- 1Key Laboratory for Water Quality and Conservation of the Pearl River Delta, Ministry of Education, Institute of Environmental Research at Greater Bay, Guangzhou University, Guangzhou, China
- 2School of Chemistry and Chemical Engineering, Shandong University, Jinan, China
- 3School of Environmental Science and Engineering, Shandong University, Jinan, China
Dendritic cells (DCs) are the primary antigen-presenting cells and play key roles in the orchestration of the innate and adaptive immune system. Targeting DCs by nanotechnology stands as a promising strategy for cancer immunotherapy. The physicochemical properties of nanoparticles (NPs) influence their interactions with DCs, thus altering the immune outcome of DCs by changing their functions in the processes of maturation, homing, antigen processing and antigen presentation. In this review, we summarize the recent progress in targeting DCs using NPs as a drug delivery carrier in cancer immunotherapy, the recognition of NPs by DCs, and the ways the physicochemical properties of NPs affect DCs' functions. Finally, the molecular pathways in DCs that are affected by NPs are also discussed.
Introduction
The human body relies on two well-coordinated immune mechanisms for foreign invader defense, antigen non-specific innate immunity and highly evolved antigen specific adaptive immunity. The major functions of innate immunity are to rapidly eliminate pathogens and in a late stage, to transmit risk signals to adaptive immunity to activate the specific responses. While macrophages and natural killer (NK) cells primarily degrade and remove pathogens in non-specific ways, antigen processing cells (APCs) process and present pathogen signals to adaptive effector cells including T cells (1) and B cells (2, 3). Dendritic cells (DCs) are professional APCs. Originating from the bone marrow as progenitor cells with high phagocytic capabilities, DCs undergo maturation in the peripheral lymphatic organs. Maturation of DCs is triggered by pathogen uptake and is characterized by morphological changes, the expression of co-stimulatory molecules and the release of cytokines. During the maturation process, DCs migrate to lymphatic organs, where they activate both memory and naïve T cells, and thus are regarded as the most potent APCs. Because of their central role in inducing adaptive immunity, in recent decades, DCs have been extensively studied toward the aim of vaccine development and cancer immunotherapy (4, 5).
Cancer immunotherapy is regarded as an important progress for cancer treatment in the first decade of the 21st century. The success of some small-scale trials based on two major strategies, checkpoint blockage with antibodies and ex vivo T cells engineering, has boosted the development of cancer immunotherapy in recent years (6, 7). Because of the limits of these approaches (8), a third strategy, DC vaccination has been considered (9). Although less developed and with unknown efficacy, some clinical trials based on this approach have shown promise (10, 11). In this strategy, DCs are manipulated by either ex vivo or in vivo approaches. Compared to an in vivo approach in which molecules are directly applied to patients to target DCs, in an ex vivo approach, DC precursors isolated from patients are stimulated in the laboratory for maturation by specific antigen and adjuvants, and then are applied back to the patient to activate adaptive immunity. Until now, the functional equality was unknown between ex vivo and in vivo matured DCs, but studies implicate that DCs undergo maturation differently ex vivo than in vivo.
Nanoparticles (NPs) in human blood and lymph are primarily captured by macrophages in the circulation and in tissues composed of the reticuloendothelial system, such as the liver and spleen. The latest studies revealed that precursor DCs patrolling the blood and immature DCs residing in peripheral tissues, such as the kidney and skin, also actively capture NPs, and subject their functions to alteration. These discoveries encouraged interests in using NPs to control DC functions in favor of cancer immunotherapy. The physicochemical attributes of NPs make them especially intriguing for both ex vivo and in vivo DC manipulation. As a novel DC targeting tool, NPs have at least the following advantages compared to traditional tools. (1) NPs such as gold nanorods (12) and carbon black NPs (13), are adjuvants per se and are able to prime DC maturation, thus enhancing humoral and cellular immune responses by synergizing the immunogenicity of antigens. (2) By optimizing the physicochemical properties, NPs can carry antigens or vaccines and directly deliver them to the mature DCs within the secondary lymph organs. In this way, stronger immune responses can be achieved without the accompanying immune tolerance induced by premature DCs (14–16). (3) NPs protect some antigens, such as peptides, from degradation by proteases (17, 18). (4) Using NPs as a platform, co-delivery of two or even more moieties can be realized to achieve stronger immune responses (17–25). Widely used combinations include tumor antigens together with adjuvants such as Toll-like receptor agonists (18, 23–30), or antigens with siRNAs, which silence immunosuppressive genes (31). This property is especially important for some antigens with weak immunogenicity (18). (5) Within DCs, a sustainable release of therapeutics can be achieved by NP carriers through chemical modification on their surface, thus activating DCs more efficiently (22, 32). Until now, different NPs, such as carbon nanotubes (CNTs) (23–25, 33), gold NPs (16, 34, 35), natural NPs [starch (36) and chitosan (37)] or synthetic polymer NPs (38–40) have been studied as vaccine carriers and/or adjuvants by distinct strategies. Even though the rapid advancement, the status quo is that this is still a newly emerging field and its maturation has been hindered by hurdles such as safety of NPs, and a costly and time-consuming process to harvest DCs from patients. An example of using NPs to stimulate the ex vivo maturation of immature DCs (iDCs) for immunotherapy was shown in Figure 1. NP applications for DC manipulation have been well reviewed in some recent excellent literature (41–43). In this article, we first summarize the latest progress of using NPs for cancer immunotherapy. With the aim of providing mechanistic insights on NPs-DCs interactions, we next focus on the latest knowledge in the recognition and uptake of NPs by DCs, and the ways NPs affect DC functions. We also discuss possible mechanisms underlying these effects.
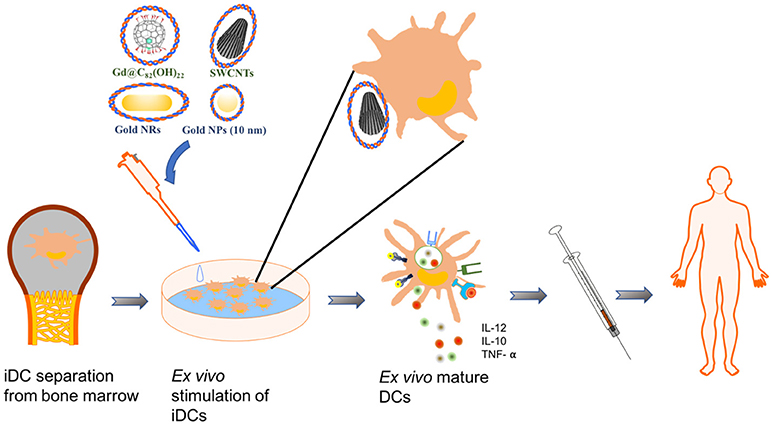
Figure 1. Schematics of an example using NPs for DC-mediated immunotherapy. In this example, immature DCs (iDCs) are harvested from patients' bone marrow. NPs loaded with effect molecules are used to pulse and stimulate the maturation of iDCs ex vivo. The mature DCs were reapplied to patients for immunotherapy.
DC-Targeting Strategy Using NPs for Cancer Immunotherapy
By using NPs as a multifunctional drug delivery carrier for in vivo DC-targeting, some promising cancer immunotherapeutic outcomes have been achieved. In some cases, animal survival rates improved because of the success of tumor growth inhibition. For example, multi-walled CNTs (MWCNTs) loaded with cancer testis antigen and Toll-like receptor agonist were quickly taken up by DCs after administered to animals. Inside DCs, the carried antigen was slowly released, driving DCs to continually activate CD4+ and CD8+ T cell immune responses. Consequently, tumor development was greatly delayed and mouse survival was prolonged (23). In another study, co-delivery of OVA and immune-adjuvants by MWCNTs to DCs dramatically inhibited the growth of OVA-expressing melanoma cells in mice (44).
