- 1Department of Radiation Oncology, Emory University, Atlanta, GA, United States
- 2Department of Microbiology and Immunology, Emory University, Atlanta, GA, United States
- 3Department of Radiation Oncology, Henry Ford Health System, Detroit, MI, United States
- 4Erlanger UT Radiation Oncology, Chattanooga, TN, United States
- 5Mitchell Cancer Institute, University of Southern Alabama, Mobile, AL, United States
- 6Georgia Cancer Center, Augusta University, Augusta, GA, United States
The combination of radiation and immunotherapy is currently an exciting avenue of pre-clinical and clinical investigation. The synergy between these two treatment modalities has the potential to expand the role of radiation from a purely local therapy, to a role in advanced and metastatic disease. Tumor regression outside of the irradiated field, known as the abscopal effect, is a recognized phenomenon mediated by lymphocytes and enhanced by checkpoint blockade. In this review, we summarize the known mechanistic data behind the immunostimulatory effects of radiation and how this is enhanced by immunotherapy. We also provide pre-clinical data supporting specific radiation timing and optimal dose/fractionation for induction of a robust anti-tumor immune response with or without checkpoint blockade. Importantly, these data are placed in a larger context of understanding T-cell exhaustion and the impact of immunotherapy on this phenotype. We also include relevant pre-clinical studies done in non-tumor systems. We discuss the published clinical trials and briefly summarize salient case reports evaluating the abscopal effect. Much of the data discussed here remains at the preliminary stage, and a number of interesting avenues of research remain under investigation.
Introduction
Traditionally, radiation therapy (RT) is considered a local form of cancer treatment with an “in-field” anti-tumor effect. RT has been used to treat localized malignancies with curative intent or to palliate painful, bleeding or otherwise problematic metastases. Over time radiation delivery has changed (2-D vs. 3-D vs. IMRT), however, the basic philosophy focused on controlling local disease has persisted. Interestingly, in patients with multiple lesions, tumor regression occurs, although rarely, outside the RT field. This is known as an abscopal effect or “ab”- away from, “scopus”–target. This was first described by Mole et al. (1) with over 46 cases of a RT-induced abscopal effect subsequently documented including a prominent report from Memorial Sloan Kettering (2, 3). Patients with several distinct cancer histologies and across a range of ages have benefited from this phenomenon. The abscopal response is now being interrogated with increasing vigor with the goal of improved therapeutic outcomes for metastatic cancer patients, especially in combination with emerging immunotherapy agents (3).
T-cell checkpoints (CTLA-4, PD-1) are cell surface molecules which prevent T-cell activation or reinvigoration following chronic antigen exposure (4–6). Inhibiting these T-cell checkpoints leads to greater anti-tumor T-cell activity. Checkpoint inhibitors are now the most frequently prescribed immunotherapy and have shown great promise in many different malignancies (7–11). Interestingly, the relatively rare abscopal effect has been observed with increasing frequency as checkpoint inhibitors are being given in close temporal proximity or concurrently with RT (12). There are many questions that remain unanswered regarding the safety, efficacy, optimal dose/fractionation and timing of immune-checkpoint inhibitors in combination with RT. Here we present several mechanisms responsible for the abscopal effect and summarize relevant basic science findings, clinical trials, and clinical case reports. We also provide data which may inform optimization of RT dose, fractionation and timing of administration of immune-checkpoint blockade/immuno-modulators in order to maximize the RT-induced abscopal effect.
Radiation and the Immune System
Classically, RT was thought to be immunosuppressive due to the exquisite radio-sensitivity of leukocytes; but, more recently, data has shown that RT can enhance various components of the antigen processing and presentation pathway (13–15). Reits et al. demonstrated in vitro and in vivo, a dose dependent increase in cell-surface MHC-I levels in response to RT in a transcription independent manner (16). This is thought to be due to an increased intracellular peptide pool from both increased protein translation and increased protein degradation leading to a larger epitope repertoire to be presented following tumor cell death.
Liberation of antigens and increased MHC-I expression alone, however, would not be sufficient for effective anti-tumor T-cell priming. For this, maturation of antigen presenting cells (APCs) is necessary. APC maturation involves, in addition to MHC-I and II upregulation, increased expression of costimulatory ligands B7-1, B7-2 as well as cytokine production important for T-cell proliferation and phenotypic skewing (17). This can occur via APC pathogen recognition receptor (PRR) ligation by non-self-derived adjuvants, pathogen-associated molecular patterns (PAMPs), or endogenous damage associated molecular patterns (DAMPs) (18). Importantly, RT can induce immunogenic cell death (ICD), which, in contrast to apoptosis, releases tumor cell contents, including DAMPs, in a disorganized fashion which can be highly pro-inflammatory. In the context of RT induced ICD, DAMPs include high-motility group box 1 (HMGB1), heat shock protein 70 (HSP 70), GP96 and calreticulin membrane exposure (19–21). Calreticulin, an endoplasmic reticulum resident molecular chaperone, can stimulate phagocytosis of cancer cells by dendritic cells (22) while HMBG1, a critical chromatin protein, promotes antigen presentation (23). Radiation-induced calrecticulin exposure increases T-cell mediated tumor lysis, and in the presence of a calreticulin-blocking peptide this effect was abrogated (24). Wang et al. have shown that RT, over a wide dose range, induced HMGB1 extracellular release and cytoplasmic translocation in a dose and time-dependent manner (25). The subsequent HMGB1 mediated APC maturation is TLR-4 dependent (26). An integral role for APCs in anti-tumor T-cell priming and the abscopal effect was shown in a bilateral syngeneic mouse model of breast cancer wherein immunoadjuvant treatment with FMS-like tyrosine kinase receptor 3 ligand (FLT3L), which promote DC development and bone marrow egress (27), resulted in growth delay in an irradiated flank tumor as well as the untreated, contralateral tumor (28). Together these data support an intimate relationship between an anti-tumor immune response and RT mediated tumor cell killing.
Radiation Sequencing With Immunotherapy
How does this immunogenic antigen bolus released by RT and presented by APCs synergize with checkpoint inhibitors to enhance the anti-tumor immune response, and how does this inform the sequencing of these two treatment modalities? Two candidate mechanisms to explain this synergy are proposed: (1) neo-antigens released in response to RT may act in concert with anti-PD-1 immunotherapy to only reinvigorate exhausted intratumoral CD8 T-cells, or (2) RT may stimulate proliferation and differentiation of naïve T-cells in response to liberated neo-antigens while anti-PD-1 may potentiate naïve T-cell activation in addition to reinvigorating exhausted T-cells. Each mechanism leads to a more robust immune response, but would result in a different response amplitude and carries different implications for combined modality therapy. If the immunogenic effect arises from naïve T-cell proliferation and activation, very close sequencing of RT and anti-PD-1 will be required for anti-PD-1 to potentiate early T-cell activation. Whereas, if the reinvigoration of exhausted T-cells is the dominant mechanism, this temporal overlap may be less critical and the effect would be additive rather than synergistic. Current evidence suggests that RT acts primarily to stimulate proliferation and differentiation of naïve T based on a broadening of the T-cell receptor repertoire post-RT although this may reflect an expansion of low frequency exhausted clones (29). These two mechanisms described are not mutually exclusive, however, pre-clinical tumor data has demonstrated that initiating anti-PD-L1 7 days following RT was inferior to starting on either the first or the last day (30). These data support (2), however, a deeper understanding of the underlying mechanism can be found in models of acute viral infections.
