- 1School of Basic Pharmaceutical and Toxicological Sciences, College of Pharmacy, University of Louisiana Monroe, Monroe, LA, United States
- 2Department of Pharmaceutical Sciences, Texas Tech University Health Sciences Center, School of Pharmacy, Amarillo, TX, United States
Tumor-induced angiogenesis has been a significant focus of anti-cancer therapies for several decades. The immature and “leaky” tumor vasculature leads to significant cancer cell intravasation, increasing the metastatic potential, while the disoriented and hypo-perfused tumor vessels hamper the anti-tumor efficacy of immune cells and prevent the efficient diffusion of chemotherapeutic drugs. Therefore, tumor vascular normalization has emerged as a new treatment goal, aiming to provide a mature tumor vasculature, with higher perfusion, decreased cancer cell extravasation, and higher efficacy for anti-cancer therapies. Here we propose an overview of the nanodelivery approaches that target tumor vasculature, aiming to achieve vascular normalization. At the same time, abnormal vascular architecture and leaky tumor vessels have been the cornerstone for nanodelivery approaches through the enhanced permeability and retention (EPR) effect. Vascular normalization presents new opportunities and requirements for efficient nanoparticle delivery against the tumor cells and overall improved anti-cancer therapies.
Introduction
Anti-angiogenic therapy has been a major focus area of anti-cancer research for several decades (1). Blocking the immature, disorganized tumor-derived vessels led to significant tumor inhibitory effects in preclinical models and rendered anti-angiogenic therapy as a promising approach for cancer treatment, especially in combination with chemotherapy. A large volume of preclinical data with angiogenesis inhibitors led to the FDA approval and release of anti-angiogenic therapies in the clinic (2, 3). The most characteristic target is vascular endothelial growth factor (VEGF), where anti-VEGF therapy, such as bevacizumab, a humanized monoclonal anti-VEGF antibody, or sorafenib and sunitinib, VEGF receptor tyrosine kinase inhibitors, were incorporated in anti-cancer treatment options either as single agents or adjuvant therapy (3, 4). However, the clinical outcome of anti-angiogenic therapy did not meet the expectations: although progression-free survival was increased in some cases, such as metastatic colorectal (5) and ovarian cancer (6), or renal cell (7) and hepatocellular carcinoma (8), in other cancers, such as breast, melanoma, pancreatic and prostate, progression-free survival and overall survival were not increased (4, 9). The main pitfall of anti-angiogenic treatment is the impaired tumor perfusion, which limits the access to chemotherapeutic agents, impedes the tumoricidal activity of immune cells, and increases hypoxia, further driving tumor aggressiveness and metastasis (Figure 1) (4, 10).
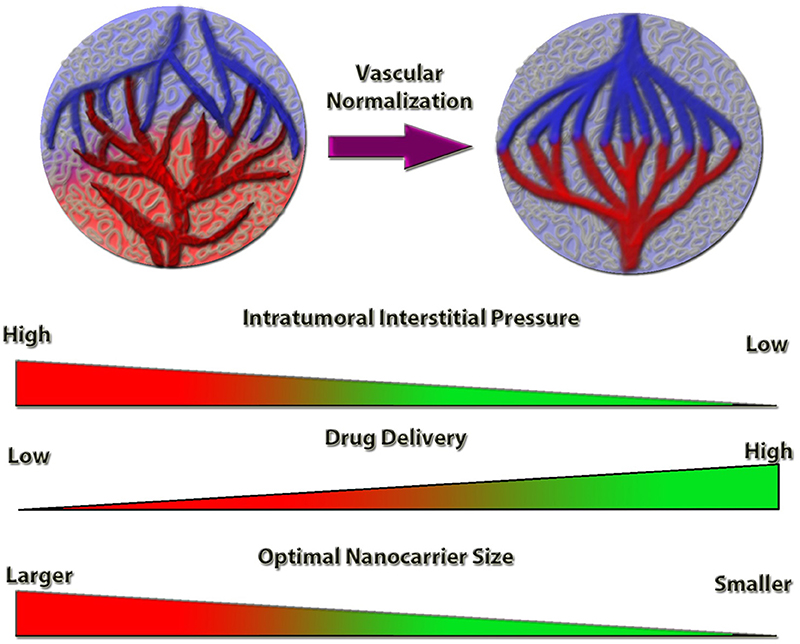
Figure 1. Schematic representation of the tumor vessel normalization's impact on intratumoral interstitial pressure and drug delivery efficiency. Normalized tumor vessels decrease tumor hypoxia and intratumoral interstitial pressure, which increases the anti-cancer drug delivery efficiency. Nanocarrier size is a limiting factor for optimal targeting and delivery, the efficiency of which is inversely proportional to the nanocarrier size, under vessel normalization conditions.
The rapid growth of solid tumors induces the secretion of angiogenic factors by the tumor cells to accommodate the needs of their increased proliferation rate. This results to the rapid development of imperfect vascularization in the tumor area, characterized by tortuous and leaky vessels. The imperfections of the rapidly growing vasculature have been identified as porous-like structures of ~400–600 nm in diameter, leading to the enhanced permeability and retention (EPR) effect (11). The features of the EPR include a size-dependent accumulation of molecules and structures due to the leaky vessels, where particles (such as nanoparticles) and macromolecules will diffuse out of the tumor vessels that bear the imperfections, compared to any healthy tissue, where these imperfections are absent. This can induce an augmented drug concentration in the tumor area, further supported by the impaired lymphatic drainage associated with the abnormal tumor vasculature (12).