In some other studies, cancer killing molecules and cytotoxic T cells were activated by a NPs mediated targeting strategy. For example, single-walled CNTs (SWCNTs) carrying a peptide tumor antigen to DCs induced specific IgG responses against this antigen in mice, while there were no such responses when mice were challenged with antigen alone (18). When uploaded by upconversion NPs, the injected ovalbumin enhanced the homing capability of DCs to draining lymph nodes in mice, and significantly induced cytotoxic T lymphocytes and the production of cancer killing molecules such as IFN-γ (17). After administration to mice, gold NPs with polyelectrolyte multilayer coatings increased DC activation and antigen presentation and induced a high level of antigen specific CD8+ T cell immune response in blood (45). Although in these studies cancer inhibition or animal survival data were not reported, the stimulation of cancer killing cytokines and cytotoxic T cells is an indication of better cancer therapeutic efficacy. Some more examples of NPs that were studied for DC-targeted antigen delivery were summarized in Table 1.
Recognition and Uptake of NPs by DCs
In vitro studies using DC models derived from different species (54–56) revealed that, as in other cell types, the uptake of NPs by DCs is either energy dependent or independent (57). Depending on the physicochemical properties of NPs, all four endocytosis pathways are reportedly used by DCs for NPs uptake (Table 2) (52, 60, 63, 64). For example, small sized NPs take a clathrin- and scavenger receptors-dependent pathway (55), while those with diameters larger than 250 nm enter DCs primarily by clathrin independent ways (60–62).
Physicochemical properties of NPs dictate the recognition and uptake process. Recent studies consistently reveal that smaller NPs are easier for DCs to take up. For example, compared to those with a diameter larger than 1 μm, PLGA NPs of 300 nm have the higher uptake efficiency by DCs (64–66). Another example is that polypropylene sulfide NPs with a size of 20 nm accumulated in DCs in the lymph nodes more efficiently than those of 45 and 100 nm, thus inducing more greater immunity (67). Shorter MWCNTs showed better cellular uptake compared to longer ones, and consequently induced more potent immune responses (25, 33). However, there are paradoxical opinions on the relationship between NPs size and their adjuvant activity, since NPs with different material components have their own optimum size for the induction of immune response (68).
Surface chemistry, such as charge and ligand organization pattern, affects NP internalization by DCs. An example is the positive charge on the gold NP surface led to a higher uptake efficiency by human monocyte-derived DCs (69). Early studies showed that the surface hydrophobicity of NPs was correlated with DCs mediated immune effects (70, 71). For example, hydrophobic segments in amphiphilic γ-PGA NPs' surface significantly increased their interactions with DCs and the consequent immune responses (72, 73). Another example is the zwitterionic ligand coated gold NPs (<3 nm in size) had a higher DCs uptake efficiency compared to those coated with PEGylated ligands (58). In one study, DCs took up NPs coated with PEG-3000 in a higher efficiency compared to those coated with shorter or longer PEG chain (74). Phosphatidylserine modification increased the internalization of SWCNTs by DCs compared to pristine ones or those coated with phosphocholine (75). The presence of aromatic structures on the NP surface resulted in the enhanced uptake of negatively charged GNPs and the activation of DCs (76). Not only chemistry type but also structural organization of surface chemical molecules affects the uptake process by DCs. For example, gold NPs coated with organized striations of alternating anionic and hydrophobic groups penetrated the plasma membrane and entered the cytosol, whereas those with randomly organized functional groups of the same composition were mostly trapped in endosomes of DCs (57).
The shape of the NPs also affects the recognition and uptake by DCs. Compared to spherical counterparts of the same size, gold nanorods and peptide nanofibers showed higher uptake efficiency by DCs in regards to the number of internalized NPs per cell (34, 77). Interestingly, only higher uptake of peptide nanofibers led to a stronger adjuvant efficiency, and gold nanorods actually did not. In another study, the uptake efficiency of rod-shaped PEG-based hydrogel NPs by mouse bone marrow-derived DCs was lower than that of disc-shaped NPs with similar volume and dimensions (78).
In in vivo models, physicochemical properties of NPs again govern their interaction with DCs. In one study, cationic NPs more readily associated with both CD11b and CD103 DC subtypes in the lung than anionic ones, thus resulting in a higher expression level of Ccl2 and Cxc10, two important chemokines that recruit DCs into the drainage lymph nodes (79). NPs uptake by DCs in administration sites and the accumulation in drainage lymph nodes was reported after administration by different routes (67, 80–84). In some studies, the uptake of NPs by DCs residing in lymph nodes was also detected (67, 80, 85, 86). Detailed studies showed that the locally injected NPs migrate to lymph nodes by two routes, i.e., through direct draining of NPs, or through DCs migration from injection site to lymph nodes, where NPs are re-taken up (86). Studies suggest that depending on the composition, NPs with a diameter less than 200 nm usually take the first route, while larger ones take the second route (27, 67, 85–88).
NPs Affect DC Functions
In peripheral organs and in the blood, premature DCs internalize pathogens or foreign antigens and migrate to draining lymphoid tissue, where they undergo the maturation process characterized by morphological changes and increased expression of cytokines required for priming T cell and membrane molecules, such as CD40, CD80, CD86, DEC205, and MHC molecules (89). In the draining lymphoid tissues, mature DCs activate effector T cells (90). Mature DCs strongly stimulate naïve and memory T cells by presenting antigens. Depending on the way DCs are activated, T cells are stimulated by DCs to differentiate into distinct lineages of T helper (Th) cells, primarily including Th1, Th2, and Th17, which lead to cellular immunity, humoral immunity, and tissue inflammation, respectively. Recent studies revealed NPs affect all steps of DCs induced immunity.
NPs Affect DC Maturation
Long-term immune protection against tumors or pathogens requires the expansion of antigen-specific effector and memory T cells. Naïve T cell expansion can only be efficiently stimulated by mature DCs; therefore, the maturation of premature DCs is critical for the realization of efficient immunotherapy. Recent studies have shown that NPs favor the maturation process of DCs. For example, in in vitro culture, γ-PGA-Phe NPs induced a significant increase in the expression of maturation markers of DCs, and this capability is size-dependent (39, 86, 87). Gold nanorods were also reported to promote DC maturation and downstream immunity, and this effect depended on their surface chemistry (35, 91). Chitosan and other polymer NPs induced DC maturation in a similar way to LPS treatment (92, 93). According to recent research, ZnO NPs at a concentration of 30 μg/mL upregulated the expression of costimulatory molecules CD80 and CD86, and the secretion of IL-6 and TNF-α (94), but they did not show such effects at lower concentrations (10 μg/mL) (95). These effects are shape-independent, since spherical and sheet-shaped ZnO NPs with similar specific surface area showed similar effects (94). C60 fullerenes (96), carbon black (97, 98), (Gd@C82(OH)22)n (99, 100), and layered double hydroxide (LDH) NPs (101) were all reported to increase the expression of MHC and co-stimulatory molecules on DC surface. In another study, polyanhydrides NPs activated DCs with an efficiency that was dependent on their shape and surface hydrophilicity/hydrophobicity (102).
However, NPs are also reported to inhibit the maturation of DCs. For example, treatment with negatively charged QD655-COOH (18 nm) suppressed the expression of CD80/CD86 stimulated by LPS in porcine monocyte-derived DCs (55). In human DCs, gold NPs with a diameter of 10 nm inhibited the expression of CD86, CD83, and IL-12p70 induced by LPS treatment (103). These findings warrant further investigations to avoid side effects of NPs when used for cancer immunotherapy.
NPs Affect Homing Capability of DCs
To activate T cells, DCs must migrate to lymphoid organs and localize closely to the residence T cells, a process known as DCs homing. A high homing efficiency is critical for a successful DCs-based immunotherapy. The homing of DCs can be improved by approaches including pre-injection of pro-inflammatory cytokines or DC homing receptors (104), and optimization of DCs' administration route and times (105). Even with this, the DC homing efficiency remains unsatisfactory (106). Recently, magnetic NPs have been used under an external magnetic field to promote the homing capability of DCs after in vitro activation (107). Some studies suggested that in vitro pulsed DCs by NPs accumulate in draining lymph nodes (17, 18), however, very little is known about NPs' effects on homing capability of DCs. One study suggested that NPs may improve DC homing by increasing the expression of chemokine receptor 7 (CCR7) on DCs surface and leading to the rearrangement of the cytoskeleton (16).
In addition to chemokines on the surface, it is well known that the maturation status of DCs determines their migration to draining lymph nodes (108). It is possible that NPs affects DCs' homing capability by influencing the maturation status of DCs. Future studies are necessary to further understanding of this aspect.
