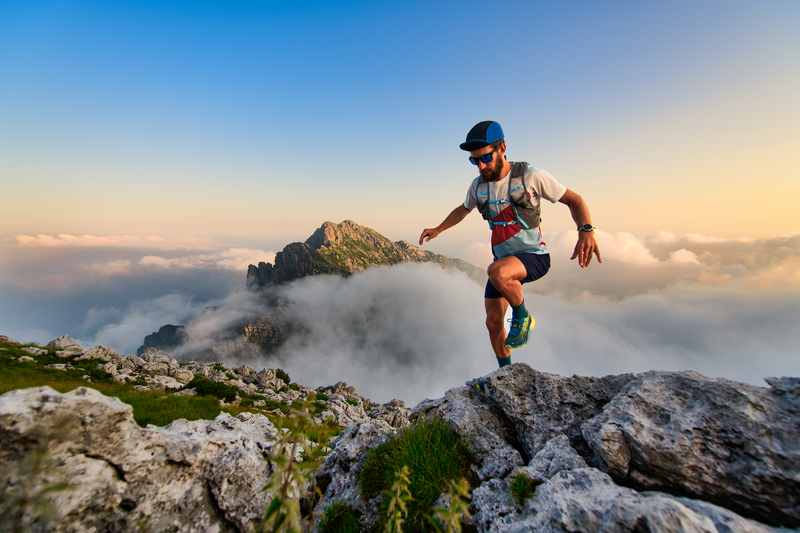
95% of researchers rate our articles as excellent or good
Learn more about the work of our research integrity team to safeguard the quality of each article we publish.
Find out more
MINI REVIEW article
Front. Oncol. , 06 November 2018
Sec. Cancer Immunity and Immunotherapy
Volume 8 - 2018 | https://doi.org/10.3389/fonc.2018.00508
This article is part of the Research Topic Immunotherapy in Multiple Myeloma View all 19 articles
The advent of immune checkpoint (ICP) blockade has introduced an unprecedented paradigm shift in the treatment of cancer. Though very promising, there is still a substantial proportion of patients who do not respond or develop resistance to ICP blockade. In vitro and in vivo models are eagerly needed to identify mechanisms to maximize the immune potency of ICP blockade and overcome primary and acquired resistance to ICP blockade. Vγ9Vδ2 T cells isolated from the bone marrow (BM) from multiple myeloma (MM) are excellent tools to investigate the mechanisms of resistance to PD-1 blockade and to decipher the network of mutual interactions between PD-1 and the immune suppressive tumor microenvironment (TME). Vγ9Vδ2 T cells can easily be interrogated to dissect the progressive immune competence impairment generated in the TME by the long-lasting exposure to myeloma cellss. BM MM Vγ9Vδ2 T cells are PD-1+ and anergic to phosphoantigen (pAg) stimulation; notably, single agent PD-1 blockade is insufficient to fully recover their anti-tumor activity in vitro indicating that additional players are involved in the anergy of Vγ9Vδ2 T cells. In this mini-review we will discuss the value of Vγ9Vδ2 T cells as investigational tools to improve the potency of ICP blockade and immune interventions in MM.
Multiple myeloma (MM) is a disease characterized by the malignant growth of clonal plasma cells (hereafter referred to as myeloma cells) driven by intrinsic and extrinsic mechanisms. MM is uniformly preceded by a premalignant phase, termed monoclonal gammopathy of undetermined significance (MGUS). The risk of progression from MGUS to MM varies from 1 to 5% per year (1). Interestingly, myeloma cells isolated from the BM of MGUS already harbor many of the genetic and epigenetic abnormalities of myeloma cells isolated from patients with overt disease. Interestingly, long-term follow up has shown that almost 50% of high-risk MGUS never progresses to overt MM (2). These clinical data strongly support the concept that other factors, in addition to intrinsic myeloma cell features, are important to determine the fate and aggressiveness of myeloma cells.
The nature and relevance of the tumor microenvironment (TME) in MM have comprehensively been described elsewhere, including the role of immune cells (3, 4). We have anticipated these insights in the mid ‘80s, when we have shown a defective CD73 expression in CD8+ cells which was correlated with the proliferative activity of BM PC in both MGUS and MM (5). These initial findings have been corroborated by many other preclinical studies leading to the pioneeristic development of active specific immunotherapy approaches. The unique expression of idiotype (Id) by clonal B cells encouraged the generation of a variety of Id-specific vaccines (from protein- to DNA-based vaccines) which were able to induce long-lasting and tumor-specific immune responses (6).
Clinical results in allo-transplanted MM patients have strengthened the perception that the only chance to permanently eliminate residual myeloma cells [including those surviving high dose melphalan and autologous stem cell transplantation (ASCT)] is the recognition and elimination by allogeneic immune effector cells (7). The development of immunomodulatory imide drugs (IMiDs) and the clinical results obtained with lenalidomide (including maintenance treatment after ASCT) have brought further evidences that immune cells in the TME are key targets to interrupt the myeloma cell prosurvival network (8).
These approaches have significantly impacted on the clinical outcome, but none of them has generated such an impressive cure rate to definitely change the natural history of the disease (Figure 1).
