNA-181b inhibits glycolysis in gastric cancer cells via targeting hexokinase 2 gene. Cancer Biomark. (2016) 17:75–81. doi: 10.3233/CBM-160619
76. Sonego M, Schiappacassi M, Lovisa S, Dall'Acqua A, Bagnoli M, Lovat F, et al. Stathmin regulates mutant p53 stability and transcriptional activity in ovarian cancer. EMBO Mol Med. (2013) 5:707–22. doi: 10.1002/emmm.201201504
77. Matsumoto M, Furihata M, Kurabayashi A, Sasaguri S, Araki K, Hayashi H, et al. Prognostic significance of serine 392 phosphorylation in overexpressed p53 protein in human esophageal squamous cell carcinoma. Oncology. (2004) 67:143–50. doi: 10.1159/000081001
78. Larrea MD, Liang J, Da Silva T, Hong F, Shao SH, Han K, et al. Phosphorylation of p27Kip1 regulates assembly and activation of cyclin D1-Cdk4. Mol Cell Biol. (2008) 28:6462–72. doi: 10.1128/MCB.02300-07
79. Larrea MD, Hong F, Wander SA, da Silva TG, Helfman D, Lannigan D, et al. RSK1 drives p27Kip1 phosphorylation at T198 to promote RhoA inhibition and increase cell motility. Proc Natl Acad Sci USA. (2009) 106:9268–73. doi: 10.1073/pnas.0805057106
80. Dai W, Wang F, Lu J, Xia Y, He L, Chen K, et al. By reducing hexokinase 2, resveratrol induces apoptosis in HCC cells addicted to aerobic glycolysis and inhibits tumor growth in mice. Oncotarget. (2015) 6:13703–17. doi: 10.18632/oncotarget.3800
81. Geng C, Li J, Ding F, Wu G, Yang Q, Sun Y, et al. Curcumin suppresses 4-hydroxytamoxifen resistance in breast cancer cells by targeting SLUG/Hexokinase 2 pathway. Biochem Biophys Res Commun. (2016) 473:147–53. doi: 10.1016/j.bbrc.2016.03.067
82. Li W, Zheng M, Wu S, Gao S, Yang M, Li Z, et al. Benserazide, a dopadecarboxylase inhibitor, suppresses tumor growth by targeting hexokinase 2. J Exp Clin Cancer Res. (2017) 36:58. doi: 10.1186/s13046-017-0530-4
83. Lis P, Dylag M, Niedzwiecka K, Ko YH, Pedersen PL, Goffeau A. The HK2 Dependent “Warburg effect” and mitochondrial oxidative phosphorylation in Cancer: targets for effective therapy with 3-Bromopyruvate. Molecules. (2016) 21:E1730. doi: 10.3390/molecules21121730
84. Lin H, Zeng J, Xie R, Schulz MJ, Tedesco R, Qu J, et al. Discovery of a Novel 2,6-disubstituted glucosamine series of potent and selective hexokinase 2 inhibitors. ACS Med Chem Lett. (2016) 7:217–22. doi: 10.1021/acsmedchemlett.5b00214
Keywords: head and neck cancer, hexokinase 2, cellular malignancy, metabolic shift, therapeutic efficacy
Citation: Li W-C, Huang C-H, Hsieh Y-T, Chen T-Y, Cheng L-H, Chen C-Y, Liu C-J, Chen H-M, Huang C-L, Lo J-F and Chang K-W (2020) Regulatory Role of Hexokinase 2 in Modulating Head and Neck Tumorigenesis. Front. Oncol. 10:176. doi: 10.3389/fonc.2020.00176
Received: 26 November 2019; Accepted: 31 January 2020;
Published: 03 March 2020.
Edited by:
Victor C. Kok, Asia University, TaiwanReviewed by:
Lo-Lin Tsai, Taipei Medical University, TaiwanPei-ling Hsieh, China Medical University, Taiwan
Copyright © 2020 Li, Huang, Hsieh, Chen, Cheng, Chen, Liu, Chen, Huang, Lo and Chang. This is an open-access article distributed under the terms of the Creative Commons Attribution License (CC BY). The use, distribution or reproduction in other forums is permitted, provided the original author(s) and the copyright owner(s) are credited and that the original publication in this journal is cited, in accordance with accepted academic practice. No use, distribution or reproduction is permitted which does not comply with these terms.
*Correspondence: Wan-Chun Li, d2NsaUB5bS5lZHUudHc=