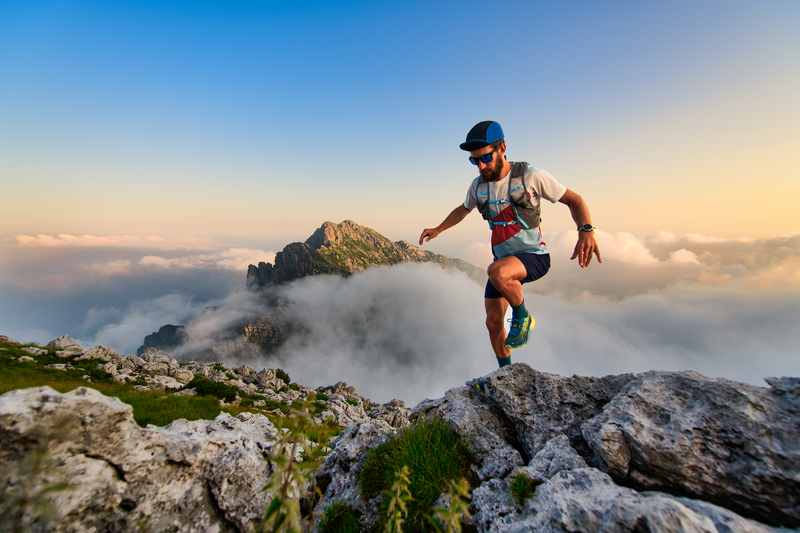
94% of researchers rate our articles as excellent or good
Learn more about the work of our research integrity team to safeguard the quality of each article we publish.
Find out more
REVIEW article
Front. Oncol. , 27 August 2019
Sec. Molecular and Cellular Oncology
Volume 9 - 2019 | https://doi.org/10.3389/fonc.2019.00799
This article is part of the Research Topic Cell Signaling Mediating Critical Radiation Responses View all 15 articles
Repair of DNA damage protects genomic integrity, which is key to tissue functional integrity. In cancer, the type and fidelity of DNA damage response is the fundamental basis for clinical response to cytotoxic therapy. Here we consider the contribution of transforming growth factor-beta (TGFβ), a ubiquitous, pleotropic cytokine that is abundant in the tumor microenvironment, to therapeutic response. The action of TGFβ is best illustrated in head and neck squamous cell carcinoma (HNSCC). Survival of HNSCC patients with human papilloma virus (HPV) positive cancer is more than double compared to those with HPV-negative HNSCC. Notably, HPV infection profoundly impairs TGFβ signaling. HPV blockade of TGFβ signaling, or pharmaceutical TGFβ inhibition that phenocopies HPV infection, shifts cancer cells from error-free homologous-recombination DNA double-strand-break (DSB) repair to error-prone alternative end-joining (altEJ). Cells using altEJ are more sensitive to standard of care radiotherapy and cisplatin, and are sensitized to PARP inhibitors. Hence, HPV-positive HNSCC is an experiment of nature that provides a strong rationale for the use of TGFβ inhibitors for optimal therapeutic combinations that improve patient outcome.
DNA repair is executed by multiple pathways that must be coordinated to deal with different types of DNA damage, including oxidative damage, single strand breaks (SSB), and double strand breaks (DSB). Complex intracellular mechanisms have developed to ensure an appropriate DNA damage response (DDR). In cancer, gene mutations and altered cell signaling can give rise to dysregulated and aberrant DNA repair mechanisms that presumably contribute to genomic instability and mutational burden that are associated with cancer progression.
Cancer cells are actively involved in crosstalk with host cells of the tumor microenvironment (TME), which includes the vasculature, immune cells and stroma, constitutes a robust but skewed signaling network distinct from normal tissue. Though the TME is critical in shaping the biology of a tumor, the impact of context-related signaling on the tumor cell's DNA repair proficiency is poorly understood. This article reviews cell intrinsic execution of DSB repair proficiency and pathway competency to discuss DNA repair in the context of the TME.
We focus on transforming growth factor-beta (TGFβ), which is critically involved in extrinsic control of pathway competency in DSB repair. Recently, we determined that compromised TGFβ signaling caused by human papilloma virus (HPV) in head and neck squamous cell carcinoma (HNSCC) shifts DSB repair to error prone and inefficient alternative end-joining (altEJ). HPV is an experiment of nature that provides compelling evidence that signaling from TGFβ, and thus the TME, is critical for DNA repair execution and pathway choice. The insight gained from understanding of the mechanisms by which TGFβ signaling, DDR, and TME are functionally linked, paves the way to further exploit weakness in specific cancers and develop pertinent therapeutic strategies.
Tens of thousands of DNA lesions are produced in a cell's daily life as a result of endogenous metabolic activities such as DNA replication or reactive oxygen species (ROS), as well as exposure to exogenous agents like ultraviolet (UV) or ionizing radiation (1, 2). Of the many types of DNA damage, DSBs are among the most dangerous. Failure to repair DSBs may lead to mutations, genomic, and chromosomal rearrangements, or cell death. In order to maintain genomic integrity, two predominant DSB repair pathways have developed to deal with different types of lesions: classical non-homologous end-joining (c-NHEJ) and homologous recombination repair (HRR) [Figure 1; (3)]. In mammalian cells, c-NHEJ is the predominant DSB repair pathway that can efficiently rejoin most DSBs (4). Although c-NHEJ functions throughout the cell cycle, it is particularly critical in the G1 phase when the cell has yet to replicate its DNA (5, 6). C-NHEJ is initiated by the binding of the Ku70/Ku80 heterodimer to the ends of the DSB, which is then recognized with high affinity by the catalytic subunit of DNA-dependent protein kinase (DNA-PKcs), forming the DNA-PK complex (7). This enables the recruitment of nucleases, including Artemis, to trim any short overhangs that are present on the DSB ends (8), and polymerases, including Polμ, to fill in any gaps (9–11). The final ligation step involves a ligase complex comprised of DNA ligase IV, X-ray repair cross-complementing protein 4 (XRCC4), and XRCC4-like factor (XLF), which is responsible for bridging and ligating the two processed ends (12). Because DSB ends often require processing to remove damaged nucleotides to enable ligation, c-NHEJ is considered an error-prone form of repair that can lead to short insertions and/or deletions. However, growing evidence suggests the context under which c-NHEJ is used in DSB repair is a critical determinant of the repair outcome, i.e., error-prone or error-prevention (13, 14). Although it awaits further evidence, it has been speculated that orchestrated repair with c-NHEJ in normal cells prevents chromosome instability, but in cancer cells with dysregulated repair pathways, inappropriate implementation of c-NHEJ-dependent end joining of non-contiguous ends can cause genomic alterations and lead to chromosome instability (13, 14).
Figure 1. DSB repair pathways. Once a DSB is induced by a DNA damaging agent, cancer cells will try to repair it by one of these three mechanisms. Critical repair proteins in each pathway are shown.
The repair of DSBs by HRR is a highly complex process that requires the generation of single-stranded 3′ overhangs at each end, after which the homologous sequence on the sister chromatid is used to accurately fill in the gaps and restore the original DNA sequence (15, 16). In the first step of HRR, the DSB ends are recognized by the MRN complex, which is composed of meiotic recombination 11 homolog A (MRE11), RAD50, and Nijmegen breakage syndrome 1 (NBS1). Once bound to the DSB, the MRN complex activates ataxia telangiectasia mutated (ATM), a serine-threonine kinase that initiates the DDR by phosphorylating a plethora of substrates, thus facilitating the recognition of the DSB and the activation of downstream repair factors. In addition to its role in the MRN complex, MRE11 also associates with C-terminal binding protein interacting protein (CtIP), an endonuclease that allows for the removal of ~100 nucleotides from the 3′ end of the DSB (17, 18).
Following the initial processing step by MRE11 and CtIP, more extensive resection is subsequently mediated by either the EXO1 exonuclease or a combination of the RECQ helicase (BLM or RECQL4) and the DNA2 exonuclease (19). The single-stranded DNA formed by these resection steps is very quickly coated with and stabilized by replication protein A (RPA), preventing them from being degraded or forming DNA hairpins (20). For the final phase of HRR, RPA is replaced with RAD51 to facilitate homology search, strand invasion into the sister chromatid, and initiation of DNA synthesis at the region of the DSB (21). This process requires several proteins known as recombination mediators, e.g., the tumor suppressor breast cancer 1, early onset 2 (BRCA2) (22, 23). Repair is then completed upon resolution of crossover junctions by resolvases (24). Because HRR requires a homologous template, it can only be used to repair DNA that has been replicated (i.e., during the S and G2 phases of the cell cycle).
