- N.N. Blokhin National Medical Research Center of Oncology, Moscow, Russia
A large number of studies have presented a great deal of information about tumor and immune system interaction. Nevertheless, the problem of tumor evasion from the immune reaction is still difficult to resolve. Understanding the ways in which immunosuppressive tumor microenvironment develops and maintains its potential is of utmost importance to ensure the best use of the suppressed immune functions. The study presents a review covering the data on tumor-associated antigens, mechanisms of tumor evasion from the immune reactions, and search for common immunosuppressive processes of tumor growth and normal wound healing. The study discusses the important role of monocytes/macrophages in the regulation of immune system reactions. We suggest that the simultaneous actions of growth factors and pro-inflammatory cytokines may result in the suppression of the immune system. The study describes intracellular signaling molecules that take part in the regulation of the myeloid cell functions. If the hypothesis is proved correct, the indicated interaction of cytokines could be regarded as a prospective target for antitumor therapy.
Introduction
The immune system can recognize malignantly transformed cells due to the antigens that differentiate a tumor cell from the normal one. Inflammation in the tumor microenvironment causes an accumulation of immune cells at the site. Therefore, the tumor has some mechanisms of immune suppression in the microenvironment to evade immune surveillance. Besides, an obscure inflammatory phenomenon associated with immunosuppression has been observed. Immunosuppression requires no new mechanisms of action in the tumor microenvironment, but it boosts existing normal regulatory mechanisms, such as those that participate in inflammation resolution, wound healing, etc. Better understanding of these mechanisms is crucially important. The paper provides evidence that the concurrent presence of pro-inflammatory cytokines and growth factors affecting monocytes/macrophages in the tumor microenvironment may act as such a regulatory mechanism. This combination of cytokines and growth factors can have a significant immunosuppressive effect.
Mechanisms of the Immunological Recognition of Tumors
Immune cells can act against tumors in different ways, such as by absorbing and presenting tumor antigens, releasing cytokines that activate and recruit other immune cells, or directly killing tumor cells. This section describes the most well-studied tumor antigens that distinguish a tumor cell from a normal one, which help the immune system eventually eliminate the tumor. Some immune mechanisms, such as phagocytosis, involve the recognition and elimination of apoptotic and stressed cells. Many cell types have a special function of efferocytosis, i.e., elimination of apoptotic cells. They include both professional (macrophages and immature dendritic cells) and non-professional phagocytes (fibroblasts and epithelial cells).
Phosphatidylserines
Phosphatidylserines are phospholipid components located on the inner (cytosolic) cell membranes. In apoptotic cells, phosphatidylserines come out on the cell surface. As a result, phagocytes receive the signal for the absorption of the apoptotic cells. Phosphatidylserine can be recognized by a number of receptors (1, 2). Some studies showed that tumor cells may have an increased level of surface phosphatidylserines (3).
Calreticulin
Another pro-phagocyte signal is calreticulin expressed on the cell surface. Normally, calreticulin is located in endoplasmic/sarcoplasmic reticulum (4), in the cell nucleus (5), and partly on the surface membrane (6). Cellular stress induces its surface expression. In this case, calreticulin acts as a pro-phagocyte signal binding to CD91 receptor on phagocytes, which leads to the absorption of the target cell. Normal cells with a low level of surface calreticulin are not destroyed because they send anti-phagocytic signals with their surface CD47 (7). Certain cancers present super-expression of surface calreticulin, but most normal cells have low calreticulin levels. Enhanced CD47 expression correlates with high calreticulin expression, and that is necessary to avoid calreticulin mediated phagocytosis (8–10).
Heat Shock Proteins (HSP) and NK-cells
Unlike normal cells, tumor ones have an elevated expression of heat shock proteins (HSP). These proteins play a different role in the intracellular or extracellular settings. On the one hand, intracellular HSPs defend tumor cells from the stressful impact of the microenvironment (11, 12), which becomes a problem for the antitumor therapy. On the other hand, membrane and extracellular Hsp70 have a stimulating immune effect (13–15). Some HSPs can bind intracellular antigen peptides. Such peptide complex may come out on the cell surface as a result of cell lysis and other processes. APCs have surface receptors that capture the complex and engulf it. APCs can incorporate antigens linked to HSPs and on activation present these antigens to CD8+ T-cells, thus promoting cytotoxic lymphocyte activity (16, 17). Moreover, surface Hsp70 mediates cytotoxic NK functions. Surface Hsp70 was found on plasmatic membranes in different tumor cell cultures (18) and tumors of cancer patients (19), while normal tissues had no Hsp70 (20). Cytokine activated NKs recognize and lyse tumor cells with surface Hsp70 (21, 22). CD94 receptor on the NK probably participates in the Hsp70 recognition. Tumor cell surface HLA-E serves as an inhibiting signal, whereas Hsp70 is an activating signal for different complexes of CD94/NKG2D receptors on the NKs (23, 24).
B7-H6 and NK-cells
Tumor cell surface B7-H6 is a ligand for NKp30 activating receptor on the NKs (25). Interaction of B7-H6 and NKp30 induces cytotoxic functions of IL-2 activated NKs (26, 27). Generally, B7-H6 protein has not been found in normal tissues (26), though it is expressed on the CD14+/CD16+ pro-inflammatory monocytes in sepsis (28). However, some studies occasionally detected B7-H6 by immunohistochemistry in normal tissues and showed no essential differences in B7-H6 expression between a tumor and normal tissue (29, 30). Other authors showed elevated surface B7-H6 in breast (31) and ovarian cancers (32), melanoma (33), and glioma (34), while normal tissues were negative of this parameter (34). Therefore, it seems that surface B7-H6 rate may vary with the tumor type. Some authors noted that higher expression of both surface and soluble B7-H6 in ovarian cancer was associated with the down regulation of the NK function (35). This fact may partly explain the immune system failure to recognize tumor cells with overexpressed B7-H6.
MIC A/B, NK and T-cells
Many studies indicate NKG2D as an activating receptor that helps the immune system to distinguish tumor from normal cells. Homodimer NKG2D is expressed on all NKs as well as CD8+ αβ, γδ T-cells, and some NKT-cells (36–38). NKG2D receptor can recognize highly polymorphic stress-induced molecules MICA and MICB (major histocompatibility complex class I chain-related protein A or B) related to MHC I (39). MICA/B proteins are absent on the normal cells or a minor number of them is found on the intestinal epithelial cells (40). However, these proteins are often expressed in patients with cancer (41), such as lung carcinoma, renal, prostate, ovarian, and colon cancer (42), hepatocellular carcinoma (43), melanoma (44), and leukemia (45). MICA/B expression increased in non-tumor cell lines in various stress conditions including DNA damage (46) and viral infection (47). Moreover, NKG2D receptor can recognize other proteins expressed on the stressed cells, such as ULBP (UL16-binding proteins) (48). T-cell activation requires firstly, the signal from T-cell receptor, secondly, the co-stimulating factor CD28, substituted by NKG2D in some cases (47). MICA or MICB ligand interaction with NKG2D is a potent activating signal for NKs that can result in NK recognizing and lysing the target cell (36, 49). However, the decision of NK killing a tumor cell will be made based on the summarized effects of the activating and inhibiting receptors (50, 51).
Besides direct cytotoxicity, NK-cells can stimulate T-cell response by inducing dendritic cell maturation (52, 53). Pre-activated NKT-cells also induced DC maturation in some experimental models (54). These mechanisms facilitate the adaptive immune system to fight against the tumor. On the whole, to activate the adaptive immune system, APC should recognize the tumor and the tumor antigens should be presented to the adaptive immune cells. DCs are considered the most important APCs. DC maturation is mediated by certain cytokines produced by NK and other cells after tumor recognition and stimulated by DAMP (Damage-associated molecular patterns) released in stress and cell death. However, DAMP functions are ambiguous since they can have an antitumor effect on the one hand, and may boost tumor development on the other hand (55).
Cancer-Testis Antigens, T-Cells
Although cancer-testis (CT) antigen expression in normal tissues of the adults is restricted to the male germ cells, CT spontaneous expression can be registered in tumor cells (56). Male germ cells lack HLA-I molecules (57); they are located at the immune privileged sites and cannot present antigens to T-cells. CT antigen expression was detected in the thymic epithelial cells that are responsible for negative selection of autoreactive T-cells (58). Nevertheless, patients with cancer often develop immune reactions to CT antigens (59), which involve both cellular and humoral responses. At present, the number of CT antigens includes over 200 protein families (60). The cancer testis database presents a lot of studies that have demonstrated immune response to these proteins with NY-ESO-1 being the most immunogenic one (60). Correlation of low functional activity of T-cells recognizing PRAME and an enhanced number of immune suppressive cells was observed in CML (61), which may explain inefficient immune response and tumor progression.
Mutant Proteins (Neoantigens), T-Cells
Many mutations occur in the tumor as a result of its genetic instability (62, 63). Recent studies have shown that tumor antigens appearing after the mutations of normal genes are highly immunogenic. Quite a few examples demonstrate T-cell recognition of mutant proteins presented in the HLA-I context (64). On the one hand, mutations increase tumor immunogenicity, while on the other hand, they are involved in different pathways, including immunosuppression, that contribute to tumor evasion from the immune surveillance. The situation changes when immunosuppression declines due to PD-1 or CTLA-4 blockade. The studies showed that a higher mutation load of the tumor was associated with higher sensitivity to the PD-1 blocker therapy in the studied cancer types (65–68). The findings imply that tumors with a larger number of mutations were more immunogenic. The murine sarcoma model showed that mostly mutant neoantigens were responsible for recognizing the tumor during anti- PD-1 and CTLA-4 treatment (69). Personalized vaccines that induce immune response to the mutant tumor neoantigens demonstrated an effective clinical outcome though the trials involved a small number of vaccinated patients (70, 71). Therefore, the tumor has a large number of antigens to be recognized and destroyed by the immune system.
The above discussed information refers to the established tumors. However, some authors studied the immune surveillance of pre-malignant cells. Kang et al. introduced a genetic construction into the livers of mice, which activated Nras oncogene. Normal hepatocytes with the genetic construction entered the cellular senescence program, which prevented the tumor growth. The livers of these mice with senescent hepatocytes were infiltrated by immune cells, expressed pro-inflammatory cytokines, and therefore had decreased numbers of Nras-positive cells. As a result, normal mice did not develop any tumors. However, if monocytes/macrophages or CD4+T-cells, but not others, were removed, these mice developed hepatocellular carcinoma (HCC) (72). This study demonstrated the immune surveillance even at the stage of pre-malignant cells.
Mechanisms of Immunosuppression in the Tumor Microenvironment
Despite the presence of tumor-associated antigens the immune system destroys the established tumors very rarely. Tumor microenvironment includes immunosuppressive factors as well. We assume that it is the immunosuppression which contributes most of all to the tumor evasion from the immune reactions. Mechanisms of immunosuppression in the tumor microenvironment have been studied in detail in many profound reviews (73, 74). This section presents some of those mechanisms. Part of them is generated by the tumor cells, while other mechanisms are triggered by the recruited normal cells of the tumor microenvironment. The section does not classify the mechanisms with regards to their origin. We have found just a vague design of immunosuppressive mechanisms hierarchy and classification. Therefore, firstly, we describe them in general and in the following section we will suggest a structure of the immunosuppressive cells' hierarchy.
Surface Expression of Ligands for Immune Cell Inhibitor Receptors
As shown above, tumor cells express CD47 to defend from phagocytosis induced by calreticulin (8). Surface PD-L1 expression is frequently detected on tumor cells or on the cells of the tumor microenvironment. Binding to its PD-1 receptor PD-L1 molecule can inhibit T-cell activation (75). Besides PD-1, T-cells have other inhibiting receptors, such as LAG-3, which ligands may be expressed in tumors (76). Another inhibitor CTLA-4 is mainly expressed on regulatory T-cells (Treg) and on the activated conventional T- cells (Tconv). CTLA-4 blockers may enhance anti-tumor T-cell activity. The studies showed that CTLA-4 can reduce the number of CD80 and CD86 ligands on APCs by transendocytosis leading to the inability of APCs to activate T-cells (77, 78).
Immunosuppressive Cells
It is now well known that quite a few of the immune cell populations can have suppressive functions and are found in the tumor microenvironment. The most substantially studied lymphoid cells are Tregs and NKT-cells type II. Tumor-associated macrophages (TAM) of M2 type and myeloid-derived suppressor cells (MDSC) are the most studied myeloid cells. Immature dendritic cells that cannot present tumor antigens have a significant impact on tumor evasion from the immune surveillance.
Secretion of Soluble Immunosuppressive Factors
Tumor microenvironment accumulates an increased number of immunosuppressive cytokines such as TGF-β (79) and IL-10 (80). Multifunctional factors PGE2 (81), IL-6 (82), and others exert their immunosuppressive functions in the settings of tumor microenvironment. Besides, extracellular adenosine accumulates there and binds to its receptors on the immune cells, which fosters suppressor activity of the immune cells (83). Lactate presence in tumor microenvironment can stimulate immunosuppression, as well (84).
Most tumors express at least one type of NKG2D ligands and therefore they must be sensitive to NKG2D-dependent immune response. However, soluble forms of NKG2D ligands shed from the tumor cell surface and thus facilitate tumor evasion from the immune surveillance. The serum amount of soluble NKG2D ligands correlates with tumor progression in some cancer types (85).