The Armstrong strain of lymphocytic choriomeningitis virus (LCMV) is a well characterized system for studying acute T-cell responses and naïve T-cell differentiation (31). In a recently published study, it was demonstrated that exposure to anti-PD-L1 during early T-cell differentiation to an acute Armstrong infection impacts T-cell effector function (32). The authors showed that the acute T-cell response is inhibited by endogenous PD-1 activity, but that anti-PD-L1 during initial T-cell activation increases granzyme B expression in virus-specific CD8 T-cells, resulting in faster clearance of infection (32). While the role of PD-1 in mediating CD8 T-cell reinvigoration in chronic infection is well established (33), this finding supports previous reports by Barber et al. that showed in acute infection of PD-L1−/− mice with LCMV resulted in a heightened CD8 T-cell response. Furthermore, both found the CD8 T-cell response was also improved in chronic infection characterized by T-cell exhaustion (6). Together, these data support close sequencing of RT and checkpoint blockade as late administration of anti-PD-L1 reinvigorates exhausted T-cells without the added benefit of influencing initial T-cell activation and differentiation. Data to directly support these findings in a tumor model combining RT and checkpoint blockade is still lacking.
The kinetics of T-cell tumor infiltration following RT also helps inform the sequencing and timing of anti-PD-1/L1 administration. Following tumor irradiation with 12 Gy on 2 consecutive days, it was shown that overall leukocyte and CD8 T-cell frequencies peak at 5 days post-RT and then gradually decline to pre-RT levels (34). Five-days post-RT also reflects the highest effector to Treg ratio suggesting an ideal time point for checkpoint blockade. These data further support RT dosing with hypofractionation in a limited number of fractions as additional fractions may ablate recently infiltrated lymphocytes. The work by Frey et al. reinforce these findings (35). They showed that following 5 Gy × 2 fractions, CD8 T-cells peak at day 8 and decline significantly by day 9 while Treg have a bimodal peak on days 8 and 10 (35). Together these studies suggest that while the exact T-cell tumor infiltration kinetics may vary depending on the murine model and RT dose, close sequencing of checkpoint blockade following RT should be the goal to take advantage of the peak in tumor effector CD8 T-cells.
Sequencing Depends on the Checkpoint Agent
Optimal RT and immunotherapy sequencing may also depend on the immuno-modulatory agent utilized. As articulated earlier, anti-PD-L1 appears to have the greatest synergy with RT when administered concurrently (30). In contrast, Young et al. have shown data in support of pre-treating with a TGF-β inhibitor in a mouse model of multiple different cancer types including colorectal cancer (36). TGF-β is a factor critical for Treg differentiation, and it is capable of impairing CD8 T-cell effector function. Using a small molecule inhibitor of TGF-β, the authors found an increase in intra-tumoral activated CD8 T-cells and fewer CD4 Treg. For the colorectal cancer experiments, mice were treated with 20 Gy × 1 fraction 7 days after the initiation of the anti-TGF-β therapy. They demonstrated improved survival in mice pre-treated with anti-TGF-β and RT compared to RT alone.
More recently, the same group directly compared the sequencing of two different immuno-modulatory agents relative to RT. In this pre-clinical study, they first evaluated whether administering a CTLA-4 antagonist 7 days prior, 1 day following or 5 days following RT (20 Gy × 1 fraction) changed outcomes. The best outcomes were observed when anti-CTLA-4 was delivered before RT. Interestingly, they showed that all mice that cleared the tumors were resistant to re-challenge with the same cell line at 100 days, suggesting the development of T-cell memory. The group then tested sequencing of anti-OX40. OX40, a secondary co-stimulatory molecule expressed by activated T-cells, was stimulated with the same schedule as anti-CTLA-4 and the highest percent survival was seen in the 1 day post-RT group (37). The authors concluded that the effect of sequencing is dependent on the mechanism of the immunotherapy being used. Given that anti-CTLA-4 may act on naïve T-cells and Treg (38) and anti-PD-1 acts on newly activated and exhausted T-cells (6, 32, 39), these differences in optimal timing are not surprising.
We propose that ideally anti-PD-1/L1 and RT should be given concurrently but that if not RT should precede the administration of checkpoint blockade. RT delivered to the tumor following anti-PD-1/L1 may obliterate the recently infiltrated and reinvigorated T-cell response (Figure 1). In contrast, if RT is delivered before anti-PD-1/L1, RT stimulated naïve T-cell differentiation will synergize with checkpoint blockade and RT induced T-cell death of anti-PD-1/L1 reinvigorated T-cells may be avoided (Figure 2).
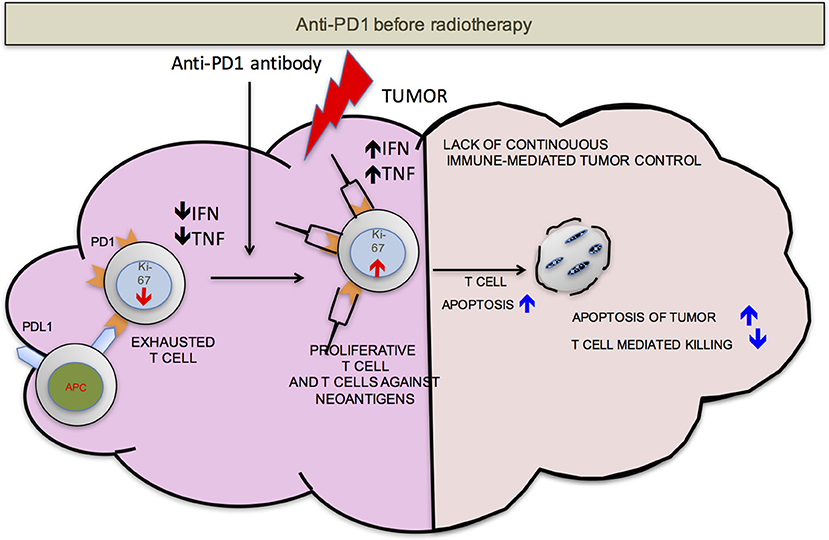
Figure 1. PD-1 before RT. Once the anti-PD-1 antibody is infused into the host, the exhausted cells start proliferating as detected by increase in Ki-67, become functionally active (increased production of IFN-γ and TNF-α) and traffic to the tumor from secondary lymphoid organs. Radiation delivered to the tumor at this point may be detrimental to the anti-tumor responses due to radiation induced T-cell apoptosis. This may compromise control.
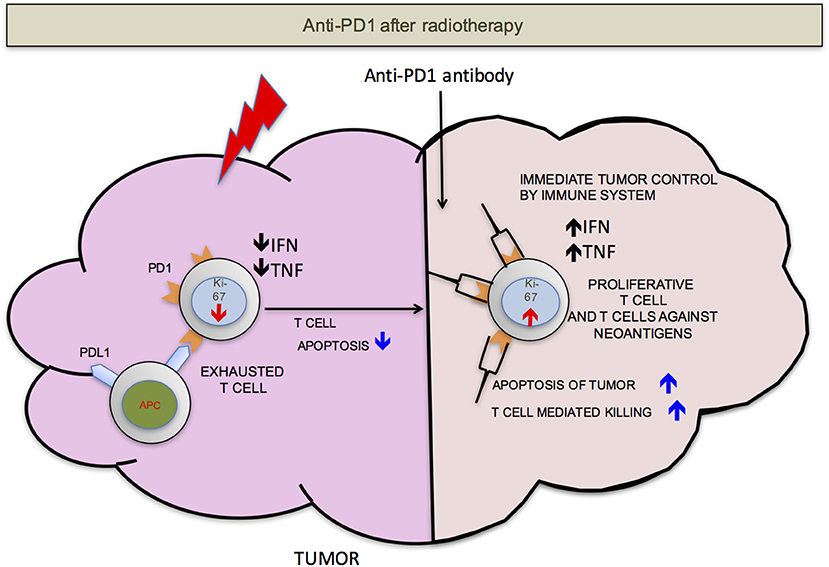
Figure 2. PD-1 after RT. If the radiation is delivered before anti-PD-1 therapy, there will be less T-cells apoptosis. T-cells that infiltrate after radiation mediated neo-antigens release have a better chance to mount an effective anti-tumor response. For simplicity, PD-L1 is shown on only antigen presenting cells (APCs).