A third mechanism for repair of DSBs is altEJ, which is also referred to as backup end joining, or microhomology-mediated end joining (25, 26). A comparison of repair strategies is depicted in Figure 1. AltEJ uses poly [ADP-ribose] polymerase 1 (PARP1) to tether broken DNA ends (27), DNA polymerase theta (Pol θ) coded by the POLQ gene to initiate DNA replication at sites within two single-stranded 3′ overhangs (28), and DNA ligase I (LIG1) or DNA ligase III (LIG3) to join the DNA ends (29). AltEJ commonly occurs at sites containing short complementary sequences, known as microhomology, that are exposed after end resection; this requirement for resection and minimal homology means that altEJ has low fidelity and therefore frequently results in small deletions, insertions, and gross chromosomal rearrangements (30, 31). Because its execution increases genomic instability, altEJ is believed to be more active in certain cancers (32).
Other DSB repair pathways, such as single-strand annealing (SSA), can result in large deletions during repair by annealing of longer (e.g., >100 nt) repeats following extensive end-resection. These are rarely used in mammalian cells and have been reviewed recently (24), and will not be discussed herein.
The mechanism by which DSB are repaired is determined by a variety of factors, although the outcome is ultimately determined by the presence or absence of end resection. The initial phase of c-NHEJ, i.e., binding of the Ku heterodimer to DSB ends, minimizes end resection to allow accurate end-joining. End processing and resection are therefore tightly regulated by Ku70/Ku80, along with WRN and 53BP1, which together protect DNA ends during the G1 phase when HRR cannot occur due to the absence of a sister chromatid. Resection is also normally limited to late S or G2 due to the cell-cycle dependent expression of CtIP and its activation by CDK1 or CDK2 (33, 34). Importantly, resection requires the repositioning of 53BP1 on DSB ends by BRCA1, and therefore the loss of BRCA1 inhibits HRR, which was demonstrated by the fact that a deficiency in 53BP1 rescues the defect in HRR caused by the absence of BRCA1 (35). Noordermeer et al. recently demonstrated that 53BP1 effector complex, shieldin, localizes to DSB to prioritize c-NHEJ repair (36). In BRCA1-deficient cells, loss of shieldin or its subunits can restore HRR and resistance to PARP inhibition (37).
AltEJ was initially believed to be a backup pathway for c-NHEJ and HRR (26). The Ku heterodimer has much higher affinity for DSB ends relative to PARP1; thus, c-NHEJ is highly favored over altEJ in most circumstances (38). A higher frequency of altEJ-mediated repair was observed after the depletion of HRR factors such as RPA, BRCA1, and BRCA2 (39), suggesting HRR is used with priority in normal settings. In addition, because both HRR and altEJ require an initial resection step at DSB ends, both pathways are inhibited by c-NHEJ factors. Conversely, end resection is sufficient to block repair by c-NHEJ, as Ku70/Ku80 has very low affinity for single stranded DNA (40). Notably, accumulating evidence suggests that altEJ also competes with HRR for the repair of DSB (28, 41). For example, by studying dysfunctional telomeres and accumulation of RAD51 at DSBs, Mateos-Gomez and co-authors found that the loss of a critical component in altEJ, Pol θ, increased HRR in mice (28). Similar findings have been reported in ovarian cancers: HRR was upregulated when Pol θ expression was inhibited, while Pol θ expression blocks RAD51-mediated HRR due to RAD51 binding motifs in Pol θ (41).
Cell cycle phase plays an important role in DSB repair pathway choice. In S and G2 phases, HRR is preferentially used to repair DSB due to the presence of CYREN, an inhibitor of c-NHEJ (42). AltEJ is largely inactive in normal cells, but in quickly dividing cancer cells, altEJ may be increased to handle the increased level of DNA damage and, as a result, generate more mutations as by-products. Although the cell cycle dependency of altEJ is not clear, it is possible that HRR-deficient cells use altEJ mainly in S or G2 phases, while c-NHEJ defects may increase altEJ in G1 phase. In addition, host cell type, chromosomal location, and epigenetic modification are also important factors for pathway competence at DSBs, which has been reviewed elsewhere (43).
Many DNA repair genes are tumor suppressors, and are frequently mutated during tumor progression. Loss of functions in some DNA repair genes increases compensating mechanisms of repair that may expose a targetable “Achilles heel.” Synthetic lethality, which is defined as a cytotoxic response to the loss or inhibition of a gene or pathway that only happens in the presence of another specific genetic deficit, can specifically target cancer cells containing a defect in a DNA repair gene. The clinical application of synthetic lethality is best exemplified using PARP inhibitors in cells that have germ line or somatic mutations in BRCA1 or BRCA2 (44, 45). PARP inhibition results in the accumulation of single-strand breaks (SSB) that produce DSBs upon collision with a DNA replication fork, which require HRR to repair. This is one mechanism by which BRCA1/2-deficient cancers are highly sensitized to PARP inhibition, although other functions of PARP may contribute as well. For example, PARP is directly involved in detoxifying endogenous ROS (46, 47), while BRCA1 down-regulates cellular levels of ROS (48). Thus, BRCA deficient cells may be more sensitive to increased cytotoxic ROS that result from PARP inhibition. Although the clinical benefits of PARP inhibition are clear in BRCA1/2-deficient cancers, tumors with HRR defects in the absence of BRCA gene mutations (i.e., BRCAness) are also responsive to PARP inhibition (49). Hence, mutation of genes that are directly or indirectly involved in HRR or the Fanconi anemia pathway, a DNA repair pathway that intersects HRR, can contribute to so-called BRCAness, i.e., tumors that behave as if BRCA1 or BRCA2 are mutated (49). Biomarkers to assess BRCAness, which include transcriptional signatures, genomic scars, or levels of Rad51 foci, are under intensive investigation (50–52).
Besides its key role in SSB repair, PARP1 is also a critical component of altEJ; thus, PARP inhibition can cause synthetic lethality in tumors that rely on altEJ (25). Other altEJ components are promising therapeutic targets for tumors that depend on this repair pathway. Indeed Pol θ -mediated end joining becomes critical when either HRR or c-NHEJ fails. A recent genetic screen reported that both Pol θ and another altEJ component, the structure-specific endonuclease FEN1, are synthetic lethal with BRCA2 (53). The potential synergy of HRR and altEJ is indicated by embryonic lethality of combined loss of Brca1/Fancd2 and Polq in mice (28, 41). Depletion of Pol θ in human cancer cells deficient in HRR due to absence of BRCA1/2 increases chromosomal aberrations and impaired cell survival (28). Pol θ loss also increases HRR-impaired cells sensitivity to DNA-damage (41).
Moreover, there is growing evidence that defects in HRR can lead to increased dependence on altEJ for repair, particularly in the context of fork stalling during replication stress (26, 54). In addition to its relationship with HRR, altEJ may also be synthetic lethal with c-NHEJ. Combined deletion of POLQ and Ku70, as well as POLQ and 53BP1, leads to markedly reduced cell proliferation and survival associated with excessive end resection and chromosomal aberrations (55). Notably, POLQ is highly expressed in a subset of cancer types (56, 57) and its expression is associated with poor prognosis (58, 59).
Cancer cells react to endogenous (e.g., replication stress) or exogenous (e.g., radiation, chemotherapy) DNA damage in the context of their TME. The TME plays an important role in determining cancer clinical behavior and progression, and can influence cancer cell response to therapy (60, 61). Components of the TME include cellular constituents of bone-marrow derived cells, fibroblasts and vessels, insoluble extracellular matrix, and soluble cytokines and chemokines (62). These TME components closely collaborate with cancer cells for development of a neoplastic phenotype. In this “team,” frequent interactions and crosstalk in a complicated signaling network unite them as a whole. Better understanding of “tumor as a whole” could provide information about the optimal use of therapies, and improve the development of personalized therapy based on integrated features of a tumor derived from both cancer cells and TME composition.