Exhausting the Nutrients in Tumor Microenvironment
TAM and MDSC produce arginase-1 enzyme resulting in exhausted arginine in the microenvironment. L-arginine is an amino acid needed for T-cell proliferation and ζ-chain synthesis of the T-cell receptor (TCR). Arginase-1 destroys arginine, causes TCR ζ-chain impairment, and eventually blocks activation and proliferation of T-cells (86). MDSC can exhaust L-cysteine by consumption and engulfment. This amino acid is important for T-cell activation. It is present in the form of cystine in the microenvironment. Although T-cells cannot absorb cystine, they depend on cysteine, which is produced mainly by mature dendritic cells and macrophages when they present antigens. These cells absorb cystine, split it to cysteine, and partially transfer it to T-cells. MDSCs absorb cystine but do not transfer it to T-cells (87). Tumor cells, DCs, macrophages, and MDSCs can produce immunosuppressive intracellular enzyme indolamin-2,3-dioxygenase (IDO). IDO inhibiting effect on T-cells is associated with the depletion of the essential amino acid tryptophan and formation of suppressive tryptophan metabolites as a result of this process (88). Therefore, antitumor immune responses can be inhibited by a number of mechanisms.
Common Mechanisms for Wound Healing and Tumor Microenvironment
It has already been shown that the malignant process has some similar features with wound healing (89–91). It is therefore reasonable to search for and discuss their common mechanisms. Wound healing is a complex process that is divided into several phases. They have 3 major stages: inflammation, proliferation, and tissue remodeling. It should be noted that the definition refers mainly to skin wound healing because they were studied most intensively. To summarize the features of this process, we will use the term trauma healing.
1. Once the trauma has occurred, constriction of the blood vessels and platelet aggregation develop in order to stop bleeding. Then different inflammation related cells are recruited to the site: neutrophils are recruited at the early phase and monocyte/macrophages appear at the later phase. Inflammation reaction is triggered by various cytokines and chemokines, as well as DAMP and PAMP. Inflammatory phase is characterized by hemostasis that prevents further damage and closes the wound. The phase also includes chemotaxis and enhanced vascular permeability that helps cell migration to eliminate cellular debris and bacteria.
2. Proliferation phase develops when the wound defect is filled with granulation tissue. Fibroblasts proliferate and produce new collagens and glycosaminoglycans that promote wound stabilization. Consequently new blood vessels develop and, finally, wound edges are sealed by an immature scar.
3. Maturation phase develops when the damaged site is restored; it reaches its maximum strength and the scar is formed. If it is a skin wound, epithelization develops and the wound edges are pulled together (92, 93).
Further we will discuss some mechanisms of immunosuppression which occur at the trauma site and during tumorigenesis. However, these mechanisms are not necessarily the same in all types of wound healing.
The studies showed that mRNA-related PD-L1 expression was high in normal human organs including heart, skeletal muscles, placenta, and lungs (94). However, protein-related PD-L1 expression was not observed in healthy subjects (95), or it was low and increased with inflammation (96, 97). For instance, the studies of experimental skin inflammation showed PD-L1 expression on some cells of microvessels and keranocytes though they were not detected in healthy skin (98).
Purinergic regulation is involved in the resolution of inflammation. This system is rather complex requiring counter-regulatory mechanisms. We will describe it in a simple schematic way and it may be found in the referred review in detail (99). Normally, ATP molecules are located intracellularly and just a small number is found in the extracellular matrix. ATP is rapidly released into the extracellular matrix in case of cellular stress or cell damage. ATP has chemotactic and stimulating effect on immune cells when its high concentration accumulates in the extracellular matrix. Enzymes split ATP on the immune cell membranes to continue the proliferation phase. CD39 molecules can split ATP and ADP down to AMP. CD73 can split AMP to immunosuppressive adenosine. Adenosine binds to its receptors on a great number of immune cells and has an anti-inflammatory effect.
Lactate accumulates in wounds in some cases (100, 101). However, the data about its function are ambiguous: on the one hand, experimental addition of lactate improved wound healing (102, 103), on the other hand, high lactate concentrations have a negative effect on fibroblast and endothelial cell viability (101).
Some authors suggest that the major Treg function is the defense against autoimmune reactions. Besides, other experimental studies showed that activated Foxp3+Tregs accumulated in the skin wound site and improved its healing (104). Similar effects were observed in healing of some other organs (105).
Many authors consider that macrophages play a significant role in the wound-related processes (106, 107). Back in the 1970s it was found that macrophage depletion significantly delayed wound healing in animals (108). Similar results were obtained in the studies on genetically modified mice where it was possible to achieve specific depletion of macrophages in wounds (109, 110). The authors found that macrophage depletion was especially critical at the inflammatory or proliferation phases (110). At the early stage of wound healing infiltrating monocytes and residential macrophages are affected by pro-inflammatory cytokines, interferons, PAMP, or DAMP; they become activated and acquire mainly pro-inflammatory phenotype M1. They eliminate microorganisms by phagocytosis, remove dead cells and cellular debris and produce pro-inflammatory mediators and chemokines for additional recruitment of leukocytes. Later, macrophages shift from pro-inflammatory M1 phenotype to reparative M2 type in the healing process, and express anti-inflammatory mediators and growth factors facilitating fibroblast proliferation and angiogenesis. M1–M2 transition is of ultimate importance for inflammation resolution and shifting balance to tissue regeneration (111). It is worth looking in more detail at the mechanisms which macrophages use to make final decision of polarization to phenotype M2. So far, a number of such mechanisms have been studied (111, 112). We would like to identify potential polarization mechanisms at the trauma site. And most likely, this is not just a decrease of inflammatory mediators, RAMP and DAMP in the microenvironment, but the presence of active counter-regulatory mechanisms. It should be noted that IL-4 and IL-13 cytokines, considered necessary for alternative macrophage activation in the in vitro experiments, were not found in the wound microenvironment in mice in vivo (113). There is a mechanism associated with the elimination of apoptotic neutrophils. At an early inflammatory stage, many neutrophils are found in the wound microenvironment, which help wound cleaning. However, if they persist for long, they may damage surrounding tissues (114). Macrophages induce apoptosis in neutrophils to eliminate them from the wound (115). Afterwards, macrophages remove apoptotic neutrophils by phagocytosis (116). Interestingly, phagocytosis of neutrophils is important for macrophages polarization from pro-inflammatory M1 phenotype to reparative M2 (117, 118). However, according to the most recent data, not all neutrophils die via apoptosis at the trauma site, but many of them return to the vascular system (119). Grinberg et al. discovered a counter-regulating mechanism of restricting inflammation that functions with Toll-like receptors. Toll-like receptor (TLR) 4 ligands and adenosine A (2A) ligands switched macrophages from inflammatory M1 to angiogenic M2-like phenotype (120). Immune complexes with LPS or IL-1 mediate M2 polarization, as well (121). This may imply another type of a counter-regulating mechanism.
Though some authors noted that lactate can shift macrophage polarization to M2 in tumor microenvironment (84), we consider that the mentioned mechanism may play only a supplementary role in case of wound healing because of ambivalent lactate features. Many studies showed that PGE2 can shift macrophage phenotype to M2 (122). It is well known that PGE2 has pro-inflammatory function (at the early stages of inflammation), as well as anti-inflammatory activity (at the final stages when PGE2 mediates wound healing) (123). In this regard, there are doubts that PGE2 is an independent factor affecting macrophage polarization. Perhaps its functions are associated with other mediators currently present in the microenvironment. Therefore, it may be assumed that the transition from inflammation to proliferation requires counter-regulatory mechanisms.
Besides macrophages in the trauma site, an increased number of CD14+/HLA-DRlow/− monocytes were registered in the peripheral blood (124, 125). A similar increase of these cells was found in case of malignant process (126–129). The reports show that such monocytes of cancer patients have immunosuppressive functions and are referred to as MDSC (126, 127). They are less studied in case of trauma; though some data indicate that the increase in these cell numbers is associated with the risk of secondary infections (130). MDSCs were found in the trauma site in the mice studies (131). Another report showed that MDSCs supported trauma healing (132). It is highly likely that M2 macrophages and MDSCs are the same cells of different status with similar functions since MDSC in tumor microenvironment can differentiate into TAM (133). Moreover, the studies on murine models showed that monocytes accumulated in the trauma site and could present either pro-inflammatory or anti-inflammatory functions similar to those of M1/M2 macrophages (134–136). Therefore, it is not always possible to distinguish these cells, and this paper will regard monocytes, macrophages, immature DC, and monocyte-derived MDSC as a single system of myeloid cells. There is a term of mononuclear phagocytic system, but this paper will regard them as monocytes/macrophages.
When comparing wound healing with the tumor process, there arise some issues. For instance, why similar mechanisms lead to inflammation resolution in injury, but do not stop inflammation in tumors. And there are certain differences between a malignant process and inflammation caused by chronic infections (137). A vivid comparison was made for the tumor as a “non-healing wound” (89). Another definition may be “continuous immunosuppressive inflammation.” The condition looks like a frozen process at some transitional stage between inflammation and proliferation. Studying the role of stem cells in trauma healing will help better understanding of this phenomenon. Probably, the interaction between myeloid and stem cells has common characteristics with the “seed and soil” hypothesis of metastases formation (138).
Wound healing involves such important stem cells as mesenchymal stem cells (MSC), hematopoietic stem cells (HSC), adipose tissue stem cells (ADSC), and endothelial progenitor cells (EPC) (139). We will use the term “stem cell” to describe their common features or indicate a certain cell type where appropriate. It is well known that stem cells can migrate to the trauma site (139, 140). Stem cells probably can improve wound healing by two major mechanisms–by secreting mediators necessary for healing (as a result of the release of inflammatory mediators together with the key cytokines and growth factors) and by differentiation into the cell types necessary for the wound closure. However, mechanisms of stem cell action in the wound healing have not been characterized in detail, yet.
Pathologic inflammatory reaction to the trauma can disrupt stem cell functions. For instance, polymorphonuclear cells recruited to the site of injury caused necrosis of endothelial precursor cells (EPC), possibly, as a result of reactive oxygen species action (141). Therefore, it is more probable that stem cell functions of tissue reparation are realized mainly after inflammatory phase and thus, stem cells should be able to control inflammation independently. It is already well known that MSCs have immunosuppressive functions (142, 143).
Some reports demonstrate that inflammatory cytokines induce MSC immunoregulatory functions (144–146). In fact, such microenvironment is observed at the inflammatory phase of wound healing. Pro-inflammatory cytokines, toxins of infectious agents and hypoxia can stimulate MSCs to produce growth factors including epidermal growth factor (EGF), fibroblast growth factor (FGF), platelet growth factor (PDGF), transforming growth factor β (TGF-β), vascular endothelial growth factor (VEGF), hepatocyte growth factor (HGF), insulin-like growth factor-1 (IGF-1), angiopoietin-1 (Ang-1), keratinocyte growth factor (KGF), and stromal cell factor-1 (SDF-1). These growth factors consequently promote development of fibroblasts, endothelial cells, and tissue precursor cells that build up tissue regeneration and restoration (147).
Some interesting specific features of the interaction between stem and immune cells, particularly myeloid ones, are worth mentioning. Numerous experiments showed that MSCs regulate macrophage and DC functions by soluble mediators; although intercellular contacts play an important role as well (148, 149). For instance, MSCs inhibit macrophage phenotype polarization to M1 type in the animal model of sepsis (150); similar results of macrophage polarization were obtained on the rat model of trauma (151). MSCs also inhibit DC maturation (152, 153). M2 macrophages and immature DCs are usually found in the tumor microenvironment. The papers present several descriptions of mechanisms of suppressive MSC effect on myeloid cells. For example, MSCs produce PGE2 (122, 154) and interleukine-1 receptor antagonist (IL1RA) (155).
The interaction between pro-inflammatory cytokines and growth factors that may simultaneously present at the wound site during the transition process from inflammation to proliferation, which, in fact, has been poorly studied so far, is also worth being considered. That brings up a some questions: “is simultaneous presence of pro-inflammatory cytokines and growth factors in the microenvironment immunosuppressive?,” and “doesn't that give a signal for macrophage phenotype polarization to M2 type and for inflammation resolution move forward to proliferation phase?” No such investigations of wound healing have been identified, although there are some reports that partly support this possibility. Mesenchymal stem cells, derived from the umbilical cord, suppressed monocyte differentiation into DC leading to the phenotype that produced IL-10. This was the result of the MSC production of Il-6 and HGF cytokines (156). A similar study generated DCs by monocyte cultivation in the presence of IL-4 and GM-CSF. Multipotent MSCs added into the culture stopped monocyte differentiation and shifted the phenotype to produce IL-10. The effect was associated with IL-6 cytokine production by multipotent MSCs (157). It should be noted that the mentioned culture contained IL-6 and GM-CSF; and that could have had immunomodulating effect on monocytes. Huen et al. found that GM-CSF stimulated alternative macrophage activation after renal ischemic/reperfusion injury (158). GM-CSF is regarded as a pro-inflammatory factor if no additional stimuli are involved.
It is unclear whether the immunosuppressive effect of pro-inflammatory cytokines and growth factors plays an essential role in injury healing. Can it represent one of the mechanisms triggering macrophage polarization to M2 phenotype? So far, the data are insufficient to answer the question. But the described mechanism seems to be of great importance in oncology, which we will discuss below.