NPs Compromised Antigen Processing and Presentation Capability of DCs
After digestion by APC cells, antigen fragments will be displayed on the cell surface together with either class I or class II MHC molecules, and consequently are recognized by CD4+ (helper) or CD8+ (cytotoxic) T cells, respectively. This process is known as processing and presentation of APC (109–111). It is well reported that NPs change the antigen processing capability of DCs. In vivo, after pharyngeal aspiration, SWCNTs inhibited DCs' functions of antigen capture/processing and presentation but not their maturation process, thus causing decreased proliferation of splenic T cells (112). In in vitro culture, murine DCs treated with graphene oxide (GO) engulfed antigen normally but showed an impaired capability to process antigen and activate antigen-specific T lymphocytes (96). This effect was specific to GO and was not found for other carbon-based NPs, probably due to its planar and negative charged surface. Another example is that treatment with super-paramagnetic iron oxide NPs (PVA-SPIONs) compromised DCs' capability of processing model antigen DQ-OVA with or without concomitant LPS exposure but did not impair their maturation after antigen uptake. As a consequence, the expression of MHCII and the capacity to stimulate autologous CD4+ T cells in vitro were compromised (113).
Harnessing the ability of DCs to induce antigen-specific CD8+ T cell immunity (cross-presentation) is crucial for development of antitumor vaccines. As potential vaccine carriers, NPs could enhance antigen cross-presentation of DCs, thereby produce stronger antitumor immunity. For example, as shown in both in vivo and in vitro experiments, treatment with polymer NPs such as γ-PGA NPs (114, 115), PLGA NPs (116), polyethyleneimine NPs (117) and poly(propylene sulfide) NPs (52) promoted OVA-mediated cross-presentation in DCs by different mechanisms. Changing size and surface chemistry of NPs were effective ways to regulate their effects on antigen cross-presentation intensity of DCs (118, 119). Some metal oxide NPs such as aluminum hydroxide (120) and super-paramagnetic iron oxide NPs (SPIONs) (121) were also reported to improve the cross-presentation ability of DCs. These above studies suggested a potential strategy of using nanotechnology to develop DCs-based cancer immunotherapy.
NPs Affect DC Induced T Cell Differentiation
NPs have long been known to affect DCs' induction of T cell differentiation. For example, exposure to carbon black NPs or diesel exhaust of different sizes significantly enhanced the capacity of bone marrow-derived DCs to stimulate T-cell proliferation (97, 98). When cultured with CD4+ T cells, human monocyte-derived DCs after treatment with poly(vinylalcohol)-coated SPIONs (PVA-SPIONs) showed an impaired capability to activate CD4+ T cell and altered the cytokine release profiles (113). Mechanistically, NP exposure suppressed DCs' capacity to process and present antigen. In another study, porous silicon NPs modified by high C-H structures strongly enhanced DCs' activation of T cell differentiation (122).
Some NPs promoted DCs capability to stimulate both Th1 and Th2 differentiation (123). However, recent studies revealed that treatment with NPs may bias DC induced T cell differentiation directions. For example, SWCNTs (124), (Gd@C82(OH)22)n (99, 100), magnetic iron oxide NPs (MIONs) (125), oxidative TiO2 NPs (126), and PLGA NPs (127) were reported to increase Th1 cell proliferations, while 10 nm gold NPs (103) and CeO2 NPs (126) potentiate DCs' capability to promote Th2 polarization. Moreover, this polarization effect is related to the surface chemistry. For example, gold nanorods coated with poly(diallydimethylammonium chloride) (PDDAC) and polyethyleneimine (PEI) showed a Th2 polarization activity, while those coated with cetyltrimethylammonium bromide did not (35). In another study, TiO2 NPs induced DC maturation and polarized T cells toward Th1-based responses, while CeO2 NPs treated DCs induced Th2-dominated T cell profile (128). Some NPs, such as polystyrene NPs (50 nm) (129), were found to inhibit Th2 polarization without affecting Th1 immunity. Not only NPs composition but also the NPs treatment conditions determines DCs induction effects on T cell differentiation. For example, SWCNTs at 0.5 μg/mL increased Th1 cell proliferation, while suppressing it at 10 μg/mL (124).
NPs were also reported to affect DCs' induction of T cells to differentiate into Th17 cells. For example, carbon black induced Th17-dependent inflammation in mice (130) and gold NPs of 50 nm (but not 10 nm) favored Th17 polarization (103). In comparison, PLGA NP induced nasal tolerance and inhibited T-cell differentiation into Th17 cells (131).
To sum up, the uptake of NPs affects DCs' functional steps from maturation to induction of T cell differentiation (Figure 2). The NPs' physicochemical properties and exposure scenarios govern the outcomes and intensity of these effects. The interactions between NPs and DCs may favor or impair the functions of DCs in immunotherapy. While some molecular mechanisms have been proposed, more are yet to be revealed.
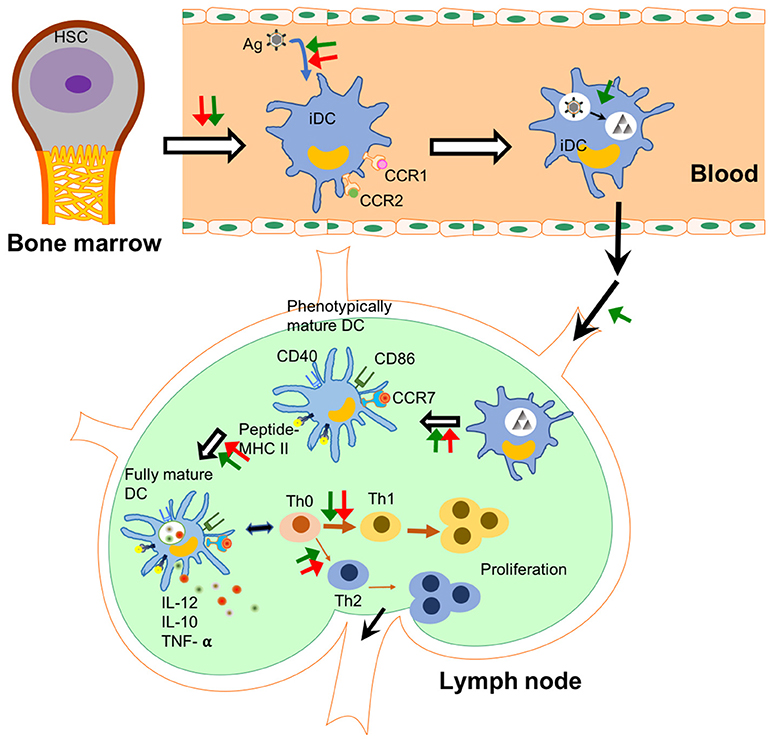
Figure 2. NPs affect DCs' functions in different steps. The checkpoints of DC immunology are shown in this figure and the steps under the probable influence of NPs are summarized. NPs affect the differentiation from haematopoietic stem cells (HSCs) to immature dendritic cells (iDCs) in the bone marrow (132). They change the capability of DCs to uptake and process antigens in peripheral tissues. Some NPs enhance the homing capability of DCs into lymph node. In lymph node, NPs affect antigen presentation capability and maturation of DCs including the release of pro-inflammatory cytokines. Finally, NPs lead to polarization of T cell differentiation induced by DCs. Green arrows show an enhancement effect, while red arrows show an inhibition effect. Black arrows indicate the flow of immune cells.
Possible Mechanisms: Molecular Pathways in DCs Affected by NPs
Understanding the immunomodulatory mechanisms is a premise to optimize the functions of NPs for immunotherapy. Currently available literature supports that the effects of NPs on DCs may be triggered by binding with extracellular membrane receptors or acting on intracellular molecules. Both interactions depend heavily on the physicochemical properties, especially the surface chemistry of NPs.