The limited clinical outcome of anti-angiogenic therapy has driven the last decade the concept of vascular normalization as a complementary therapeutic approach for anti-cancer treatment. Normalization of tumor vasculature is expected to provide a properly oriented, well-constructed vascular network with reduced vascular density, increased perfusion and limited hypoxia, which will lead to better drug delivery and anti-cancer efficacy (13, 14). An increasing number of studies demonstrate the promising outcome of vascular normalization strategies. Lower doses of current anti-angiogenic therapies, such as bevacizumab, are reported to achieve tumor vessel normalization (4, 13). Even in aggressive tumors, such as glioblastoma, treatment with cediranib, an anti-angiogenic agent, improved vessel perfusion in a subset of patients and increased overall survival (15). Tumor vessel normalization has also been achieved by non-pharmacological approaches; aerobic exercise can drive vascular normalizing effects and improve chemotherapeutic efficacy. The leading player, in that case, is considered to be the shear stress, which, when increased, enhances vascular integrity through secretion of vascular normalization mediators, such as thrombospondin-1 (16–19).
The limitations of vascular normalization approaches follow, to a certain extent, the limitations of anti-angiogenic therapy. The most common is the evasive resistance, the acquired resistance of the endothelium towards anti-angiogenic therapy that targets a growth factor, by upregulating others, which will compensate for its inhibition (4). The main goal for tumor vascular normalization is the improvement of anti-cancer drug delivery. However, the window for anti-cancer therapy is normally short, not easily identifiable, and does not occur uniformly in the patient population (13, 14). The extension and identification of this therapeutic window consist the primary goal of current studies focusing on vascular normalization, and one of the main goals is the identification of markers denoting the potent therapeutic window for vascular normalization. An example is Anterior gradient 2, a plasma protein secreted from tumor cells, which was proposed as a vascular normalization marker during anti-angiogenic treatment (20). Certain approaches to overcome that therapeutic window have been proposed, such as the simultaneous administration of anti-angiogenic and chemotherapeutic drugs through nanoparticle delivery (21).
Nanodelivery methods ensure the selective targeting of a specific tissue, offering improved delivery with significantly fewer side-effects (11). Nanoformulations are known to significantly enhance the effect of certain compounds compared to direct administration (22) and nanodelivery methods are incorporated into therapeutics of multiple diseases, including cancer (23). In this mini-review we summarize the current knowledge from studies, where nanodelivery is utilized for or in conjunction with tumor vascular normalization and discuss advantages, limitations and potentials.
Nanoformulations and Cancer Nanotherapeutics
Traditional drug delivery systems have been unable to address the complex therapeutic and physicochemical necessities presented by the traditional and new active molecules, including poor aqueous solubility, poor specificity, unfavorable pharmacokinetics and high toxicity (11). Nanotechnology has steadily grown to a promising field of research and application for the diagnosis and treatment of various diseases, among which is cancer. Nanocarriers are colloidal systems used for drug delivery, capable of entrapping, encapsulating and delivering active molecules to tissues and cells (11). As their name suggests, nanocarriers have a particle size at the submicron range (<1 μm), though it is generally regarded that nanocarriers used through systemic administration will typically have a size below 200–250 nm. This stems from the natural filtering mechanism of the body, where nanoparticles of larger dimensions are retained and removed from the circulation through splenic filtration (24). Nanotechnology has yielded significant advantages over traditional pharmaceutical formulations, such as: (a) improved drug stability; (b) improved pharmacokinetics/biodistribution; (c) reduced non-specific toxicity; (d) reduction in drug dosage and dosing frequency, and; (e) high drug loading for compounds insoluble in water (11).
The growing field of nanotechnology has yielded new and innovative carriers with distinct and multifaceted properties, while new formulations and approaches are constantly being developed. We provide here a brief overview of the most important aspects of nanotechnology, describing the most frequently studied nanocarriers. Though there are overlaps or combinations of the technological advancements, the classification of the nanocarriers typically relies on their composition, having three major categories: (a) lipid-based; (b) polymer-based, and (c) inorganic nanocarriers (25).
Among the lipid-based formulations, liposomes are the best known and studied nanocarriers. They have achieved broad recognition for their capacity to protect and deliver active compounds, with improved biodistribution profiles and reduced toxicity (11, 25). Liposomes are primarily used for hydrophilic compounds, though their lipid bilayer allows the entrapment of hydrophobic compounds as well. More importantly, liposomal formulations have received FDA approval for use in cancer treatment, i.e., liposomal formulation of doxorubicin—Doxil® (26), among others, which constitutes them as a reliable, safe, tested, and thus attractive nanocarrier model for human treatment or new drug development (27, 28).
Solid lipid nanoparticles (SLNs) are lipid-based nanocarriers, commonly prepared by dispersing melted solid lipids in water in the presence of a stabilizing emulsifier (29). Similarly to the oil-in-water (o/w) emulsions, the hydrophobic environment inside the nanocarriers makes them ideal for the entrapment and delivery of molecules with low aqueous solubility, though, in contrast to the o/w emulsions, the hydrophobic core is solid and not liquid (29). Unlike o/w emulsions, which require oils that may present significant toxicity or biocompatibility limitations (30), both the liposomes and the SLNs utilize lipids commonly found in cells (i.e., phospholipids) that can be of natural source or synthetically made/modified. Not surprisingly, liposomes and SLNs are considered biocompatible and biodegradable, with an excellent safety record (31). In fact, synthetic approaches using polymer synthesis and chemical attachment of antibodies or targeting moieties have advanced the development of multifunctional lipids for long-circulating nanocarriers that may actively target a variety of cells, such as macrophages, endothelial or tumor cells (32–34).