Monocytes/macrophages in Tumor Process
Most authors assume that macrophages play the key role in inflammation resolution and transition to the proliferation phase in wound healing. Since the tumor involves natural mechanisms of immunosuppression, it is presumed that myeloid cells such as monocytes/macrophages (including monocytes, macrophages, immature DC, monocytic MDSC) play an essential part in these mechanisms as well. A large number of studies proved macrophage presence in the tumor microenvironment (159). TAM (160) and MDSC (129) functions in the malignant process were well described in some studies. The results of animal studies showed that macrophage (161) or MDSC (162) depletion was associated with the reduction of tumor burden.
However, the authors may have different understandings of the regulatory cell hierarchy. And most likely, T-regulatory CD4+/CD25+/FoxP3+ cells rather than monocytes/macrophages can have the key role in tumor immunosuppression. Regarding this assumption, it should be noted that adaptive immunity is activated by the signals received from the cells of the innate immunity. Treg cells function in cooperation with APCs. Most APCs are DCs and macrophages. Treg cells need antigen stimulation via APC to implement their suppressive function. In turn, Treg suppressive mechanisms function mainly as a result of their interaction with APCs decreasing APC ability to activate effector cells (77, 163). Therefore, macrophages and DCs probably regulate Treg accumulation and activation; thus Treg cells depend on these APCs. We consider that induced Tregs contribute significantly to the tumor tolerance as compared with natural (thymic) Tregs. Normal function of the induced Tregs in maintaining tolerance can be seen in the lungs and intestines. Numerous non-dangerous antigens enter the body through these organs; the reaction to such antigens may result in more harm than good. Immune tolerance to inhaled antigens in the lungs is mainly mediated by T-regulatory cells, which can inhibit effector T cells with a variety of mechanisms. The reports show that regulatory antigen-presenting cells (macrophages and DCs) are crucial for Treg generation and maintenance of the suppressive microenvironment in the lungs (164, 165). Moreover, the studies showed that DCs promote not only Treg accumulation, but, conversely, confine Treg differentiation (166). In fact, there are few reports of this kind regarding tumor microenvironment. Jitschin et al. showed Treg dependence on MDSCs in vitro (127). Hoechst et al. showed that monocytic MDSCs induce Treg generation in vitro (167, 168). The studies on tumor models found that MDSCs caused tolerance to the tumor as a result of Treg accumulation (169, 170).
In regard to the cell hierarchy, we do not insist that monocytes/macrophages have more significant suppressive effect on antitumor immunity than Tregs. Regarding the cell hierarchy, there is only a suggestion that Tregs have a dependent position in relation to monocytes/macrophages. According to these findings, myeloid cells may be a more promising therapeutic target. Thus, in case of effective targeting monocytes/macrophages, Tregs will be automatically affected as well.
Cytokine Interaction in Tumor Microenvironment
This section discusses the impact of certain soluble factors of tumor microenvironment on the polarization of monocytes/macrophages. P53 mediates the cellular aging program, thus protecting the cell from malignant transformation (171). Lujambio et al. showed that senescent stellate cells with unmodified p53 in the liver express factors that promote macrophage polarization to M1 phenotype. These macrophages were able to attack aging cells in culture. At the same time, proliferating p53-deficient stellate cells secrete factors that stimulate macrophage polarization to M2 phenotype (172). Another study evaluated the immuno-mediated clearance of aging hepatocytes to prevent tumor development, a process also called “senescence surveillance.” The study found that “senescence surveillance” requires recruitment and maturation of CCR2+myeloid cells, while their depletion causes HCC growth. On the other hand, the tumor cells prevent maturation of recruited myeloid precursors, and, in addition, these myeloid cells become immunosuppressive (173). Besides HCC, some other cancer types affect myeloid cells in the same mode. Lechner et al. studied about 100 different tumor cell lines cultured in the presence of mononuclear cells of healthy donors. The results showed that 45 cell lines stimulated monocyte transformation into CD33+ MDSC-like cells that could inhibit T-cells (174). Similar results were obtained in the studies of CLL cell cultured with the mononuclear cells of healthy donors (127).
Naturally, the question arises: “what tumor-produced factors lead to immunosuppression of monocytes/macrophages?” Lechner et al. studied 15 immune factors of the tumor cell lines by RT-PCR. Cytokine mixtures were tested for their ability to generate suppressive CD33+ cells from healthy donor mononuclear cells in vitro. The combination of GM-CSF and IL-6 cytokines demonstrated the highest immunosuppressive effect, and the combinations of GM-CSF and IL-1β, PGE2, TNF-α, or VEGF showed immunosuppressive activity, as well (175). Pleiotropic IL-6 role in tumor immunosuppression (176) may be reasonably explained by interaction with other soluble factors. However, when considering GM-CSF, the situation is somewhat more complicated. The GM-CSF immunostimulating and regulatory functions have been discussed for long, but the problem still remains unresolved (177, 178). The above mentioned papers describe in detail the controversial issues related to the problem, however, they propose only their own subjective opinion. The issue is complicated; a number of studies received the opposite results when cultivation of myeloid precursors with GM-CSF led to tolerogenicity (179, 180) or DC maturation (181). However, some authors tried to explain the controversial effects of GM-CSF by its different concentrations. Progenitor cells derived from bone marrow treated with a low dose of GM-CSF may develop into tolerant immature DCs, while the same cells treated with a higher dose of GM-CSF may develop into a mixture of mature and immature DCs (182). This phenomenon can be explained by the fact that the culture with DC precursors could have included low concentrations of pro-inflammatory cytokines, and after addition of GM-CSF in a low dose, they had a joint immunosuppressive effect. In case of GM-CSF high doses, the difference between GM-CSF and pro-inflammatory cytokine concentrations was far more significant, and therefore GM-CSF manifested its immunostimulating effect. On the other hand, there are some in vitro studies where GM-CSF had a suppressive effect in high concentrations, and it is more difficult to explain that phenomenon. Though, it should be noted that the latter studies had certain differences in the methodology as compared with the studies where GM-CSF showed a pro-inflammatory effect (181, 183). Nevertheless, Marigo et al. failed to generate immunosuppressive myeloid cells when cultured with GM-CSF only, but received them in the culture with a combination of GM-CSF + IL-6 (184). Similar results were achieved in some other studies. Immunosuppressive MDSC were obtained in vitro with combinations of such cytokines as GM-CSF + IL-6 (185–187), GM-CSF + IL-6 + PGE2 (188), GM-CSF + IL-6 + G-CSF (189), PGE2 + GM-CSF + IL-4 (190), GM-CSF + IL-6 + IL-1β (191). Cytokine combination IL-6 + G-CSF inhibited differentiation and activation of dendritic cells (192). At this point it is worth remembering the suppressive effect of mesenchymal stem cells on monocytes, which decreased after the blockade of some pro-inflammatory cytokines and growth factors, as it was described above in the section on wound healing.
Furthermore, one may ask the question: “is there such a combination of cytokines in the tumor microenvironment?” It is now assumed that tumors are often associated with persistent unresolved inflammation; therefore, pro-inflammatory cytokines are found in the tumor microenvironment. A thoroughly studied HCC is a good example. We described above the fact that HCC development is normally prevented by inflammation and macrophages with “senescence surveillance” (75), but myeloid cells of the tumor microenvironment become immunosuppressive in the established HCC (173). Besides inflammation, a certain number of growth factors appear in the HCC microenvironment. Later, we will talk about the results of patients' tumor studies. M-CSF high expression and increased macrophage distribution in peritumoral region was associated with HCC progression (193). The enhanced circulating TGF-β1 concentration was associated with the worse survival rate of patients with HCC (194). Serum VEGF levels in patients with HCC were significantly higher than those of healthy donors (195). FGF19 expression correlated with tumor progression and worse prognosis in HCC (196). High serum HGF levels in patients with HCC were associated with poor prognosis after liver resection (197). Pancreatic cancer is also associated with inflammation. Two experimental studies of pancreatic cancer detected GM-CSF in patients' tumor samples after immunohistochemical staining (179, 180). Another review (198) showed the important role of inflammatory cytokines and TAM in the development and progression of pancreatic cancer and found growth factors in the tumor microenvironment. Multiple myeloma is an example of hematological malignancies, which includes the enhanced rate of some growth and pro-inflammatory factors (199–201). The studies found that HGF, bFGF and G-CSF expressions in head and neck squamous cell cancer were negative prognostic factors for patient survival (202). The studies of breast cancer found cytokine G-CSF, IL-6 and IL-17 expression in the serum of cancer patients, but not of healthy volunteers (203). The study of serum cytokines in melanoma reported that the concentrations of several factors (IL-1α, IL-1β, IL-6, IL-8, IL-12p40, IL-13, G-CSF, MCP-1, MIP-1α, MIP-1β, IFN-α, TNF-α, EGF, VEGF, and TNF-RII) were significantly higher in patients with high-risk resected melanoma compared to those of healthy donors. In addition, IFN-α-2 therapy led to a significant decrease in the levels of growth factors, such as VEGF, EGF, and HGF (204). Another paper reviews the evaluation of serum cytokine profile in patients with several cancer types (205). Although it pays less attention to growth factors but it shows their presence. It is worth mentioning that the concentration of cytokines directly in the tumor microenvironment should be regarded as of higher importance.
We suggest a hypothesis with regards to the above discussed data that may explain such functions of soluble factors in tumor microenvironments. According to the hypothesis, a tumor cell acquires some characteristics of a stem cell, including the ability to regulate immunity in a similar way to the interaction between mesenchymal stem cells and macrophages, as it is described in the section on wound healing. Yet, in case of injury, MSCs are found in the inflammatory microenvironment, but they do not produce it. This location stimulates them to produce growth factors. The combination of soluble mediators (pro-inflammatory cytokines and growth factors) promotes monocyte/macrophage immunosuppressive activity. The process in wound healing is most likely a short-term one and helps the transition from the inflammatory to proliferation phase. Tumor cells are supposed to produce or support growth factors and pro-inflammatory cytokines in an independent manner for a long time, using this mechanism to avoid immune reactions (Figures 1A,B, Table 1). However, it is difficult to identify the necessary ratio of growth factors and pro-inflammatory cytokines that leads to immunosuppression; it may be 1:1 or even 1:5. That should be determined in the experiments. It is yet unknown whether all pro-inflammatory and growth factors have such effect. Most likely, these are IL-6 and GM-CSF. It is also important to emphasize that immunosuppression results from the combination of growth factors and pro-inflammatory cytokines, but not from the dominating immunosuppressive growth factors, such as TGF-β. Therefore, it is counter-regulating mechanism that requires two types of signaling. Undoubtedly, besides this mechanism tumor immunosuppression involves other ones. But its role is obviously underrated, and it has the potential of becoming a therapeutic target.
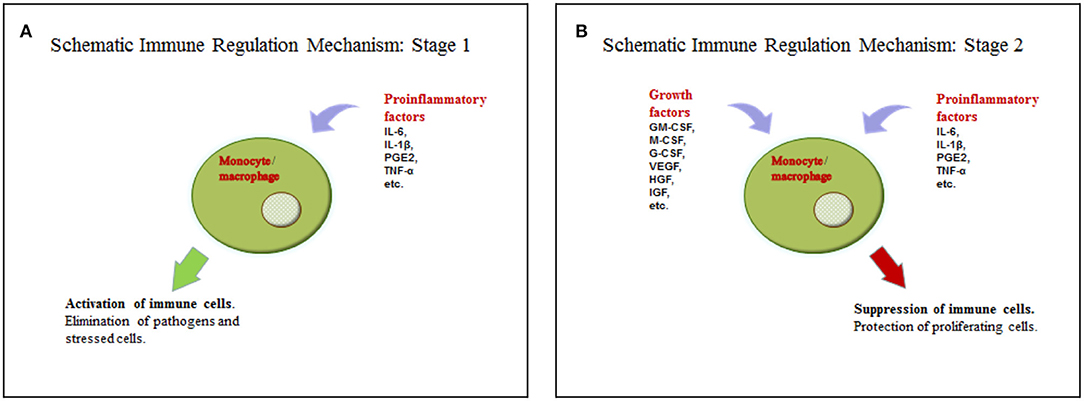
Figure 1. (A) Activation of the immune cells by pro-inflammatory cytokines. (B) Suppression of the immune cells by the combination of pro-inflammatory cytokines and growth factors.
The studies showed that different strategies of resolving tumor-associated inflammation can adversely affect tumor growth and development (206, 207). Yet, assuming the decrease of inflammation should reduce immune functions seems controversial. Our hypothesis explains such effects of reduced inflammation by the alteration of cytokine balance.
Though unexpected, elevated levels of growth factors and pro-inflammatory cytokines were observed in some autoimmune diseases. However, the processes associated with these diseases are opposite–not decrease, but excessive activation of the immune functions–and it is difficult to detect the exact cause of such processes. We suggest there might be mechanisms that are hierarchically higher than immunosuppression caused by the combined effects of inflammatory cytokines and growth factors, such as some super-antigens, and these mechanisms can block immunosuppression.
Signaling Molecules Mediating Monocyte/Macrophage Polarization to the Immunosuppressive Phenotype
The characteristics of the signaling pathways promoting monocyte/macrophage immunosuppression are far from being complete, though some data are already available. A more detailed study of signaling could provide additional data for understanding our hypothesis. At the initial stages, the signal transmission from the receptors of growth factors and pro-inflammatory cytokines is achieved with the “integrated” tyrosine kinases, Jak-STAT, MyD88, TRAF, etc. Understanding the process is rather difficult because of the fact that growth factors such as EGF, PDGF, VEGF, M-CSF use “integrated” tyrosine kinases, whereas colony-stimulating factors such as GM-CSF and some pro-inflammatory cytokines, including IL-6, use Jak–Stat signaling. Therefore, it is difficult to identify any regular patterns at the initial stages of the signaling pathways of growth factors and pro-inflammatory cytokines.