One possible mechanism by which NPs affect DCs' fate is via the interference of the intracellular signaling pathways after recognition by cell surface receptor(s). Recent studies have proposed TLR (Toll-like receptor)-MyD88 signaling (133) as one of the most likely pathways that mediates NPs' effects. This mechanism was first identified by a micro-array analysis (134) and was later supported by observations in MyD88-knockout and TLR4-deficient DC models and mice (39). For example, after treatment with γ-PGA NPs, the maturation of DCs with MyD88- or TLR4-knockout but not wild-type was impaired. In addition, in wild-type mice, NPs augmented OVA-induced adaptive immune responses, including T cell activation and anti-OVA antibody production, but these effects were largely diminished in TLR4-deficient mice (39). In some studies, the activity of NF-κB and MAPK signaling pathways, both of which are downstream of TLR-MyD88 signaling, was found changed by NPs treatment in DCs (135). These results further support that TLR4-MyD88 is at least one of the signaling pathways that mediated the effects of NPs (Figure 3).
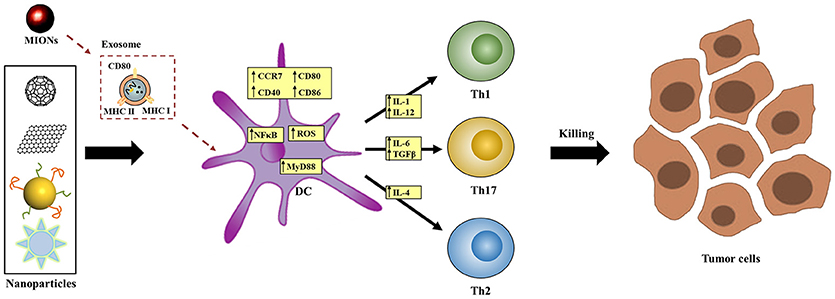
Figure 3. Proposed molecular mechanisms by which NPs affect DCs' functions. NPs may interfere signal transduction (e.g., TLR-MyD88 signaling), exosome-mediated process, intracellular redox balance, or calcium oscillation inside DCs to affect DCs' functional process.
Another reported mechanism is related to the generation of exosomes. In vivo investigations showed that magnetic iron oxide NPs (MIONs) stimulated the generation of exosomes in the alveolar region of mice after respiratory exposure (125, 136). These exosomes transferred to the reticuloendothelial and immune systems, where the maturation of DCs was induced. Ex vivo studies indicated that when incubated with MION-Exo, immature DCs underwent maturation, as shown by the stimulated expression of MHC class II I-Ad, MHC class I H-2Kd, CD80, and CD86, and differentiated into DC1 subtype as shown by the increased secretion of cytokine IL-12. In contrast, MIONs per se did not have the same effects, suggesting that MIONs-induced exosomes mediated the effects of MIONs in stimulating host immunity (Figure 3).
Some other plausible mechanisms have also been proposed. Based on the link between redox equilibrium with phenotypic and functional maturation of DCs (137), oxidative stress may play a key role for DC activation after treatment with NPs including carbon blacks (CBs) (130, 138) and SWCNTs (124). In one study, 10 nm gold NPs were found to inhibit the change of Ca2+ oscillation during LPS-induced DC maturation (103). However, blocking Ca2+ oscillation cannot totally impair DCs' maturation, suggesting that calcium oscillations-dependent signaling is not the sole target of gold NPs in the regulation of DCs' maturation.
Till now, our knowledge about the ways NPs modulate intracellular molecular pathways in DCs is very limit. Since the interactions between NPs and intracellular molecules may be dominated by the physicochemical properties, especially the surface chemistry of NPs, future studies of the structure-activity relationship will help rational design of NPs-based tools to harness immunotherapeutic functions of DCs.
Perspective
For successful DCs-based immunotherapy, three strategies have been considered. First, to deliver tumor specific antigens to DCs and to stimulate their maturation ex vivo followed by re-infusion back to patients. Second, in vivo targeting of DCs with DC-specific targeting molecules together with tumor antigens and activators to induce cytotoxic T cell activation. Finally, in vivo targeting of DCs augments tumor rejection inflammation in the tumor microenvironment (9). For all of these strategies, NPs are perfect antigen or adjuvant delivery carriers of high molecular quantity and variety. In vivo manipulation can better mimic the natural maturation process of DCs, thus can probably produce safer and more efficient immunotherapeutic outcomes. In the future, a deeper understanding of the mechanisms by which the physicochemical properties of NPs affect DCs' functions, such as maturation, homing, antigen process, and induction of T cell differentiation, will be required for safe and efficient DCs-based cancer immunotherapy.
Author Contributions
JJ, YZ and SZ designed this work of review. YX and CJ performed the literature search of the databases. JJ, YZ, and SZ wrote the manuscript. YZ and BY revised the manuscript. All authors approved the paper for publication.
Conflict of Interest Statement
The authors declare that the research was conducted in the absence of any commercial or financial relationships that could be construed as a potential conflict of interest.
Acknowledgments
This work was supported by the National Natural Science Foundation of China (21677090, 91543204 and 91643204), the National Key Research and Development Program of China (2016YFA0203103), the Strategic Priority Research Program of the Chinese Academy of Sciences (XDB14030401), China Postdoctoral Science Foundation (2016T90634), and the Science Starting Foundation of Guangzhou University (69-18ZX10332).
References
1. Shortman K, Liu YJ. Mouse and human dendritic cell subtypes. Nat Rev Immunol. (2002) 2:151–61. doi: 10.1038/nri746
2. Batista FD, Harwood NE. The who, how and where of antigen presentation to B cells. Nat Rev Immunol. (2009) 9:15–27. doi: 10.1038/nri2454
3. Wykes M, MacPherson G. Dendritic cell-B-cell interaction: dendritic cells provide B cells with CD40-independent proliferation signals and CD40-dependent survival signals. Immunology (2000) 100:1–3. doi: 10.1046/j.1365-2567.2000.00044.x
4. Cruz LJ, Tacken PJ, Rueda F, Carles Domingo J, Albericio F, Figdor CG. Targeting nanoparticles to dendritic cells for immunotherapy. Methods Enzymol. (2012) 509:143–63. doi: 10.1016/B978-0-12-391858-1.00008-3
5. Engleman EG. Dendritic cell-based cancer immunotherapy. Semin Oncol. (2003) 30(Suppl. 8):23–9. doi: 10.1016/S0093-7754(03)00229-X
6. Couzin-Frankel J. Cancer Immunotherapy. Science (2013) 342:1432–3. doi: 10.1126/science.342.6165.1432
7. Fan Q, Chen Z, Wang C, Liu Z. Toward biomaterials for enhancing immune checkpoint blockade therapy. Adv Funct Mater (2018) 28:1802540. doi: 10.1002/adfm.201802540
8. Jackson HJ, Rafiq S, Brentjens RJ. Driving CAR T-cells forward. Nat Rev Clin Oncol. (2016) 13:370–83. doi: 10.1038/nrclinonc.2016.36
9. Palucka K, Banchereau J. Cancer immunotherapy via dendritic cells. Nat Rev Cancer (2012) 12:265–77. doi: 10.1038/nrc3258
10. Zhang ZQ, Kim T, Bao MS, Facchinetti V, Jung SY, Ghaffari AA, et al. DDX1, DDX21, and DHX36 helicases form a complex with the adaptor molecule TRIF to sense dsRNA in dendritic cells. Immunity (2011) 34:866–78. doi: 10.1016/j.immuni.2011.03.027