Figure 1. Immune-based approaches in MM patients (A–C) and major hurdles to their definitive clinical success (D-G). (A) The monoclonal immunoglobulin produced by myeloma cells is a very specific TAA. The antigenic determinants localized in the complementary determining regions of monoclonal heavy and light chains (yellow and green rectangles) are termed idiotypes (Id) and are tumor-specific. Id specifities have been used to address tumor-specific immune responses. A vaccine formulation consisting of Id-specific proteins conjugated with KLH as immunogenic carrier has been shown to generate very specific and long-lasting anti-myeloma immune responses (6). (B) The ultimate goals of allogeneic transplantation (allo-tx) are to ensure a rapid blood engraftment mediated by donor HSC and concurrently address donor immune effector cells to eliminate residual malignant cells (GVL) and to control post-transplant infections (GVI; green lines). Ideally, these goals are achieved in the absence of graft rejection and/or GVHD (red lines) (72). So far, it has not been possible to clearly separate GVL from GVHD in the clinical practice. (C) Immunomodulatory drugs like lenalidomide fine-tune multiple immune functions in MM patients: (i) they enhance and potentiate the cytototoxic and ADCC activity of T cells and NK cells, respectively; (ii) they inhibit myeloma cell growth and induce apoptosis; (iii) they inhibit osteoclasts, ECs, and Tregs suppressor functions. (D) The role of innate effector cells such as NK cells, NKT cells and γδ T cells has been neglected when initial immunotherapy approaches have been developed; the need to overcome or neutralize the suppressor role of MDSCs, Tregs, TAMs, and suppressive neutrophils type II (GN2) was unkown and not addressed. (E) Another major hurdle is represented by the ICP/ICP-L immune suppressive circuitry. The interactions between ICP expressed by effector cells (ICOS, CTLA-4, PD-, BTLA, TIM-3) and ICP-L expressed by myeloma cells and bystander cells in the TME (ICOS-L, CD80/CD86, PDL-1/PDL-2, HVEM, GAL-9) impair anti-myeloma immunity. (F) MM is characterized by clonal and subclonal diversity which is shaped over time by repeated treatments, responses, and relapses. This clonal heterogeneity facilitates the immune escape of myeloma cells. (G) The TME immune infiltration discriminates between cold and hot tumors. The former are characterized by the local recruitment and/or activation of immune suppressor cells like Tregs and MDSC; the latter are characterized by the presence of cytotoxic cells (NK, CD8, γδ T cells). Clonal diversity, mutational load, and treatments are key factors to drive the immune infiltration of cold vs. hot tumors. Hot tumors are more sensitive to immunotherapy than cold tumors. The MM TME is closer to cold than hot tumors. Id, idiotype; KLH, keyhole limpet hemocyanin; allo-tx, allogeneic transplantation; HSC, hematopoietic stem cells; MM, multiple myeloma; graft-vs.-leukemia, GVL; graft-vs.-infections, GVI; graft-vs. host desease (GVHD); IMIDs, immunomodulatory drugs; Tregs, regulatory T cells; MDSC, myeloid derived suppressor cell; tumor-associated macrophages (TAM); GN, granulocyte neutrofils; NK, natural killer; ADCC, antibody-dependent-cellular-cytotoxicity; NKT, natural killer T cells; ICP, immune checkpoint; ICP-L, immune checkpoint ligands; TAA: tumor associated antigen.
The unsatisfactory results of immune-based approaches in MM should not generate a pessimistic view. The reasons are rooted in the increased knowledge about the pathogenesis of the disease, the pathophysiology of immune responses, and the innovative technologies available to monitor the disease, assess clinical responses, and develop novel strategies of immune interventions. Additional progresses have been made by shedding some misconceptions like the wisdom that MGUS are immunologically blessed conditions in which myeloma cells are hold in check by very effective immune responses. This misconception was based on mouse models and preclinical results obtained in humans when much less was known about the mechanisms of immune surveillance and immune escape (9). Only recently, this misconception has been breached by us and others revealing that multiple immune dysfunctions are already present in MGUS (10–13).
Another misconception to be abandoned is that the remission state after ASCT represents a unique opportunity for immune interventions since it is possible to achieve a minimal residual disease (MRD) condition in this setting. We have shown more than 10 years ago that the T-cell receptor (TCR) repertoire is highly disrupted in patients in remission after ASCT (14). These results have been confirmed and consolidated (15) explaining why Id vaccination could not fulfil clinical expectations and why lenalidomide maintenance, even nowadays, significantly extends progression free survival (PFS), but does not definitely protect MM patients from late or very late relapse (8).
The time is ripe to apply more informative assays to investigate the immune competence of MGUS and MM. The aim of this minireview is to recapitulate how interrogating the immune competence of BM Vγ9Vδ2T cells has deepen our knowledge about the immune derangement occurring in MGUS and MM patients and how these informations can be applied to design more effective immune interventions in MM.
Vγ9Vδ2 T-cells are non-conventional T cells half-way between adaptive and innate immunity with a natural inclination to react against malignant B cells, including malignant myeloma cells (16). These cells are able to sense supra-physiological concentrations of phosphorylated metabolites (pAgs) generated in the mevalonate (Mev) pathway of mammalian cells. Isopentenyl pyrophosphate (IPP) is the prototypic pAg recognized by Vγ9Vδ2 T cells. The pAgs-reacitivity of Vγ9Vδ2 T cells can be tested in vivo and in vitro by stimulating monocytes or dendritic cells (DC) with aminobisphosphonates like pamidronate or zoledronate (ZA). Both compounds inhibit farnesylpyrophosphate synthase in the Mev pathway (17, 18) and induce intracellular IPP accumulation and extracellular IPP release that are detected by Vγ9Vδ2 T cells. IPP recognition by Vγ9Vδ2 T cells is mediated by the γδ TCR in association with the isoform A1 of the butyrophilin-3 (BTN3A1) protein family (19, 20).
Vγ9Vδ2 T cells are endowed with peculiar functional properties which make them very good candidates for immunotherapy: they do not require MHC restriction and co-stimulation; they produce pro-inflammatory cytokines (IFN-γ and TNF-α); they recognize antigens shared by a variety of stressed and tumor cells; they behave as professional antigen-presenting cells (21); they can provide help to B cells to produce antibodies (22); and they can induce DC maturation boosting αβ T cell priming and MHC-restricted antigen-specific T-cell responses (23). We believe that this multifaceted array of immune functions gives a unique predisposition to Vγ9Vδ2 T cells to behave as very sentitive biosensors of the immune suppressive TME commitment occurring in the BM of MGUS and MM patients (24).
We have previously shown in a large series of patients (MGUS: n = 10; MM at diagnosis: n = 70; MM in remission: n = 52; MM in relapse: n = 24) that BM MM Vγ9Vδ2 T cells are unable to properly react to pAgs stimulation in terms of proliferation, CD107 expression and IFN-γ production. This is an early and long-lasting immune dysfunction, already detectable in MGUS individuals, largely anticipating that of CD8+ T cells and not disappearing even when most of tumor cells have been cleared by ASCT as in MM in remission. The investigation of pAgs reactivity of BM MM Vγ9Vδ2 T cells has been instrumental to show that the frequency of immune suppressor cells in the TME [bone marrow stromal cells (BMSC), regulatory T cells (Tregs) and myeloid-derived suppressor cells (MDSC)] are similar in the BM of MGUS, MM at diagnosis and MM in remission.