Similarly, polymer-based nanocarriers have emerged as promising nanocarriers for drug delivery. The progress on polymer chemistry has allowed the development of new polymer structures with multi-faceted and highly adjustable properties, advancing the development of nanosized micelles, solid-core nanoparticles, polymersomes and dendrimers (11). These carriers have tunable characteristics, defined by the physicochemical properties of the used polymer or combination of polymers, capable of delivering unstable hydrophilic and hydrophobic compounds, or molecules that otherwise would not be capable of crossing the cell membrane, such as nucleic acids (i.e., si/miRNAs and plasmids).
Finally, inorganic nanoparticles are frequently composed of magnetic iron oxide, silica oxide and gold, among other materials. Similar to the other categories, the inorganic nanoparticles can be surface-modified to achieve long-circulating properties, actively target specific cells and tissues, and protect active compounds. Furthermore, inorganic nanoparticles, such as iron oxide/magnetic nanoparticles, can respond to external stimuli, such as magnetism, which permits their detection or active targeting to specific parts of the body, or demonstrate unique optical properties for improved in vivo imaging, such as quantum dots and up-converting nanoparticles, which lipids and polymers cannot provide (35–37).
The efficacy of nanodelivery in different tumors largely varies, guided by the variable tumor vascular characteristics, such as vessel architecture, interstitial fluid and extracellular matrix composition, phagocyte infiltration and presence of necrotic areas. Parameters, such as the extravasation of the nanoparticles from tumor blood vessels, their diffusion through the extracellular tissue and their interaction with the tumor microenvironment constitute the EPR effect, elegantly analyzed by Bertrand et al. (23). The EPR effect in solid tumors was initially described ~3 decades ago, and was one of the driving forces for the scientific advancements taking place in the field of nanotechnology. The goal of nanotechnology-based treatment is to utilize or enhance the EPR effect in tumors, allowing better pharmacological targeting of the tumor tissue, leading to an increasing build-up of the nanocarriers with the active compound to the tumor area, which is further supported by the impaired lymphatic drainage in solid tumors (38). Alternatively, sonoporation, the combination of ultrasound and microbubbles, has improved liposome accumulation and their penetration through the tumor vasculature into the tumor interstitium (39).
The EPR effect has received criticism recently, regarding its significance in the passive targeting to tumors, its dependency on the stage and the type of tumor (40), and whether it is present in human tumors (41). There is a potential sift on the paradigm on the use of nanoformulations and their drug delivery capacity under rapidly growing vs. slowly growing tumors, as well as the influence of the vascular architectural structure. Below we summarize the up-to-date literature for nanotherapeutics targeting vessel normalization and their potential for anti-angiogenic therapies.
Vessel Normalization
The need for vascular normalization has been further highlighted with the recent advances in tumor immunotherapy. Several antibodies targeting the immune checkpoint proteins, such as pembrolizumab, nivolumab and ipilimumab have been approved for clinical practice (42–44), and immune checkpoint inhibitions consist a revolutionary anti-cancer approach for solid tumors (45). However, a subset of patients does not benefit, and the reasons are not known (46). A potential reason for the ineffectiveness of tumor immunotherapy for the non-responding patients could be the inability of the immune cells to sufficiently access the tumor mass, and tumor vascular normalization looks a promising solution (14, 47).
A groundbreaking study for nanodelivery and tumor vasculature normalization was from Rakesh Jain's lab, where they showed that vascular endothelial growth factor receptor-2 (VEGFR2) targeting led to tumor vessel normalization and the subsequent decrease of the intratumoral interstitial pressure, improving nanoparticle delivery. It was also demonstrated that smaller nanoparticles, of 12 nm diameter, are more potent to invade rapidly to the tumor area than the larger ones (48). Although the increasing optimization of surface modifications renders these size constrains not easily applicable in biomedical applications (49), it was later demonstrated that tumor vascular normalization through VEGFR2 inhibition improved accumulation of also larger nanoparticles, of 20 and 40 nm size, in the tumoral bed. However, inside the tumor, smaller nanoparticles presented a more homogeneous distribution (50).
Increased tumor vascularity increases nanoparticle delivery, but increased collagen deposition, which also leads to increased interstitial pressure, is an inhibitory factor (51). For this, recent attempts to induce tumor vessel normalization targeted both the tumor microenvironment, as well as the extracellular matrix (ECM). An example is the co-administration of antibodies targeting vascular endothelial growth factor (VEGF) and transforming growth factor β1 (TGF-β1), which led to a combined vascular and ECM normalization and thus improved intratumoral nanomedicine delivery (52).
Gold nanoparticles have been studied for vascular normalization in several tumor types. Endostatin is an endogenous angiogenesis inhibitor. Gold nanoparticle-encapsulated human recombinant endostatin led to a transient tumor vascular normalization in non-small cell lung cancer. Chemotherapy administered during the normalization window was significantly more potent than when administered as a monotherapy (53). Gold nanoparticles have been successfully used to block metastasis in melanoma by increasing tumor vascular normalization (54). Treatment of cediranib, a vascular endothelial growth factor receptor inhibitor, normalized tumor vessels in a breast cancer model, enhancing tumor retention of enzyme responsive size-changeable gold nanoparticles, further demonstrating that combinatorial treatment could be a potent approach for efficient tumor diagnosis and treatment (55).
Epidermal growth factor receptor (EGFR) tyrosine kinase inhibitors, such as erlotinib, are considered effective therapies for EGFR mutation positive non-small cell lung cancers. The promising outcome is often compromised by resistance driven by upregulation of the anti-apoptotic protein survivin, in the cancer cells. A novel approach using chloroquine to normalize the tumor vasculature, combined with anti-EGFR aptamer-mediated delivery of erlotinib and survivin shRNA co-administration significantly hampered tumor growth (56).