The influence of TME conditions on DNA repair is complex. Although DNA repair is largely regulated through autonomous signaling cascades, tissue-wide stress responses from DNA damage may be networked among tumor cells, stromal cells, and other TME components. TME composition influences DNA repair efficiency by transmitting inter- and intra-cellular signals in a tissue-specific fashion. Many TME factors, which include cytokines, extracellular matrix, stromal cells, hypoxia, and inflammation, are known to modulate DNA repair efficiency (63–68).
Here we focus on TGFβ, a highly pleiotropic cytokine and a canonical tumor suppressor. Because TGFβ suppresses epithelial cell cycle progression, all carcinomas must escape TGFβ growth regulation (69). Notably, high TGFβ expression and signaling is associated with poor prognosis in multiple cancer types (52, 70) because TGFβ becomes a tumor promoter that is involved in tumor progression by modifying the TME, suppressing immune response, and promoting metastasis (71). Due to these critical functions and association with poor outcomes, TGFβ is an intriguing therapeutic target in clinical trials (72, 73).
Understanding the biology of TGFβ is rooted in understanding when and where it is active. There are three mammalian TGFβ isoforms, each encode a polypeptide that is cleaved intracellularly to form a roughly 24 kD TGFβ that is non-covalently associated with an 80 kD dimer of its pre-pro peptide, called latency associated peptide (LAP). This complex, TGFβ and LAP, is secreted as the latent TGFβ complex and is often sequestered in forms bound to extracellular matrix. TGFβ canonical signaling is initiated upon the release of TGFβ ligand from its latent complex, and subsequent binding to the type II TGFβ receptor (TβRII), which causes recruitment and phosphorylation of type I receptor (TβRI). Activated TβRI kinase phosphorylates the carboxy-terminal serine residue of the mothers against DPP Homolog proteins SMAD2 or SMAD3, which induces oligomerization of SMAD2 or SMAD3 with SMAD4, and DNA binding of the complex to mediate transcriptional activation or repression of target genes. TGFβ also transduces signals through non-canonical signaling pathways, such as MAPK/ERK, PI3K/AKT, Rho/Rock. Activation of TGFβ is highly controlled in normal tissues. In cancers, TGFβ signaling is highly dysregulated (70, 74, 75). Defective TGFβ signaling, which can be caused by mutations in SMAD4 and TGFBR2, are frequent in certain types of cancer (52, 73).
Both cancer cells and stromal cells produce TGFβ that may, when activated, elicit paracrine or autocrine signaling to stimulate fibroblasts, endothelial cells and immune cells that further alter the TME. Moreover, TGFβ is a potent immunosuppressive cytokine involved in shaping TME by inhibiting the activation and function of T cells (76, 77), inducing immune suppressive myeloid cells (78), as well as by other multifaceted mechanisms (79–81). These complex interactions are just a few of TGFβ's roles in the TME.
An unexpected role for TGFβ, an intrinsically extracellular signal, is response to intracellular DNA damage. Glick et al. (82) was likely the first study to implicate TGFβ in the cellular response to DNA damage. They showed that Tgfb1 null murine keratinocytes were highly genomically unstable, independent of G1 arrest and p53 function (82). Consistent with a role of TGFβ in maintaining genomic stability, Maxwell et al. (83) demonstrated more centrosome aberrations and aneuploidy in irradiated Tgfb1-null compared to TGFβ-competent keratinocytes. Notably, this effect is phenocopied by TGFβ inhibition in human epithelial cells (83). In a subsequent study, Glick and colleagues revealed a DNA repair deficit due to hypermethylated O(6)-methylguanine DNA methyltransferase (MGMT) that affected the DDR of Tgfb1-null keratinocytes (84). Similarly, Kirshner et al. (85) showed that inhibiting TGFβ signaling attenuates DDR by compromising the function of ATM, the DDR kinase involved in DSB recognition (85). Consequently, pharmaceutical inhibition of TβRI kinase or knockout of Tgfb1 reduces ATM autophosphorylation and phosphorylation of its substrates, e.g., p53, Chk2, and Rad17, inhibits formation of radiation-induced γH2AX foci, and increases radiosensitivity (85). In support of this, Wiegman et al. (86) showed that exogenous TGFβ stimulates ATM and p53 phosphorylation in irradiated cells in a SMAD-independent fashion (86). Notably, TGFβ inhibition also reduces LIG4 expression, which is required in c-NHEJ (87).
Nucleotide excision repair (NER) is a versatile DNA repair pathway that eliminates a wide variety of helix-distorting base lesions induced by environmental carcinogenic sources. UVB radiation downregulates E-cadherin, a cell adhesion protein, in mouse skin and skin tumors whereas inhibiting the TGFβ pathway in these cells increases the NER of UV-induced DNA damage (88). E-cadherin inhibition in keratinocytes suppresses NER through activating the TGF-β pathway and increasing TGFβ1 mRNA levels. Interestingly, TGFβ is activated by ionizing radiation and in turn, promotes epithelial-mesenchymal transition characterized by loss of E-cadherin (89). Treatment of cells with exogenous TGFβ enhances NER of DNA damage formed by polycyclic aromatic hydrocarbons and UVC radiation independent of the cell cycle (90).
Consistent with role of TGFβ in DDR, the SMAD proteins, which are the critical transducers of TGFβ intracellular signaling, are involved in DSB repair. Both pSmad2 and Smad7 can co-localize with nuclear γH2AX foci at DSB, while pSmad2 foci formation is ATM dependent (91). Studies in Smad4 conditional knockout mice confirmed that TGFβ is critical in maintaining genomic stability through regulation of genes in the Fanconi anemia/BRCA DNA repair pathway (92). SMAD4 suppresses a micro RNA, miR182, which inhibits FOXO3, which is required for ATM kinase activity (32, 52). MiR182 also suppresses BRCA1 expression (93, 94). Hence, TGFβ signaling through SMAD4 promotes HRR in part by suppressing miR182 (52). C-NHEJ is also partially compromised because LIG4 expression and ATM activity are reduced once TGFβ signaling is blocked (85, 87).
Although tumors must evade TGFβ growth control, at the time of clinical appearance, many tumors maintain signaling competency. Indeed, squamous cell carcinomas may take advantage of TGFβ signaling to maintain a sub-population of cells at a quiescent state for chemo-resistance (95). Moreover, the high TGFβ activity of TME could promote tumor-intrinsic resistance to cytotoxic agents due to its role in DNA damage recognition and repair. If so, pharmacological TGFβ blockade could sensitize certain tumors to radiation and other cytotoxic therapies. Exploration of brain, breast, and lung cancer pre-clinical models is consistent with this concept, since TGFβ inhibition radiosensitized 38 of 43 murine and human cancer cell lines in vitro (85, 96–98). Because TGFβ provides extrinsic control of several aspects of intracellular DNA repair pathway competency, one prediction is that tumors that are insensitive to TGFβ can be exploited by targeting their deficiency in DNA repair.
The contribution of TGFβ signaling as a barrier compromising therapeutic response to cytotoxic therapy is exemplified by HPV-positive (HPV+) HNSCC (52), which have much better (70%) survival at 5 years compared to HPV-negative (HPV-) cancers that attain only 30% survival, even when HNSCC location and stage are similar. The considerable difference in outcomes has stimulated significant interest based on the idea that the mechanism of sensitivity of HPV+ cancer to standard-of-care chemoradiation therapy, which could provide insights that can be therapeutically exploited to achieve better response in HPV- cancer.
Consistent with a cell-intrinsic effect, HPV+ cancer cell lines exhibit altered expression of DNA repair proteins (99) and increased sensitivity to cytotoxic therapy (100, 101). Most research has focused on oncogenic impairment of p53 and retinoblastoma (Rb) proteins by HPV E6 or E7, respectively. However, HPV proteins E5, E6, and E7 target both the type I and II TGFβ receptors and SMAD 2, 3, and 4, the transducers of ligand binding, for degradation (102, 103). We examined the impact of HPV on TGFβ signaling in HNSCC at multiple levels. Functionally, TGFβ induced phosphorylation of SMAD2 (pSMAD), indicative of signaling competency, is significantly reduced in HPV+ cell line, patient derived xenografts, and primary tumor explants, compared to HPV− specimens (52). Notably, HPV+ specimens in a HNSCC tumor array with 130 HPV− and 65 HPV+ samples exhibit less pSMAD compared to HPV− specimens. In addition, TCGA HPV+ tumors are identified by low activity (243 HPV− vs. 36 HPV+ ones) of a TGFβ pathway signature, which contains 50 TGFβ-regulated genes that were derived from epithelial cells chronically stimulated or inhibited for the corresponding pathway.