RT Dose, Fractionation and the Immune Response
Varying dose and fractionation of RT in combination with immunotherapy and evaluating the anti-tumor immune response is an active area of investigation. Recent experiments from Morisada et al. in which primary tumor and abscopal tumor control rates were measured in a syngeneic mouse model of head and neck squamous cell carcinoma (SCC) following high-dose hypofractionated (8 Gy × 2) or low-dose daily fractionated (2 Gy × 10) RT in combination with concurrent anti-PD-1 showed that daily fractionated RT preserved peripheral and tumor-infiltrating CD8 T-cell accumulation and activation, reduced peripheral and tumor granulocytic myeloid derived suppressor cell (gMDSC) accumulation and did not impact Treg (40). Similarly, Type I IFN levels and expression of IFN-responsive MHC class I and PD-L1 was greater in those subjected to the daily low-dose fractionated regimen, and primary and abscopal tumor control improved when combined with anti-PD-1. Importantly, the local and abscopal effects appears to be similar for different hypofractionated regimens with similar biological equivalent dose (BED) (3 × 9.18 Gy in 3 or 5 days or 5 × 6.43 Gy in 10 days) (41).
Investigators have tested different total doses and fractionation schemes in a variety of pre-clinical models to maximize the abscopal effect (Table 1). Mice engrafted with the B16 melanoma cell line were treated with 15 Gy × 1 or 5 Gy × 3 fractions. The single fraction dose increased antigen availability and the number of tumor specific T-cells secreting IFN-γ in the tumor-draining lymph node to a larger extent than fractionated RT (42). They also showed that tumors receiving 15 Gy had greater infiltration of APCs and CD8 T-cells compared to 5 Gy × 3. To determine the dose for optimal tumor and immunologic response, Schaue et al. conducted a single fraction dose escalation study with doses from 5 to 15 Gy and demonstrated that doses of 7.5 Gy and above are immuno-stimulatory, defined by an increased number of tumor-reactive T-cells (43). However, at high dose, 15 Gy × 1, there was an increase in the splenic Treg fraction. They showed that if they instead fractionated the 15 Gy into 2–5 fractions, fewer Treg and more effector T-cells were identified in the spleens with an optimal dose fractionation of 7.5 Gy × 2. The authors do not offer a clear mechanism for the increased splenic Treg frequency at higher dose or whether this was mirrored in the tumor, but it may depend on the immunologic milieu generated by high dose fractions. Dewan et al. investigated dose and fractionation in a murine syngeneic breast cancer cell line subcutaneously injected at two distinct sites to assess the abscopal response. RT was delivered to one tumor site in 3 different regimens (20 Gy × 1, 8 Gy × 3, or 6 Gy × 5) with or without anti-CTLA-4 (44). The primary site and the secondary site were then monitored for response. They found that a significant abscopal effect was only induced when RT was administered with anti-CTLA-4 in either of the fractionated regimens. They concluded that a single dose, despite, or perhaps because of its size, was insufficient to induce an abscopal effect. These data taken together suggest that there is an optimal range (typically high dose per fraction) for the abscopal effect induction which is further supported by the new data concerning the cGAS-STING pathway.
The importance of the cGAS-STING pathway on the anti-tumor immune response stimulated by both radiation and anti-PD1/L1 has now been established. cGAS (cGAMP synthase), a sensor of cytosolic DNA, a PAMP, catalyzes the formation of second messenger cGAMP which induces type I interferons via the adaptor protein STING. It was shown that cGAS –deficient mice bearing a B16 melanoma had a reduced response to anti-PD-L1 treatment relative to wild-type controls (46). In the cGAS knockout mice there was a decrease in the number of tumor specific CD4 and CD8 T-cells relative to wild-type anti-PD-L1 treated. The effect of anti-PD-L1 blockade was enhanced by intramuscular injections of cGAMP (cGAS product). In the RT context, it has been previously shown that type I interferons induced by RT are important for mediating the anti-tumor immune response (47). Deng at al. demonstrated that the STING signaling axis is activated in DCs, and cGAS is essential for the sensing by the DC of irradiated-tumor cell derived dsDNA. Additionally, they showed that STING promotes an anti-tumor CD8 T-cell response with an increased frequency of IFN-γ+ CD8 T-cells in the tumor-draining lymph node. Interestingly, there appears to be a link between radiation dose per fraction, the cGAS STING axis and radiation's synergy with immunotherapy. The exonuclease, TREX1, is upregulated by an RT dose per fraction greater than 10–12 Gy, and its expression degrades cytosolic dsDNA. This leads to a decreased synergy between radiation and immunotherapy (48, 49). This pathway is now a focus of ongoing and active investigation.
The upregulation of checkpoint molecules, the target of anti-PD-L1, can be induced in the tumor following RT, and the magnitude and kinetics of the induction may vary by dose and fractionation. 10 Gy in 5 fractions has been shown to robustly upregulate PD-L1 on CT26 tumors with a peak at Day 3 post-RT completion (30). In another elegant study, Derer et al. investigated the impact of RT, chemotherapy, and chemoRT on PD-L1 expression in a variety of murine tumor cell lines and found that standard fractionation and hypofractionated RT led to significant increases of PD-L1 expression in both melanoma and glioblastoma cell lines (50). In vivo, fractionated RT with dacarbazine induced PD-L1 expression on B16-F10 tumors, but not RT alone. In the context of human rectal cancer, Lim et al. evaluated pre chemoRT biopsies and post-chemoRT surgical specimens for expression of PD-L1 (51). The chemoRT regimen consisted of 50.4 Gy of radiation in 28 fractions with concurrent 5-fluorouracil and capecitabine. They found that PD-L1 is induced on tumor cells following chemoRT. Interestingly, if they then divided patients into 4 PD-L1 groups based on their biopsy and surgical expression levels, they showed that patients with high levels on biopsy and surgical specimens had the shortest overall survival. Importantly, however, patients that went from low to high levels of PD-L1 did not have shorter survival times suggesting that the PD-L1 induction by chemoRT is not deleterious and may provide an additional opportunity for checkpoint blockade.
On occasion, it is difficult or impractical to deliver this higher dose per fraction ideal for eliciting an anti-tumor immune response. Under these circumstances, the RT may be delivered by irradiating a fractional tumor volume, thereby reducing adverse effects. Using a 3-dimensional lattice radiation therapy (LRT) system, we have shown in a preclinical abscopal model that 20% volume irradiation (delivered to two 10% volumes) of the tumor resulted in significant growth delay in both the irradiated and unirradiated tumors (52). These abscopal effects were mediated by the down-modulation of TH2 functions and induction of robust IFN-γ and TH1 response in addition to increased T-cell infiltration and expression of TRAIL in the irradiated and unirradiated tumors (52). Interestingly, significant radiation-induced abscopal effects were observed in two of seven patients where only the hypoxic region of the tumor was irradiated with a single fraction of high dose radiation (53, 54). Immunomodulatory effects of the treatment were not assessed. These studies suggest that by partial irradiation of tumor volumes, high doses of radiation can be delivered with enhanced immunomodulatory potential, however, more studies are required to examine these novel approaches.