Nogo-B is a potent growth factor mediating endothelial cell functions, such as wound healing angiogenesis and chemotaxis, through binding to the Nogo-B receptor (57). Nogo-B receptor knockdown was achieved through nanoparticles with charge convention in the acidic tumor microenvironment, leading to breast cancer vessel normalization in vivo and inhibition of metastatic incidence (58).
Cyclooxygenase-2 (COX-2) is upregulated in several cancer-related pathways regulating cell proliferation, apoptosis, multi-drug resistance and angiogenesis (59). Celecoxib, a clinically-relevant COX-2 inhibitor, was reported to normalize the tumor microenvironment, including the tumor vessels, thus improving the uptake of paclitaxel-loaded micelles in xenografts of human lung adenocarcinomas (60).
Brain vascular normalization and blood-brain barrier restoration are important for glioblastoma. Liposomal formulation of the chemotherapeutic drugs irinotecan, doxorubicin and vincristine improved their pharmacokinetic profile and increased their potency in tumor inhibition. Apart from the size, mostly irinotecan- treated tumors led to vascular normalization, characterized by increased perfusion, assessed by Hoechst uptake, decreased extend of the discontinuous basement membrane, increased number of pericyte-covered capillaries and decreased vessel diameter (61).
miRNAs play a major role in tumor aggressiveness and metastasis. miRNA-200 was initially reported to block epithelial-mesenchymal transition (EMT) in tumors through ZEB1 and ZEB2 downregulation (62–64). miRNA-200 blocks tumor angiogenesis through IL-8 and CXCL1 inhibition. Nanoparticle-mediated miRNA-200 delivery reduced tumor angiogenesis and induced tumor vessel normalization, leading to tumor growth and metastasis inhibition in ovarian, lung, renal and breast adenocarcinomas (65).
Nanoparticle-based approaches are used not only for the delivery of vascular normalizing agents, but also for their development and evaluation. An example is NGR-TNF, a chimeric protein that couples the tumor homing peptide CNGRCG, which targets aminopeptidase N or myeloid plasma membrane glycoprotein CD13, also expressed in angiogenic vessels, with the N-terminus of the tumor necrosis factor-α (TNF). It is a vascular targeting agent, which presents antitumor effects and is in clinical trials for tumors either as monotherapy or in combination with chemotherapeutic drugs. Low dose treatment inhibited angiogenesis by inducing endothelial cell apoptosis, whereas at the later stages it led to tumor vascular normalization, assessed by the increased pericyte and smooth muscle cell coverage. The CD31 targeting was verified in vivo by coupling of the CNGRCG peptide to fluorescent nanoparticles (quantum dots, described above) (66). The studies are summarized in Table 1.
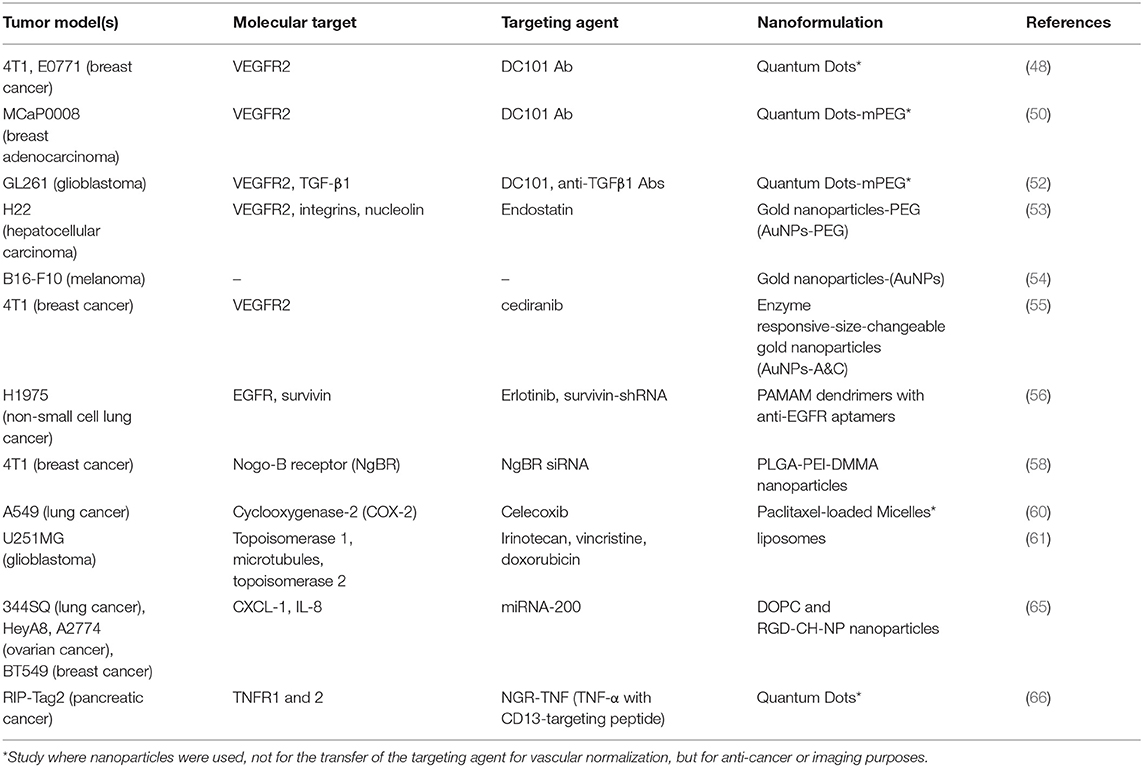
Table 1. Table summarizing the data regarding vascular normalization, including tumor models, molecular targets, targeting agents, and nanoformulations.