Cytokine IL-6, which has a dual role in the anti-tumor immunity, activates signaling proteins Stat1 and Stat3 in addition to its other functions. Stat1 is known for its anti-tumor activity, whereas Stat3 is known for promoting tumor progression and immunosuppression (208). The balance between the opposite effects of Stat1 and Stat3 is considered to be one of the mechanisms regulating the inflammatory status of macrophages. Some authors believe that Stat3 activation is the key factor responsible for the tolerance associated with tumor escape from the immune surveillance (209, 210).
Transcription factor C/Ebpß plays an important role in the differentiation of myeloid precursors into functional MDSC (184). Moreover, C/Ebpß expression in myeloid precursors was associated with immunosuppression in the murine model of sepsis (211). Other studies demonstrated some correlation between Stat3 and C/EBPβ expression in MDSC in sepsis (212) and in granulocytes during “emergency” granulopoiesis (213). Kaneda et al. report that phosphoinositide-3-kinase γ (PI3Kγ) controls the transition between suppressive and pro-inflammatory macrophages in inflammation and tumor microenvironment. Signal transduction through Akt PI3Kγ and mTor signaling pathways inhibits NFκB activation and stimulates C/EBPβ activation, thereby inducing transcriptional programs that contribute to immune suppression during inflammation and tumor growth (214). Earlier, Chen et al. found that mTOR pathway is an important element in the regulation of monocyte differentiation into TAM (215).
Some signal proteins may be mentioned, which are presumably less likely to participate in immunosuppression associated with pro-inflammatory cytokines and growth factors. A number of studies presented many details of NFkB role in TAM polarization (216), and sometimes they are controversial (217). We will describe only some of the main issues. It is considered that NFkB dimer consisting of P65/P50 subunits plays a pro-inflammatory role, while the dimer comprising P50/P50 (NFκB1, inactive status) plays an anti-inflammatory role in the immune system. Panzer et al. showed NFkB1 role in the resolution of renal inflammation. After induction of immune glomerular injury in rats, mostly NF-kB P65/P50 heterodimer complexes moved to the cell nucleus, while after inflammation resolution mainly P50/P50 homodimers were found in the cell nucleus (218). Enhanced P50/P50 expression supported pro-tumor M2 phenotype of macrophages, and blocked polarization toward M1 (219, 220). Strauss et al. found that protein RORC1/RORγ promotes TAM and MDSC formation during “emergency” granulomonocytopoiesis in cancer (221). Pello et al. demonstrated that c-Myc transcriptional factor is necessary for macrophage polarization to M2 phenotype (222). A number of studies reported that IRF 4 transcription factor (interferon regulatory factor) can participate in an alternative macrophage activation (223, 224).
Conclusion
The paper summarizes the available data on the tumor interaction with the immune system. Cell stress and mutations result in emerging antigens that make a difference between a tumor and a normal cell. Such antigens could have become the targets for immune system recognition. This makes it extremely important for the tumor to have immunosuppressive mechanisms. It is presumed that the tumor does not develop any new mechanisms for inhibiting immune reactions but uses the existing normal mechanisms. Therefore, we made an attempt to draw analogies of immunosuppressive mechanisms in the tumor microenvironment and in wound healing. At the same time, we outlined some common features and regular patterns of the microenvironment, which we put as the basis for our hypothesis. Wound healing is characterized by the simultaneous presence of growth factors and pro-inflammatory cytokines in the MSC microenvironment during the transition stage from inflammation to proliferation. We suggest that these cytokines function in cooperation and thus have a regulatory effect on monocytes/macrophages. The affected monocytes/macrophages transfer the immunosuppressive pattern onto the cells of the adaptive immune system. The above presented data demonstrate that such effects of pro-inflammatory cytokines and growth factors can be used by tumor cells to evade immune surveillance. The described details help to explain the phenomenon of the immunosuppressive inflammation in the tumor microenvironment. The combined effect of growth factors and pro-inflammatory cytokines on monocytes/macrophages has been poorly studied, yet. In case if this hypothesis is proved, the cytokine interaction could become a promising therapeutic target and have a very wide range of applications, both in oncology and treatment of some other conditions associated with abnormalities in the immune system.
Author Contributions
AP wrote the manuscript. IS critically reviewed the manuscript and contributed to the figures. All authors agree to be accountable for the content of the work.
Conflict of Interest
The authors declare that the research was conducted in the absence of any commercial or financial relationships that could be construed as a potential conflict of interest.
References
1. Fadeel B, Xue D, Kagan V. Programmed cell clearance: molecular regulation of the elimination of apoptotic cell corpses and its role in the resolution of inflammation. Biochem Biophys Res Commun. (2010) 396:7–10. doi: 10.1016/j.bbrc.2010.02.106
2. Arandjelovic S, Ravichandran KS. Phagocytosis of apoptotic cells in homeostasis. Nat Immunol. (2015) 16:907–17. doi: 10.1038/ni.3253
3. Vallabhapurapu SD, Blanco VM, Sulaiman MK, Vallabhapurapu SL, Chu Z, Franco RS, et al. Variation in human cancer cell external phosphatidylserine is regulated by flippase activity and intracellular calcium. Oncotarget. (2015) 6:34375–88. doi: 10.18632/oncotarget.6045
4. Ostwald TJ, MacLennan DH. Isolation of a high affinity calcium-binding protein from sarcoplasmic reticulum. J Biol Chem. (1974) 249:974–9.
5. Burns K, Duggan B, Atkinson EA, Famulski KS, Nemer M, Bleackley RC, et al. Modulation of gene expression by calreticulin binding to the glucocorticoid receptor. Nature. (1994) 367:476–80. doi: 10.1038/367476a0
6. Arosa FA, de Jesus O, Porto G, Carmo AM, de Sousa M. Calreticulin is expressed on the cell surface of activated human peripheral blood T lymphocytes in association with major histocompatibility complex class I molecules. J Biol Chem. (1999) 274:16917–22. doi: 10.1074/jbc.274.24.16917
7. Gardai SJ, McPhillips KA, Frasch SC, Janssen WJ, Starefeldt A, Murphy-Ullrich JE, et al. Cell-surface calreticulin initiates clearance of viable or apoptotic cells through trans-activation of LRP on the phagocyte. Cell. (2005) 123:321–34. doi: 10.1016/j.cell.2005.08.032
8. Chao MP, Jaiswal S, Weissman-Tsukamoto R, Alizadeh AA, Gentles AJ, Volkmer J, et al. Calreticulin is the dominant pro-phagocytic signal on multiple human cancers and is counterbalanced by CD47. Sci Transl Med. (2010) 2:63ra94. doi: 10.1126/scitranslmed.3001375
9. Feng M, Chen JY, Weissman-Tsukamoto R, Volkmer JP1, Ho PY, McKenna KM, et al. Macrophages eat cancer cells using their own calreticulin as a guide: roles of TLR and Btk. Proc Natl Acad Sci USA. (2015) 112:2145–50. doi: 10.1073/pnas.1424907112
10. Matlung HL, Szilagyi K, Barclay NA, van den Berg TK. The CD47-SIRPα signaling axis as an innate immune checkpoint in cancer. Immunol Rev. (2017) 276:145–64. doi: 10.1111/imr.12527
11. Lazarev VF, Nikotina AD, Mikhaylova ER, Nudler E, Polonik SG, Guzhova IV, et al. Hsp70 chaperone rescues C6 rat glioblastoma cells from oxidative stress by sequestration of aggregating GAPDH. Biochem Biophys Res Commun. (2016) 470:766–71. doi: 10.1016/j.bbrc.2015.12.076
12. Garrido C, Fromentin A, Bonnotte B, Favre N, Moutet M, Arrigo AP, et al. Heat shock protein 27 enhances the tumorigenicity of immunogenic rat colon carcinoma cell clones. Cancer Res. (1998) 58:5495–9.
13. Radons J, Multhoff G. Immunostimulatory functions of membrane-bound and exported heat shock protein 70. Exerc Immunol Rev. (2005) 11:17–33.
14. Murshid A, Gong J, Stevenson MA, Calderwood SK. Heat shock proteins and cancer vaccines: developments in the past decade and chaperoning in the decade to come. Expert Rev Vaccines. (2011) 10:1553–68. doi: 10.1586/erv.11.124
15. Zininga T, Ramatsui L, Shonhai A. Heat shock proteins as immunomodulants. Molecules. (2018) 23:E2846. doi: 10.3390/molecules23112846
16. Noessner E, Gastpar R, Milani V, Brandl A, Hutzler PJ, Kuppner MC, et al. Tumor-derived heat shock protein 70 peptide complexes are cross-presented by human dendritic cells. J Immunol. (2002) 169:5424–32. doi: 10.4049/jimmunol.169.10.5424
17. Murshid A, Gong J, Calderwood SK. Heat shock protein 90 mediates efficient antigen cross presentation through the scavenger receptor expressed by endothelial cells-I. J Immunol. (2010) 185:2903–17. doi: 10.4049/jimmunol.0903635
18. Shin BK, Wang H, Yim AM, Le Naour F, Brichory F, Jang JH, et al. Global profiling of the cell surface proteome of cancer cells uncovers an abundance of proteins with chaperone function. J Biol Chem. (2003) 278:7607–16. doi: 10.1074/jbc.M210455200
19. Hantschel M, Pfister K, Jordan A, Scholz R, Andreesen R, Schmitz G, et al. Hsp70 plasma membrane expression on primary tumor biopsy material and bone marrow of leukemic patients. Cell Stress Chaperones. (2000) 5:438–22. doi: 10.1379/1466-1268(2000)005<0438:HPMEOP>2.0.CO;2
20. Multhoff G, Botzler C, Wiesnet M, Müller E, Meier T, Wilmanns W, et al. A stress-inducible 72-kDa heat-shock protein (HSP72) is expressed on the surface of human tumor cells, but not on normal cells. Int J Cancer. (1995) 61:272–9. doi: 10.1002/ijc.2910610222
21. Multhoff G, Botzler C, Wiesnet M, Eissner G, Issels R. CD3- large granular lymphocytes recognize a heat-inducible immunogenic determinant associated with the 72-kD heat shock protein on human sarcoma cells. Blood. (1995) 86:1374–82. doi: 10.1182/blood.V86.4.1374.bloodjournal8641374
22. Gross C, Schmidt-Wolf IG, Nagaraj S, Gastpar R, Ellwart J, Kunz-Schughart LA, et al. Heat shock protein 70-reactivity is associated with increased cell surface density of CD94/CD56 on primary natural killer cells. Cell Stress Chaperones. (2003) 8:348–602. doi: 10.1379/1466-1268(2003)008<0348:HSPRIA>2.0.CO;2
23. Stangl S, Gross C, Pockley AG, Asea AA, Multhoff G. Influence of Hsp70 and HLA-E on the killing of leukemic blasts by cytokine/Hsp70 peptide-activated human natural killer (NK) cells. Cell Stress Chaperones. (2008) 13:221–30. doi: 10.1007/s12192-007-0008-y
24. Gross C, Holler E, Stangl S, Dickinson A, Pockley AG, Asea AA, et al. An Hsp70 peptide initiates NK cell killing of leukemic blasts after stem cell transplantation. Leuk Res. (2008) 32:527–34. doi: 10.1016/j.leukres.2007.03.027
25. Chen Y, Mo J, Jia X, He Y. The B7 family member B7-H6: a new bane of tumor. Pathol Oncol Res. (2018) 24:717–21. doi: 10.1007/s12253-017-0357-5
26. Brandt CS, Baratin M, Yi EC, Kennedy J, Gao Z, Fox B, et al. The B7 family member B7-H6 is a tumor cell ligand for the activating natural killer cell receptor NKp30 in humans. J Exp Med. (2009) 206:1495–503. doi: 10.1084/jem.20090681
27. Fiegler N, Textor S, Arnold A, Rolle A, Oehme I, Breuhahn K, et al. Downregulation of the activating NKp30 ligand B7-H6 by HDAC inhibitors impairs tumor cell recognition by NK cells. Blood. (2013) 122:684–93. doi: 10.1182/blood-2013-02-482513
28. Matta J, Baratin M, Chiche L, Forel JM, Cognet C, Thomas G, et al. Induction of B7-H6, a ligand for the natural killer cell-activating receptor NKp30, in inflammatory conditions. Blood. (2013) 122:394–404. doi: 10.1182/blood-2013-01-481705
29. Chen XJ, Shen J, Zhang GB, Chen WC. B7-H6 protein expression has no prognostic significance in human gastric carcinoma. Pathol Oncol Res. (2014) 20:203–7. doi: 10.1007/s12253-013-9686-1
30. Zhang X, Zhang G, Qin Y, Bai R, Huang J. B7-H6 expression in non-small cell lung cancers. Int J Clin Exp Pathol. (2014) 7:6936–42.