Immune checkpoints (ICP) are key regulators of immune activation, immune homeostasis, and autoimmunity driven by interactions with the corresponding ligands (ICP-L) expressed by surrounding cells (25). In cancer, the ICP/ICP-L network is often hijacked by tumor cells to suppress anti-tumor immune responses. This has led to the development of anti-ICP/ICP-L monoclonal antibodies (mAbs) to treat a variety of cancers with heterogenous results.
Among the ICP/ICP-L pairs identified so far, the PD-1/PD-L1 axis plays a major role in the generation of the immune suppressive TME in MM. PD-L1 expression in myeloma cells is higher in MM and SMM than in MGUS and predicts an increased risk of disease progression (26, 27). Paiva et al. have shown a significant upregulation of PD-L1 expression in residual myeloma cells of MM patients who are in first complete remission (27). PD-L1 expression can protect residual myeloma cells from the immune modulation driven by lenalidomide and promote their immune escape and regrowth. Beside myeloma cells, MDSC, and BMSC also express high levels of PD-L1 cells in the BM microenvironment [24 and our unpublished data], underlining a redundancy of immune suppressor cells exploiting the ICP/ICP-L circuitry to hamper anti-myeloma immunity in the TME.
PD-L1 expression is paired by PD-1 overexpression in CD4+ and CD8+ T cells, and NK cells (28–30) isolated from PB and BM of MM patients creating a very effective network to protect myeloma cells from immune recognition and killing. Preliminary data from our laboratory indicate that multiple ICP can be expressed by effector cells, as already reported by Koyama's group in solid tumors (31).
These and other pre-clinical evidences (30, 32, 33) have been the groundwork to introduce anti-PD-1/PD-L1 treatment in MM patients, but clinical results have not met clinical expectations (34–36). These data have confirmed the complexity of the ICP/ICP-L and shown that single PD-1/PD-L1 blockade is insufficient to recover anti-tumor immune responses in MM patients. Investigating the defective pAg reactivity of BM MM Vγ9Vδ2 T cells represent a unique opportunity to identify potential partners and strategies to improve the efficacy of ICP/ICP-L blockade and immune interventions in MGUS and MM.
The unsatisfactory results of anti-PD-1/PD-L1 monotherapy have stimulated the hunt for combinatorial treatment including lenalidomide (28, 37), elotuzumab (anti-SLAMF7) (38), histone deacetylase inhibitors, oncolytic reovirus (39), and radiation therapy (40). Lenalidomide and pomalidomide in combination with pembrolizumab (anti-PD-1) and dexamethasone have progressed up to phase III first-line trials, but unexpected toxicity in the pembrolizumab arm has led to the temporary discontinuation of these trials (https://www.onclive.com/web-exclusives/fda-discloses-data-on-halted-pembrolizumab-myeloma-trials). These hitches are paradigmatic examples how difficult is to carry on immunotherapy studies without a full knowledge about the TME landscape and the local conundrum of tumor-host interactions.
We have shown that a significant fraction of Vγ9Vδ2 T cells that are anergic to pAg stimulation in the TME of MGUS individuals and MM patients are PD-1+ (24). The attempts to fully recover anti-myeloma BM Vγ9Vδ2 T-cell activity in vitro by single PD-1 blockade has failed (24). Investigating the mechanisms of resistance to PD-1 blockade in PD-1+ BM MM Vγ9Vδ2 T cells can provide useful hints to improve the potency of ICP blockade in MM and other diseases.
Multiple ICP expression by immune cells, paired by multiple ICP-L expression in tumor cells and surrounding cells in the TME is emerging as a general mechanism of cancer resistance to ICP blockade. Our preliminary results show that BM MM Vγ9Vδ2 T cells express multiple ICP engaged by the corresponding ICP-L expressed by myeloma cells and bystander cells. ICP-L overexpression in MDSC reinforces their intrinsic immune suppressive commitment, but ICP-L overexpression in endothelial cells and BMSC reflects a contranatural protumoral recruitment operated by myeloma cells in the TME. Our data showing that anergic PD-1+ Vγ9Vδ2 T cells up-regulate PD-1 and express alternative ICP (TIM3, LAG3; that we have defined super-anergic state), if stimulated with pAgs in the presence of single PD-1 blockade, indicates that the TME is reprogrammed to resist any mild and/or insufficient attempt to recover antitumor immune function (Figure 2). This is not very different from what we have learned from chemotherapy when polychemotherapy has replaced single-agent chemotherapy (i.e., ABVD for Hodgkin's disease, R-CHOP for diffuse large B-cell lymphoma, ICE for acute myeloid leukemia etc).
Figure 2. Potential contribution of rescued Vγ9Vδ2 T cells to immune treatments in MM. (A) BM Vγ9Vδ2 T cells in the TME are PD-1+ and anergic to pAg-stimulation. This anergy is not overcome by pAg stimulation in the presence of anti-PD-1. Paradoxically, the pAg + anti-PD-1 combination deepens the anergy of BM MM Vγ9Vδ2 T cells (super-anergic state). One possible hallmark of super-anergic immune effector cells is the expression of multiple ICP (i.e., LAG-3. TIM-3) on the same cells. Combinations of multiple anti-ICP antibodies (anti-PD-1/anti-LAG3/anti-TIM-3) is necessary to overcome the super-anergic state. If rescued from the anergic or super-anergic state, Vγ9Vδ2 T cells can become attractive candidates for immune interventions as proposed in panels B-E. Compared to conventional T cells, Vγ9Vδ2 T cells are not MHC-restricted and can be activated by pAgs (IPP), and stress-induced self ligands (i.e., MICA/B) other than CARs, tumor-specific transferred αβ TCR genes, and BITEs (see also text). (B) The “costimulatory only” CAR Vγ9Vδ2 T cells approach (58). In these cells, the cytotoxic capacity of CD3 (signal 1) is mediated through the native γδ-TCR recognition of IPP, whereas costimulation (signal 2) is provided by a CAR recognizing BCMA with an endodomain consisting of the innate NKG2D signaling molecule, DAP10. The cytotoxic ability of CAR Vγ9Vδ2 T cells is improved by the recognition of other molecules expressed by myeloma cells like MICA/B via NKG2D. (C) BITEs can be used to re-direct CD16-expressing Vγ9Vδ2 T cells against MM antigen (i.e., BCMA) and enhance their cytotoxic anti-tumor activity. (D) Vγ9Vδ2 T cells can act as APC to present TAA to MHC-restricted CD4+ and CD8+ T cells. APC-Vγ9Vδ2 T cells express many APC-related cell surface receptors like MHC-I and II, and co-stimulatory proteins (CD80, CD86). (E) The beneficial activity of Vγ9Vδ2 T cells in the allo-tx settings can be exploited as follows: i) direct infusion of unmanipulated grafts containing small amounts of donor circulating Vγ9Vδ2 T cells; these cells can increase in number after infusion depending on several factors like infections etc; (ii) donor Vγ9Vδ2 T cells from healthy donors are expanded ex-vivo before reinfusion in graft recipients using pAg like zoledronic acid and IL-2 (73); (iii) donor Vγ9Vδ2 T cells are expanded in vivo in the recipient after allo-tx with pAgs like zoledronic acid and IL-2. BM, bone marrow; pAg, phosphoantigen; CAR-T, chimeric antigen receptor-T; co-stim-CAR, “costimulatory only” CAR; BITEs, bispecifc T-cell engager; APC, antigen-presenting cell; TAA, tumor-associated-antigen; allo-Tx, allogenic-transplantation; GVHD, graft-vs.-host disease; GVL, graft-vs.-leukemia; GVI, graft-vs.-infections; IL-2, interleukin-2.