In summary, optimal radiation dose appears to be somewhere between 8 and 10 Gy per fraction in 1–3 fractions, and appears to be critical to an effective anti-tumor response. Although CD8 T-cells may be present in a tumor prior to RT, they may be downregulated by PD-L1-PD-1 mediated immune exhaustion, may not be able to find tumor cells that they were activated against, or there may be an immune suppressive environment induced by multiple cell types. An ideal radiation dose will induce tumor cell mitotic catastrophe (IDC), release tumor neo-antigens and endogenous adjuvants, increase APC maturation and antigen presentation, increase CD8 T-cell proliferation and migration to the tumor, and lead to effective anti-tumor response. A sub-optimal radiation dose may be effective in activating CD8 T-cells, but will fail to achieve standard of care treatment goals such as local control. An excessively high dose will induce tumor cell death and improve local control, but may also damage normal tissue and tumor vasculature with the added disadvantage of inducing widespread CD8 T-cell apoptosis, compromising immune priming, distant control, and the opportunity for induction of the abscopal effect (Figure 3) (55).
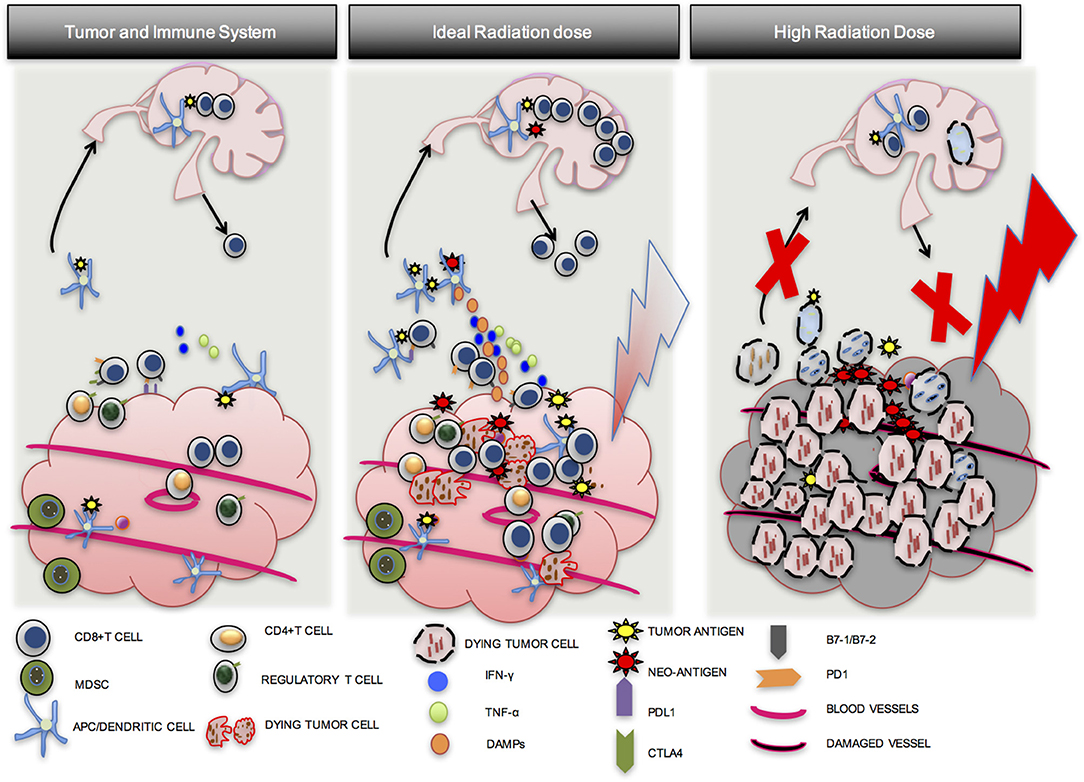
Figure 3. Importance of optimal radiation dose. A number of factors lead to the establishment of tumors in a host. Although CD8 T cells may be present in the tumor, they are exhausted, may not be able to find tumor cells that they were activated against, or there may be an immune suppressive environment induced by multiple cell types (1st Column). An ideal dose of radiation will induce inflammatory tumor cell death and activate an anti-tumor T-cell response via APC maturation (2nd Column). A high dose of radiation may induce tumor cell death but may also damage blood vessels and induce more CD8 T cell apoptosis. Local control from the direct effects of RT may be good, but effective immune priming and distant control may be compromised (3rd Column).
Clinical Data
Dose, Fractionation and Sequencing
The earliest and best trial evaluating different RT doses and immunotherapy was published in 2012. This phase I trial combined three different doses of stereotactic body radiotherapy (SBRT) and IL-2 where tumor and immune responses were evaluated in patients with metastatic melanoma or RCC. Patients received one of three regimens: SBRT 20 Gy × 1, 2, or 3 fractions on a Monday, Wednesday, and Friday schedule, followed by high dose IL-2 (600,000 IU) on the following Monday (72 h after completion of RT). The authors observed an objective response of 66% (8 of 12 patients with a complete or partial response) as measured in the Response Evaluation Criteria in Solid Tumors (RECIST). Additionally, the responding patients had a higher frequency of proliferating effector memory CD4 and CD8 T-cells without a difference in the frequency of proliferating Treg (56). They concluded that SBRT and IL-2 could be administered safely. Interestingly, they did not demonstrate a relationship between SBRT dose and overall response, however, the very small number of patients in this trial and the use of IL-2 allow limited conclusions to be drawn. Additionally, as described previously, the specific immuno-modulatory drug administered with RT is expected to influence optimal timing as well as dose. Finally, it is also well known that different tumor histologies have different radiosensitivities (57), therefore, it is conceivable that the optimal dose for antigen release and immunologic activation is tumor specific. Despite these limitations, this is a landmark trial with one patient with widely metastatic disease achieving PET complete response—an abscopal effect.
More recent studies have confirmed the safety of combination checkpoint blockade and RT without supporting a specific RT dose or RT/checkpoint sequencing (58, 59). An exciting study out of the University of Chicago showed an increased immune score (median expression level of normalized pre-selected genes) in the irradiated metastasis correlated with a greater change in the unirradiated lesion (60). The dose used varied from 30 Gy in 3 fractions to 50 Gy in 5 fractions determined by anatomic site with anti-PD-1 given every 3 weeks and initiated within 7 days after the final SBRT fraction. Additional data suggests synergy between RT and anti-CTLA-4 or anti-PD-1 in metastatic castration resistant prostate cancer and advanced non-small cell lung cancer (NSCLC), respectively (61, 62). The majority of on-going clinical trials prescribe concurrent administration of immunomodulatory agents and RT guided by the preclinical data (63). Of note, the recently published PACIFIC trial of stage III NSCLC demonstrated an overall survival benefit to adjuvant durvalumab (anti-PD-L1) following chemoradiation (64). As NSCLC has a very high rate of distant failure, this suggests that durvalumab improved local control as well as the micrometastatic disease (abscopal effect). There is now an actively enrolling trial evaluating the benefit of concurrent durvalumab with chemoradiation in stage III NSCLC (NCT03519971).