Interestingly, Wang and colleagues engineered a conditional Smad4 deletion in oral mucosa that gave rise to spontaneous HNSCC accompanied by high rates of genomic instability (92). Subsequent studies by this group showed that Smad4 deletion leads to decreased Brca1 expression in mice and that loss of SMAD4 protein correlates with decreased BRCA1 and RAD51 proteins in human HNSCC. BRCA1 is crucial for HRR during S-phase/G2, acts upon the cell cycle machinery, and affects gene expression and cell fate decisions via chromatin remodeling and transcriptional activity (104). Wang and colleagues showed that BRCA1 is transcriptionally down-regulated by SMAD4-dependent CtPB1 (105). A second, more direct route by which TGFβ controls of BRCA1 is via TGFβ suppression of miR-182, which targets BRCA1 message stability and translation in mouse and human cells. Thus, pharmacologically or genetically compromised TGFβ increase levels of miR-182 and consequently suppress BRCA1 (94).
Consistent with our earlier studies in breast, lung and brain cancer cells (85, 96–98), HPV+ HNSCC cell lines were more sensitive to radiation than HPV− cell lines (52). Indeed, the degree of pSMAD response to TGFβ and cellular radiosensitivity are highly correlated. Radiation sensitivity reflects the cumulative damage and inherent capacity to repair the damage based on the cells ability to recognize DNA damage, assemble the repair machinery, and execute repair; abrogation of any of these components decreases cell survival. As noted above, BRCA1 is critical for HRR-mediated DNA repair. HRR requires RAD51 binding to 3′-single-stranded DNA overhangs from processed DSB and strand pairing; thus, the formation of RAD51 foci is evidence of HRR. Significantly, fewer RAD51 foci are formed in HPV- HNSCC cells and tumor specimens if TGFβ is pharmacologically blocked, which is not observed in HPV+ HNSCC cells.
A deficiency in HRR should increase the proportion of cells that are killed in response to PARPi. As predicted, TGFβ-unresponsive HPV+ cell lines are more sensitive to olaparib alone compared to TGFβ-responsive HPV- cancer cells, which were sensitized 4-fold by TGFβ inhibition (52).
Loss of effective HRR can activate altEJ, which competes with HRR for repair of DSBs in S-phase (41) and/or acts as backup repair when HRR or c-NHEJ are compromised (26). As mentioned earlier, altEJ requires PARP1 and Pol θ, the product of the POLQ gene (28). To evaluate altEJ, we established TGFβ-responsive cells with a reporter construct detecting altEJ events (106). As expected, a specific PARP1 inhibitor reduced altEJ events, while TGFβ inhibitors significantly increased altEJ events.
Thus, either pharmaceutical blockade of TGFβ signaling in HPV− cells or intrinsic-defects in TGFβ signaling in HPV+ cells shifts DSB repair to altEJ (Figure 2). This shift may result from decreased implementation of HRR and c-NHEJ, or may indicate an increase in altEJ competency. Notably, BRCA1 heterozygous cells exhibit preferential use of altEJ (107), suggesting that the decrease in BRCA1 that occurs upon loss of TGFβ signaling phenocopies the genetic BRCA1 loss. As c-NHEJ is also partially compromised because LIG4 expression and ATM activity are reduced (85, 87), cells with deficient TGFβ signaling may increase altEJ to compensate for a deficiency in both HRR and c-NHEJ.
Figure 2. Defective TGFβ signaling in HNSCC increases altEJ (52). (A) TGFβ signaling suppresses miR182, which suppresses expression of BRCA1 and FOXO3. DNA damage elicits activates ATM autophosphorylation and its phosphorylation of BRCA1, which gives rise to use of HRR in S-phase. (B) In contrast, deficient TGFβ signaling, which can be caused by HPV infection, TGFβ receptor kinase inhibitors (TBRi) or SMAD4 mutations (SMAD4 mut), leads to increased miR182 that suppresses BRCA1 and FOXO3. Loss of FOXO3 inhibits ATM auto-activation, which together with decreased BRCA1, impedes HRR. This is accompanied by increased altEJ.
TGFβ-unresponsive cells that depend on altEJ are still capable of rejoining most DSBs, which hinders the maximal cytotoxic response to DNA damage. As mentioned above, recent studies demonstrate that Pol θ is required in altEJ (28, 41). HRR-deficient ovarian and breast cancers exhibit increased POLQ expression, perhaps indicative of altEJ (41). Consistent with this, POLQ expression is increased in HPV+ vs. HPV− HNSCC TCGA (52). Notably, POLQ shRNA expressing HPV− cells treated with TGFβ inhibitors were more sensitive DNA damage, supporting the idea that Pol θ-mediated altEJ is increasingly used when TGFβ signaling is abrogated. These data suggest that combining altEJ inhibitors with pharmaceutical blockade of TGFβ signaling would induce synthetic lethality, thus creating a novel route that could boost the sensitivity of TGFβ-active tumors to therapies that involve DNA damage. Moreover, since altEJ is a backup mechanism that operates preferentially in cancer cells, inhibiting altEJ may pre-dominantly sensitize tumor cells and spare normal cells. Alternatively, one might select cancers that are defective in TGFβ to target drugs that interfere with altEJ, since these drugs will both improve the treatment effectiveness and the toxicity profile.
The importance of alt-EJ in TGFβ-deficient cells discovered in HNSCC raises several questions. We are following this lead to determine if the same mechanism is evident in other cancers in which TGFβ signaling is compromised. Almost all cervical cancers are HPV positive, which we would expect to show similar DDR choices as HNSCC. However, TGFβ signaling is compromised by various mechanisms in many cancers (108). Our analysis across the spectrum of cancers suggest that there is probably a generic mechanism (unpublished). We also find that the repair shift to altEJ in TGFβ-deficient cells occurs independent of miR-182. Further studies are necessary to decipher the underlying mechanisms.
Although most evidence supports TGFβ as a factor enforcing DNA-repair proficiency to protect against genomic instability, conflicting evidence about the role of TGFβ in DDR also exists (109–112). For example, Pal et al. reported that TGFβ hinders DSB repair in cancer stem cells by reducing HRR gene expression, which was proposed to heighten genetic diversity and adaptability of cancer stem cells (112). This subpopulation may regulate DDR differently, but it is interesting that in another study, glioblastoma cancer stem cells make 5-fold more TGFβ than bulk cultures (97). Considering that TGFβ is a pleotropic cytokine, the extensive technical and conditional differences in these studies may lead to a different conclusion.
For example, TGFβ signaling induces squamous cell carcinoma cancer stem cells quiescence, which would be expected to affect repair pathway competency in a manner that contributes to chemo-resistance (95). However, many cancer cells have escaped TGFβ cell growth control, as stated above. For example, in our HNSCC study, the cell cycle was not significantly changed upon TGFβ stimulation or inhibition of HNSCC cell lines (52), which indicates that TGFβ can regulate DNA repair pathway by mechanisms independent of cell cycle effects.
Intensive investigation of the TME has advanced our knowledge about the tumor as a whole, whereas in-depth analysis of DDR now provides mechanisms of DNA repair strategies and their implementation in cancer cells. How DDR is executed in different tumors is an area of growing complexity. The highly heterogeneous TME is a function of cancer cell genetics, epigenetics, host cell composition that result in complicated signaling networks. However, opportunities also lie in these challenges. DDR-related signaling pathways regulated by the TME components may contain biomarkers for cancer stratification, as well as molecular or cellular targets for drug development, which deserves significant investigation.
QL, JM, and MB-H wrote the review. TH and KL reviewed and revised the manuscript.
This research was supported by UCSF Department of Radiation Oncology funds to MB-H.
The authors declare that the research was conducted in the absence of any commercial or financial relationships that could be construed as a potential conflict of interest.