Currently, the most common strategies to overcome the onset of alternative ICP are combinations of multiple anti-ICP antibodies. This approach, supported by in vitro and in vivo data, is impeded by the prohibitive costs and increased side effects and toxicity in the clinical setting. The analysis of the molecular interactions between different ICP (PD-1, TIM-3, LAG-3) in anergic Vγ9Vδ2 T cells could help to identify mechanistic interventions to prevent alternative ICP uregulation and boost the immune potency of ICP inhibitors.
The spectrum of immune interventions has significantly broadened in MM over the last few years thanks to novel findings and technical advances. Immune responses mediated by non-conventional T cells like Vγ9Vδ2 T cells, NKT cells, and CD1a-restricted T cells have gained significant consideration similar to MHC-restricted immune responses mediated by CD8+ cells. The characterization of suppressor cells like MDSC, Tregs, BMSC, and very importantly, the discovery of the ICP/ICP-L network have been other important steps to promote the renaissance of immunotherapy in MM. The identification of additional targets other than Id has led to an unprecedented surge of mAbs directed against myeloma cells (CD38, CD138, SLAMF7, CD138, BCMA), the TME (ICP/ICP-L), or both (CD38, SLAMF7, anti-PD-L1) (41, 42). Notably, CD38-targeted therapy with daratumumab has emerged as of the most effective passive immunotherapy ever developed in MM (43).
Current adoptive immunotherapy approaches under preclinical or clinical investigation include ex-vivo (CAR-T, TCR-engineered T cells) or in vivo redirected T cells [bispecifc T-cell engager (BiTEs)] (44, 45). Clinical trials testing BCMA-redirected CAR-T cells are producing impressive results in heavily pretreated relapsed and/or refractory MM patients (44–49).
TCR-engineered T cells are genetically modified in order to express αβ TCR with enhanced affinity for selected TAA. In contrast to CAR, αβTCR gene transferred cells retain HLA restriction of Ag recognition and are sensitive to intracellular peptides (44, 45). Cancer testis antigens are under investigation as potential TAA in MM patients (50, 52).
Despite a growing enthusiasm, immunotherapy progresses are still facing many hurdles. The majority of MM treated with anti-CD38 mAbs (daratumumab) eventually progress and the mechanisms involved in resistance to daratumumab are largely unknown. CAR T cells also are not free from handicaps like reduced expression of BCMA on myeloma cells, short persistence or loss in vivo of functional CAR T cells (44–49). Bispecific CAR T cells targeting simultaneously two myeloma associated antigens may compensate the decreased BCMA expression, but it may also increase on-target off-tumor toxicity. MHC down-regulation on tumor cells may compromise the therapeutic efficacy of αβTCR gene transferred T cells, whereas the eventual recognition of cross reactive epitopes from alternative target antigens may account for considerable on-target off-tumor toxicity. Autoimmune fatal complications have occurred with MAGE-A3 enhanced affinity αβTCR gene transferred T cells (51). Another drawback of αβTCR gene transfer to conventional CD3+ αβ T cells is the formation of mixed TCR dimers with unknown specificities due to pairing of endogenous and introduced α and β TCR chains (53).
BM Vγ9Vδ2 T cells can be very attractive candidates to deliver antitumor responses in MM, provided that they are rescued from the immune dysfunction they are afflicted. These cells recognize a broader range of targets (including metabolic targets like IPP and self-induced stress ligands) and possess a more favorable safety profile than conventional T cells (16). This unique feature has been exploited to reduce the potential “off target” toxicity of CAR Vγ9Vδ2 T cells (54–57). Fisher et at (58) have designed “costimulatory only” CAR Vγ9Vδ2 T cells in which activation signals 1 and 2 are provided by separate receptors. In these dual-receptor CAR Vγ9Vδ2 T cells, the cytotoxic capacity of CD3 (signal 1) is mediated via the native γδ-TCR recognizing IPP, whereas costimulation (signal 2) is provided by a CAR-mediated recognition of TAA mediated by DAP10, the endodomain consisting of the NKG2D receptor (Figure 2B). Normal healthy tissues which do not express IPP do not activate Vγ9Vδ2 TCR and are spared from Vγ9Vδ2 T cell cytotoxicity. Interestingly, these “costimulation only” CAR Vγ9Vδ2 T cells express lower levels of PD1 and TIM3 than traditional CAR Vγ9Vδ2 T cells after long term culture (58).
Vγ9Vδ2 T cells are excellent candidates for αβTCR gene transfer without the risk of expression of undesired mixed TCR dimers (59). Another interesting approach is to engineer αβ T cells to express tumor-specific Vγ9Vδ2 TCRs (TEGs) to redirect αβ T cells against cancer cells (60). Vγ9Vδ2 TCR-redirected αβ T cells very efficiently kill cancer cell lines in vitro and primary acute myeloid leukemia blasts in a humanized mouse model. Very recently, TEGs have also been generated in MM patients and shown to be able to recognize and kill myeloma cells in a 3D model (61). Vγ9Vδ2 T cells can also be redirected against myeloma cells with BITEs (Figure 2C). The bispecific antibody [(HER2)2xCD16] has been used to re-direct CD16+ Vγ9Vδ2 T cells against Her2+ tumor cells that were killed with very high efficiency (62). HLA-independent recognition of TAA by tumor-redirected CAR Vγ9Vδ2 T cells or BITEs-activated Vγ9Vδ2 T cells may prelude to the development of allogeneic “off the shelf” CAR products.