Data from the brain metastasis literature also supports close sequencing of RT and checkpoint blockade, although most of these data evaluate local control rather than an abscopal response. In one of the larger retrospective analyses, 75 melanoma patients with 566 brain metastases were evaluated. They received SRS and immune checkpoint therapy between 2007 and 2015 at Yale University (65). SRS was given in a single fraction to a median of 20 Gy (range, 12–24 Gy). Seventy-two percent of patients received anti-CTLA-4 and 28% received anti-PD-L1. Fifty-five percent of lesions were treated with concurrent SRS and immunotherapy (SRS administered within 4 weeks of immunotherapy). It was shown that, compared to non-concurrent treatment, concurrent use of immunotherapy and SRS resulted in a significant greater median percent reduction in lesion volume. Another study of 46 patients with metastatic melanoma who received ipilimumab and SRS found that patients treated with SRS during or before ipilimumab had higher overall survival and less regional recurrence suggesting an abscopal response compared to those treated with SRS after ipilimumab (66).
In totality, these data as consistent with preclinical results and our model (Figures 1, 2) that the concurrent use of RT and immunotherapy, results in a more pronounced treatment response.
Data supporting concurrent administration with RT for agents other than checkpoint inhibitors has also been evaluated. Forty-One patients with metastatic solid tumors treated with concurrent RT and granulocyte-macrophage colony-stimulating factor (GM-CSF) had stable or progressing metastatic solid tumors with at least three measurable metastatic sites and were on single chemotherapy or hormonal therapy (14, 67). Of the 41 patients, the most common tumor types were non-small cell lung cancer (44%) and breast cancer (34%). Two metastatic lesions were sequentially treated in each patient. Each lesion received 35 Gy of RT in ten fractions in two consecutive weeks, with daily subcutaneous GM-CSF injections lasting for 2 weeks starting during the second week of RT. The same process was repeated for the second metastatic lesion. Abscopal response (here defined as at least 30% decrease in the longest dimension of the best responding lesion) was observed in 19 (46%) patients. This is despite a non-optimal protracted regimen of 35 Gy in 10 fractions. Fourteen grade 3 or 4 toxicities attributable to either RT or immunotherapy were observed, with fatigue being the most common.
Finally, to date, at least 46 RT-induced abscopal effect case reports have been published from 1969 to 2014 (2). Table 2 displays a selection of representative studies. Histologies that have demonstrated abscopal effects include hepatocellular carcinoma, adenocarcinoma of the lung and esophagus, medullary thyroid carcinoma, Merkel cell carcinoma, follicular lymphoma, lymphocytic lymphoma, Hodgkin's lymphoma, CLL, renal cell carcinoma, and melanoma. Of the reported cases, the median age was 64 years (range: 28–83), the median RT dose was 31 Gy (range: 0.45–60.75), and the median dose per fraction was 3 Gy. The median time to an abscopal effect was 2 months (range: 0–24 months) and the median time to progression was 6 months (range: 0.7–14 months). Of these 46 published cases, only five patients had immunotherapy during treatment, four of which were melanoma patients. Therefore, relying on currently published case reports to guide timing of RT with immunotherapy is difficult. However, what can be gleaned from these case reports is that the abscopal effect does occur in multiple different cancer histologies.
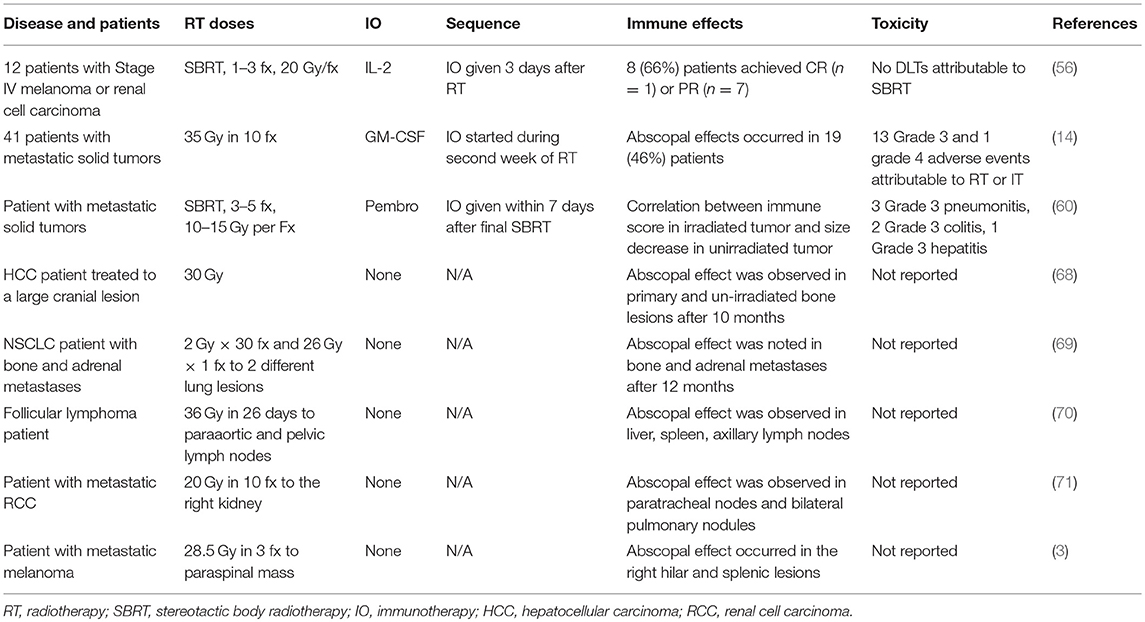
Table 2. A selection of clinical trials and case reports that evaluated immune-stimulatory effects of RT.
Discussion
Many of the topics addressed in this review remain areas of active inquiry with a number of smaller checkpoint and RT studies having been published. Our lab is also investigating questions of fractionation and timing. Although there appears to be a consensus that hypo-fractionation is superior to conventional fractionation, the optimal dose for an abscopal or local immune response may depend on tumor histology and non-synonymous mutation burden due to varying radio-sensitivities and neo-antigen load (72). Additionally, the optimal interaction may also vary with the specific immunotherapy administered as CTLA-4 and PD-1/PD-L1 antagonists have distinct and non-redundant mechanisms. These numerous variables add complexity to any proposed clinical trial design.
We recommend including different fractionation schemes in any proposed immunotherapy and radiation clinical trials and suggest potentially varying the fractionation schemes from one tumor histology to another. These data suggest that a dose per fraction of close to 10 Gy with 1–3 fractions is likely optimal for abscopal effect induction. Importantly, a dose and fractionation regimen optimized for a robust local response may be expected to differ from that optimized for a distant abscopal response and additional data are needed to elucidate these likely tumor-specific thresholds.
We also encourage further investigation involving the sequencing of radiation and immunotherapy. Evidence presented here suggests immunotherapy should be initiated at the start of radiation when employing single or high dose per fraction RT as this is the time when a bolus of neo-antigens is released, followed later by more limited T-cell epitope availability. Conventional fractionation may instead lead to a steady release of tumor antigens throughout treatment and the exact point of immunotherapy initiation may be less critical, although earlier initiation of immunotherapy is likely to remain superior. Finally, the mechanism of radiation and immunotherapy for T-cell activation is specific (29), and understanding why close sequencing rather than more remote administration of immunotherapy improves control in several contexts is an important avenue of investigation.
Conclusion
The synergy between RT and immunotherapy has now definitively entered the mainstream. A deep and clear mechanistic understanding of RT's immune system stimulation and its synergy with immunotherapy affirms the value in pursuing and expanding this avenue of research. There are, however, still many unanswered questions in the optimization of the abscopal response including, but not limited to: RT and immunotherapy sequencing, RT dose and fractionation, and RTs specific interactions with different immuno-modulatory agents and individual tumor subtypes. It is our hope that the research community continues to vigorously pursue these and other vital questions surrounding the induction of the abscopal effect. The solution to transforming RT from a purely local or palliative therapy to a treatment important for long-term metastatic control, we believe, may lie in the answer to these questions.