11. Barrat FJ, Meeker T, Gregorio J, Chan JH, Uematsu S, Akira S, et al. Nucleic acids of mammalian origin can act as endogenous ligands for toll-like receptors and may promote systemic lupus erythematosus. J Exp Med. (2005) 202:1131–9. doi: 10.1084/jem.20050914
12. Matsusaki M, Larsson K, Akagi T, Lindstedt M, Akashi M, Borrebaeck CA. Nanosphere induced gene expression in human dendritic cells. Nano Lett. (2005) 5:2168–73. doi: 10.1021/nl050541s
13. de Haar C, Kool M, Hassing I, Bol M, Lambrecht BN, Pieters R. Lung dendritic cells are stimulated by ultrafine particles and play a key role in particle adjuvant activity. J Allergy Clin Immunol. (2008) 121:1246–54. doi: 10.1016/j.jaci.2008.01.010
14. Su H, Mou Y, An Y, Han W, Huang X, Xia G, et al. The migration of synthetic magnetic nanoparticle labeled dendritic cells into lymph nodes with optical imaging. Int J Nanomed. (2013) 8:3737–44. doi: 10.2147/IJN.S52135
15. Chua BY, Al Kobaisi M, Zeng W, Mainwaring D, Jackson DC. Chitosan microparticles and nanoparticles as biocompatible delivery vehicles for peptide and protein-based immunocontraceptive vaccines. Mol Pharm. (2011) 9:81–90. doi: 10.1021/mp200264m
16. Zhou Q, Zhang Y, Du J, Li Y, Zhou Y, Fu Q, et al. Different-sized gold nanoparticle activator/antigen increases dendritic cells accumulation in liver-draining lymph nodes and CD8+ T cell responses. ACS Nano (2016) 10:2678–92. doi: 10.1021/acsnano.5b07716
17. Xiang J, Xu L, Gong H, Zhu W, Wang C, Xu J, et al. Antigen-Loaded upconversion nanoparticles for dendritic cell stimulation, tracking, and vaccination in dendritic cell-based immunotherapy. ACS Nano (2015) 9:6401–11. doi: 10.1021/acsnano.5b02014
18. Villa CH, Dao T, Ahearn I, Fehrenbacher N, Casey E, Rey DA, et al. Single-walled carbon nanotubes deliver peptide antigen into dendritic cells and enhance IgG responses to tumor-associated antigens. ACS Nano (2011) 5:5300–11. doi: 10.1021/nn200182x
19. Sokolova V, Knuschke T, Kovtun A, Buer J, Epple M, Westendorf AM. The use of calcium phosphate nanoparticles encapsulating Toll-like receptor ligands and the antigen hemagglutinin to induce dendritic cell maturation and T cell activation. Biomaterials (2010) 31:5627–33. doi: 10.1016/j.biomaterials.2010.03.067
20. Carrillo-Conde B, Song E-H, Chavez-Santoscoy A, Phanse Y, Ramer-Tait AE, Pohl NL, et al. Mannose-functionalized “pathogen-like” polyanhydride nanoparticles target C-type lectin receptors on dendritic cells. Mol Pharm. (2011) 8:1877–86. doi: 10.1021/mp200213r
21. Petersen LK, Ramer-Tait AE, Broderick SR, Kong CS, Ulery BD, Rajan K, et al. Activation of innate immune responses in a pathogen-mimicking manner by amphiphilic polyanhydride nanoparticle adjuvants. Biomaterials (2011) 32:6815–22. doi: 10.1016/j.biomaterials.2011.05.063
22. Leleux J, Roy K. Micro and nanoparticle-based delivery systems for vaccine immunotherapy: an immunological and materials perspective. Adv Healthc Mater (2013) 2:72–94. doi: 10.1002/adhm.201200268
23. de Faria PCB, dos Santos LI, Coelho JP, Ribeiro HB, Pimenta MA, Ladeira LO, et al. Oxidized multiwalled carbon nanotubes as antigen delivery system to promote superior CD8(+) T cell response and protection against cancer. Nano Lett. (2014) 14:5458–70. doi: 10.1021/nl502911a
24. Sun Z, Wang W, Meng J, Chen S, Xu H, Yang XD. Multi-walled carbon nanotubes conjugated to tumor protein enhance the uptake of tumor antigens by human dendritic cells in vitro. Cell Res. (2010) 20:1170–3. doi: 10.1038/cr.2010.133
25. Hassan HA, Smyth L, Rubio N, Ratnasothy K, Wang JTW, Bansal SS, et al. Carbon nanotubes' surface chemistry determines their potency as vaccine nanocarriers in vitro and in vivo. J Control Release (2016) 225:205–16. doi: 10.1016/j.jconrel.2016.01.030
26. Ballester M, Jeanbart L, De Titta A, Nembrini C, Marsland BJ, Hubbell JA, et al. Nanoparticle conjugation enhances the immunomodulatory effects of intranasally delivered CpG in house dust mite-allergic mice. Sci Rep. (2015) 5:14274. doi: 10.1038/srep14274
27. Nembrini C, Stano A, Dane KY, Ballester M, van der Vlies AJ, Marsland BJ, et al. Nanoparticle conjugation of antigen enhances cytotoxic T-cell responses in pulmonary vaccination. Proc Natl Acad Sci USA. (2011) 108:E989–97. doi: 10.1073/pnas.1104264108
28. Molino NM, Anderson AK, Nelson EL, Wang S-W. Biomimetic protein nanoparticles facilitate enhanced dendritic cell activation and cross-presentation. ACS Nano (2013) 7:9743–52. doi: 10.1021/nn403085w
29. Chong CS, Cao M, Wong WW, Fischer KP, Addison WR, Kwon GS, et al. Enhancement of T helper type 1 immune responses against hepatitis B virus core antigen by PLGA nanoparticle vaccine delivery. J Control Release (2005) 102:85–99. doi: 10.1016/j.jconrel.2004.09.014
30. Ruiz-de-Angulo A, Zabaleta A, Gómez-Vallejo V, Llop J, Mareque-Rivas JC. Microdosed lipid-coated 67Ga-magnetite enhances antigen-specific immunity by image tracked delivery of antigen and CpG to lymph nodes. ACS Nano (2015) 10:1602–18. doi: 10.1021/acsnano.5b07253
31. Heo MB, Lim YT. Programmed nanoparticles for combined immunomodulation, antigen presentation and tracking of immunotherapeutic cells. Biomaterials (2014) 35:590–600. doi: 10.1016/j.biomaterials.2013.10.009
32. Vela Ramirez J, Roychoudhury R, Habte H, Cho M, Pohl N, Narasimhan B. Carbohydrate-functionalized nanovaccines preserve HIV-1 antigen stability and activate antigen presenting cells. J Biomater Sci-Polym Ed. (2014) 25:1387–406. doi: 10.1080/09205063.2014.940243
33. Parra J, Abad-Somovilla A, Mercader JV, Taton TA, Abad-Fuentes A. Carbon nanotube-protein carriers enhance size-dependent self-adjuvant antibody response to haptens. J Control Release (2013) 170:242–51. doi: 10.1016/j.jconrel.2013.05.019
34. Niikura K, Matsunaga T, Suzuki T, Kobayashi S, Yamaguchi H, Orba Y, et al. Gold nanoparticles as a vaccine platform: influence of size and shape on immunological responses in vitro and in vivo. ACS Nano (2013) 7:3926–38. doi: 10.1021/nn3057005
35. Xu L, Liu Y, Chen Z, Li W, Liu Y, Wang L, et al. Surface-engineered gold nanorods: promising DNA vaccine adjuvant for HIV-1 treatment. Nano Lett. (2012) 12:2003–12. doi: 10.1021/nl300027p
36. Heritage PL, Loomes LM, Jianxiong J, Brook MA, Underdown BJ, McDermott MR. Novel polymer-grafted starch microparticles for mucosal delivery of vaccines. Immunology (1996) 88:162–8. doi: 10.1046/j.1365-2567.1996.d01-639.x
38. Uto T, Wang X, Sato K, Haraguchi M, Akagi T, Akashi M, et al. Targeting of antigen to dendritic cells with poly(gamma-glutamic acid) nanoparticles induces antigen-specific humoral and cellular immunity. J Immunol. (2007) 178:2979–86. doi: 10.4049/jimmunol.178.5.2979
39. Uto T, Akagi T, Yoshinaga K, Toyama M, Akashi M, Baba M. The induction of innate and adaptive immunity by biodegradable poly (γ-glutamic acid) nanoparticles via a TLR4 and MyD88 signaling pathway. Biomaterials (2011) 32:5206–12. doi: 10.1016/j.biomaterials.2011.03.052