31. Sun J, Tao H, Li X, Wang L, Yang J, Wu P, et al. Clinical significance of novel costimulatory molecule B7-H6 in human breast cancer. Oncol Lett. (2017) 14:2405–9. doi: 10.3892/ol.2017.6417
32. Zhou Y, Xu Y, Chen L, Xu B, Wu C, Jiang J. B7-H6 expression correlates with cancer progression and patient's survival in human ovarian cancer. Int J Clin Exp Pathol. (2015) 8:9428–33.
33. Schlecker E, Fiegler N, Arnold A, Altevogt P, Rose-John S, Moldenhauer G, et al. Metalloprotease-mediated tumor cell shedding of B7-H6, the ligand of the natural killer cell-activating receptor NKp30. Cancer Res. (2014) 74:3429–40. doi: 10.1158/0008-5472.CAN-13-3017
34. Jiang T, Wu W, Zhang H, Zhang X, Zhang D, Wang Q, et al. High expression of B7-H6 in human glioma tissues promotes tumor progression. Oncotarget. (2017) 8:37435–47. doi: 10.18632/oncotarget.16391
35. Pesce S, Tabellini G, Cantoni C, Patrizi O, Coltrini D, Rampinelli F, et al. B7-H6-mediated downregulation of NKp30 in NK cells contributes to ovarian carcinoma immune escape. Oncoimmunology. (2015) 4:e1001224. doi: 10.1080/2162402X.2014.1001224
36. Bauer S, Groh V, Wu J, Steinle A, Phillips JH, Lanier LL, et al. Activation of NK cells and T cells by NKG2D, a receptor for stress-inducible MICA. Science. (1999) 285:727–9. doi: 10.1126/science.285.5428.727
37. Jamieson AM, Diefenbach A, McMahon CW, Xiong N, Carlyle JR, Raulet DH. The role of the NKG2D immunoreceptor in immune cell activation and natural killing. Immunity. (2002) 17:19–29. doi: 10.1016/S1074-7613(02)00333-3
38. Raulet DH, Gasser S, Gowen BG, Deng W, Jung H. Regulation of ligands for the NKG2D activating receptor. Annu Rev Immunol. (2013) 31:413–41. doi: 10.1146/annurev-immunol-032712-095951
39. Li P, Morris DL, Willcox BE, Steinle A, Spies T, Strong RK. Complex structure of the activating immunoreceptor NKG2D and its MHC class I-like ligand MICA. Nat Immunol. (2001) 2:443–51. doi: 10.1038/87757
40. Groh V, Bahram S, Bauer S, Herman A, Beauchamp M, Spies T. Cell stress-regulated human major histocompatibility complex class I gene expressed in gastrointestinal epithelium. Proc Natl Acad Sci USA. (1996) 93:12445–50. doi: 10.1073/pnas.93.22.12445
41. Spear P, Wu MR, Sentman ML, Sentman CL. NKG2D ligands as therapeutic targets. Cancer Immun. (2013) 13:8.
42. Groh V, Rhinehart R, Secrist H, Bauer S, Grabstein KH, Spies T. Broad tumor-associated expression and recognition by tumor-derived gamma delta T cells of MICA and MICB. Proc Natl Acad Sci USA. (1999) 96:6879–84. doi: 10.1073/pnas.96.12.6879
43. Jinushi M, Takehara T, Tatsumi T, Kanto T, Groh V, Spies T, et al. Expression and role of MICA and MICB in human hepatocellular carcinomas and their regulation by retinoic acid. Int J Cancer. (2003) 104:354–61. doi: 10.1002/ijc.10966
44. Vetter CS, Groh V, thor Straten P, Spies T, Brocker EB, Becker JC. Expression of stress-induced MHC class I related chain molecules on human melanoma. J Invest Dermatol. (2002) 118:600–5. doi: 10.1046/j.1523-1747.2002.01700.x
45. Salih HR, Antropius H, Gieseke F, Lutz SZ, Kanz L, Rammensee HG, et al. Functional expression and release of ligands for the activating immunoreceptor NKG2D in leukemia. Blood. (2003) 102:1389–96. doi: 10.1182/blood-2003-01-0019
46. Gasser S, Orsulic S, Brown EJ, Raulet DH. The DNA damage pathway regulates innate immune system ligands of the NKG2D receptor. Nature. (2005) 436:1186–90. doi: 10.1038/nature03884
47. Groh V, Rhinehart R, Randolph-Habecker J, Topp MS, Riddell SR, Spies T. Costimulation of CD8alphabeta T cells by NKG2D via engagement by MIC induced on virus-infected cells. Nat Immunol. (2001) 2:255–60. doi: 10.1038/85321
48. Cosman D, Mullberg J, Sutherland CL, Chin W, Armitage R, Fanslow W, et al. ULBPs, novel MHC class I-related molecules, bind to CMV glycoprotein UL16 and stimulate NK cytotoxicity through the NKG2D receptor. Immunity. (2001) 14:123–33. doi: 10.1016/S1074-7613(01)00095-4
49. Diefenbach A, Tomasello E, Lucas M, Jamieson AM, Hsia JK, Vivier E, et al. Selective associations with signaling proteins determine stimulatory versus costimulatory activity of NKG2D. Nat Immunol. (2002) 3:1142–9. doi: 10.1038/ni858
50. Regunathan J, Chen Y, Wang D, Malarkannan S. NKG2D receptor-mediated NK cell function is regulated by inhibitory Ly49 receptors. Blood. (2005) 105:233–40. doi: 10.1182/blood-2004-03-1075
51. Bryceson YT, Ljunggren HG, Long EO. Minimal requirement for induction of natural cytotoxicity and intersection of activation signals by inhibitory receptors. Blood. (2009) 114:2657–66. doi: 10.1182/blood-2009-01-201632
52. Mocikat R, Braumuller H, Gumy A, Egeter O, Ziegler H, Reusch U, et al. Natural killer cells activated by MHC class I(low) targets prime dendritic cells to induce protective CD8 T cell responses. Immunity. (2003) 19:561–9. doi: 10.1016/S1074-7613(03)00264-4
53. Kelly JM, Darcy PK, Markby JL, Godfrey DI, Takeda K, Yagita H, et al. Induction of tumor-specific T cell memory by NK cell-mediated tumor rejection. Nat Immunol. (2002) 3:83–90. doi: 10.1038/ni746
54. Fujii S1, Shimizu K, Smith C, Bonifaz L, Steinman RM. Activation of natural killer T cells by alpha-galactosylceramide rapidly induces the full maturation of dendritic cells in vivo and thereby acts as an adjuvant for combined CD4 and CD8 T cell immunity to a coadministered protein. J Exp Med. (2003) 198:267–79. doi: 10.1084/jem.20030324
55. Hernandez C, Huebener P, Schwabe RF. Damage-associated molecular patterns in cancer: a double-edged sword. Oncogene. (2016) 35:5931–41. doi: 10.1038/onc.2016.104
56. da Silva VL, Fonseca AF, Fonseca M, da Silva TE, Coelho AC, Kroll JE, et al. Genome-wide identification of cancer/testis genes and their association with prognosis in a pan-cancer analysis. Oncotarget. (2017) 8:92966–77. doi: 10.18632/oncotarget.21715
57. Janitz M, Fiszer D, Michalczak-Janitz K, Lukaszyk A, Fernandez N, Skorupski W, et al. Analysis of mRNA for class I HLA on human gametogenic cells. Mol Reprod Dev. (1994) 38:231–7. doi: 10.1002/mrd.1080380215
58. Gotter J, Brors B, Hergenhahn M, Kyewski B. Medullary epithelial cells of the human thymus express a highly diverse selection of tissue-specific genes colocalized in chromosomal clusters. J Exp Med. (2004) 199:155–66. doi: 10.1084/jem.20031677
59. Gjerstorff MF, Andersen MH, Ditzel HJ. Oncogenic cancer/testis antigens: prime candidates for immunotherapy. Oncotarget. (2015) 6:15772–87. doi: 10.18632/oncotarget.4694
60. Almeida LG, Sakabe NJ, deOliveira AR, Silva MC, Mundstein AS, Cohen T, et al. CTdatabase: a knowledge-base of high-throughput and curated data on cancer-testis antigens. Nucleic Acids Res. (2009) 37:D816–9. doi: 10.1093/nar/gkn673
61. Hughes A, Clarson J, Tang C, Vidovic L, White DL, Hughes TP, et al. CML patients with deep molecular responses to TKI have restored immune effectors and decreased PD-1 and immune suppressors. Blood. (2017) 129:1166–76. doi: 10.1182/blood-2016-10-745992
62. Vogelstein B, Papadopoulos N, Velculescu VE, Zhou S, Diaz LA Jr, Kinzler KW. Cancer genome landscapes. Science. (2013) 339:1546–58. doi: 10.1126/science.1235122
63. Lawrence MS, Stojanov P, Mermel CH, Robinson JT, Garraway LA, Golub TR, et al. Discovery and saturation analysis of cancer genes across 21 tumour types. Nature. (2014) 505:495–501. doi: 10.1038/nature12912
64. Sensi M, Anichini A. Unique tumor antigens: evidence for immune control of genome integrity and immunogenic targets for T cell-mediated patient-specific immunotherapy. Clin Cancer Res. (2006) 12:5023–32. doi: 10.1158/1078-0432.CCR-05-2682
65. Rizvi NA, Hellmann MD, Snyder A, Kvistborg P, Makarov V, Havel JJ, et al. Cancer immunology. Mutational landscape determines sensitivity to PD-1 blockade in non-small cell lung cancer. Science. (2015) 348:124–8. doi: 10.1126/science.aaa1348
66. Hugo W, Zaretsky JM, Sun L, Song C, Moreno BH, Hu-Lieskovan S, et al. Genomic and transcriptomic features of response to Anti-PD-1 therapy in metastatic melanoma. Cell. (2016) 165:35–44. doi: 10.1016/j.cell.2016.02.065
67. Rosenberg JE, Hoffman-Censits J, Powles T, van der Heijden MS, Balar AV, Necchi A, et al. Atezolizumab in patients with locally advanced and metastatic urothelial carcinoma who have progressed following treatment with platinum-based chemotherapy: a single-arm, multicentre, phase 2 trial. Lancet. (2016) 387:1909–20. doi: 10.1016/S0140-6736(16)00561-4
68. Samstein RM, Lee CH, Shoushtari AN, Hellmann MD, Shen R, Janjigian YY, et al. Tumor mutational load predicts survival after immunotherapy across multiple cancer types. Nat Genet. (2019) 51:202–6. doi: 10.1038/s41588-018-0312-8
69. Gubin MM, Zhang X, Schuster H, Caron E, Ward JP, Noguchi T, et al. Checkpoint blockade cancer immunotherapy targets tumour-specific mutant antigens. Nature. (2014) 515:577–81. doi: 10.1038/nature13988
70. Ott PA, Hu Z, Keskin DB, Shukla SA, Sun J, Bozym DJ, et al. An immunogenic personal neoantigen vaccine for patients with melanoma. Nature. (2017) 547:217–21. doi: 10.1038/nature22991
71. Sahin U, Derhovanessian E, Miller M, Kloke BP, Simon P, Lower M, et al. Personalized RNA mutanome vaccines mobilize poly-specific therapeutic immunity against cancer. Nature. (2017) 547:222–6. doi: 10.1038/nature23003
72. Kang TW, Yevsa T, Woller N, Hoenicke L, Wuestefeld T, Dauch D, et al. Senescence surveillance of pre-malignant hepatocytes limits liver cancer development. Nature. (2011) 479:547–51. doi: 10.1038/nature10599
73. Munn DH, Bronte V. Immune suppressive mechanisms in the tumor microenvironment. Curr Opin Immunol. (2016) 39:1–6. doi: 10.1016/j.coi.2015.10.009
74. Pitt JM, Marabelle A, Eggermont A, Soria JC, Kroemer G, Zitvogel L. Targeting the tumor microenvironment: removing obstruction to anticancer immune responses and immunotherapy. Ann Oncol. (2016) 27:1482–92. doi: 10.1093/annonc/mdw168
75. Kythreotou A, Siddique A, Mauri FA, Bower M, Pinato DJ. PD-L1. J Clin Pathol. (2018) 71:189–94. doi: 10.1136/jclinpath-2017-204853
76. Solinas C, Migliori E, De Silva P, Willard-Gallo K. LAG3: The biological processes that motivate targeting this immune checkpoint molecule in human cancer. Cancers. (2019) 11:E1213. doi: 10.3390/cancers11081213
77. Qureshi OS, Zheng Y, Nakamura K, Attridge K, Manzotti C, Schmidt EM, et al. Trans-endocytosis of CD80 and CD86: a molecular basis for the cell-extrinsic function of CTLA-4. Science. (2011) 332:600–3. doi: 10.1126/science.1202947
78. Walker LSK. EFIS Lecture: Understanding the CTLA-4 checkpoint in the maintenance of immune homeostasis. Immunol Lett. (2017) 184:43–50. doi: 10.1016/j.imlet.2017.02.007
79. Batlle E, Massague J. Transforming growth factor-β signaling in immunity and cancer. Immunity. (2019) 50:924–40. doi: 10.1016/j.immuni.2019.03.024
80. Geginat J, Larghi P, Paroni M, Nizzoli G, Penatti A, Pagani M, et al. The light and the dark sides of Interleukin-10 in immune-mediated diseases and cancer. Cytokine Growth Factor Rev. (2016) 30:87–93. doi: 10.1016/j.cytogfr.2016.02.003
81. O'Callaghan G, Houston A. Prostaglandin E2 and the EP receptors in malignancy: possible therapeutic targets? Br J Pharmacol. (2015) 172:5239–50. doi: 10.1111/bph.13331
82. Tsukamoto H, Fujieda K, Senju S, Ikeda T, Oshiumi H, Nishimura Y. Immune-suppressive effects of interleukin-6 on T-cell-mediated anti-tumor immunity. Cancer Sci. (2018) 109:523–30. doi: 10.1111/cas.13433
83. Sek K, Molck C, Stewart GD, Kats L, Darcy PK, Beavis PA. Targeting adenosine receptor signaling in cancer immunotherapy. Int J Mol Sci. (2018) 19:E3837. doi: 10.3390/ijms19123837
84. Colegio OR, Chu NQ, Szabo AL, Chu T, Rhebergen AM, Jairam V, et al. Functional polarization of tumour-associated macrophages by tumour-derived lactic acid. Nature. (2014) 513:559–63. doi: 10.1038/nature13490
85. Chitadze G, Bhat J, Lettau M, Janssen O, Kabelitz D. Generation of soluble NKG2D ligands: proteolytic cleavage, exosome secretion and functional implications. Scand J Immunol. (2013) 78:120–9. doi: 10.1111/sji.12072
86. Rodriguez PC, Ochoa AC. Arginine regulation by myeloid derived suppressor cells and tolerance in cancer: mechanisms and therapeutic perspectives. Immunol Rev. (2008) 222:180–91. doi: 10.1111/j.1600-065X.2008.00608.x
87. Srivastava MK, Sinha P, Clements VK, Rodriguez P, Ostrand-Rosenberg S. Myeloid-derived suppressor cells inhibit T-cell activation by depleting cystine and cysteine. Cancer Res. (2010) 70:68–77. doi: 10.1158/0008-5472.CAN-09-2587
88. Munn DH, Mellor AL. IDO in the tumor microenvironment: inflammation, counter-regulation, and tolerance. Trends Immunol. (2016) 37:193–207. doi: 10.1016/j.it.2016.01.002
89. Dvorak HF. Tumors: wounds that do not heal. Similarities between tumor stroma generation and wound healing. N Engl J Med. (1986) 315:1650–9. doi: 10.1056/NEJM198612253152606
90. Rybinski B, Franco-Barraza J, Cukierman E. The wound healing, chronic fibrosis, and cancer progression triad. Physiol Genomics. (2014) 46:223–44. doi: 10.1152/physiolgenomics.00158.2013
91. Thorsson V, Gibbs DL, Brown SD, Wolf D, Bortone DS, Ou Yang TH, et al. The Immune landscape of cancer. Immunity. (2018) 48:812–830.e14. doi: 10.1016/j.immuni.2018.03.023