Author Contributions
ZB, JW, SG, and SZ: wrote the manuscript; TN: created the figures; SZ: created the tables; WM, MK, and SK: edited the manuscript and provided expertise.
Conflict of Interest Statement
The authors declare that the research was conducted in the absence of any commercial or financial relationships that could be construed as a potential conflict of interest.
References
1. Mole RH. Whole body irradiation; radiobiology or medicine? Br J Radiol. (1953) 26:234–41. doi: 10.1259/0007-1285-26-305-234
2. Abuodeh Y, Venkat P, Kim S. Systematic review of case reports on the abscopal effect. Curr Probl Cancer (2016) 40:25–37. doi: 10.1016/j.currproblcancer.2015.10.001
3. Postow MA, Callahan MK, Barker CA, Yamada Y, Yuan J, Kitano S, et al. Immunologic correlates of the abscopal effect in a patient with melanoma. N Engl J Med. (2012) 366:925–31. doi: 10.1056/NEJMoa1112824
4. Krummel MF, Allison JP. del CD28 and CTLA-4 have opposing effects on the response of T cells to stimulation. J Exp Med. (1995) 182:459–65. doi: 10.1084/jem.182.2.459
5. Okazaki T, Maeda A, Nishimura H, Kurosaki T, Honjo T. del PD-1 immunoreceptor inhibits B cell receptor-mediated signaling by recruiting src homology 2-domain-containing tyrosine phosphatase 2 to phosphotyrosine. Proc Natl Acad Sci USA. (2001) 98:13866–71. doi: 10.1073/pnas.231486598
6. Barber DL, Wherry EJ, Masopust D, Zhu B, Allison JP, Sharpe AH, et al. Restoring function in exhausted CD8 T cells during chronic viral infection. Nature (2006) 439:682–7. doi: 10.1038/nature04444
7. Antonia SJ, López-Martin JA, Bendell J, Ott PA, Taylor M, Eder JP, et al. Nivolumab alone and nivolumab plus ipilimumab in recurrent small-cell lung cancer (CheckMate 032): a multicentre, open-label, phase 1/2 trial. Lancet Oncol. (2016) 17:883–95. doi: 10.1016/S1470-2045(16)30098-5
8. Reck M, Rodríguez-Abreu D, Robinson AG, Hui R, Csoszi T, Fülöp A, et al. Pembrolizumab versus chemotherapy for PD-L1-positive non-small-cell lung cancer. N Engl J Med. (2016) 375:1823–33. doi: 10.1056/NEJMoa1606774
9. Powles T, O'Donnell PH, Massard C, Arkenau HT, Friedlander TW, Hoimes CJ, et al. Efficacy and safety of durvalumab in locally advanced or metastatic urothelial carcinoma: updated results from a phase 1/2 open-label study. JAMA Oncol. (2017) 3:e172411. doi: 10.1001/jamaoncol.2017.2411
10. Ascierto PA, Del Vecchio M, Robert C, Mackiewicz A, Chiarion-Sileni V, Arance A, et al. Ipilimumab 10 mg/kg versus ipilimumab 3 mg/kg in patients with unresectable or metastatic melanoma: a randomised, double-blind, multicentre, phase 3 trial. Lancet Oncol. (2017) 18:611–22. doi: 10.1016/S1470-2045(17)30231-0
11. Leach DR, Krummel MF, Allison JP. Enhancement of antitumor immunity by CTLA-4 blockade. Science (1996) 271:1734–6. doi: 10.1126/science.271.5256.1734
12. Ngwa W, Irabor OC, Schoenfeld JD, Hesser J, Demaria S, Formenti SC. Using immunotherapy to boost the abscopal effect. Nat Rev Cancer (2018) 18:313–22. doi: 10.1038/nrc.2018.6
13. Kalbasi A, June CH, Haas N, Vapiwala N. Radiation and immunotherapy: a synergistic combination. J Clin Invest. (2013) 123:2756–63. doi: 10.1172/JCI69219
14. Golden EB, Pellicciotta I, Demaria S, Barcellos-Hoff MH, Formenti SC. The convergence of radiation and immunogenic cell death signaling pathways. Front Oncol. (2012) 2:88. doi: 10.3389/fonc.2012.00088
15. Buchwald ZS, Efstathiou JA. Immunotherapy and radiation – a new combined treatment approach for bladder cancer? Bladder Cancer (2015) 1:15–27. doi: 10.3233/BLC-150014
16. Reits EA, Hodge JW, Herberts CA, Groothuis TA, Chakraborty M, Wansley EK, et al. Radiation modulates the peptide repertoire, enhances MHC class I expression, and induces successful antitumor immunotherapy. J Exp Med. (2006) 203:1259–71. doi: 10.1084/jem.20052494
17. Rescigno M, Granucci F, Ricciardi-Castagnoli P. Dendritic cells at the end of the millennium. Immunol Cell Biol. (1999) 77:404–10. doi: 10.1046/j.1440-1711.1999.00854.x
18. Cao X. Self-regulation and cross-regulation of pattern-recognition receptor signalling in health and disease. Nat Rev Immunol. (2016) 16:35–50. doi: 10.1038/nri.2015.8
19. Galluzzi L, Buqué A, Kepp O, Zitvogel L, Kroemer G. Immunogenic cell death in cancer and infectious disease. Nat Rev Immunol. (2017) 17:97–111. doi: 10.1038/nri.2016.107
20. Pandolfi F, Altamura S, Frosali S, Conti P. Key role of DAMP in inflammation, cancer, and tissue repair. Clin Ther. (2016) 38:1017–28. doi: 10.1016/j.clinthera.2016.02.028
21. Kono H, Rock KL. How dying cells alert the immune system to danger. Nat Rev Immunol. (2008) 8:279–89. doi: 10.1038/nri2215
22. Obeid M, Tesniere A, Ghiringhelli F, Fimia GM, Apetoh L, Perfettini JL, et al. Calreticulin exposure dictates the immunogenicity of cancer cell death. Nat Med. (2007) 13:54–61. doi: 10.1038/nm1523
23. Ghiringhelli F, Apetoh L, Tesniere A, Aymeric L, Ma Y, Ortiz C, et al. Activation of the NLRP3 inflammasome in dendritic cells induces IL-1beta-dependent adaptive immunity against tumors. Nat Med. (2009) 15:1170–8. doi: 10.1038/nm.2028
24. Gameiro SR, Jammeh ML, Wattenberg MM, Tsang KY, Ferrone S, Hodge JW. Radiation-induced immunogenic modulation of tumor enhances antigen processing and calreticulin exposure, resulting in enhanced T-cell killing. Oncotarget (2014) 5:403–16. doi: 10.18632/oncotarget.1719
25. Wang L, He L, Bao G, He X, Fan S, Wang H. Ionizing radiation induces HMGB1 cytoplasmic translocation and extracellular release. Guo Ji Fang She Yi Xue He Yi Xue Za Zhi (2016) 40:91–9.