Another unique feature of Vγ9Vδ2 T cells is their capacity to act as antigen-presenting cells (APC) to boost antigen-specific immune responses mediated by CD8+ cells (21, 63) (Figure 2D). Combination therapy of Vγ9Vδ2 T-APC-based vaccines with ICP blockade may have synergistic activity leading to enhanced anti-tumor immune responses and long-lived immuno-surveillance (64, 65). These adjuvant properties are not lost even after chimerization of Vγ9Vδ2 T cells as demonstrated by Capsomidis A. (57)
Lastly, the multifunctional properties of Vγ9Vδ2 T cells may also be beneficial in the allo-tx setting (allo-tx) (Figure 2E) (66). Vγ9Vδ2 T cells have been reported to cause less graft-vs.-host disease (GVHD) than αβ T cells while retaining graft-vs.-leukemia activity (GVL) (67, 68). A protective effect of Vγ9Vδ2 T cells against both leukemia cell regrowth and infections has been reported in haploidentical HSCT depleted of TCR-αβ/CD19 lymphocytes (69). Lastly, recent studies suggest an overall favorable effect of high Vγ9Vδ2 T cells immune reconstitution after HSCT; patients with elevated numbers of Vγ9Vδ2 T cells had a significantly higher overall survival rate and a decreased rate of acute GVHD compared to patients with low Vγ9Vδ2 T cell counts (70).
Investigation of BM MM Vγ9Vδ2 T cells has been useful to gather a faithfully picture of the immune suppressive TME in MGUS and MM. Understanding the mechanisms that are responsible for BM Vγ9Vδ2 T-cell dysfunction, with special regard to resistance to PD-1 blockade, can help to overcome ICP resistance and safely integrate ICP/ICP-L blockade in the immune treatments of MGUS and MM patients. The use of nanotechnologies may improve delivery of antagonistic antibodies to block ICP inhibitory receptors compared to free antibodies and improve T cell activation (71).
Finally, the functional rescue of BM Vγ9Vδ2 T cells is an attractive opportunity to exploit their multifaceted immune functions to carry on ex-vivo and in vivo adoptive immunotherapy interventions.
All authors have made a substantial contributions to text and figures and have approved the manuscript for submission
This study was supported by the Italian Association for Cancer Research (AIRC) (IG15232 to CR and IG 16985 to MM). B.C. is a post-doc research fellow supported by AIRC (IG 16985).
The authors declare that the research was conducted in the absence of any commercial or financial relationships that could be construed as a potential conflict of interest.
1. Van Nieuwenhuijzen N, Spaan I, Raymakers R, Peperzak V. From MGUS to multiple myeloma, a paradigm for clonal evolution of premalignant cells. Cancer Res. (2018) 78:2449–56. doi: 10.1158/0008-5472.CAN-17-3115
2. Brendan M Weiss. Advances in understanding monoclonal gammopathy of undetermined significance as a precursor of multiple myeloma. Expert Rev Hematol. (2010) 3:165–74. doi: 10.1586/ehm.10.13
3. Bianchi G, Munshi NC. Pathogenesis beyond the cancer clone(s) in multiple myeloma. Blood (2015) 125:3049–58. doi: 10.1182/blood-2014-11-568881
4. Ghobrial IM, Detappe A, Anderson KC, Steensma DP. The bone-marrow niche in MDS and MGUS: implications for AML and MM. Nat Rev Clin Oncol. (2018) 15:219–33. doi: 10.1038/nrclinonc.2017.197
5. Massaia M, Ma DD, Boccadoro M, Golzio F, Gavarotti P, Dianzani U, et al. Decreased ecto-5'nucleotidase activity of peripheral blood lymphocytes in human monoclonal gammopathies: correlation with tumor cell kinetics. Blood (1985) 65:530–4.
6. Massaia M, Borrione P, Battaglio S, Mariani S, Beggiato, Napoli P, et al. Idiotype Vaccination in human myeloma: generation of tumor-specific immune responses after high-dose chemotherapy. Blood (1999) 94:673–83.
7. Rosenblatt J, Avigan D. Role of immune therapies for myeloma. JNCCN (2015) 13:1440–7 doi: 10.6004/jnccn.2015.0168
8. Fostier K, Caers J, Meuleman N, Broos K, Corthals J, Thielemans K, et al. Impact of lenalidomide maintenance on the immune environment of multiple myeloma patients with low tumor burden after autologous stem cell transplantation. Oncotarget (2018) 9:20476–89. doi: 10.18632/oncotarget.24944
9. Calcinotto A, Ponzoni M, Ria R, Grioni M, Cattaneo E, Villa I, et al. Modifications of the mouse bone marrow microenvironment favor angiogenesis and correlate with disease progression from asymptomatic to symptomatic multiple myeloma. Oncoimmunology (2015) 4:e1008850. doi: 10.1080/2162402X.2015.1008850
10. Das R, Strowig T, Verma R, Koduru S, Hafemann A, Hopf S, et al. Microenvironment-dependent growth of preneoplastic and malignant plasma cells in humanized mice. Nat Med. (2016) 22:1351–7. doi: 10.1038/nm.4202
11. Zheng MM, Zhang Z, Bemis K, Belch AR, Pilarski LM, Shively JE, et al. The systemic cytokine environment is permanently altered in multiple myeloma. PLoS ONE (2013) 8:e58504. doi: 10.1371/journal.pone.0058504
12. Foglietta M, Castella B, Mariani S, Coscia M, Godio L, Ferracini R, et al. The bone marrow of myeloma patients is steadily inhabited by a normal-sized pool of functional regulatory T cells irrespective of the disease status. Haematologica (2014) 99:1605–10. doi: 10.3324/haematol.2014.105866
13. Feng X, Acharya C, An G, Wen, Li Zhang K, Kalbasi A, et al. Targeting CD38 suppresses induction and function of T regulatory cells to reverse immunosuppression in multiple myeloma. Blood (2016) 128:2106. doi: 10.1158/1078-0432.CCR-16-3192
14. Toubai T, Hirate D, Shono Y, Ota S, Ibata M, Mashiko S, et al. Chimerism and T-cell receptor repertoire analysis after unrelated cord blood transplantation with a reduced-intensity conditioning regimen following autologous stem cell transplantation for multiple myeloma. Int J Lab Hematol. (2008) 30:75–81. doi: 10.1111/j.1751-553X.2007.00903
15. Chung D J, Katherine B. Pronschinske, Justin A. Shyer, Sneh Sharma, et al. Young. T cell exhaustion in multiple myeloma relapse after autotransplant: optimal timing of immunotherapy. Cancer Immunol Res. (2016) 4:61–71. doi: 10.1158/2326-6066
16. Castella B, Vitale C, Coscia M, Massaia M. Vc9Vd2 T cell-based immunotherapy in hematological malignancies: from bench to bedside. Cell. Mol. Life Sci. (2011) 68:2419–2432. doi: 10.1007/s00018-011-0704-8
17. Kunzmann V, Bauer E, Feurle J, Weissinger F, Tony HP, Wilhelm M. Stimulation of gammadelta T cells by aminobisphosphonates and induction of antiplasmacell activity in multiplemyeloma. Blood (2000) 96:384–92.