40. Mundargi RC, Babu VR, Rangaswamy V, Patel P, Aminabhavi TM. Nano/micro technologies for delivering macromolecular therapeutics using poly(D,L-lactide-co-glycolide) and its derivatives. J Control Release (2008) 125:193–209. doi: 10.1016/j.jconrel.2007.09.013
41. Klippstein R, Pozo D. Nanotechnology-based manipulation of dendritic cells for enhanced immunotherapy strategies. Nanomed-Nanotechnol Biol Med (2010) 6:523–9. doi: 10.1016/j.nano.2010.01.001
42. Paulis LE, Mandal S, Kreutz M, Figdor CG. Dendritic cell-based nanovaccines for cancer immunotherapy. Curr Opin Immunol. (2013) 25:389–95. doi: 10.1016/j.coi.2013.03.001
43. Wang C, Ye Y, Hu Q, Bellotti A, Gu Z. Tailoring biomaterials for cancer immunotherapy: emerging trends and future outlook. Adv Mater. (2017) 29:1606036. doi: 10.1002/adma.201606036
44. Hassan HAFM, Smyth L, Wang JTW, Costa PM, Ratnasothy K, Diebold SS, et al. Dual stimulation of antigen presenting cells using carbon nanotube-based vaccine delivery system for cancer immunotherapy. Biomaterials (2016) 104:310–22. doi: 10.1016/j.biomaterials.2016.07.005
45. Zhang P, Chiu Y-C, Tostanoski LH, Jewell CM. Polyelectrolyte multilayers assembled entirely from immune signals on gold nanoparticle templates promote antigen-specific T cell response. ACS Nano (2015) 9:6465–77. doi: 10.1021/acsnano.5b02153
46. Cruz LJ, Rueda F, Simón L, Cordobilla B, Albericio F, Domingo JC. Liposomes containing NY-ESO-1/tetanus toxoid and adjuvant peptides targeted to human dendritic cells via the Fc receptor for cancer vaccines. Nanomedicine (2014) 9:435–49. doi: 10.2217/NNM.13.66
47. Van Broekhoven CL, Parish CR, Demangel C, Britton WJ, Altin JG. Targeting dendritic cells with antigen-containing liposomes: a highly effective procedure for induction of antitumor immunity and for tumor immunotherapy. Cancer Res. (2004) 64:4357–65. doi: 10.1158/0008-5472.CAN-04-0138
48. Yoshizaki Y, Yuba E, Sakaguchi N, Koiwai K, Harada A, Kono K. Potentiation of pH-sensitive polymer-modified liposomes with cationic lipid inclusion as antigen delivery carriers for cancer immunotherapy. Biomaterials (2014) 35:8186–96. doi: 10.1016/j.biomaterials.2014.05.077
49. Jahan ST, Sadat SM, Haddadi A. Design and immunological evaluation of anti-CD205-tailored PLGA-based nanoparticulate cancer vaccine. Int J Nanomed. (2018) 13:367. doi: 10.2147/IJN.S144266
50. Cruz LJ, Tacken PJ, Fokkink R, Joosten B, Stuart MC, Albericio F, et al. Targeted PLGA nano-but not microparticles specifically deliver antigen to human dendritic cells via DC-SIGN in vitro. J Control Release (2010) 144:118–26. doi: 10.1016/j.jconrel.2010.02.013
51. Yoshikawa T, Okada N, Oda A, Matsuo K, Matsuo K, Mukai Y, et al. Development of amphiphilic γ-PGA-nanoparticle based tumor vaccine: potential of the nanoparticulate cytosolic protein delivery carrier. Biochem Biophys Res Commun (2008) 366:408–13. doi: 10.1016/j.bbrc.2007.11.153
52. Hirosue S, Kourtis IC, van der Vlies AJ, Hubbell JA, Swartz MA. Antigen delivery to dendritic cells by poly (propylene sulfide) nanoparticles with disulfide conjugated peptides: cross-presentation and T cell activation. Vaccine (2010) 28:7897–906. doi: 10.1016/j.vaccine.2010.09.077
53. Hangalapura BN, Oosterhoff D, de Groot J, Boon L, Tuting T, van den Eertwegh AJ, et al. Potent anti-tumor immunity generated by a CD40-targeted adenoviral vaccine. Cancer Res. (2011) 71:5827–37. doi: 10.1158/0008-5472.CAN-11-0804
54. Le Guével X, Perez Perrino M, Fernández TD, Palomares F, Torres M-J, Blanca M, et al. Multivalent glycosylation of fluorescent gold nanoclusters promotes increased human dendritic cell targeting via multiple endocytic pathways. ACS Appl Mater Interfaces (2015) 7:20945–56. doi: 10.1021/acsami.5b06541
55. Zhang LW, Bäumer W, Monteiro-Riviere NA. Cellular uptake mechanisms and toxicity of quantum dots in dendritic cells. Nanomedicine (2011) 6:777–91. doi: 10.2217/nnm.11.73
56. Silva JM, Vandermeulen G, Oliveira VG, Pinto SN, Rodrigues C, Salgado A, et al. Development of functionalized nanoparticles for vaccine delivery to dendritic cells: a mechanistic approach. Nanomedicine (2014) 9:2639–56. doi: 10.2217/nnm.14.135
57. Verma A, Uzun O, Hu Y, Hu Y, Han H-S, Watson N, et al. Surface-structure-regulated cell-membrane penetration by monolayer-protected nanoparticles. Nat Mater (2008) 7:588–95. doi: 10.1038/nmat2202
58. Fernández TD, Pearson JR, Leal MP, Torres MJ, Blanca M, Mayorga C, et al. Intracellular accumulation and immunological properties of fluorescent gold nanoclusters in human dendritic cells. Biomaterials (2015) 43:1–12. doi: 10.1016/j.biomaterials.2014.11.045
59. Migdal C, Rahal R, Rubod A, Callejon S, Colomb E, Atrux-Tallau N, et al. Internalisation of hybrid titanium dioxide/para-amino benzoic acid nanoparticles in human dendritic cells did not induce toxicity and changes in their functions. Toxicol Lett. (2010) 199:34–42. doi: 10.1016/j.toxlet.2010.07.017
60. Coester C, Nayyar P, Samuel J. In vitro uptake of gelatin nanoparticles by murine dendritic cells and their intracellular localisation. Eur J Pharm Biopharm. (2006) 62:306–14. doi: 10.1016/j.ejpb.2005.09.009
61. Elamanchili P, Diwan M, Cao M, Samuel J. Characterization of poly(d,l-lactic-co-glycolic acid) based nanoparticulate system for enhanced delivery of antigens to dendritic cells. Vaccine (2004) 22:2406–12. doi: 10.1016/j.vaccine.2003.12.032
62. Lutsiak MEC, Robinson D, Coester C, Kwon G, Samuel J. Analysis of poly(D,L-Lactic-Co-Glycolic Acid) nanosphere uptake by human dendritic cells and macrophages in vitro. Pharm Res. (2002) 19:1480–7. doi: 10.1023/a:1020452531828
63. Pelkmans L. Secrets of caveolae- and lipid raft-mediated endocytosis revealed by mammalian viruses. Biochim Biophys Acta Mol Cell Res. (2005) 1746:295–304. doi: 10.1016/j.bbamcr.2005.06.009
64. Xiang SD, Scholzen A, Minigo G, David C, Apostolopoulos V, Mottram PL, et al. Pathogen recognition and development of particulate vaccines: does size matter? Methods (2006) 40:1–9. doi: 10.1016/j.ymeth.2006.05.016
65. Oyewumi MO, Kumar A, Cui Z. Nano-microparticles as immune adjuvants: correlating particle sizes and the resultant immune responses. Expert Rev Vaccines (2010) 9:1095–107. doi: 10.1586/erv.10.89
66. Joshi VB, Geary SM, Salem AK. Biodegradable particles as vaccine delivery systems: size matters. AAPS J. (2013) 15:85–94. doi: 10.1208/s12248-012-9418-6
67. Reddy ST, van der Vlies AJ, Simeoni E, Angeli V, Randolph GJ, O'Neil CP, et al. Exploiting lymphatic transport and complement activation in nanoparticle vaccines. Nat Biotechnol. (2007) 25:1159–64. doi: 10.1038/nbt1332
68. Yan S, Gu W, Xu ZP. Re-considering how particle size and other properties of antigen–adjuvant complexes impact on the immune responses. J Colloid Interface Sci. (2013) 395:1–10. doi: 10.1016/j.jcis.2012.11.061