92. Wallace HA, Zito PM. Wound Healing Phases. StatPearls. Treasure Island (FL): StatPearls Publishing (2019).
93. Ellis S, Lin EJ, Tartar D. Immunology of Wound Healing. Curr Dermatol Rep. (2018) 7:350–8. doi: 10.1007/s13671-018-0234-9
94. Dong H, Zhu G, Tamada K, Chen L. B7-H1, a third member of the B7 family, co-stimulates T-cell proliferation and interleukin-10 secretion. Nat Med. (1999) 5:1365–9. doi: 10.1038/70932
95. Dong H, Strome SE, Salomao DR, Tamura H, Hirano F, Flies DB, et al. Tumor-associated B7-H1 promotes T-cell apoptosis: a potential mechanism of immune evasion. Nat Med. (2002) 8:793–800. doi: 10.1038/nm730
96. Grabie N, Gotsman I, DaCosta R, Pang H, Stavrakis G, Butte MJ, et al. Endothelial programmed death-1 ligand 1 (PD-L1) regulates CD8+ T-cell mediated injury in the heart. Circulation. (2007) 116:2062–71. doi: 10.1161/CIRCULATIONAHA.107.709360
97. Keir ME, Butte MJ, Freeman GJ, Sharpe AH. PD-1 and its ligands in tolerance and immunity. Annu Rev Immunol. (2008) 26:677–704. doi: 10.1146/annurev.immunol.26.021607.090331
98. Mazanet MM, Hughes CC. B7-H1 is expressed by human endothelial cells and suppresses T cell cytokine synthesis. J Immunol. (2002) 169:3581–8. doi: 10.4049/jimmunol.169.7.3581
99. Cekic C, Linden J. Purinergic regulation of the immune system. Nat Rev Immunol. (2016) 16:177–92. doi: 10.1038/nri.2016.4
100. Trabold O, Wagner S, Wicke C, Scheuenstuhl H, Hussain MZ, Rosen N, et al. Lactate and oxygen constitute a fundamental regulatory mechanism in wound healing. Wound Repair Regen. (2003) 11:504–9. doi: 10.1046/j.1524-475X.2003.11621.x
101. Britland S, Ross-Smith O, Jamil H, Smith AG, Vowden K, Vowden P. The lactate conundrum in wound healing: clinical and experimental findings indicate the requirement for a rapid point-of-care diagnostic. Biotechnol Prog. (2012) 28:917–24. doi: 10.1002/btpr.1561
102. Porporato PE, Payen VL, De Saedeleer CJ, Préat V, Thissen JP, Feron O, et al. Lactate stimulates angiogenesis and accelerates the healing of superficial and ischemic wounds in mice. Angiogenesis. (2012) 15:581–92. doi: 10.1007/s10456-012-9282-0
103. Sun S, Li H, Chen J, Qian Q. Lactic acid: no longer an inert and end-product of glycolysis. Physiology. (2017) 32:453–63. doi: 10.1152/physiol.00016.2017
104. Nosbaum A, Prevel N, Truong HA, Mehta P, Ettinger M, Scharschmidt TC, et al. Cutting edge: regulatory t cells facilitate cutaneous wound healing. J Immunol. (2016) 196:2010–4. doi: 10.4049/jimmunol.1502139
105. Zhang C, Li L, Feng K, Fan D, Xue W, Lu J. 'Repair' Treg cells in tissue injury. Cell Physiol Biochem. (2017) 43:2155–69. doi: 10.1159/000484295
106. Brancato SK, Albina JE. Wound macrophages as key regulators of repair: origin, phenotype, and function. Am J Pathol. (2011) 178:19–25. doi: 10.1016/j.ajpath.2010.08.003
107. Kim SY, Nair MG. Macrophages in wound healing: activation and plasticity. Immunol Cell Biol. (2019) 97:258–67. doi: 10.1111/imcb.12236
108. Leibovich SJ, Ross R. The role of the macrophage in wound repair. A study with hydrocortisone and antimacrophage serum. Am J Pathol. (1975) 78:71–100.
109. Goren I, Allmann N, Yogev N, Schurmann C, Linke A, Holdener M, et al. A transgenic mouse model of inducible macrophage depletion: effects of diphtheria toxin-driven lysozyme M-specific cell lineage ablation on wound inflammatory, angiogenic, and contractive processes. Am J Pathol. (2009) 175:132–47. doi: 10.2353/ajpath.2009.081002
110. Mirza R, DiPietro LA, Koh TJ. Selective and specific macrophage ablation is detrimental to wound healing in mice. Am J Pathol. (2009) 175:2454–62. doi: 10.2353/ajpath.2009.090248
111. Landen NX, Li D, Stahle M. Transition from inflammation to proliferation: a critical step during wound healing. Cell Mol Life Sci. (2016) 73:3861–85. doi: 10.1007/s00018-016-2268-0
112. Mantovani A, Biswas SK, Galdiero MR, Sica A, Locati M. Macrophage plasticity and polarization in tissue repair and remodelling. J Pathol. (2013) 229:176–85. doi: 10.1002/path.4133
113. Daley JM, Brancato SK, Thomay AA, Reichner JS, Albina JE. The phenotype of murine wound macrophages. J Leukoc Biol. (2010) 87:59–67. doi: 10.1189/jlb.0409236
114. Dovi JV, Szpaderska AM, DiPietro LA. Neutrophil function in the healing wound: adding insult to injury? Thromb Haemost. (2004) 92:275–80. doi: 10.1160/TH03-11-0720
115. Meszaros AJ, Reichner JS, Albina JE. Macrophage-induced neutrophil apoptosis. J Immunol. (2000) 165:435–41. doi: 10.4049/jimmunol.165.1.435
116. Meszaros AJ1, Reichner JS, Albina JE. Macrophage phagocytosis of wound neutrophils. J Leukoc Biol. (1999) 65:35–42. doi: 10.1002/jlb.65.1.35
117. Fadok VA, Bratton DL, Konowal A, Freed PW, Westcott JY, Henson PM. Macrophages that have ingested apoptotic cells in vitro inhibit proinflammatory cytokine production through autocrine/paracrine mechanisms involving TGF-beta, PGE2, and PAF. J Clin Invest. (1998) 101:890–8. doi: 10.1172/JCI1112
118. Chernykh ER, Sakhno LV, Shevela E., Tikhonova MA, Khonina NA, Ostanin AA. The influence of apoptotic neutrophils on production of cytokines and prostaglandin E2 by human M1 macrophages. Immunologiya. (2017) 38:193–8. doi: 10.18821/0206-4952-2017-38-4-193-198
119. Wang J, Hossain M, Thanabalasuriar A, Gunzer M, Meininger C, Kubes P. Visualizing the function and fate of neutrophils in sterile injury and repair. Science. (2017) 358:111–6. doi: 10.1126/science.aam9690
120. Grinberg S, Hasko G, Wu D, Leibovich SJ. Suppression of PLCbeta2 by endotoxin plays a role in the adenosine A(2A) receptor-mediated switch of macrophages from an inflammatory to an angiogenic phenotype. Am J Pathol. (2009) 175:2439–53. doi: 10.2353/ajpath.2009.090290
121. Edwards JP, Zhang X, Frauwirth KA, Mosser DM. Biochemical and functional characterization of three activated macrophage populations. J Leukoc Biol. (2006) 80:1298–307. doi: 10.1189/jlb.0406249
122. Vasandan AB, Jahnavi S, Shashank C, Prasad P, Kumar A, Prasanna SJ. Human Mesenchymal stem cells program macrophage plasticity by altering their metabolic status via a PGE2-dependent mechanism. Sci Rep. (2016) 6:38308. doi: 10.1038/srep38308
123. Nakanishi M, Rosenberg DW. Multifaceted roles of PGE2 in inflammation and cancer. Semin Immunopathol. (2013) 35:123–37. doi: 10.1007/s00281-012-0342-8
124. Livingston DH, Appel SH, Wellhausen SR, Sonnenfeld G, Polk HC Jr. Depressed interferon gamma production and monocyte HLA-DR expression after severe injury. Arch Surg. (1988) 123:1309–12. doi: 10.1001/archsurg.1988.01400350023002
125. Vester H, Dargatz P, Huber-Wagner S, Biberthaler P, van Griensven M. HLA-DR expression on monocytes is decreased in polytraumatized patients. Eur J Med Res. (2015) 20:84. doi: 10.1186/s40001-015-0180-y
126. Filipazzi P, Valenti R, Huber V, Pilla L, Canese P, Iero M, et al. Identification of a new subset of myeloid suppressor cells in peripheral blood of melanoma patients with modulation by a granulocyte-macrophage colony-stimulation factor-based antitumor vaccine. J Clin Oncol. (2007) 25:2546–53. doi: 10.1200/JCO.2006.08.5829
127. Jitschin R, Braun M, Buttner M, Dettmer-Wilde K, Bricks J, Berger J, et al. CLL-cells induce IDOhi CD14+HLA-DRlo myeloid-derived suppressor cells that inhibit T-cell responses and promote TRegs. Blood. (2014) 124:750–60. doi: 10.1182/blood-2013-12-546416
128. Ponomarev AV. Myeloid-derived suppressor cells in some oncohematological diseases. Clin Oncohematol. (2017) 10:29–38. doi: 10.21320/2500-2139-2017-10-1-29-38
129. Veglia F, Perego M, Gabrilovich D. Myeloid-derived suppressor cells coming of age. Nat Immunol. (2018) 19:108–19. doi: 10.1038/s41590-017-0022-x
130. Cheron A, Monneret G, Landelle C, Floccard B, Allaouchiche B. Low monocytic HLA-DR expression and risk of secondary infection. Ann Fr Anesth Reanim. (2010) 29:368–76. doi: 10.1016/j.annfar.2010.02.015
131. Hosomi S, Koyama Y, Watabe T, Ohnishi M, Ogura H, Yamashita T, et al. Myeloid-derived suppressor cells infiltrate the brain and suppress neuroinflammation in a mouse model of focal traumatic brain injury. Neuroscience. (2019) 406:457–66. doi: 10.1016/j.neuroscience.2019.03.015
132. Saiwai H, Kumamaru H, Ohkawa Y, Kubota K, Kobayakawa K, Yamada H, et al. Ly6C+ Ly6G- Myeloid-derived suppressor cells play a critical role in the resolution of acute inflammation and the subsequent tissue repair process after spinal cord injury. J Neurochem. (2013) 125:74–88. doi: 10.1111/jnc.12135
133. Corzo CA, Condamine T, Lu L, Cotter MJ, Youn JI, Cheng P, Cho HI, et al. HIF-1α regulates function and differentiation of myeloid-derived suppressor cells in the tumor microenvironment. J Exp Med. (2010) 207:2439–53. doi: 10.1084/jem.20100587
134. Dal-Secco D, Wang J, Zeng Z, Kolaczkowska E, Wong CH, Petri B, et al. A dynamic spectrum of monocytes arising from the in situ reprogramming of CCR2+ monocytes at a site of sterile injury. J Exp Med. (2015) 212:447–56. doi: 10.1084/jem.20141539
135. Ikeda N, Asano K, Kikuchi K, Uchida Y, Ikegami H, Takagi R, et al. Emergence of immunoregulatory Ym1+Ly6Chi monocytes during recovery phase of tissue injury. Sci Immunol. (2018) 3:eaat0207. doi: 10.1126/sciimmunol.aat0207
136. Ogle ME, Segar CE, Sridhar S, Botchwey EA. Monocytes and macrophages in tissue repair: Implications for immunoregenerative biomaterial design. Exp Biol Med. (2016) 241:1084–97. doi: 10.1177/1535370216650293
137. Speiser DE, Ho PC, Verdeil G. Regulatory circuits of T cell function in cancer. Nat Rev Immunol. (2016) 16:599–611. doi: 10.1038/nri.2016.80
138. Fidler IJ. The pathogenesis of cancer metastasis: the ‘seed and soil’ hypothesis revisited. Nat Rev Cancer. (2003) 3:453–8. doi: 10.1038/nrc1098
139. Thurairajah K, Broadhead ML, Balogh ZJ. Trauma and stem cells: biology and potential therapeutic implications. Int J Mol Sci. (2017) 18:E577. doi: 10.3390/ijms18030577
140. Rennert RC, Sorkin M, Garg RK, Gurtner GC. Stem cell recruitment after injury: lessons for regenerative medicine. Regen Med. (2012) 7:833–50. doi: 10.2217/rme.12.82
141. Henrich D, Zimmer S, Seebach C, Frank J, Barker J, Marzi I. Trauma-activated polymorphonucleated leukocytes damage endothelial progenitor cells: probable role of CD11b/CD18-CD54 interaction and release of reactive oxygen species. Shock. (2011) 36:216–22. doi: 10.1097/SHK.0b013e3182236eba