1. Lindahl T, Barnes DE. Repair of endogenous DNA damage. Cold Spring Harb Symp Quant Biol. (2000) 65:127–33. doi: 10.1101/sqb.2000.65.127
2. Tubbs A, Nussenzweig A. Endogenous DNA damage as a source of genomic instability in cancer. Cell. (2017) 168:644–56. doi: 10.1016/j.cell.2017.01.002
3. Krejci L, Altmannova V, Spirek M, Zhao X. Homologous recombination and its regulation. Nucleic Acids Res. (2012) 40:5795–818. doi: 10.1093/nar/gks270
4. Beucher A, Birraux J, Tchouandong L, Barton O, Shibata A, Conrad S, et al. ATM and Artemis promote homologous recombination of radiation-induced DNA double-strand breaks in G2. EMBO J. (2009) 28:3413–27. doi: 10.1038/emboj.2009.276
5. Takata M, Sasaki MS, Sonoda E, Morrison C, Hashimoto M, Utsumi H, et al. Homologous recombination and non-homologous end-joining pathways of DNA double-strand break repair have overlapping roles in the maintenance of chromosomal integrity in vertebrate cells. EMBO J. (1998) 17:5497–508. doi: 10.1093/emboj/17.18.5497
6. Bozzella M, Seluanov A, Gorbunova V. DNA repair by nonhomologous end joining and homologous recombination during cell cycle in human cells AU - Mao, Zhiyong. Cell Cycle. (2008) 7:2902–6. doi: 10.4161/cc.7.18.6679
7. Meek K, Dang V, Lees-Miller SP. DNA-PK: the means to justify the ends? Adv Immunol. (2008) 99:33–58. doi: 10.1016/S0065-2776(08)00602-0
8. Stiff T, Walker SA, Cerosaletti K, Goodarzi AA, Petermann E, Concannon P, et al. ATR-dependent phosphorylation and activation of ATM in response to UV treatment or replication fork stalling. Embo J. (2006) 25:5775–82. doi: 10.1038/sj.emboj.7601446
9. Bebenek K, Pedersen LC, Kunkel TA. Structure-function studies of DNA polymerase lambda. Biochemistry. (2014) 53:2781–92. doi: 10.1021/bi4017236
10. Moon AF, Pryor JM, Ramsden DA, Kunkel TA, Bebenek K, Pedersen LC. Sustained active site rigidity during synthesis by human DNA polymerase mu. Nat Struct Mol Biol. (2014) 21:253–60. doi: 10.1038/nsmb.2766
11. Pryor JM, Conlin MP, Carvajal-Garcia J, Luedeman ME, Luthman AJ, Small GW, et al. Ribonucleotide incorporation enables repair of chromosome breaks by nonhomologous end joining. Science. (2018) 361:1126–9. doi: 10.1126/science.aat2477
12. Ahnesorg P, Smith P, Jackson SP. XLF interacts with the XRCC4-DNA ligase IV complex to promote DNA nonhomologous end-joining. Cell. (2006) 124:301–13. doi: 10.1016/j.cell.2005.12.031
13. Betermier M, Bertrand P, Lopez BS. Is non-homologous end-joining really an inherently error-prone process? PLoS Genet. (2014) 10:e1004086. doi: 10.1371/journal.pgen.1004086
14. Sishc BJ, Davis AJ. The role of the core non-homologous end joining factors in carcinogenesis and cancer. Cancers. (2017) 9:E81. doi: 10.3390/cancers9070081
15. Powell SN, Kachnic LA. Roles of BRCA1 and BRCA2 in homologous recombination, DNA replication fidelity and the cellular response to ionizing radiation. Oncogene. (2003) 22:5784–91. doi: 10.1038/sj.onc.1206678
16. Li X, Heyer WD. Homologous recombination in DNA repair and DNA damage tolerance. Cell Res. (2008) 18:99–113. doi: 10.1038/cr.2008.1
17. Sartori AA, Lukas C, Coates J, Mistrik M, Fu S, Bartek J, et al. Human CtIP promotes DNA end resection. Nature. (2007) 450:509–14. doi: 10.1038/nature06337
18. Zhu Z, Chung WH, Shim EY, Lee SE, Ira G. Sgs1 helicase and two nucleases Dna2 and Exo1 resect DNA double-strand break ends. Cell. (2008) 134:981–94. doi: 10.1016/j.cell.2008.08.037
19. Nimonkar AV, Genschel J, Kinoshita E, Polaczek P, Campbell JL, Wyman C, et al. BLM-DNA2-RPA-MRN and EXO1-BLM-RPA-MRN constitute two DNA end resection machineries for human DNA break repair. Genes Dev. (2011) 25:350–62. doi: 10.1101/gad.2003811
20. Chen H, Lisby M, Symington LS. RPA coordinates DNA end resection and prevents formation of DNA hairpins. Mol Cell. (2013) 50:589–600. doi: 10.1016/j.molcel.2013.04.032
21. Langberg CW, Hauer-Jensen M, Sung C-C, Kane CJM. Expression of fibrogenic cytokines in rat small intestine after fractionated irradiation. Radiother Oncol. (1994) 32:29–36. doi: 10.1016/0167-8140(94)90446-4
22. Yang H, Jeffrey PD, Miller J, Kinnucan E, Sun Y, Thoma NH, et al. BRCA2 function in DNA binding and recombination from a BRCA2-DSS1-ssDNA structure. Science. (2002) 297:1837–48. doi: 10.1126/science.297.5588.1837
23. Lord CJ, Ashworth A. PARP inhibitors: synthetic lethality in the clinic. Science. (2017) 355:1152–8. doi: 10.1126/science.aam7344
24. Jasin M, Rothstein R. Repair of strand breaks by homologous recombination. Cold Spring Harb Perspect Biol. (2013) 5:a012740. doi: 10.1101/cshperspect.a012740
25. Frit P, Barboule N, Yuan Y, Gomez D, Calsou P. Alternative end-joining pathway(s): bricolage at DNA breaks. DNA Repair. (2014) 17:81–97. doi: 10.1016/j.dnarep.2014.02.007
26. Iliakis G, Murmann T, Soni A. Alternative end-joining repair pathways are the ultimate backup for abrogated classical non-homologous end-joining and homologous recombination repair: Implications for the formation of chromosome translocations. Mutat Res Genet Toxicol Environ Mutagen. (2015) 793:166–75. doi: 10.1016/j.mrgentox.2015.07.001
27. Robert I, Dantzer F, Reina-San-Martin B. Parp1 facilitates alternative NHEJ, whereas Parp2 suppresses IgH/c-myc translocations during immunoglobulin class switch recombination. J Exp Med. (2009) 206:1047–56. doi: 10.1084/jem.20082468
28. Mateos-Gomez PA, Gong F, Nair N, Miller KM, Lazzerini-Denchi E, Sfeir A. Mammalian polymerase theta promotes alternative NHEJ and suppresses recombination. Nature. (2015) 518:254–7. doi: 10.1038/nature14157
29. Li X, Lu Y, Liang K, Pan T, Mendelsohn J, Fan Z. Requirement of hypoxia-inducible factor-1alpha down-regulation in mediating the antitumor activity of the anti-epidermal growth factor receptor monoclonal antibody cetuximab. Mol Cancer Ther. (2008) 7:1207–17. doi: 10.1158/1535-7163.MCT-07-2187
30. Ferguson DO, Sekiguchi JM, Chang S, Frank KM, Gao Y, Depinho RA, et al. The nonhomologous end-joining pathway of DNA repair is required for genomic stability and the suppression of translocations. Proc Natl Acad Sci USA. (2000) 97:6630–3. doi: 10.1073/pnas.110152897
31. Simsek D, Jasin M. Alternative end-joining is suppressed by the canonical NHEJ component Xrcc4-ligase IV during chromosomal translocation formation. Nat Struct Mol Biol. (2010) 17:410–6. doi: 10.1038/nsmb.1773
32. Tsai W-B, Chung YM, Takahashi Y, Xu Z, Hu MCT. Functional interaction between FOXO3a and ATM regulates DNA damage response. Nat Cell Biol. (2008) 10:460–7. doi: 10.1038/ncb1709