Discussion
It is important to note that tumor vessel normalization does not automatically correspond to better distribution of all nanodelivery systems. Tumor vessel normalization induced by imatinib mesylate limited the distribution of large (~110 nm) but enhanced the distribution of small (~23 nm) nanoparticles in human lung adenocarcinoma. However, the nanoparticle distribution inside the tumors was overall reduced, compared to that of micelles, and micelle-based delivery of paclitaxel significantly improved its potency (67).
For the concept that vessel normalization is significantly affecting the efficiency for drug delivery using nanoformulations, nanotechnology has undoubtedly allowed the delivery, protection and targeting of compounds that other drug formulations (i.e., implants, microparticles, free drug) are incapable of achieving (68). The controversial EPR effect, along with the vessel normalization approaches, only illustrate the potential of new methodologies, such as smaller nanocarriers and active targeting. It is now widely accepted that mild anti-angiogenic therapy leads to tumor vessel normalization and tumor vessel normalization induces the uptake of nanoparticle-based delivery, leading to more potent anti-tumor activity (48, 69). This process is also accompanied by mathematical models simulating the events and predicting the penetrance of drugs into the tumor area (70). There is significant potential for novel compounds treating the vascular endothelium to be actively delivered by nanocarriers to the tumor area, safely, with reduced toxicity and high specificity, while avoiding in vivo degradation (68). It is the authors' opinion that nanotechnology will play a significant role in the development of these therapies in the future. With the existence of several biological barriers for the successful delivery of active molecules and nanocarriers, some of which we described here, the optimal physicochemical parameters of the nanocarriers will need to be carefully considered, with their size and shape being paramount (48, 50). Finally, the combination of surface modification for cellular specificity and the achieved vascular normalization may enhance and prolong nanocarrier presence in the tumor microenvironment for improved pharmacological activity. Overall, nanoparticle-mediated drug delivery targeting both tumor cells and tumor vessels could be a promising approach for efficient anti-cancer therapies.
Author Contributions
GM and CM contributed to the conception of the article, wrote and revised the final manuscript, and agreed on its submission to this journal.
Funding
This work was supported for GM by the College of Pharmacy, University of Louisiana Monroe start-up funding and the National Institutes of Health (NIH) through the National Institute of General Medical Science Grants 5 P20 GM103424-15, 3 P20 GM103424-15S1 and for CM in part by National Institutes of Health Grant (NCI) R15CA231339, Texas Tech University Health Sciences Center (TTUHSC) School of Pharmacy Start-up funds and TTUHSC Office of Research grant. The funders had no role in study design, decision to write and preparation of the manuscript.
Conflict of Interest
The authors declare that the research was conducted in the absence of any commercial or financial relationships that could be construed as a potential conflict of interest.
References
1. Folkman J. Angiogenesis: an organizing principle for drug discovery? Nat Rev Drug Discov. (2007) 6:273–86. doi: 10.1038/nrd2115
2. Carmeliet P, Jain RK. Molecular mechanisms and clinical applications of angiogenesis. Nature. (2011) 473:298–307. doi: 10.1038/nature10144
3. Leite de Oliveira R, Hamm A, Mazzone M. Growing tumor vessels: more than one way to skin a cat - implications for angiogenesis targeted cancer therapies. Mol Aspects Med. (2011) 32:71–87. doi: 10.1016/j.mam.2011.04.001
4. Wong PP, Bodrug N, Hodivala-Dilke KM. Exploring novel methods for modulating tumor blood vessels in cancer treatment. Curr Biol. (2016) 26:R1161–6. doi: 10.1016/j.cub.2016.09.043
5. Giantonio BJ, Catalano PJ, Meropol NJ, O'Dwyer PJ, Mitchell EP, Alberts SR, et al. Bevacizumab in combination with oxaliplatin, fluorouracil, and leucovorin (FOLFOX4) for previously treated metastatic colorectal cancer: results from the Eastern Cooperative Oncology Group Study E3200. J Clin Oncol. (2007) 25:1539–44. doi: 10.1200/JCO.2006.09.6305
6. Aghajanian C, Blank SV, Goff BA, Judson PL, Teneriello MG, Husain A, et al. OCEANS: a randomized, double-blind, placebo-controlled phase III trial of chemotherapy with or without bevacizumab in patients with platinum-sensitive recurrent epithelial ovarian, primary peritoneal, or fallopian tube cancer. J Clin Oncol. (2012) 30:2039–45. doi: 10.1200/JCO.2012.42.0505
7. Motzer RJ, Hutson TE, Cella D, Reeves J, Hawkins R, Guo J, et al. Pazopanib versus sunitinib in metastatic renal-cell carcinoma. N Engl J Med. (2013) 369:722–31. doi: 10.1056/NEJMoa1303989