26. Apetoh L, Ghiringhelli F, Tesniere A, Obeid M, Ortiz C, Criollo A, et al. Toll-like receptor 4-dependent contribution of the immune system to anticancer chemotherapy and radiotherapy. Nat Med. (2007) 13:1050–9. doi: 10.1038/nm1622
27. Salmon H, Idoyaga J, Rahman A, Leboeuf M, Remark R, Jordan S, et al. Expansion and activation of CD103(+) dendritic cell progenitors at the tumor site enhances tumor responses to therapeutic PD-L1 and BRAF inhibition. Immunity (2016) 44:924–38. doi: 10.1016/j.immuni.2016.03.012
28. Demaria S, Ng B, Devitt ML, Babb JS, Kawashima N, Liebes L, et al. Ionizing radiation inhibition of distant untreated tumors (abscopal effect) is immune mediated. Int J Radiat Oncol Biol Phys. (2004) 58:862–70. doi: 10.1016/j.ijrobp.2003.09.012
29. Twyman-Saint Victor C, Rech AJ, Maity A, Rengan R, Pauken KE, Stelekati E, et al. Radiation and dual checkpoint blockade activate non-redundant immune mechanisms in cancer. Nature (2015) 520:373–7. doi: 10.1038/nature14292
30. Dovedi SJ, Adlard AL, Lipowska-Bhalla G, McKenna C, Jones S, Cheadle EJ, et al. Acquired resistance to fractionated radiotherapy can be overcome by concurrent PD-L1 blockade. Cancer Res. (2014) 74:5458–68. doi: 10.1158/0008-5472.CAN-14-1258
31. Zhou X, Ramachandran S, Mann M, Popkin DL. Role of lymphocytic choriomeningitis virus (LCMV) in understanding viral immunology: past, present and future. Viruses (2012) 4:2650–69. doi: 10.3390/v4112650
32. Ahn E, Araki K, Hashimoto M, Li W, Riley JL, Cheung J, et al. Role of PD-1 during effector CD8 T cell differentiation. Proc Natl Acad Sci USA. (2018) 115:4749–54. doi: 10.1073/pnas.1718217115
33. Hashimoto M, Kamphorst AO, Im SJ, Kissick HT, Pillai RN, Ramalingam SS, et al. CD8 T cell exhaustion in chronic infection and cancer: opportunities for interventions. Annu Rev Med. (2018) 69:301–18. doi: 10.1146/annurev-med-012017-043208
34. Hettich M, Lahoti J, Prasad S, Niedermann G. Checkpoint antibodies but not T cell-recruiting diabodies effectively synergize with TIL-inducing gamma-irradiation. Cancer Res. (2016) 76:4673–83. doi: 10.1158/0008-5472.CAN-15-3451
35. Frey B, Rückert M, Weber J, Mayr X, Derer A, Lotter M, et al. Hypofractionated irradiation has immune stimulatory potential and induces a timely restricted infiltration of immune cells in colon cancer tumors. Front Immunol. (2017) 8:231. doi: 10.3389/fimmu.2017.00231
36. Young KH, Newell P, Cottam B, Friedman D, Savage T, Baird JR, et al. TGFbeta inhibition prior to hypofractionated radiation enhances efficacy in preclinical models. Cancer Immunol Res. (2014) 2:1011–22. doi: 10.1158/2326-6066.CIR-13-0207
37. Young KH, Baird JR, Savage T, Cottam B, Friedman D, Bambina S, et al. Optimizing timing of immunotherapy improves control of tumors by hypofractionated radiation therapy. PLoS ONE (2016) 11:e0157164. doi: 10.1371/journal.pone.0157164
38. Korman AJ, Peggs KS, Allison JP. Checkpoint blockade in cancer immunotherapy. Adv Immunol. (2006) 90:297–339. doi: 10.1016/S0065-2776(06)90008-X
39. Iwai Y, Ishida M, Tanaka Y, Okazaki T, Honjo T, Minato N. Involvement of PD-L1 on tumor cells in the escape from host immune system and tumor immunotherapy by PD-L1 blockade. Proc Natl Acad Sci USA. (2002) 99:12293–7. doi: 10.1073/pnas.192461099
40. Morisada M, Clavijo PE, Moore E, Sun L, Chamberlin M, Van Waes C, et al. PD-1 blockade reverses adaptive immune resistance induced by high-dose hypofractionated but not low-dose daily fractionated radiation. Oncoimmunology (2018) 7:e1395996. doi: 10.1080/2162402X.2017.1395996
41. Zhang X, Niedermann G. Abscopal effects with hypofractionated schedules extending into the effector phase of the tumor-specific T-cell response. Int J Radiat Oncol Biol Phys. (2018) 101:63–73. doi: 10.1016/j.ijrobp.2018.01.094
42. Lugade AA, Moran JP, Gerber SA, Rose RC, Frelinger JG, Lord EM. Local radiation therapy of B16 melanoma tumors increases the generation of tumor antigen-specific effector cells that traffic to the tumor. J Immunol. (2005) 174:7516–23. doi: 10.4049/jimmunol.174.12.7516
43. Schaue D, Ratikan JA, Iwamoto KS, McBride WH. Maximizing tumor immunity with fractionated radiation. Int J Radiat Oncol Biol Phys. (2012) 83:1306–10. doi: 10.1016/j.ijrobp.2011.09.049
44. Dewan MZ, Galloway AE, Kawashima N, Dewyngaert JK, Babb JS, Formenti SC, et al. Fractionated but not single-dose radiotherapy induces an immune-mediated abscopal effect when combined with anti-CTLA-4 antibody. Clin Cancer Res. (2009) 15:5379–88. doi: 10.1158/1078-0432.CCR-09-0265
45. Kulzer L, Rubner Y, Deloch L, Allgäuer A, Frey B, Fietkau R, et al. Norm- and hypo-fractionated radiotherapy is capable of activating human dendritic cells. J Immunotoxicol. (2014) 11:328–36. doi: 10.3109/1547691X.2014.880533
46. Wang H, Hu S, Chen X, Shi H, Chen C, Sun L, et al. cGAS is essential for the antitumor effect of immune checkpoint blockade. Proc Natl Acad Sci USA. (2017) 114:1637–42. doi: 10.1073/pnas.1621363114
47. Burnette BC, Liang H, Lee Y, Chlewicki L, Khodarev NN, Weichselbaum RR, et al. The efficacy of radiotherapy relies upon induction of type i interferon-dependent innate and adaptive immunity. Cancer Res. (2011) 71:2488–96. doi: 10.1158/0008-5472.CAN-10-2820
48. Vanpouille-Box C, Formenti SC, Demaria S. TREX1 dictates the immune fate of irradiated cancer cells. Oncoimmunology (2017) 6:e1339857. doi: 10.1080/2162402X.2017.1339857
49. Vanpouille-Box C, Alard A, Aryankalayil MJ, Sarfraz Y, Diamond JM, Schneider RJ, et al. DNA exonuclease Trex1 regulates radiotherapy-induced tumour immunogenicity. Nat Commun. (2017) 8:15618. doi: 10.1038/ncomms15618
50. Derer A, Spiljar M, Bäumler M, Hecht M, Fietkau R, Frey B, et al. Chemoradiation increases PD-L1 expression in certain melanoma and glioblastoma cells. Front Immunol. (2016) 7:610. doi: 10.3389/fimmu.2016.00610
51. Lim YJ, Koh J, Kim S, Jeon SR, Chie EK, Kim K, et al. Chemoradiation-induced alteration of programmed death-ligand 1 and CD8(+) tumor-infiltrating lymphocytes identified patients with poor prognosis in rectal cancer: a matched comparison analysis. Int J Radiat Oncol Biol Phys. (2017) 99:1216–24. doi: 10.1016/j.ijrobp.2017.07.004