142. Han Z, Jing Y, Zhang S, Liu Y, Shi Y, Wei L. The role of immunosuppression of mesenchymal stem cells in tissue repair and tumor growth. Cell Biosci. (2012) 2:8. doi: 10.1186/2045-3701-2-8
143. Qi K, Li N, Zhang Z, Melino G. Tissue regeneration: the crosstalk between mesenchymal stem cells and immune response. Cell Immunol. (2018) 326:86–93. doi: 10.1016/j.cellimm.2017.11.010
144. Ren G, Zhang L, Zhao X, Xu G, Zhang Y, Roberts AI, et al. Mesenchymal stem cell-mediated immunosuppression occurs via concerted action of chemokines and nitric oxide. Cell Stem Cell. (2008) 2:141–50. doi: 10.1016/j.stem.2007.11.014
145. Ren G, Su J, Zhang L, Zhao X, Ling W, L'huillie A, et al. Species variation in the mechanisms of mesenchymal stem cell-mediated immunosuppression. Stem Cells. (2009) 27:1954–62. doi: 10.1002/stem.118
146. Jitschin R, Bottcher M, Saul D, Lukassen S, Bruns H, Loschinski R, et al. Inflammation-induced glycolytic switch controls suppressivity of mesenchymal stem cells via STAT1 glycosylation. Leukemia. (2019) 33:1783–96. doi: 10.1038/s41375-018-0376-6
147. Ma S, Xie N, Li W, Yuan B, Shi Y, Wang Y. Immunobiology of mesenchymal stem cells. Cell Death Differ. (2014) 21:216–25. doi: 10.1038/cdd.2013.158
148. Luz-Crawford P, Jorgensen C, Djouad F. Mesenchymal stem cells direct the immunological fate of macrophages. Results Probl Cell Differ. (2017) 62:61–72. doi: 10.1007/978-3-319-54090-0_4
149. Cheung TS, Dazzi F. Mesenchymal-myeloid interaction in the regulation of immunity. Semin Immunol. (2018) 35:59–68. doi: 10.1016/j.smim.2018.01.002
150. Zheng YH, Deng YY, Lai W, Zheng SY, Bian HN, Liu ZA, et al. Effect of bone marrow mesenchymal stem cells on the polarization of macrophages. Mol Med Rep. (2018) 17:4449–59. doi: 10.3892/mmr.2018.8457
151. Cho DI, Kim MR, Jeong HY, Jeong HC, Jeong MH, Yoon SH, et al. Mesenchymal stem cells reciprocally regulate the M1/M2 balance in mouse bone marrow-derived macrophages. Exp Mol Med. (2014) 46:e70. doi: 10.1038/emm.2013.135
152. Nauta AJ, Kruisselbrink AB, Lurvink E, Willemze R, Fibbe WE. Mesenchymal stem cells inhibit generation and function of both CD34+-derived and monocyte-derived dendritic cells. J Immunol. (2006) 177:2080–7. doi: 10.4049/jimmunol.177.4.2080
153. Spaggiari GM, Abdelrazik H, Becchetti F, Moretta L. MSCs inhibit monocyte-derived DC maturation and function by selectively interfering with the generation of immature DCs: central role of MSC-derived prostaglandin E2. Blood. (2009) 113:6576–83. doi: 10.1182/blood-2009-02-203943
154. Nemeth K, Leelahavanichkul A, Yuen PS, Mayer B, Parmelee A, Doi K, Robey PG, et al. Bone marrow stromal cells attenuate sepsis via prostaglandin E(2)-dependent reprogramming of host macrophages to increase their interleukin-10 production. Nat Med. (2009) 15:42–9. doi: 10.1038/nm.1905
155. Luz-Crawford P, Djouad F, Toupet K, Bony C, Franquesa M, Hoogduijn MJ, et al. Mesenchymal stem cell-derived interleukin 1 receptor antagonist promotes macrophage polarization and inhibits B cell differentiation. Stem Cells. (2016) 34:483–92. doi: 10.1002/stem.2254
156. Deng Y, Zhang Y, Ye L, Zhang T, Cheng J, Chen G, et al. Umbilical cord-derived mesenchymal stem cells instruct monocytes towards an IL10-producing phenotype by secreting IL6 and HGF. Sci Rep. (2016) 6:37566. doi: 10.1038/srep37566
157. Melief SM, Geutskens SB, Fibbe WE, Roelofs H. Multipotent stromal cells skew monocytes towards an anti-inflammatory interleukin-10-producing phenotype by production of interleukin-6. Haematologica. (2013) 98:888–95. doi: 10.3324/haematol.2012.078055
158. Huen SC, Huynh L, Marlier A, Lee Y, Moeckel GW, Cantley LG. GM-CSF Promotes macrophage alternative activation after renal ischemia/reperfusion injury. J Am Soc Nephrol. (2015) 26:1334–45. doi: 10.1681/ASN.2014060612
159. Qian BZ, Pollard JW. Macrophage diversity enhances tumor progression and metastasis. Cell. (2010) 141:39–51. doi: 10.1016/j.cell.2010.03.014
160. Mantovani A, Marchesi F, Malesci A, Laghi L, Allavena P. Tumour-associated macrophages as treatment targets in oncology. Nat Rev Clin Oncol. (2017) 14:399–416. doi: 10.1038/nrclinonc.2016.217
161. Franklin RA, Liao W, Sarkar A, Kim MV1, Bivona MR, Liu K, et al. The cellular and molecular origin of tumor-associated macrophages. Science. (2014) 344:921–5. doi: 10.1126/science.1252510
162. De Veirman K, Van Ginderachter JA, Lub S, De Beule N, Thielemans K, Bautmans I, et al. Multiple myeloma induces Mcl-1 expression and survival of myeloid-derived suppressor cells. Oncotarget. (2015) 6:10532–47. doi: 10.18632/oncotarget.3300
163. Takahashi T, Kuniyasu Y, Toda M, Sakaguchi N, Itoh M, Iwata M, et al. Immunologic self-tolerance maintained by CD25+CD4+ naturally anergic and suppressive T cells: induction of autoimmune disease by breaking their anergic/suppressive state. Int Immunol. (1998) 10:1969–80. doi: 10.1093/intimm/10.12.1969
164. Soroosh P, Doherty TA, Duan W, Mehta AK, Choi H, Adams YF, et al. Lung-resident tissue macrophages generate Foxp3+ regulatory T cells and promote airway tolerance. J Exp Med. (2013) 210:775–88. doi: 10.1084/jem.20121849
165. Duan W, Croft M. Control of regulatory T cells and airway tolerance by lung macrophages and dendritic cells. Ann Am Thorac Soc. (2014) 11:S306–13. doi: 10.1513/AnnalsATS.201401-028AW
166. Tu L, Chen J, Zhang H, Duan L. nterleukin-4 inhibits regulatory T cell differentiation through regulating CD103+ dendritic cells. Front Immunol. (2017) 8:214. doi: 10.3389/fimmu.2017.00214
167. Hoechst B, Ormandy LA, Ballmaier M, Lehner F, Kruger C, Manns MP, et al. A new population of myeloid-derived suppressor cells in hepatocellular carcinoma patients induces CD4(+)CD25(+)Foxp3(+) T cells. Gastroenterology. (2008) 135:234–43. doi: 10.1053/j.gastro.2008.03.020
168. Hoechst B, Gamrekelashvili J, Manns MP, Greten TF, Korangy F. Plasticity of human Th17 cells and iTregs is orchestrated by different subsets of myeloid cells. Blood. (2011) 117:6532–41. doi: 10.1182/blood-2010-11-317321
169. Serafini P, Mgebroff S, Noonan K, Borrello I. Myeloid-derived suppressor cells promote cross-tolerance in B-cell lymphoma by expanding regulatory T cells. Cancer Res. (2008) 68:5439–49. doi: 10.1158/0008-5472.CAN-07-6621
170. Huang B, Pan PY, Li Q, Sato AI, Levy DE, Bromberg J, et al. Gr-1+CD115+ immature myeloid suppressor cells mediate the development of tumor-induced T regulatory cells and T-cell anergy in tumor-bearing host. Cancer Res. (2006) 66:1123–31. doi: 10.1158/0008-5472.CAN-05-1299
171. Collado M, Serrano M. Senescence in tumours: evidence from mice and humans. Nat Rev Cancer. (2010) 10:51–7. doi: 10.1038/nrc2772
172. Lujambio A, Akkari L, Simon J, Grace D, Tschaharganeh DF, Bolden JE, et al. Non-cell-autonomous tumor suppression by p53. Cell. (2013) 153:449–60. doi: 10.1016/j.cell.2013.03.020
173. Eggert T, Wolter K, Ji J, Ma C, Yevsa T, Klotz S, et al. Distinct functions of senescence-associated immune responses in liver tumor surveillance and tumor progression. Cancer Cell. (2016) 30:533–47. doi: 10.1016/j.ccell.2016.09.003
174. Lechner MG, Megiel C, Russell SM, Bingham B, Arger N, Woo T, et al. Functional characterization of human Cd33+ and Cd11b+ myeloid-derived suppressor cell subsets induced from peripheral blood mononuclear cells co-cultured with a diverse set of human tumor cell lines. J Transl Med. (2011) 9:90. doi: 10.1186/1479-5876-9-90
175. Lechner MG, Liebertz DJ, Epstein AL. Characterization of cytokine-induced myeloid-derived suppressor cells from normal human peripheral blood mononuclear cells. J Immunol. (2010) 185:2273–84. doi: 10.4049/jimmunol.1000901
176. Fisher DT, Appenheimer MM1, Evans SS. The two faces of IL-6 in the tumor microenvironment. Semin Immunol. (2014) 26:38–47. doi: 10.1016/j.smim.2014.01.008
177. Bhattacharya P, Budnick I, Singh M, Thiruppathi M, Alharshawi K, Elshabrawy H, et al. Dual role of GM-CSF as a pro-inflammatory and a regulatory cytokine: implications for immune therapy. J Interferon Cytokine Res. (2015) 35:585–99. doi: 10.1089/jir.2014.0149
178. Hong IS. Stimulatory versus suppressive effects of GM-CSF on tumor progression in multiple cancer types. Exp Mol Med. (2016) 48:e242. doi: 10.1038/emm.2016.64
179. Bayne LJ, Beatty GL, Jhala N, Clark CE, Rhim AD, et al. Tumor-derived granulocyte-macrophage colony-stimulating factor regulates myeloid inflammation and T cell immunity in pancreatic cancer. Cancer Cell. (2012) 21:822–35. doi: 10.1016/j.ccr.2012.04.025
180. Pylayeva-Gupta Y, Lee KE, Hajdu CH, Miller G, Bar-Sagi D. Oncogenic Kras-induced GM-CSF production promotes the development of pancreatic neoplasia. Cancer Cell. (2012) 21:836–47. doi: 10.1016/j.ccr.2012.04.024
181. Min L, Mohammad Isa SA, Shuai W, Piang CB, Nih FW, Kotaka M, et al. Cutting edge: granulocyte-macrophage colony-stimulating factor is the major CD8+ T cell-derived licensing factor for dendritic cell activation. J Immunol. (2010) 184:4625–9. doi: 10.4049/jimmunol.0903873
182. Lutz MB1, Suri RM, Niimi M, Ogilvie AL, Kukutsch NA, Rössner S, et al. Immature dendritic cells generated with low doses of GM-CSF in the absence of IL-4 are maturation resistant and prolong allograft survival in vivo. Eur J Immunol. (2000) 30:1813–22. doi: 10.1002/1521-4141(200007)30:7<1813::AID-IMMU1813>3.0.CO;2-8
183. Bhattacharya P, Gopisetty A, Ganesh BB, Sheng JR, Prabhakar BS. GM-CSF-induced, bone-marrow-derived dendritic cells can expand natural Tregs and induce adaptive Tregs by different mechanisms. J Leukoc Biol. (2011) 89:235–49. doi: 10.1189/jlb.0310154
184. Marigo I, Bosio E, Solito S, Mesa C, Fernandez A, Dolcetti L, et al. Tumor-induced tolerance and immune suppression depend on the C/EBPbeta transcription factor. Immunity. (2010) 32:790–802. doi: 10.1016/j.immuni.2010.05.010
185. Hammami I, Chen J, Murschel F, Bronte V, De Crescenzo G, Jolicoeur M. Immunosuppressive activity enhances central carbon metabolism and bioenergetics in myeloid-derived suppressor cells in vitro models. BMC Cell Biol. (2012) 13:18. doi: 10.1186/1471-2121-13-18