18. Thompson K, Rogers MJ. Statins prevent bisphosphonate-induced gammadelta-T-cell proliferation and activation in vitro. J Bone Miner Res. (2004) 19:278–88. doi: 10.1359/JBMR.0301230
19. Riganti C, Castella B, Massaia M. ABCA1, apoA-I, and BTN3A1: a legitimate ménage à trois in dendritic cells. Front Immunol. (2018) 9:1246. doi: 10.3389/fimmu.2018.01246
20. Castella B, Kopecka J, Sciancalepore P, Mandili G, Foglietta M, Mitro N, et al. The ATP-binding cassette transporter A1 regulates phosphoantigen release and Vγ9Vδ2 T cell activation by dendritic cells. Nat Commun. (2017) 8:15663. doi: 10.1038/ncomms15663
21. Brandes M, Willimann K, Bioley G, Lévy N, Eberl M, Luo M, et al. Cross-presenting human gammadelta T cells induce robust CD8+ alphabeta T cell responses. Proc Natl Acad Sci USA (2009) 106:2307–12. doi: 10.1073/pnas.0810059106
22. Born WK, Huang Y, Reinhardt RL, Huang H, Sun D, O'Brien RL. γδ T cells and B cells. Adv Immunol. (2017) 134:1–45. doi: 10.1016/bs.ai.2017.01.002
23. Petrasca A, Doherty DG. Human Vδ2(+) γδ T cells differentially induce maturation, cytokine production, and alloreactive T cell stimulation by dendritic cells and B cells. Front Immunol. (2014) 5:650. doi: 10.3389/fimmu.2014.00650
24. Castella B, Foglietta M, Sciancalepore P, Rigoni M, Coscia M, Griggio V, et al. Anergic bone marrow V9V2 T cells as early and long-lasting markers of PD-1-targetable microenvironment-induced immune suppression in human myeloma. Oncoimmunology (2015) 4:e1047580. doi: 10.1080/2162402X.2015.1047580
25. Pardoll DM. The blockade of immune checkpoints in cancer immunotherapy. Nat Rev Cancer (2012) 12:252–64. doi: 10.1038/nrc3239
26. Liu J, Hamrouni A, Wolowiec D, Coiteux V, Kuliczkowski K, Hetuin D, et al. Plasma cells from multiple myeloma patients express B7-H1 (PD-L1) and increase expression after stimulation with IFN-{gamma} and TLR ligands via a MyD88-, TRAF6-, and MEK-dependent pathway. Blood (2007) 110:296–304. doi: 10.1182/blood-2006-10-051482
27. Paiva B, Azpilikueta A, Puig N, Ocio EM, Sharma R, Oyajobi BO, et al. PD-L1/PD-1 presence in the tumor microenvironment and activity of PD-1 blockade in multiple myeloma. Leukemia (2015) 29:2110–3. doi: 10.1038/leu.2015.79
28. Görgün G, Samur MK, Cowens KB, Paula S, Bianchi G, Anderson JE, et al. Lenalidomide enhances immune checkpoint blockade-induced immune response in multiple myeloma. Clin Cancer Res. (2015) 21:4607–18. doi: 10.1158/1078-0432.CCR-15-0200
29. Rosenblatt J, Glotzbecker B, Mills H, Vasir B, Tzachanis D, Levine JD, et al. PD-1 blockade by CT-011, anti-PD-1 antibody, enhances ex vivo T-cell responses to autologous dendritic cell/myeloma fusion vaccine. J Immunother. (2011) 34:409–18. doi: 10.1097/CJI.0b013e31821ca6ce
30. Benson DM Jr, Bakan CE, Mishra A, Hofmeister CC, Efebera Y, Becknell B, et al. The PD-1/PD-L1 axis modulates the natural killer cell versus multiple myeloma effect: a therapeutic target for CT-011, a novel monoclonal anti-PD-1 antibody. Blood (2010) 116:2286–94. doi: 10.1182/blood-2010-02-271874
31. Koyama S, Akbay EA, Li YY, Herter-Sprie GS, Buczkowski KA, Richards WG, et al. Adaptive resistance to therapeutic PD-1 blockade is associated with upregulation of alternative immune checkpoints. Nat Commun (2016) 7:10501. doi: 10.1038/ncomms10501
32. Hallett WH, Jing W, Drobyski WR, Johnson BD. Immunosuppressive effects of multiple myeloma are overcome by PD-L1 blockade. Biol Blood Marrow Transplant. (2011) 17:1133–45. doi: 10.1016/j.bbmt.2011.03.011
33. Ray A, Das DS, Song Y, Richardson P, Munshi NC, Chauhan D, et al. Targeting PD1-PDL1 immune checkpoint in plasmacytoid dendritic cell interactions with T cells, natural killer cells and multiple myeloma cells. Leukemia (2015) 29:1441–4. doi: 10.1038/leu.2015.11
34. Lesokhin AM, Ansell SM, Armand P, Scott EC, Halwani A, Gutierrez M, et al. Nivolumab in patients with relapsed or refractory hematologic malignancy: preliminary results of a phase Ib Study. J Clin Oncol. (2016) 34:2698–704. doi: 10.1200/JCO.2015.65.9789
35. Suen H, Brown R, Yang S, Ho PJ, Gibson J, Joshua D. The failure of immune checkpoint blockade in multiple myeloma with PD-1 inhibitors in a phase 1 study. Leukemia (2015) 29:1621–2. doi: 10.1038/leu.2015.104
36. Rosenblatt J, Avigan D. Targeting the PD-1/PD-L1 axis in multiple myeloma: a dream or a reality? Blood (2017) 129:275–79. doi: 10.1182/blood-2016-08-731885
37. Giuliani M, Janji B, Berchem G. Activation of NK cells and disruption of PD-L1/PD-1 axis: two different ways for lenalidomide to block myeloma progression. Oncotarget (2017) 8:24031–44. doi: 10.18632/oncotarget.15234
38. Bezman NA, Jhatakia A, Kearney AY, Brender T, Maurer M, Henning K et al. PD-1 blockade enhances elotuzumab efficacy in mouse tumor models. Blood Adv. (2017) 1:753–65. doi: 10.1182/bloodadvances.2017004382