69. Fytianos K, Rodriguez-Lorenzo L, Clift MJ, Blank F, Vanhecke D, Von Garnier C, et al. Uptake efficiency of surface modified gold nanoparticles does not correlate with functional changes and cytokine secretion in human dendritic cells in vitro. Nanomed Nanotechnol Biol Med. (2015) 11:633–44. doi: 10.1016/j.nano.2014.11.004
70. Moyano DF, Goldsmith M, Solfiell DJ, Landesman-Milo D, Miranda OR, Peer D, et al. Nanoparticle hydrophobicity dictates immune response. J Am Chem Soc. (2012) 134:3965–7. doi: 10.1021/ja2108905
71. Seong SY, Matzinger P. Hydrophobicity: an ancient damage-associated molecular pattern that initiates innate immune responses. Nat Rev Immunol. (2004) 4:469–78. doi: 10.1038/nri1372
72. Shima F, Akagi T, Akashi M. Effect of hydrophobic side chains in the induction of immune responses by nanoparticle adjuvants consisting of amphiphilic poly (γ-glutamic acid). Bioconj Chem. (2015) 26:890–8. doi: 10.1021/acs.bioconjchem.5b00106
73. Shima F, Akagi T, Uto T, Akashi M. Manipulating the antigen-specific immune response by the hydrophobicity of amphiphilic poly (γ-glutamic acid) nanoparticles. Biomaterials (2013) 34:9709–16. doi: 10.1016/j.biomaterials.2013.08.064
74. Cruz LJ, Tacken PJ, Fokkink R, Figdor CG. The influence of PEG chain length and targeting moiety on antibody-mediated delivery of nanoparticle vaccines to human dendritic cells. Biomaterials (2011) 32:6791–803. doi: 10.1016/j.biomaterials.2011.04.082
75. Konduru NV, Tyurina YY, Feng W, Basova LV, Belikova NA, Bayir H, et al. Phosphatidylserine targets single-walled carbon nanotubes to professional phagocytes in vitro and in vivo. PLoS ONE (2009) 4:e4398. doi: 10.1371/journal.pone.0004398
76. Yang H, Zhou Y, Fung S-Y, Wu L, Tsai K, Tan R, et al. Amino acid structure determines the immune responses generated by peptide-gold nanoparticle hybrids. Part Part Syst Charact. (2013) 30:1039–43. doi: 10.1002/ppsc.201300213
77. Mammadov R, Cinar G, Gunduz N, Goktas M, Kayhan H, Tohumeken S, et al. Virus-like nanostructures for tuning immune response. Sci Rep. (2015) 5:16728. doi: 10.1038/srep16728
78. Agarwal R, Singh V, Jurney P, Shi L, Sreenivasan S, Roy K. Mammalian cells preferentially internalize hydrogel nanodiscs over nanorods and use shape-specific uptake mechanisms. Proc Natl Acad Sci USA. (2013) 110:17247-52. doi: 10.1073/pnas.1305000110
79. Fromen CA, Rahhal TB, Robbins GR, Kai MP, Shen TW, Luft JC, et al. Nanoparticle surface charge impacts distribution, uptake and lymph node trafficking by pulmonary antigen-presenting cells. Nanomed Nanotechnol Biol Med. (2016) 12:677–87. doi: 10.1016/j.nano.2015.11.002
80. Reddy ST, Rehor A, Schmoekel HG, Hubbell JA, Swartz MA. In vivo targeting of dendritic cells in lymph nodes with poly (propylene sulfide) nanoparticles. J Control Release (2006) 112:26–34. doi: 10.1016/j.jconrel.2006.01.006
81. Mahe B, Vogt A, Liard C, Duffy D, Abadie V, Bonduelle O, et al. Nanoparticle-based targeting of vaccine compounds to skin antigen-presenting cells by hair follicles and their transport in mice. J Invest Dermatol. (2009) 129:1156–64. doi: 10.1038/jid.2008.356
82. Rancan F, Gao Q, Graf C, Troppens S, Hadam S, Hackbarth S, et al. Skin penetration and cellular uptake of amorphous silica nanoparticles with variable size, surface functionalization, and colloidal stability. ACS Nano (2012) 6:6829–42. doi: 10.1021/nn301622h
83. Combadière B, Mahé B. Particle-based vaccines for transcutaneous vaccination. Comp Immunol Microbiol Infect Dis. (2008) 31:293–315. doi: 10.1016/j.cimid.2007.07.015
84. Kenney RT, Frech SA, Muenz LR, Villar CP, Glenn GM. Dose sparing with intradermal injection of influenza vaccine. N Engl J Med. (2004) 351:2295–301. doi: 10.1056/NEJMoa043540
85. Manolova V, Flace A, Bauer M, Schwarz K, Saudan P, Bachmann MF. Nanoparticles target distinct dendritic cell populations according to their size. Eur J Immunol. (2008) 38:1404–13. doi: 10.1002/eji.200737984
86. Shima F, Uto T, Akagi T, Baba M, Akashi M. Size effect of amphiphilic poly (γ-glutamic acid) nanoparticles on cellular uptake and maturation of dendritic cells in vivo. Acta Biomater. (2013) 9:8894–901. doi: 10.1016/j.actbio.2013.06.010
87. Kim H, Uto T, Akagi T, Baba M, Akashi M. Amphiphilic poly (amino acid) nanoparticles induce size-dependent dendritic cell maturation. Adv Funct Mater (2010) 20:3925–31. doi: 10.1002/adfm.201000021
88. Kourtis IC, Hirosue S, De Titta A, Kontos S, Stegmann T, Hubbell JA, et al. Peripherally administered nanoparticles target monocytic myeloid cells, secondary lymphoid organs and tumors in mice. PLoS ONE (2013) 8:e61646. doi: 10.1371/journal.pone.0061646
89. e Sousa CR. Dendritic cells in a mature age. Nat Rev Immunol. (2006) 6:476–83. doi: 10.1038/nri1845
90. Lambrecht BN, Hammad H. The role of dendritic and epithelial cells as master regulators of allergic airway inflammation. Lancet (2010) 376:835–43. doi: 10.1016/S0140-673661226-3
91. Kieng Bao V, Safina I, Darrigues E, Nedosekin D, Nima ZA, Majeed W, et al. Modifying dendritic cell activation with plasmonic nano vectors. Sci Rep. (2017) 7:5513. doi: 10.1038/s41598-017-04459-1
92. Babensee JE, Paranjpe A. Differential levels of dendritic cell maturation on different biomaterials used in combination products. J Biomed Mater Res Part A (2005) 74:503–10. doi: 10.1002/jbm.a.30429
93. Gong H, Xiang J, Xu L, Song X, Dong Z, Peng R, et al. Stimulation of immune systems by conjugated polymers and their potential as an alternative vaccine adjuvant. Nanoscale (2015) 7:19282–92. doi: 10.1039/c5nr06081h
94. Heng BC, Zhao X, Tan EC, Khamis N, Assodani A, Xiong S, et al. Evaluation of the cytotoxic and inflammatory potential of differentially shaped zinc oxide nanoparticles. Arch Toxicol. (2011) 85:1517–28. doi: 10.1007/s00204-011-0722-1
95. Andersson-Willman B, Gehrmann U, Cansu Z, Buerki-Thurnherr T, Krug HF, Gabrielsson S, et al. Effects of subtoxic concentrations of TiO2 and ZnO nanoparticles on human lymphocytes, dendritic cells and exosome production. Toxicol Appl Pharmacol. (2012) 264:94–103. doi: 10.1016/j.taap.2012.07.021
96. Tkach AV, Yanamala N, Stanley S, Shurin MR, Shurin GV, Kisin ER, et al. Graphene oxide, but not fullerenes, targets immunoproteasomes and suppresses antigen presentation by dendritic cells. Small (2013) 9:1686–90. doi: 10.1002/smll.201201546
97. Porter M, Karp M, Killedar S, Bauer SM, Guo J, Williams DA, et al. Diesel-enriched particulate matter functionally activates human dendritic cells. Am J Respir Cell Mol Biol. (2007) 37:706–19. doi: 10.1165/rcmb.2007-0199OC
98. Koike E, Takano H, Inoue KI, Yanagisawa R, Kobayashi T. Carbon black nanoparticles promote the maturation and function of mouse bone marrow-derived dendritic cells. Chemosphere (2008) 73:371–6. doi: 10.1016/j.chemosphere.2008.05.054