186. Wu WC, Sun HW, Chen HT, Liang J, Yu XJ, Wu C, et al. Circulating hematopoietic stem and progenitor cells are myeloid-biased in cancer patients. Proc Natl Acad Sci USA. (2014) 111:4221–6. doi: 10.1073/pnas.1320753111
187. Casacuberta-Serra S, Pares M, Golbano A, Coves E, Espejo C, Barquinero J. Myeloid-derived suppressor cells can be efficiently generated from human hematopoietic progenitors and peripheral blood monocytes. Immunol Cell Biol. (2017) 95:538–48. doi: 10.1038/icb.2017.4
188. Tomić S, Joksimović B, Bekić M, Vasiljević M, Milanović M, Colić M, et al. Prostaglanin-E2 potentiates the suppressive functions of human mononuclear myeloid-derived suppressor cells and increases their capacity to expand IL-10-producing regulatory T cell subsets. Front Immunol. (2019) 10:475. doi: 10.3389/fimmu.2019.00475
189. Kurko J, Vida A, Ocsko T, Tryniszewska B, Rauch TA, Glant TT, et al. Suppression of proteoglycan-induced autoimmune arthritis by myeloid-derived suppressor cells generated in vitro from murine bone marrow. PLoS ONE. (2014) 9:e111815. doi: 10.1371/journal.pone.0111815
190. Obermajer N, Muthuswamy R, Lesnock J, Edwards RP, Kalinski P. Positive feedback between PGE2 and COX2 redirects the differentiation of human dendritic cells toward stable myeloid-derived suppressor cells. Blood. (2011) 118:5498–505. doi: 10.1182/blood-2011-07-365825
191. Hsieh CC, Lin CL, He JT, Chiang M, Wang Y, Tsai YC, et al. Administration of cytokine-induced myeloid-derived suppressor cells ameliorates renal fibrosis in diabetic mice. Stem Cell Res Ther. (2018) 9:183. doi: 10.1186/s13287-018-0915-0
192. Bharadwaj U, Li M, Zhang R, Chen C, Yao Q. Elevated interleukin-6 and G-CSF in human pancreatic cancer cell conditioned medium suppress dendritic cell differentiation and activation. Cancer Res. (2007) 67:5479–88. doi: 10.1158/0008-5472.CAN-06-3963
193. Zhu XD, Zhang JB, Zhuang PY, Zhu HG, Zhang W, Xiong YQ, et al. High expression of macrophage colony-stimulating factor in peritumoral liver tissue is associated with poor survival after curative resection of hepatocellular carcinoma. J Clin Oncol. (2008) 26:2707–16. doi: 10.1200/JCO.2007.15.6521
194. Okumoto K, Hattori E, Tamura K, Kiso S, Watanabe H, Saito K, et al. Possible contribution of circulating transforming growth factor-beta1 to immunity and prognosis in unresectable hepatocellular carcinoma. Liver Int. (2004) 24:21–8. doi: 10.1111/j.1478-3231.2004.00882.x
195. Poon RT, Ho JW, Tong CS, Lau C, Ng IO, Fan ST. Prognostic significance of serum vascular endothelial growth factor and endostatin in patients with hepatocellular carcinoma. Br J Surg. (2004) 91:1354–60. doi: 10.1002/bjs.4594
196. Miura S, Mitsuhashi N, Shimizu H, Kimura F, Yoshidome H, Otsuka M, et al. Fibroblast growth factor 19 expression correlates with tumor progression and poorer prognosis of hepatocellular carcinoma. BMC Cancer. (2012) 12:56. doi: 10.1186/1471-2407-12-56
197. Chau GY, Lui WY, Chi CW, Chau YP, Li AF, Kao HL, et al. Significance of serum hepatocyte growth factor levels in patients with hepatocellular carcinoma undergoing hepatic resection. Eur J Surg Oncol. (2008) 34:333–8. doi: 10.1016/j.ejso.2006.12.007
198. Farajzadeh Valilou S, Keshavarz-Fathi M, Silvestris N, Argentiero A, Rezaei N. The role of inflammatory cytokines and tumor associated macrophages (TAMs) in microenvironment of pancreatic cancer. Cytokine Growth Factor Rev. (2018) 39:46–61. doi: 10.1016/j.cytogfr.2018.01.007
199. Cao Y, Luetkens T, Kobold S, Hildebrandt Y, Gordic M, Lajmi N, et al. The cytokine/chemokine pattern in the bone marrow environment of multiple myeloma patients. Exp Hematol. (2010) 38:860–7. doi: 10.1016/j.exphem.2010.06.012
200. Birmann BM, Neuhouser ML, Rosner B, Albanes D, Buring JE, Giles GG, et al. Prediagnosis biomarkers of insulin-like growth factor-1, insulin, and interleukin-6 dysregulation and multiple myeloma risk in the Multiple Myeloma Cohort Consortium. Blood. (2012) 120:4929–37. doi: 10.1182/blood-2012-03-417253
201. Pappa CA, Tsirakis G, Psarakis FE, Kolovou A, Tsigaridaki M, Stafylaki D, et al. Lack of correlation between angiogenic cytokines and serum insulin-like growth factor-1 in patients with multiple myeloma. Med Oncol. (2013) 30:363. doi: 10.1007/s12032-012-0363-0
202. Montag M, Dyckhoff G, Lohr J, Helmke BM, Herrmann E, Plinkert PK, et al. Angiogenic growth factors in tissue homogenates of HNSCC: expression pattern, prognostic relevance, and interrelationships. Cancer Sci. (2009) 100:1210–8. doi: 10.1111/j.1349-7006.2009.01158.x
203. Lyon DE, McCain NL, Walter J, Schubert C. Cytokine comparisons between women with breast cancer and women with a negative breast biopsy. Nurs Res. (2008) 57:51–8. doi: 10.1097/01.NNR.0000280655.58266.6c
204. Yurkovetsky ZR, Kirkwood JM, Edington HD, Marrangoni AM, Velikokhatnaya L, Winans MT, et al. Multiplex analysis of serum cytokines in melanoma patients treated with interferon-alpha2b. Clin Cancer Res. (2007) 13:2422–8. doi: 10.1158/1078-0432.CCR-06-1805
205. Capone F, Guerriero E, Sorice A, Colonna G, Ciliberto G, Costantini S. Serum cytokinome profile evaluation: a tool to define new diagnostic and prognostic markers of cancer using multiplexed bead-based immunoassays. Mediators Inflamm. (2016) 2016:3064643. doi: 10.1155/2016/3064643
206. Coussens LM, Zitvogel L, Palucka AK. Neutralizing tumor-promoting chronic inflammation: a magic bullet? Science. (2013) 339:286–91. doi: 10.1126/science.1232227
207. Atretkhany KN, Drutskaya MS. Myeloid-derived suppressor cells and proinflammatory cytokines as targets for cancer therapy. Biochemistry. (2016) 81:1274–83. doi: 10.1134/S0006297916110055
208. Yu H, Pardoll D, Jove R. STATs in cancer inflammation and immunity: a leading role for STAT3. Nat Rev Cancer. (2009) 9:798–809. doi: 10.1038/nrc2734
209. Rébé C, Végran F, Berger H, Ghiringhelli F. STAT3 activation: a key factor in tumor immunoescape. JAKSTAT. (2013) 2:e23010. doi: 10.4161/jkst.23010
210. Su YL, Banerjee S, White SV, Kortylewski M. STAT3 in tumor-associated myeloid cells: multitasking to disrupt immunity. Int J Mol Sci. (2018) 19:E1803. doi: 10.3390/ijms19061803
211. Dai J, Kumbhare A, Youssef D, Yao ZQ, McCall CE, El Gazzar M. Expression of C/EBPβ in myeloid progenitors during sepsis promotes immunosuppression. Mol Immunol. (2017) 91:165–72. doi: 10.1016/j.molimm.2017.09.008
212. McClure C, McPeak MB, Youssef D, Yao ZQ, McCall CE, El Gazzar M. Stat3 and C/EBPβ synergize to induce miR-21 and miR-181b expression during sepsis. Immunol Cell Biol. (2017) 95:42–55. doi: 10.1038/icb.2016.63
213. Zhang H, Nguyen-Jackson H, Panopoulos AD, Li HS, Murray PJ, Watowich SS. STAT3 controls myeloid progenitor growth during emergency granulopoiesis. Blood. (2010) 116:2462–71. doi: 10.1182/blood-2009-12-259630
214. Kaneda MM, Messer KS, Ralainirina N, Li H, Leem CJ, Gorjestani S, et al. PI3Kγ is a molecular switch that controls immune suppression. Nature. (2016) 539:437–42. doi: 10.1038/nature19834
215. Chen W, Ma T, Shen XN, Xia XF, Xu GD, Bai XL, et al. Macrophage-induced tumor angiogenesis is regulated by the TSC2-mTOR pathway. Cancer Res. (2012) 72:1363–72. doi: 10.1158/0008-5472.CAN-11-2684
216. Biswas SK, Lewis CE. NF-κB as a central regulator of macrophage function in tumors. J Leukoc Biol. (2010) 88:877–84. doi: 10.1189/jlb.0310153
217. Hagemann T, Biswas SK, Lawrence T, Sica A, Lewis CE. Regulation of macrophage function in tumors: the multifaceted role of NF-kappaB. Blood. (2009) 113:3139–46. doi: 10.1182/blood-2008-12-172825
218. Panzer U, Steinmetz OM, Turner JE, Meyer-Schwesinger C, von Ruffer C, Meyer TN, et al. Resolution of renal inflammation: a new role for NF-kappaB1 (p50) in inflammatory kidney diseases. Am J Physiol Renal Physiol. (2009) 297:F429–39. doi: 10.1152/ajprenal.90435.2008
219. Saccani A, Schioppa T, Porta C, Biswas SK, Nebuloni M, Vago L, et al. p50 nuclear factor-kappaB overexpression in tumor-associated macrophages inhibits M1 inflammatory responses and antitumor resistance. Cancer Res. (2006) 66:11432–40. doi: 10.1158/0008-5472.CAN-06-1867
220. Porta C, Rimoldi M, Raes G, Brys L, Ghezzi P, Di Liberto D, et al. Tolerance and M2 (alternative) macrophage polarization are related processes orchestrated by p50 nuclear factor kappaB. Proc Natl Acad Sci USA. (2009) 106:14978–83. doi: 10.1073/pnas.0809784106
221. Strauss L, Sangaletti S, Consonni FM, Szebeni G, Morlacchi S, Totaro MG, et al. RORC1 regulates tumor-promoting “Emergency” granulo-monocytopoiesis. Cancer Cell. (2015) 28:253–69. doi: 10.1016/j.ccell.2015.07.006
222. Pello OM, De Pizzol M, Mirolo M, Soucek L, Zammataro L, Amabile A, et al. Role of c-MYC in alternative activation of human macrophages and tumor-associated macrophage biology. Blood. (2012) 119:411–21. doi: 10.1182/blood-2011-02-339911
223. Negishi H, Ohba Y, Yanai H, Takaoka A, Honma K, Yui K, et al. Negative regulation of Toll-like-receptor signaling by IRF-4. Proc Natl Acad Sci USA. (2005) 102:15989–94. doi: 10.1073/pnas.0508327102
Keywords: monocytes, macrophages, hypothesis, inflammation, growth factors, immunosuppression, tumor, wound healing
Citation: Ponomarev AV and Shubina IZ (2019) Insights Into Mechanisms of Tumor and Immune System Interaction: Association With Wound Healing. Front. Oncol. 9:1115. doi: 10.3389/fonc.2019.01115
Received: 17 July 2019; Accepted: 07 October 2019;
Published: 25 October 2019.
Edited by:
Armin Ghobadi, Washington University in St. Louis, United StatesReviewed by:
Amorette Barber, Longwood University, United StatesLuis De La Cruz-Merino, Hospital Universitario Virgen Macarena, Spain
Copyright © 2019 Ponomarev and Shubina. This is an open-access article distributed under the terms of the Creative Commons Attribution License (CC BY). The use, distribution or reproduction in other forums is permitted, provided the original author(s) and the copyright owner(s) are credited and that the original publication in this journal is cited, in accordance with accepted academic practice. No use, distribution or reproduction is permitted which does not comply with these terms.
*Correspondence: Aleksandr V. Ponomarev, a2w4NTQ2JiN4MDAwNDA7eWFuZGV4LnJ1