39. Tremblay-LeMay R, Rastgoo N, Chang H. Modulating PD-L1 expression in multiple myeloma: an alternative strategy to target the PD-1/PD-L1 pathway. J Hematol Oncol. (2018) 11:46. doi: 10.1186/s13045-018-0589-1
40. Binder DC, Fu YX, Weichselbaum RR. Radiotherapy and immune checkpoint blockade: potential interactions and future directions. Trends Mol Med. (2015) 463–5. doi: 10.1016/j.molmed.2015.05.007
41. Kumar S. Emerging options in multiple myeloma: targeted, immune, and epigenetic therapies. Hematology Am Soc Hematol Educ Program. (2017) 2017:518–24. doi: 10.1182/asheducation-2017.1.518
42. Köhler M, Greil C, Hudecek M, Lonial S, Raje N, Wäsch R, et al. Current developments in immunotherapy in the treatment of multiple myeloma. Cancer (2018) 124:2075–85. doi: 10.1002/cncr.31243
43. Lokhorst HM, Plesner T, Laubach JP, Nahi H, Gimsing P, Hansson M, et al. Targeting CD38 with Daratumumab Monotherapy in Multiple Myeloma. N Engl J Med. (2015) 373:1207–19. doi: 10.1056/NEJMoa1506348
44. Vallet S, Pecherstorfer M, Podar K. Adoptive cell therapy in multiple Myeloma. Expert Opin Biol Ther. (2017) 17:1511–22. doi: 10.1080/14712598.2017.1375095
45. Danhof S, Hudecek M, Smith EL. CARs and other T cell therapies for MM: The clinical experience. Best Pract Res Clin Haematol. (2018) 31:147–57. doi: 10.1016/j.beha.2018.03.002
46. Ali SA, Shi V, Maric I, Wang M, Stroncek DF, Rose JJ, et al. T cells expressing an anti-B-cell 460 maturation antigen chimeric antigen receptor cause remissions of multiple myeloma. Blood (2016) 128:1688–700. doi: 10.1182/blood-2016-04-711903
47. Cohen AD, Garfall AL, Stadtmauer EA, Lacey SF, Lancaster E, Vogl DT et al. B-Cell Maturation Antigen (BCMA)-Specific Chimeric Antigen Receptor T Cells (CART-BCMA) for Multiple Myeloma (MM): initial safety and efficacy from a phase I study. Blood (2016) 128:1147.
48. Fan F (X), Zhao W, Liu J, He A, Chen Y, Cao X, et al. Durable remissions with BCMA-specific chimeric antigen receptor (CAR)-modified T cells in patients with refractory/relapsed multiple myeloma. J Clin Oncol. (2017) 35:abstr LBA3001. doi: 10.1200/JCO.2017.35.18_suppl.LBA3001
49. Berdeja JG, Yi Lin, Raje NS, DiCapua Siegel DS, Munshi NC, Liedtke M, et al. First-in-human multicenter study of bb2121 anti-BCMA CAR T-cell therapy for relapsed/refractory multiple myeloma: updated results. J Clin Oncol. (2017) 15:3010. doi: 10.1200/JCO.2017.35.15_suppl.3010
50. Rapoport AP, Stadtmauer EA, Binder-Scholl GK, Goloubeva O, Vogl DT, Lacey SF, et al. NY-ESO-1engineered T cells mediate sustained antigen-specific chimeric antigen receptor engineered T cells for the treatment of antitumor effects in myeloma. Nat Med. (2015) 21:914–21 doi: 10.1038/nm.3910
51. Amos SM, Duong CP, Westwood JA, Ritchie DS, Junghans RP, Darcy PK, et al. Autoimmunity associated with immunotherapy of cancer. Blood (2011) 118:499–509 doi: 10.1182/blood-2011-01-325266
52. Caballero OL, Chen YT. Cancer-testis (CT) antigens: potential targets for immunotherapy. Cancer Sci. (2009) 100:2014–21. doi: 10.1111/j.1349-7006.2009.01303.x
53. van der Veken LT, Hagedoorn RS, van Loenen MM, Willemze R, Falkenburg JH, Heemskerk MH. αβ T-cell receptor engineered γδ T cells mediate effective antileukemic reactivity. Cancer Res. (2006) 66:3331–7. doi: 10.1158/0008-5472.CAN-05-4190
54. Rischer M, Pscherer S, Duwe S, Vormoor J, Jurgens H, Rossig C. Human gammadelta T cells as mediators of chimaeric-receptor redirected anti-tumour immunity. Br J Haematol. (2004) 126:583–92. doi: 10.1111/j.1365-2141.2004.05077.x
55. Deniger DC, Switzer K, Mi T, Maiti S, Hurton L, Singh H, et al. Bispecific T-cells expressing polyclonal repertoire of endogenous γδ T-cell receptors and introduced CD19-specific chimeric antigen receptor. Mol Ther. (2013) 21:638–47. doi: 10.1038/mt.2012.267
56. Du SH, Li Z, Chen C, Tan WK, Chi Z, Kwang TW, et al. Co-expansion of cytokine-induced killer cells and Vγ9Vδ2 T cells for CAR T-cell therapy. PLoS ONE (2016) 11:e0161820. doi: 10.1371/journal.pone.0161820
57. Capsomidis A, Benthall G, Van Acker HH, Fisher J, Kramer AM, Abeln Z, et al. Chimeric antigen receptor-engineered human gamma delta T cells: enhanced cytotoxicity with retention of cross presentation. Mol Ther. (2018) 26:354–65. doi: 10.1016/j.ymthe.2017.12.001
58. Fisher J, Wisidagamage Don ND, Flutter B, Capsomidis A, Cheung GW, Gustafsson K, Anderson J. Avoidance of on-target off-tumor activation using a co-stimulation-only chimeric antigen receptor. Mol Ther. (2017) 25:1234–47. doi: 10.1016/j.ymthe.2017.03.002
59. van der Veken LT, Coccoris M, Swart E, Falkenburg JH, Schumacher TN, Heemskerk MH. αβ T cell receptor transfer to γδ T cells generates functional effector cells without mixed TCR dimers in vivo. J Immunol. (2009) 182:164–70. doi: 10.4049/jimmunol.182.1.164