8. Llovet JM, Ricci S, Mazzaferro V, Hilgard P, Gane E, Blanc JF, et al. Sorafenib in advanced hepatocellular carcinoma. N Engl J Med. (2008) 359:378–90. doi: 10.1056/NEJMoa0708857
9. Vasudev NS, Reynolds AR. Anti-angiogenic therapy for cancer: current progress, unresolved questions and future directions. Angiogenesis. (2014) 17:471–94. doi: 10.1007/s10456-014-9420-y
10. Viallard C, Larrivee B. Tumor angiogenesis and vascular normalization: alternative therapeutic targets. Angiogenesis. (2017) 20:409–26. doi: 10.1007/s10456-017-9562-9
11. Mattheolabakis G, Rigas B, Constantinides PP. Nanodelivery strategies in cancer chemotherapy: biological rationale and pharmaceutical perspectives. Nanomedicine. (2012) 7:1577–90. doi: 10.2217/nnm.12.128
12. Wong HL, Bendayan R, Rauth AM, Li Y, Wu XY. Chemotherapy with anticancer drugs encapsulated in solid lipid nanoparticles. Adv Drug Deliv Rev. (2007) 59:491–504. doi: 10.1016/j.addr.2007.04.008
13. Carmeliet P, Jain RK. Principles and mechanisms of vessel normalization for cancer and other angiogenic diseases. Nat Rev Drug Discov. (2011) 10:417–27. doi: 10.1038/nrd3455
14. Jain RK. Antiangiogenesis strategies revisited: from starving tumors to alleviating hypoxia. Cancer Cell. (2014) 26:605–22. doi: 10.1016/j.ccell.2014.10.006
15. Batchelor TT, Gerstner ER, Emblem KE, Duda DG, Kalpathy-Cramer J, Snuderl M, et al. Improved tumor oxygenation and survival in glioblastoma patients who show increased blood perfusion after cediranib and chemoradiation. Proc Natl Acad Sci USA. (2013) 110:19059–64. doi: 10.1073/pnas.1318022110
16. Jones LW, Viglianti BL, Tashjian JA, Kothadia SM, Keir ST, Freedland SJ, et al. Effect of aerobic exercise on tumor physiology in an animal model of human breast cancer. J Appl Physiol. (1985). (2010) 108:343–8. doi: 10.1152/japplphysiol.00424.2009
17. McCullough DJ, Stabley JN, Siemann DW, Behnke BJ. Modulation of blood flow, hypoxia, and vascular function in orthotopic prostate tumors during exercise. J Natl Cancer Inst. (2014) 106:dju036. doi: 10.1093/jnci/dju036
18. Betof AS, Lascola CD, Weitzel D, Landon C, Scarbrough PM, Devi GR, et al. Modulation of murine breast tumor vascularity, hypoxia and chemotherapeutic response by exercise. J Natl Cancer Inst. (2015) 107:djv040. doi: 10.1093/jnci/djv040
19. Schadler KL, Thomas NJ, Galie PA, Bhang DH, Roby KC, Addai P, et al. Tumor vessel normalization after aerobic exercise enhances chemotherapeutic efficacy. Oncotarget. (2016) 7:65429–40. doi: 10.18632/oncotarget.11748
20. Pan F, Li W, Yang W, Yang XY, Liu S, Li X, et al. Anterior gradient 2 as a supervisory marker for tumor vessel normalization induced by anti-angiogenic treatment. Oncol Lett. (2018) 16:3083–91. doi: 10.3892/ol.2018.8996
21. Du S, Xiong H, Xu C, Lu Y, Yao J. Attempts to strengthen and simplify the tumor vascular normalization strategy using tumor vessel normalization promoting nanomedicines. Biomater Sci. (2019) 7:1147–60. doi: 10.1039/C8BM01350K
22. Ardekani S, Scott HA, Gupta S, Eum S, Yang X, Brunelle AR, et al. Nanoliposomal nitroglycerin exerts potent anti-inflammatory effects. Sci Rep. (2015) 5:16258. doi: 10.1038/srep16258
23. Bertrand N, Wu J, Xu X, Kamaly N, Farokhzad OC. Cancer nanotechnology: the impact of passive and active targeting in the era of modern cancer biology. Adv Drug Deliv Rev. (2014) 66:2–25. doi: 10.1016/j.addr.2013.11.009
24. Blanco E, Shen H, Ferrari M. Principles of nanoparticle design for overcoming biological barriers to drug delivery. Nat Biotechnol. (2015) 33:941–51. doi: 10.1038/nbt.3330
25. Labatut AE, Mattheolabakis G. Non-viral based miR delivery and recent developments. Eur J Pharm Biopharm. (2018) 128:82–90. doi: 10.1016/j.ejpb.2018.04.018
26. Barenholz Y. Doxil(R)–the first FDA-approved nano-drug: lessons learned. J Control Release. (2012) 160:117–34. doi: 10.1016/j.jconrel.2012.03.020
27. Laouini A, Jaafar-Maalej C, Limayem-Blouza I, Sfar S, Charcosset C, Fessi H. Preparation, characterization and applications of liposomes: state of the art. J Colloid Sci Biotechnol. (2012) 1:147–68. doi: 10.1166/jcsb.2012.1020
28. Akbarzadeh A, Rezaei-Sadabady R, Davaran S, Joo SW, Zarghami N, Hanifehpour Y, et al. Liposome: classification, preparation, and applications. Nanoscale Res Lett. (2013) 8:102. doi: 10.1186/1556-276X-8-102
29. Mukherjee S, Ray S, Thakur RS. Solid lipid nanoparticles: a modern formulation approach in drug delivery system. Indian J Pharm Sci. (2009) 71:349–58. doi: 10.4103/0250-474X.57282
30. Cury-Boaventura MF, Gorjao R, de Lima TM, Piva TM, Peres CM, Soriano FG, et al. Toxicity of a soybean oil emulsion on human lymphocytes and neutrophils. J Parenter Enteral Nutr. (2006) 30:115–23. doi: 10.1177/0148607106030002115