33. Yu X, Chen J. DNA damage-induced cell cycle checkpoint control requires CtIP, a phosphorylation-dependent binding partner of BRCA1 C-terminal domains. Mol Cell Biol. (2004) 24:9478–86. doi: 10.1128/MCB.24.21.9478-9486.2004
34. Chen L, Nievera CJ, Lee AY, Wu X. Cell cycle-dependent complex formation of BRCA1.CtIP.MRN is important for DNA double-strand break repair. J Biol Chem. (2008) 283:7713–20. doi: 10.1074/jbc.M710245200
35. Isono M, Niimi A, Oike T, Hagiwara Y, Sato H, Sekine R, et al. BRCA1 directs the repair pathway to homologous recombination by promoting 53BP1 dephosphorylation. Cell Rep. (2017) 18:520–32. doi: 10.1016/j.celrep.2016.12.042
36. Noordermeer SM, Adam S, Setiaputra D, Barazas M, Pettitt SJ, Ling AK, et al. The shieldin complex mediates 53BP1-dependent DNA repair. Nature. (2018) 560:117–21. doi: 10.1038/s41586-018-0340-7
37. Gupta R, Somyajit K, Narita T, Maskey E, Stanlie A, Kremer M, et al. DNA Repair network analysis reveals shieldin as a key regulator of NHEJ and PARP inhibitor sensitivity. Cell. (2018) 173:972–988.e923. doi: 10.1016/j.cell.2018.03.050
38. Paddock MN, Bauman AT, Higdon R, Kolker E, Takeda S, Scharenberg AM. Competition between PARP-1 and Ku70 control the decision between high-fidelity and mutagenic DNA repair. DNA Repair. (2011) 10:338–43. doi: 10.1016/j.dnarep.2010.12.005
39. Ahrabi S, Sarkar S, Pfister SX, Pirovano G, Higgins GS, Porter AC, et al. A role for human homologous recombination factors in suppressing microhomology-mediated end joining. Nucleic Acids Res. (2016) 44:5743–57. doi: 10.1093/nar/gkw326
40. Dynan WS, Yoo S. Interaction of Ku protein and DNA-dependent protein kinase catalytic subunit with nucleic acids. Nucleic Acids Res. (1998) 26:1551–9. doi: 10.1093/nar/26.7.1551
41. Ceccaldi R, Liu JC, Amunugama R, Hajdu I, Primack B, Petalcorin MI, et al. Homologous-recombination-deficient tumours are dependent on Poltheta-mediated repair. Nature. (2015) 518:258–62. doi: 10.1038/nature14184
42. Arnoult N, Correia A, Ma J, Merlo A, Garcia-Gomez S, Maric M, et al. Regulation of DNA repair pathway choice in S and G2 phases by the NHEJ inhibitor CYREN. Nature. (2017) 549:548–52. doi: 10.1038/nature24023
43. Kakarougkas A, Jeggo PA. DNA DSB repair pathway choice: an orchestrated handover mechanism. Br J Radiol. (2014) 87:20130685. doi: 10.1259/bjr.20130685
44. Bryant HE, Schultz N, Thomas HD, Parker KM, Flower D, Lopez E, et al. Specific killing of BRCA2-deficient tumours with inhibitors of poly(ADP-ribose) polymerase. Nature. (2005) 434:913–7. doi: 10.1038/nature03443
45. Farmer H, Mccabe N, Lord CJ, Tutt AN, Johnson DA, Richardson TB, et al. Targeting the DNA repair defect in BRCA mutant cells as a therapeutic strategy. Nature. (2005) 434:917–21. doi: 10.1038/nature03445
46. Liu Q, Gheorghiu L, Drumm M, Clayman R, Eidelman A, Wszolek MF, et al. PARP-1 inhibition with or without ionizing radiation confers reactive oxygen species-mediated cytotoxicity preferentially to cancer cells with mutant TP53. Oncogene. (2018) 37:2793–805. doi: 10.1038/s41388-018-0130-6
47. Marcar L, Bardhan K, Gheorghiu L, Dinkelborg P, Pfaffle H, Liu Q, et al. Acquired resistance of EGFR-mutated lung cancer to tyrosine kinase inhibitor treatment promotes PARP inhibitor sensitivity. Cell Rep. (2019) 27:3422–32.e3424. doi: 10.1016/j.celrep.2019.05.058
48. Saha T, Rih JK, Rosen EM. BRCA1 down-regulates cellular levels of reactive oxygen species. FEBS Lett. (2009) 583:1535–43. doi: 10.1016/j.febslet.2009.04.005
49. Lord CJ, Ashworth A. BRCAness revisited. Nat Rev Cancer. (2016) 16:110–20. doi: 10.1038/nrc.2015.21
50. Willers H, Gheorghiu L, Liu Q, Efstathiou JA, Wirth LJ, Krause M, et al. DNA damage response assessments in human tumor samples provide functional biomarkers of radiosensitivity. Semin Radiat Oncol. (2015) 25:237–50. doi: 10.1016/j.semradonc.2015.05.007
51. Ganguly B, Dolfi SC, Rodriguez-Rodriguez L, Ganesan S, Hirshfield KM. Role of biomarkers in the development of PARP inhibitors. Biomark Cancer. (2016) 8:15–25. doi: 10.4137/BIC.S36679
52. Liu Q, Ma L, Jones T, Palomero L, Pujana MA, Martinez-Ruiz H, et al. Subjugation of TGFb signaling by human papilloma virus in head and neck squamous cell carcinoma shifts DNA repair from homologous recombination to alternative end joining. Clin Cancer Res. (2018) 24:6001–14. doi: 10.1158/1078-0432.CCR-18-1346
53. Mengwasser KE, Adeyemi RO, Leng Y, Choi MY, Clairmont C, D'andrea AD, et al. Genetic screens reveal FEN1 and APEX2 as BRCA2 synthetic lethal targets. Mol Cell. (2019) 73:885–99.e6. doi: 10.1016/j.molcel.2018.12.008
54. Garcia-Closas M, Couch FJ, Lindstrom S, Michailidou K, Schmidt MK, Brook MN, et al. (2013). Genome-wide association studies identify four ER negative-specific breast cancer risk loci. Nat Genet. 45:392–8. doi: 10.1038/ng.2561
55. Wyatt DW, Feng W, Conlin MP, Yousefzadeh MJ, Roberts SA, Mieczkowski P, et al. Essential roles for polymerase theta-mediated end joining in the repair of chromosome breaks. Mol Cell. (2016) 63:662–73. doi: 10.1016/j.molcel.2016.06.020
56. Kawamura K, Bahar R, Seimiya M, Chiyo M, Wada A, Okada S, et al. DNA polymerase theta is preferentially expressed in lymphoid tissues and upregulated in human cancers. Int J Cancer. (2004) 109:9–16. doi: 10.1002/ijc.11666
57. Lemee F, Bergoglio V, Fernandez-Vidal A, Machado-Silva A, Pillaire MJ, Bieth A, et al. DNA polymerase theta up-regulation is associated with poor survival in breast cancer, perturbs DNA replication, and promotes genetic instability. Proc Natl Acad Sci USA. (2010) 107:13390–5. doi: 10.1073/pnas.0910759107
58. Higgins GS, Harris AL, Prevo R, Helleday T, Mckenna WG, Buffa FM. Overexpression of POLQ confers a poor prognosis in early breast cancer patients. Oncotarget. (2010) 1:175–84. doi: 10.18632/oncotarget.124
59. Allera-Moreau C, Rouquette I, Lepage B, Oumouhou N, Walschaerts M, Leconte E, et al. DNA replication stress response involving PLK1, CDC6, POLQ, RAD51 and CLASPIN upregulation prognoses the outcome of early/mid-stage non-small cell lung cancer patients. Oncogenesis. (2012) 1:e30. doi: 10.1038/oncsis.2012.29
60. Chan N, Koch CJ, Bristow RG. Tumor hypoxia as a modifier of DNA strand break and cross-link repair. Curr Mol Med. (2009) 9:401–10. doi: 10.2174/156652409788167050
61. Sun Y, Campisi J, Higano C, Beer TM, Porter P, Coleman I, et al. Treatment-induced damage to the tumor microenvironment promotes prostate cancer therapy resistance through WNT16B. Nat Med. (2012) 18:1359–68. doi: 10.1038/nm.2890
62. Hanahan D, Coussens LM. Accessories to the crime: functions of cells recruited to the tumor microenvironment. Cancer Cell. (2012) 21:309–22. doi: 10.1016/j.ccr.2012.02.022