60. Marcu-Malina V, Heijhuurs S, van Buuren M, Hartkamp L, Strand S, Sebestyen Z, et al. Redirecting αβ T cells against cancer cells by transfer of a broadly tumor-reactive γδT-cell receptor. Blood (2011) 118:50–9. doi: 10.1182/blood-2010-12-325993
61. Braham MVJ, Minnema MC, Aarts T, Sebestyen Z, Straetemans T, Vyborova A, et al. Cellular immunotherapy on primary multiple myeloma expanded in a 3D bone marrow niche model. Oncoimmunology (2018) 7:e1434465. doi: 10.1080/2162402X.2018.1434465
62. Oberg HH, Kellner C, Gonnermann D, Sebens S, Bauerschlag D, Gramatzki M, et al. Tribody [(her2)2xcD16] is More effective Than Trastuzumab in enhancing γδ T cell and natural Killer cell cytotoxicity against her2-expressing cancer cells. Front Immunol. (2018) 9:814. doi: 10.3389/fimmu.2018.00814
63. Meuter S, Eberl M, Moser B. Prolonged antigen survival and cytosolic export in cross-presenting human gammadelta T cells. Proc Natl Acad Sci USA. (2010) 107:8730–5. doi: 10.1073/pnas.1002769107
64. Khan MWA, Eberl M, Moser B. Potential use of V9V2 T cells-based vaccines in cancer immunotherapy. Front Immunol. (2014) 5 :512. doi: 10.3389/fimmu.2014.00512
65. van Beek JJ, Wimmers F, Hato SV, de Vries IJ, Sköld AE. Dendritic cell cross talk with innate and innate-like effector cells in antitumor immunity: implications for DC vaccination. Crit Rev Immunol. (2014) 34:517–36.
66. de Witte MA, Sarhan D, Davis Z, Felices M, Vallera DA, Hinderlie P, et al. Early Reconstitution of NK and γδ T Cells and Its Implication for the Design of Post-Transplant Immunotherapy. Biol Blood Marrow Transplant. (2018) 24:1152–62. doi: 10.1016/j.bbmt.2018.02.023
67. Minculescu L, Sengeløv H. The role of gamma delta T cells in haematopoietic stem cell transplantation. Scand J Immunol. (2015) 81:459–68. doi: 10.1111/sji.12289
68. Scheper W1, Gründer C, Straetemans T, Sebestyen Z1, Kuball J1. Hunting for clinical translation with innate-like immune cells and their receptors. Leukemia (2014) 28:1181–90. doi: 10.1038/leu.2013.37
69. Irma Airoldi, Alice Bertaina, Ignazia Prigione, Alessia Zorzoli, Daria Pagliara, Claudia Cocco, et al. gd T-cell reconstitution after HLA-haploidentical hematopoietic transplantation depleted of TCR-ab1/CD191 lymphocytes. Blood (2015) 125:2349–58 doi: 10.1182/blood-2014-09-599423
70. Lamb LS, Pillai S, Langford S, Bowersock J, Stasi AD, Saad A. Clinical-scale manufacturing of γδ T cells for protection against infection and disease recurrence following haploidentical peripheral blood stem cell transplantation and cyclophosphamide gvhd prophylaxis. Bone Marrow Transplant. (2018) 53:766–9. doi: 10.1038/s41409-018-0130-8
71. Hagan CT, Medik YB, Wang AZ. Nanotechnology approaches to improving cancer immunotherapy. Adv Cancer Res. (2018) 139:35–56. doi: 10.1016/bs.acr.2018.05.003
72. Chabannon C, Kuball J, Bondanza A, Dazzi F, Pedrazzoli P, Toubert A, et al. Hematopoietic stem cell transplantation in its 60s: a platform for cellular therapies. Sci Transl Med. (2018) 10:eaap9630. doi: 10.1126/scitranslmed.aap9630
Keywords: Vγ9Vδ2 T cells, immune checkpoint blockade, immunotherapy, tumor vaccination, multiple myeloma
Citation: Castella B, Melaccio A, Foglietta M, Riganti C and Massaia M (2018) Vγ9Vδ2 T Cells as Strategic Weapons to Improve the Potency of Immune Checkpoint Blockade and Immune Interventions in Human Myeloma. Front. Oncol. 8:508. doi: 10.3389/fonc.2018.00508
Received: 02 August 2018; Accepted: 17 October 2018;
Published: 06 November 2018.
Edited by:
Nicola Giuliani, Università degli Studi di Parma, ItalyReviewed by:
Daniel Olive, Aix Marseille Université, FranceCopyright © 2018 Castella, Melaccio, Foglietta, Riganti and Massaia. This is an open-access article distributed under the terms of the Creative Commons Attribution License (CC BY). The use, distribution or reproduction in other forums is permitted, provided the original author(s) and the copyright owner(s) are credited and that the original publication in this journal is cited, in accordance with accepted academic practice. No use, distribution or reproduction is permitted which does not comply with these terms.
*Correspondence: Massimo Massaia, bWFzc2ltby5tYXNzYWlhQHVuaXRvLml0
Disclaimer: All claims expressed in this article are solely those of the authors and do not necessarily represent those of their affiliated organizations, or those of the publisher, the editors and the reviewers. Any product that may be evaluated in this article or claim that may be made by its manufacturer is not guaranteed or endorsed by the publisher.
Research integrity at Frontiers
Learn more about the work of our research integrity team to safeguard the quality of each article we publish.