31. Li C, Zhang J, Zu YJ, Nie SF, Cao J, Wang Q, et al. Biocompatible and biodegradable nanoparticles for enhancement of anti-cancer activities of phytochemicals. Chin J Nat Med. (2015) 13:641–52. doi: 10.1016/S1875-5364(15)30061-3
32. Bi Y, Hao F, Yan G, Teng L, Lee RJ, Xie J. Actively targeted nanoparticles for drug delivery to Tumor. Curr Drug Metab. (2016) 17:763–82. doi: 10.2174/1389200217666160619191853
33. Pang L, Qin J, Han L, Zhao W, Liang J, Xie Z, et al. Exploiting macrophages as targeted carrier to guide nanoparticles into glioma. Oncotarget. (2016) 7:37081–91. doi: 10.18632/oncotarget.9464
34. Tietjen GT, Hosgood SA, DiRito J, Cui J, Deep D, Song E, et al. Nanoparticle targeting to the endothelium during normothermic machine perfusion of human kidneys. Sci Transl Med. (2017) 9:eaam6764. doi: 10.1126/scitranslmed.aam6764
35. Ng SM, Koneswaran M, Narayanaswamy R. A review on fluorescent inorganic nanoparticles for optical sensing applications. RSC Adv. (2016) 6:21624–61. doi: 10.1039/C5RA24987B
36. Giner-Casares JJ, Henriksen-Lacey M, Coronado-Puchau M, Liz-Marzán LM. Inorganic nanoparticles for biomedicine: where materials scientists meet medical research. Mater Today. (2016) 19:19–28. doi: 10.1016/j.mattod.2015.07.004
37. Singh R, Dumlupinar G, Andersson-Engels S, Melgar S. Emerging applications of upconverting nanoparticles in intestinal infection and colorectal cancer. Int J Nanomed. (2019) 14:1027–38. doi: 10.2147/IJN.S188887
38. Greish K. Enhanced permeability and retention (EPR) effect for anticancer nanomedicine drug targeting. Methods Mol Biol. (2010) 624:25–37. doi: 10.1007/978-1-60761-609-2_3
39. Theek B, Baues M, Ojha T, Mockel D, Veettil SK, Steitz J, et al. Sonoporation enhances liposome accumulation and penetration in tumors with low EPR. J Control Release. (2016) 231:77–85. doi: 10.1016/j.jconrel.2016.02.021
40. Hansen AE, Petersen AL, Henriksen JR, Boerresen B, Rasmussen P, Elema DR, et al. Positron emission tomography based elucidation of the enhanced permeability and retention effect in dogs with cancer using Copper-64 liposomes. ACS Nano. (2015) 9:6985–95. doi: 10.1021/acsnano.5b01324
41. Danhier F. To exploit the tumor microenvironment: since the EPR effect fails in the clinic, what is the future of nanomedicine? J Control Release. (2016) 244(Pt A):108–21. doi: 10.1016/j.jconrel.2016.11.015
42. Poole RM. Pembrolizumab: first global approval. Drugs. (2014) 74:1973–81. doi: 10.1007/s40265-014-0314-5
43. Mazza C, Escudier B, Albiges L. Nivolumab in renal cell carcinoma: latest evidence and clinical potential. Ther Adv Med Oncol. (2017) 9:171–81. doi: 10.1177/1758834016679942
44. Gao X, McDermott DF. Ipilimumab in combination with nivolumab for the treatment of renal cell carcinoma. Expert Opin Biol Ther. (2018) 18:947–57. doi: 10.1080/14712598.2018.1513485
45. Wolchok JD, Kluger H, Callahan MK, Postow MA, Rizvi NA, Lesokhin AM, et al. Nivolumab plus ipilimumab in advanced melanoma. N Engl J Med. (2013) 369:122–33. doi: 10.1056/NEJMoa1302369
46. Nishino M, Ramaiya NH, Hatabu H, Hodi FS. Monitoring immune-checkpoint blockade: response evaluation and biomarker development. Nat Rev Clin Oncol. (2017) 14:655–68. doi: 10.1038/nrclinonc.2017.88
47. Fukumura D, Kloepper J, Amoozgar Z, Duda DG, Jain RK. Enhancing cancer immunotherapy using antiangiogenics: opportunities and challenges. Nat Rev Clin Oncol. (2018) 15:325–40. doi: 10.1038/nrclinonc.2018.29
48. Chauhan VP, Stylianopoulos T, Martin JD, Popovic Z, Chen O, Kamoun WS, et al. Normalization of tumour blood vessels improves the delivery of nanomedicines in a size-dependent manner. Nat Nanotechnol. (2012) 7:383–8. doi: 10.1038/nnano.2012.45
49. Peer D, Karp JM, Hong S, Farokhzad OC, Margalit R, Langer R. Nanocarriers as an emerging platform for cancer therapy. Nat Nanotechnol. (2007) 2:751–60. doi: 10.1038/nnano.2007.387
50. Jiang W, Huang Y, An Y, Kim BY. Remodeling tumor vasculature to enhance delivery of intermediate-sized nanoparticles. ACS Nano. (2015) 9:8689–96. doi: 10.1021/acsnano.5b02028
51. Torosean S, Flynn B, Axelsson J, Gunn J, Samkoe KS, Hasan T, et al. Nanoparticle uptake in tumors is mediated by the interplay of vascular and collagen density with interstitial pressure. Nanomedicine. (2013) 9:151–8. doi: 10.1016/j.nano.2012.07.002
52. Chen Y, Liu X, Yuan H, Yang Z, von Roemeling CA, Qie Y, et al. Therapeutic remodeling of the tumor microenvironment enhances nanoparticle delivery. Adv Sci. (2019) 6:1802070. doi: 10.1002/advs.201802070
53. Li W, Zhao X, Du B, Li X, Liu S, Yang XY, et al. Gold nanoparticle-mediated targeted delivery of recombinant human endostatin normalizes tumour vasculature and improves cancer therapy. Sci Rep. (2016) 6:30619. doi: 10.1038/srep30619
54. Li W, Li X, Liu S, Yang W, Pan F, Yang XY, et al. Gold nanoparticles attenuate metastasis by tumor vasculature normalization and epithelial-mesenchymal transition inhibition. Int J Nanomed. (2017) 12:3509–20. doi: 10.2147/IJN.S128802