63. Kumareswaran R, Ludkovski O, Meng A, Sykes J, Pintilie M, Bristow RG. Chronic hypoxia compromises repair of DNA double-strand breaks to drive genetic instability. J Cell Sci. (2012) 125:189–99. doi: 10.1242/jcs.092262
64. Kidane D, Chae WJ, Czochor J, Eckert KA, Glazer PM, Bothwell AL, et al. Interplay between DNA repair and inflammation, and the link to cancer. Crit Rev Biochem Mol Biol. (2014) 49:116–39. doi: 10.3109/10409238.2013.875514
65. Geiger-Maor A, Guedj A, Even-Ram S, Smith Y, Galun E, Rachmilewitz J. Macrophages regulate the systemic response to DNA damage by a cell nonautonomous mechanism. Cancer Res. (2015) 75:2663–73. doi: 10.1158/0008-5472.CAN-14-3635
66. Scanlon SE, Glazer PM. Multifaceted control of DNA repair pathways by the hypoxic tumor microenvironment. DNA Repair. (2015) 32:180–9. doi: 10.1016/j.dnarep.2015.04.030
67. Centurione L, Aiello FB. DNA repair and cytokines: TGF-beta, IL-6, and thrombopoietin as different biomarkers of radioresistance. Front Oncol. (2016) 6:175. doi: 10.3389/fonc.2016.00175
68. Dickreuter E, Eke I, Krause M, Borgmann K, Van Vugt MA, Cordes N. Targeting of beta1 integrins impairs DNA repair for radiosensitization of head and neck cancer cells. Oncogene. (2016) 35:1353–62. doi: 10.1038/onc.2015.212
69. Derynck R, Akhurst RJ, Balmain A. TGF-b signaling in tumor suppression and cancer progression. Nature Genet. (2001) 29:117–29. doi: 10.1038/ng1001-117
70. Costanza B, Umelo IA, Bellier J, Castronovo V, Turtoi A. Stromal modulators of TGFβ in cancer. J Clin Med. (2017) 6:7. doi: 10.3390/jcm6010007
71. Akhurst RJ. TGFβ antagonists: why suppress a tumor suppressor? J Clin Invest. (2002) 109:1533–6. doi: 10.1172/JCI0215970
72. Akhurst RJ, Hata A. Targeting the TGFbeta signalling pathway in disease. Nat Rev Drug Discov. (2012) 11:790–11. doi: 10.1038/nrd3810
73. Neuzillet C, Tijeras-Raballand A, Cohen R, Cros J, Faivre S, Raymond E, et al. Targeting the TGFbeta pathway for cancer therapy. Pharmacol Ther. (2015) 147:22–31. doi: 10.1016/j.pharmthera.2014.11.001
74. Barcellos-Hoff MH. Latency and activation in the regulation of TGFβ. J Mammary Gland Biol Neoplasia. (1996) 3:353–63. doi: 10.1007/BF02017391
75. Jobling MF, Mott JD, Finnegan MT, Jurukovski V, Erickson AC, Walian PJ, et al. Isoform-specific activation of latent transforming growth factor beta (LTGFβ) by reactive oxygen species. Radiat Res. (2006) 166:839–48. doi: 10.1667/RR0695.1
76. Ghiringhelli F, Menard C, Terme M, Flament C, Taieb J, Chaput N, et al. CD4+CD25+ regulatory T cells inhibit natural killer cell functions in a transforming growth factor-beta-dependent manner. J Exp Med. (2005) 202:1075–85. doi: 10.1084/jem.20051511
77. Da Cunha AP, Wu HY, Rezende RM, Vandeventer T, Weiner HL. In vivo anti-LAP mAb enhances IL-17/IFN-γ responses and abrogates anti-CD3-induced oral tolerance. Int Immunol. (2015) 27:73–82. doi: 10.1093/intimm/dxu083
78. Gonzalez-Junca A, Driscoll KE, Pellicciotta I, Du S, Lo CH, Roy R, et al. Autocrine TGFβ is a survival factor for monocytes and drives immunosuppressive lineage commitment. Cancer Immunol Res. (2019) 7:306–20. doi: 10.1158/2326-6066.CIR-18-0310
79. Li Z, Zhang LJ, Zhang HR, Tian GF, Tian J, Mao XL, et al. Tumor-derived transforming growth factor-β is critical for tumor progression and evasion from immune surveillance. Asian Pac J Cancer Prev. (2014) 15:5181–6. doi: 10.7314/apjcp.2014.15.13.5181
80. Yang L, Moses HL. Transforming growth factor-β: tumor suppressor or promoter? are host immune cells the answer? Cancer Res. (2008) 68:9107–11. doi: 10.1158/0008-5472.CAN-08-2556
81. Wrzesinski SH, Wan YY, Flavell RA. Transforming growth factor-beta and the immune response: implications for anticancer therapy. Clin Cancer Res. (2007) 13:5262–70. doi: 10.1158/1078-0432.CCR-07-1157
82. Glick AB, Weinberg WC, Wu IH, Quan W, Yuspa SH. Transforming growth factor beta 1 suppresses genomic instability independent of a G1 arrest, p53, and Rb. Cancer Res. (1996) 56:3645–50.
83. Maxwell CA, Fleisch MC, Costes SV, Erickson AC, Boissiere A, Gupta R, et al. Targeted and nontargeted effects of ionizing radiation that impact genomic instability. Cancer Res. (2008) 68:8304–11. doi: 10.1158/0008-5472.CAN-08-1212
84. Yamada H, Vijayachandra K, Penner C, Glick A. Increased sensitivity of transforming growth factor (TGF) β1 null cells to alkylating agents reveals a novel link between TGFbeta signaling and O(6)-methylguanine methyltransferase promoter hypermethylation. J Biol Chem. (2001) 276:19052–8. doi: 10.1074/jbc.M100615200
85. Kirshner J, Jobling MF, Pajares MJ, Ravani SA, Glick AB, Lavin MJ, et al. Inhibition of transforming growth factor-beta1 signaling attenuates ataxia telangiectasia mutated activity in response to genotoxic stress. Cancer Res. (2006) 66:10861–9. doi: 10.1158/0008-5472.CAN-06-2565
86. Wiegman EM, Blaese MA, Loeffler H, Coppes RP, Rodemann HP. TGFbeta-1 dependent fast stimulation of ATM and p53 phosphorylation following exposure to ionizing radiation does not involve TGFβ-receptor I signalling. Radiother Oncol. (2007) 83:289–95. doi: 10.1016/j.radonc.2007.05.013
87. Kim MR, Lee J, An YS, Jin YB, Park IC, Chung E, et al. TGFβ1 protects cells from γ-IR by enhancing the activity of the NHEJ repair pathway. Mol Cancer Res. (2015) 13:319–29. doi: 10.1158/1541-7786.MCR-14-0098-T
88. Qiang L, Shah P, Barcellos-Hoff MH, He YY. TGF-β signaling links E-cadherin loss to suppression of nucleotide excision repair. Oncogene. (2016) 35:3293–302. doi: 10.1038/onc.2015.390
89. Andarawewa KL, Erickson AC, Chou WS, Costes SV, Gascard P, Mott JD, et al. Ionizing radiation predisposes nonmalignant human mammary epithelial cells to undergo transforming growth factor b induced epithelial to mesenchymal transition. Cancer Res. (2007) 67:8662–70. doi: 10.1158/0008-5472.CAN-07-1294
90. Zheng H, Jarvis IWH, Bottai M, Dreij K, Stenius U. TGF beta promotes repair of bulky DNA damage through increased ERCC1/XPF and ERCC1/XPA interaction. Carcinogenesis. (2019) 40:580–91. doi: 10.1093/carcin/bgy156
91. Wang M, Saha J, Hada M, Anderson JA, Pluth JM, O'neill P, et al. Novel smad proteins localize to IR-induced double-strand breaks: interplay between TGFβ and ATM pathways. Nucleic Acids Res. (2013) 41:933–42. doi: 10.1093/nar/gks1038
92. Bornstein S, White R, Malkoski S, Oka M, Han G, Cleaver T, et al. Smad4 loss in mice causes spontaneous head and neck cancer with increased genomic instability and inflammation. J Clin Invest. (2009) 119:3408–19. doi: 10.1172/JCI38854