52. Kanagavelu S, Gupta S, Wu X, Philip S, Wattenberg MM, Hodge JW, et al. In vivo effects of lattice radiation therapy on local and distant lung cancer: potential role of immunomodulation. Radiat Res. (2014) 182:149–62. doi: 10.1667/RR3819.1
53. Tubin S, Raunik W. Hunting for abscopal and bystander effects: clinical exploitation of non-targeted effects induced by partial high-single-dose irradiation of the hypoxic tumour segment in oligometastatic patients. Acta Oncol. (2017) 56:1333–9. doi: 10.1080/0284186X.2017.1346385
54. Shareef MM, Cui N, Burikhanov R, Gupta S, Satishkumar S, Shajahan S, et al. Role of tumor necrosis factor-alpha and TRAIL in high-dose radiation-induced bystander signaling in lung adenocarcinoma. Cancer Res. (2007) 67:11811–20. doi: 10.1158/0008-5472.CAN-07-0722
55. Park HJ, Griffin RJ, Hui S, Levitt SH, Song CW. Radiation-induced vascular damage in tumors: implications of vascular damage in ablative hypofractionated radiotherapy (SBRT and SRS). Radiat Res. (2012) 177:311–27. doi: 10.1667/RR2773.1
56. Seung SK, Curti BD, Crittenden M, Walker E, Coffey T, Siebert JC, et al. Phase 1 study of stereotactic body radiotherapy and interleukin-2–tumor and immunological responses. Sci Transl Med. (2012) 4:137ra74. doi: 10.1126/scitranslmed.3003649
57. Williams JR, Zhang Y, Zhou H, Gridley DS, Koch CJ, Russell J, et al. A quantitative overview of radiosensitivity of human tumor cells across histological type and TP53 status. Int J Radiat Biol. (2008) 84:253–64. doi: 10.1080/09553000801953342
58. Qin R, Olson A, Singh B, Thomas S, Wolf S, Bhavsar NA, et al. Safety and efficacy of radiation therapy in advanced melanoma patients treated with ipilimumab. Int J Radiat Oncol Biol Phys. (2016) 96:72–7. doi: 10.1016/j.ijrobp.2016.04.017
59. Bang A, Wilhite TJ, Pike LRG, Cagney DN, Aizer AA, Taylor A, et al. Multicenter evaluation of the tolerability of combined treatment with PD-1 and CTLA-4 immune checkpoint inhibitors and palliative radiation therapy. Int J Radiat Oncol Biol Phys. (2017) 98:344–51. doi: 10.1016/j.ijrobp.2017.02.003
60. Luke JJ, Lemons JM, Karrison TG, Pitroda SP, Melotek JM, Zha Y, et al. Safety and clinical activity of pembrolizumab and multisite stereotactic body radiotherapy in patients with advanced solid tumors. J Clin Oncol. (2018) 36:1611–8. doi: 10.1200/JCO.2017.76.2229
61. Shaverdian N, Lisberg AE, Bornazyan K, Veruttipong D, Goldman JW, Formenti SC, et al. Previous radiotherapy and the clinical activity and toxicity of pembrolizumab in the treatment of non-small-cell lung cancer: a secondary analysis of the KEYNOTE-001 phase 1 trial. Lancet Oncol. (2017) 18:895–903. doi: 10.1016/S1470-2045(17)30380-7
62. Slovin SF, Higano CS, Hamid O, Tejwani S, Harzstark A, Alumkal JJ, et al. Ipilimumab alone or in combination with radiotherapy in metastatic castration-resistant prostate cancer: results from an open-label, multicenter phase I/II study. Ann Oncol. (2013) 24:1813–21. doi: 10.1093/annonc/mdt107
63. Kang J, Demaria S, Formenti S. Current clinical trials testing the combination of immunotherapy with radiotherapy. J Immunother Cancer (2016) 4:51. doi: 10.1186/s40425-016-0156-7
64. Antonia SJ, Villegas A, Daniel D, Vicente D, Murakami S, Hui R, et al. Overall survival with durvalumab after chemoradiotherapy in stage III NSCLC. N Engl J Med. (2018). doi: 10.1056/NEJMoa1809697. [Epub ahead of print].
65. Qian JM, Yu JB, Kluger HM, Chiang VL. Timing and type of immune checkpoint therapy affect the early radiographic response of melanoma brain metastases to stereotactic radiosurgery. Cancer (2016) 122:3051–8. doi: 10.1002/cncr.30138
66. Kiess AP, Wolchok JD, Barker CA, Postow MA, Tabar V, Huse JT, et al. Stereotactic radiosurgery for melanoma brain metastases in patients receiving ipilimumab: safety profile and efficacy of combined treatment. Int J Radiat Oncol Biol Phys. (2015) 92:368–75. doi: 10.1016/j.ijrobp.2015.01.004
67. Golden EB, Chhabra A, Chachoua A, Adams S, Donach M, Fenton-Kerimian M, et al. Local radiotherapy and granulocyte-macrophage colony-stimulating factor to generate abscopal responses in patients with metastatic solid tumours: a proof-of-principle trial. Lancet Oncol. (2015) 16:795–803. doi: 10.1016/S1470-2045(15)00054-6
68. Nam SW, Han JY, Kim JI, Park SH, Cho SH, Han NI, et al. Spontaneous regression of a large hepatocellular carcinoma with skull metastasis. J Gastroenterol Hepatol. (2005) 20:488–92. doi: 10.1111/j.1440-1746.2005.03243.x
69. Siva S, Callahan J, MacManus MP, Martin O, Hicks RJ, Ball DL. Abscopal [corrected] effects after conventional and stereotactic lung irradiation of non-small-cell lung cancer. J Thorac Oncol. (2013) 8:e71–2. doi: 10.1097/JTO.0b013e318292c55a
70. Nobler MP. The abscopal effect in malignant lymphoma and its relationship to lymphocyte circulation. Radiology (1969) 93:410–2. doi: 10.1148/93.2.410
71. MacManus MP, Harte RJ, Stranex S. Spontaneous regression of metastatic renal cell carcinoma following palliative irradiation of the primary tumour. Ir J Med Sci. (1994) 163:461–3. doi: 10.1007/BF02940567
Keywords: radiation, immunotherapy, checkpoint blockade, abscopal effect, PD-1, PD-L1
Citation: Buchwald ZS, Wynne J, Nasti TH, Zhu S, Mourad WF, Yan W, Gupta S, Khleif SN and Khan MK (2018) Radiation, Immune Checkpoint Blockade and the Abscopal Effect: A Critical Review on Timing, Dose and Fractionation. Front. Oncol. 8:612. doi: 10.3389/fonc.2018.00612
Received: 27 October 2018; Accepted: 29 November 2018;
Published: 13 December 2018.
Edited by:
Udo S. Gaipl, Universitätsklinikum Erlangen, GermanyReviewed by:
Rodabe N. Amaria, University of Texas MD Anderson Cancer Center, United StatesDaniel Olive, Faculté de Médecine, Aix Marseille Université, France
Copyright © 2018 Buchwald, Wynne, Nasti, Zhu, Mourad, Yan, Gupta, Khleif and Khan. This is an open-access article distributed under the terms of the Creative Commons Attribution License (CC BY). The use, distribution or reproduction in other forums is permitted, provided the original author(s) and the copyright owner(s) are credited and that the original publication in this journal is cited, in accordance with accepted academic practice. No use, distribution or reproduction is permitted which does not comply with these terms.
*Correspondence: Mohammad K. Khan, ZHJraHVycmFtMjAwMEBnbWFpbC5jb20=