55. Xiao W, Ruan S, Yu W, Wang R, Hu C, Liu R, et al. Normalizing tumor vessels to increase the enzyme-induced retention and targeting of gold nanoparticle for breast cancer imaging and treatment. Mol Pharm. (2017) 14:3489–98. doi: 10.1021/acs.molpharmaceut.7b00475
56. Lv T, Li Z, Xu L, Zhang Y, Chen H, Gao Y. Chloroquine in combination with aptamer-modified nanocomplexes for tumor vessel normalization and efficient erlotinib/Survivin shRNA co-delivery to overcome drug resistance in EGFR-mutated non-small cell lung cancer. Acta Biomater. (2018) 76:257–74. doi: 10.1016/j.actbio.2018.06.034
57. Miao RQ, Gao Y, Harrison KD, Prendergast J, Acevedo LM, Yu J, et al. Identification of a receptor necessary for Nogo-B stimulated chemotaxis and morphogenesis of endothelial cells. Proc Natl Acad Sci USA. (2006) 103:10997–1002. doi: 10.1073/pnas.0602427103
58. Wang B, Ding Y, Zhao X, Han X, Yang N, Zhang Y, et al. Delivery of small interfering RNA against Nogo-B receptor via tumor-acidity responsive nanoparticles for tumor vessel normalization and metastasis suppression. Biomaterials. (2018) 175:110–22. doi: 10.1016/j.biomaterials.2018.05.034
59. Vosooghi M, Amini M. The discovery and development of cyclooxygenase-2 inhibitors as potential anticancer therapies. Expert Opin Drug Discov. (2014) 9:255–67. doi: 10.1517/17460441.2014.883377
60. Zhang B, Jin K, Jiang T, Wang L, Shen S, Luo Z, et al. Celecoxib normalizes the tumor microenvironment and enhances small nanotherapeutics delivery to A549 tumors in nude mice. Sci Rep. (2017) 7:10071. doi: 10.1038/s41598-017-09520-7
61. Verreault M, Strutt D, Masin D, Anantha M, Yung A, Kozlowski P, et al. Vascular normalization in orthotopic glioblastoma following intravenous treatment with lipid-based nanoparticulate formulations of irinotecan (Irinophore C), doxorubicin (Caelyx(R)) or vincristine. BMC Cancer. (2011) 11:124. doi: 10.1186/1471-2407-11-124
62. Korpal M, Lee ES, Hu G, Kang Y. The miR-200 family inhibits epithelial-mesenchymal transition and cancer cell migration by direct targeting of E-cadherin transcriptional repressors ZEB1 and ZEB2. J Biol Chem. (2008) 283:14910–4. doi: 10.1074/jbc.C800074200
63. Park SM, Gaur AB, Lengyel E, Peter ME. The miR-200 family determines the epithelial phenotype of cancer cells by targeting the E-cadherin repressors ZEB1 and ZEB2. Genes Dev. (2008) 22:894–907. doi: 10.1101/gad.1640608
64. Gregory PA, Bert AG, Paterson EL, Barry SC, Tsykin A, Farshid G, et al. The miR-200 family and miR-205 regulate epithelial to mesenchymal transition by targeting ZEB1 and SIP1. Nat Cell Biol. (2008) 10:593–601. doi: 10.1038/ncb1722
65. Pecot CV, Rupaimoole R, Yang D, Akbani R, Ivan C, Lu C, et al. Tumour angiogenesis regulation by the miR-200 family. Nat Commun. (2013) 4:2427. doi: 10.1038/ncomms3427
66. Porcellini S, Asperti C, Valentinis B, Tiziano E, Mangia P, Bordignon C, et al. The tumor vessel targeting agent NGR-TNF controls the different stages of the tumorigenic process in transgenic mice by distinct mechanisms. Oncoimmunology. (2015) 4:e1041700. doi: 10.1080/2162402X.2015.1041700
67. Zhang B, Shi W, Jiang T, Wang L, Mei H, Lu H, et al. Optimization of the tumor microenvironment and nanomedicine properties simultaneously to improve tumor therapy. Oncotarget. (2016) 7:62607–18. doi: 10.18632/oncotarget.11546
68. Phillips MA, Gran ML, Peppas NA. Targeted nanodelivery of drugs and diagnostics. Nano Today. (2010) 5:143–59. doi: 10.1016/j.nantod.2010.03.003
69. Jain RK, Tong RT, Munn LL. Effect of vascular normalization by antiangiogenic therapy on interstitial hypertension, peritumor edema, and lymphatic metastasis: insights from a mathematical model. Cancer Res. (2007) 67:2729–35. doi: 10.1158/0008-5472.CAN-06-4102
Keywords: nanoparticles, delivery, tumor, vessel, normalization
Citation: Mattheolabakis G and Mikelis CM (2019) Nanoparticle Delivery and Tumor Vascular Normalization: The Chicken or The Egg? Front. Oncol. 9:1227. doi: 10.3389/fonc.2019.01227
Received: 19 August 2019; Accepted: 28 October 2019;
Published: 12 November 2019.
Edited by:
Erika Ruiz-Garcia, National Institute of Cancerology (INCan), MexicoReviewed by:
Mikhail Durymanov, Moscow Institute of Physics and Technology, RussiaKatarzyna A. Rejniak, Moffitt Cancer Center, United States
Copyright © 2019 Mattheolabakis and Mikelis. This is an open-access article distributed under the terms of the Creative Commons Attribution License (CC BY). The use, distribution or reproduction in other forums is permitted, provided the original author(s) and the copyright owner(s) are credited and that the original publication in this journal is cited, in accordance with accepted academic practice. No use, distribution or reproduction is permitted which does not comply with these terms.
*Correspondence: George Mattheolabakis, bWF0dGhhaW9sYW1wYWtpc0B1bG0uZWR1; Constantinos M. Mikelis, Y29uc3RhbnRpbm9zLm1pa2VsaXNAdHR1aHNjLmVkdQ==