93. Moskwa P, Buffa FM, Pan Y, Panchakshari R, Gottipati P, Muschel RJ, et al. miR-182-mediated downregulation of BRCA1 impacts DNA repair and sensitivity to PARP inhibitors. Mol Cell. (2011) 41:210–20. doi: 10.1016/j.molcel.2010.12.005
94. Martinez-Ruiz H, Illa-Bochaca I, Omene C, Hanniford D, Liu Q, Hernando E, et al. A TGFbeta-miR-182-BRCA1 axis controls the mammary differentiation hierarchy. Sci Signal. (2016) 9:ra118. doi: 10.1126/scisignal.aaf5402
95. Brown JA, Yonekubo Y, Hanson N, Sastre-Perona A, Basin A, Rytlewski JA, et al. TGF-β-induced quiescence mediates chemoresistance of tumor-propagating cells in squamous cell carcinoma. Cell Stem Cell. (2017) 21:650–64.e658. doi: 10.1016/j.stem.2017.10.001
96. Bouquet F, Pal A, Pilones KA, Demaria S, Hann B, Akhurst RJ, et al. TGFb1 inhibition increases the radiosensitivity of breast cancer cells in vitro and promotes tumor control by radiation in vivo. Clin Cancer Res. (2011) 17:6754–65. doi: 10.1158/1078-0432.CCR-11-0544
97. Hardee ME, Marciscano AE, Medina-Ramirez CM, Zagzag D, Narayana A, Lonning SM, et al. Resistance of glioblastoma-initiating cells to radiation mediated by the tumor microenvironment can be abolished by inhibiting transforming growth factor-beta. Cancer Res. (2012) 72:4119–29. doi: 10.1158/0008-5472.CAN-12-0546
98. Du S, Bouquet F, Lo C-H, Pellicciotta I, Bolourchi S, Parry R, et al. Attenuation of the DNA damage response by TGFβ inhibitors enhances radiation sensitivity of NSCLC cells in vitro and in vivo. Int J Radiat Oncol Biol Phys. (2014) 91:91–9. doi: 10.1016/j.ijrobp.2014.09.026
99. Weaver AN, Cooper TS, Rodriguez M, Trummell HQ, Bonner JA, Rosenthal EL, et al. DNA double strand break repair defect and sensitivity to poly ADP-ribose polymerase (PARP) inhibition in human papillomavirus 16-positive head and neck squamous cell carcinoma. Oncotarget. (2015) 6:26995–7007. doi: 10.18632/oncotarget.4863
100. Mirghani H, Amen F, Tao Y, Deutsch E, Levy A. Increased radiosensitivity of HPV-positive head and neck cancers: molecular basis and therapeutic perspectives. Cancer Treat Rev. (2015) 41:844–52. doi: 10.1016/j.ctrv.2015.10.001
101. Ziemann F, Arenz A, Preising S, Wittekindt C, Klussmann JP, Engenhart-Cabillic R, et al. Increased sensitivity of HPV-positive head and neck cancer cell lines to x-irradiation ± Cisplatin due to decreased expression of E6 and E7 oncoproteins and enhanced apoptosis. Am J Cancer Res. (2015) 5:1017–31.
102. Xu Q, Wang S, Xi L, Wu S, Chen G, Zhao Y, et al. Effects of human papillomavirus type 16 E7 protein on the growth of cervical carcinoma cells and immuno-escape through the TGF-beta1 signaling pathway. Gynecol Oncol. (2006) 101:132–9. doi: 10.1016/j.ygyno.2005.09.051
103. Perez-Plasencia C, Vazquez-Ortiz G, Lopez-Romero R, Pina-Sanchez P, Moreno J, Salcedo M. Genome wide expression analysis in HPV16 cervical cancer: identification of altered metabolic pathways. Infect Agent Cancer. (2007) 2:16. doi: 10.1186/1750-9378-2-16
104. Li ML, Greenberg RA. Links between genome integrity and BRCA1 tumor suppression. Trends Biochem Sci. (2012) 37:418–24. doi: 10.1016/j.tibs.2012.06.007
105. Deng Y, Deng H, Liu J, Han G, Malkoski S, Liu B, et al. Transcriptional down-regulation of Brca1 and E-cadherin by CtBP1 in breast cancer. Mol Carcinog. (2012) 51:500–7. doi: 10.1002/mc.20813
106. Bennardo N, Cheng A, Huang N, Stark JM. Alternative-NHEJ is a mechanistically distinct pathway of mammalian chromosome break repair. PLoS Genet. (2008) 4:e1000110. doi: 10.1371/journal.pgen.1000110
107. Bjorkman A, Qvist P, Du L, Bartish M, Zaravinos A, Georgiou K, et al. Aberrant recombination and repair during immunoglobulin class switching in BRCA1-deficient human B cells. Proc Natl Acad Sci USA. (2015) 112:2157–62. doi: 10.1073/pnas.1418947112
108. Korkut A, Zaidi S, Kanchi RS, Rao S, Gough NR, Schultz A, et al. A pan-cancer analysis reveals high-frequency genetic alterations in mediators of signaling by the TGF-β superfamily. Cell Syst. (2018) 7:422–37.e7. doi: 10.1016/j.cels.2018.08.010
109. Kanamoto T, Hellman U, Heldin CH, Souchelnytskyi S. Functional proteomics of transforming growth factor-β1-stimulated Mv1Lu epithelial cells: Rad51 as a target of TGFβ1-dependent regulation of DNA repair. EMBO J. (2002) 21:1219–30. doi: 10.1093/emboj/21.5.1219
110. Liu L, Zhou W, Cheng CT, Ren X, Somlo G, Fong MY, et al. TGFβ induces “BRCAness” and sensitivity to PARP inhibition in breast cancer by regulating DNA-repair genes. Mol Cancer Res. (2014) 12:1597–609. doi: 10.1158/1541-7786.MCR-14-0201
111. Zhang H, Kozono DE, O'connor KW, Vidal-Cardenas S, Rousseau A, Hamilton A, et al. TGF-β inhibition rescues hematopoietic stem cell defects and bone marrow failure in fanconi anemia. Cell Stem Cell. (2016) 18:668–81. doi: 10.1016/j.stem.2016.03.002
Keywords: cancer, TGFβ, DNA repair, tumor microenvironment, genomic integrity, cytotoxic therapy, therapeutic response
Citation: Liu Q, Lopez K, Murnane J, Humphrey T and Barcellos-Hoff MH (2019) Misrepair in Context: TGFβ Regulation of DNA Repair. Front. Oncol. 9:799. doi: 10.3389/fonc.2019.00799
Received: 31 May 2019; Accepted: 06 August 2019;
Published: 27 August 2019.
Edited by:
Sergio Giannattasio, Consiglio Nazionale delle Ricerche, Istituto di Biomembrane, Bioenergetica e Biotecnologie Molecolari (IBIOM), ItalyReviewed by:
Lukas Cermak, Institute of Molecular Genetics (ASCR), CzechiaCopyright © 2019 Liu, Lopez, Murnane, Humphrey and Barcellos-Hoff. This is an open-access article distributed under the terms of the Creative Commons Attribution License (CC BY). The use, distribution or reproduction in other forums is permitted, provided the original author(s) and the copyright owner(s) are credited and that the original publication in this journal is cited, in accordance with accepted academic practice. No use, distribution or reproduction is permitted which does not comply with these terms.
*Correspondence: Mary Helen Barcellos-Hoff, bWFyeWhlbGVuLmJhcmNlbGxvcy1ob2ZmQHVjc2YuZWR1
Disclaimer: All claims expressed in this article are solely those of the authors and do not necessarily represent those of their affiliated organizations, or those of the publisher, the editors and the reviewers. Any product that may be evaluated in this article or claim that may be made by its manufacturer is not guaranteed or endorsed by the publisher.
Research integrity at Frontiers
Learn more about the work of our research integrity team to safeguard the quality of each article we publish.