99. Yang D, Zhao Y, Guo H, Li Y, Tewary P, Xing G, et al. [Gd@ C82 (OH)22] n nanoparticles induce dendritic cell maturation and activate Th1 immune responses. ACS Nano (2010) 4:1178–86. doi: 10.1021/nn901478z
100. Liu Y, Jiao F, Qiu Y, Li W, Lao F, Zhou GQ, et al. The effect of Gd@C-82(OH) nanoparticles on the release of Th1/Th2 cytokines and induction of TNF-alpha mediated cellular immunity. Biomaterials (2009) 30:3934–45. doi: 10.1016/j.biomaterials.2009.04.001
101. Li A, Qin LL, Zhu D, Zhu RR, Sun J, Wang SL. Signalling pathways involved in the activation of dendritic cells by layered double hydroxide nanoparticles. Biomaterials (2010) 31:748–56. doi: 10.1016/j.biomaterials.2009.09.095
102. Petersen LK, Xue L, Wannemuehler MJ, Rajan K, Narasimhan B. The simultaneous effect of polymer chemistry and device geometry on the in vitro activation of murine dendritic cells. Biomaterials (2009) 30:5131–42. doi: 10.1016/j.biomaterials.2009.05.069
103. Tomić S, Dokić J, Vasilijić S, Ogrinc N, Rudolf R, Pelicon P, et al. Size-dependent effects of gold nanoparticles uptake on maturation and antitumor functions of human dendritic cells in vitro. PLoS ONE (2014) 9:e96584. doi: 10.1371/journal.pone.0096584
104. Okada N, Mori N, Koretomo R, Okada Y, Nakayama T, Yoshie O, et al. Augmentation of the migratory ability of DC-based vaccine into regional lymph nodes by efficient CCR7 gene transduction. Gene Ther. (2005) 12:129–39. doi: 10.1038/sj.gt.3302358
105. Xu H, Cao X. Dendritic cell vaccines in cancer immunotherapy: from biology to translational medicine. Front Med. (2011) 5:323–32. doi: 10.1007/s11684-011-0172-4
106. Verdijk P, Aarntzen E, Punt CJA, de Vries IJM, Figdor CG. Maximizing dendritic cell migration in cancer immunotherapy. Expert Opin Biol Ther. (2008) 8:865–74. doi: 10.1517/14712590802102740
107. Jin HL, Qian Y, Dai YF, Qiao S, Huang C, Lu LS, et al. Magnetic enrichment of dendritic cell vaccine in lymph node with fluorescent-magnetic nanoparticles enhanced cancer immunotherapy. Theranostics (2016) 6:2000–14. doi: 10.7150/thno.15102
108. de Vries IJM, Krooshoop D, Scharenborg NM, Lesterhuis WJ, Diepstra JHS, van Muijen GNP, et al. Effective migration of antigen-pulsed dendritic cells to lymph nodes in melanoma patients is determined by their maturation state. Cancer Res. (2003) 63:12–7.
109. Blum JS, Wearsch PA, Cresswell P. Pathways of Antigen Processing. Annu Rev Immunol. (2013) 31:443–73. doi: 10.1146/annurev-immunol-032712-095910
110. Munz C. Autophagy Beyond Intracellular MHC Class II Antigen Presentation. Trends Immunol. (2016) 37:755–63. doi: 10.1016/j.it.2016.08.017
111. Mak T, Saunders M, Jett B. Chapter 7 - Antigen Processing and Presentation. Primer to the Immune Response. 2nd ed. Boston: Academic Cell (2014). p. 161–79
112. Tkach AV, Shurin GV, Shurin MR, Kisin ER, Murray AR, Young S-H, et al. Direct effects of carbon nanotubes on dendritic cells induce immune suppression upon pulmonary exposure. ACS Nano (2011) 5:5755–62. doi: 10.1021/nn2014479
113. Blank F, Gerber P, Rothen-Rutishauser B, Sakulkhu U, Salaklang J, De Peyer K, et al. Biomedical nanoparticles modulate specific CD4+ T cell stimulation by inhibition of antigen processing in dendritic cells. Nanotoxicology (2011) 5:606–21. doi: 10.3109/17435390.2010.541293
114. Mukai Y, Yoshinaga T, Yoshikawa M, Matsuo K, Yoshikawa T, Matsuo K, et al. Induction of endoplasmic reticulum–endosome fusion for antigen cross-presentation induced by poly (γ-Glutamic Acid) nanoparticles. J Immunol. (2011):1001093. doi: 10.4049/jimmunol.1001093
115. Yoshikawa T, Okada N, Oda A, Matsuo K, Matsuo K, Kayamuro H, et al. Nanoparticles built by self-assembly of amphiphilic γ-PGA can deliver antigens to antigen-presenting cells with high efficiency: a new tumor-vaccine carrier for eliciting effector T cells. Vaccine (2008) 26:1303–13. doi: 10.1016/j.vaccine.2007.12.037
116. Song C, Noh YW, Lim YT. Polymer nanoparticles for cross-presentation of exogenous antigens and enhanced cytotoxic T-lymphocyte immune response. Int J Nanomed. (2016) 11:3753. doi: 10.2147/IJN.S110796
117. Chen J, Li Z, Huang H, Yang Y, Ding Q, Mai J, et al. Improved antigen cross-presentation by polyethyleneimine-based nanoparticles. Int J Nanomed. (2011) 6:77–84. doi: 10.2147/IJN.S15457
118. Han R, Zhu J, Yang X, Xu H. Surface modification of poly (d, l-lactic-co-glycolic acid) nanoparticles with protamine enhanced cross-presentation of encapsulated ovalbumin by bone marrow-derived dendritic cells. J Biomed Mater Res Part A (2011) 96:142–9. doi: 10.1002/jbm.a.32860
119. Yang YW, Hsu PYJ. The effect of poly (D, L-lactide-co-glycolide) microparticles with polyelectrolyte self-assembled multilayer surfaces on the cross-presentation of exogenous antigens. Biomaterials (2008) 29:2516–26. doi: 10.1016/j.biomaterials.2008.02.015
120. Dong H, Wen Z-F, Chen L, Zhou N, Liu H, Dong S, et al. Polyethyleneimine modification of aluminum hydroxide nanoparticle enhances antigen transportation and cross-presentation of dendritic cells. Int J Nanomed. (2018) 13:3353. doi: 10.2147/IJN.S164097
121. Mou Y, Xing Y, Ren H, Cui Z, Zhang Y, Yu G, et al. The effect of superparamagnetic iron oxide nanoparticle surface charge on antigen cross-presentation. Nanoscale Res Lett. (2017) 12:52. doi: 10.1186/s11671-017-1828-z
122. Shahbazi M-A, Fernández TD, Mäkilä EM, Le Guével X, Mayorga C, Kaasalainen MH, et al. Surface chemistry dependent immunostimulative potential of porous silicon nanoplatforms. Biomaterials (2014) 35:9224–35. doi: 10.1016/j.biomaterials.2014.07.050
123. Wang X, Li X, Ito A, Watanabe Y, Sogo Y, Hirose M, et al. Rod-shaped and substituted hydroxyapatite nanoparticles stimulating type 1 and 2 cytokine secretion. Colloids Surf B Biointerfaces (2016) 139:10–6. doi: 10.1016/j.colsurfb.2015.12.004
124. Inoue K-i, Yanagisawa R, Koike E, Nishikawa M, Takano H. Repeated pulmonary exposure to single-walled carbon nanotubes exacerbates allergic inflammation of the airway: possible role of oxidative stress. Free Radic Biol Med. (2010) 48:924–34. doi: 10.1016/j.freeradbiomed.2010.01.013
125. Zhu M, Tian X, Song X, Li Y, Tian Y, Zhao Y, et al. Nanoparticle-induced exosomes target antigen-presenting cells to initiate Th1-type immune activation. Small (2012) 8:2841–8. doi: 10.1002/smll.201200381
126. Holmstrom KM, Finkel T. Cellular mechanisms and physiological consequences of redox-dependent signalling. Nat Rev Mol Cell Biol. (2014) 15:411–21. doi: 10.1038/nrm3801
127. Lutsiak MEC, Kwon GS, Samuel J. Biodegradable nanoparticle delivery of a Th2-biased peptide for induction of Th1 immune responses. J Pharm Pharmacol. (2006) 58:739–47. doi: 10.1211/jpp.58.6.0004
128. Schanen BC, Das S, Reilly CM, Warren WL, Self WT, Seal S, et al. Immunomodulation and T helper TH1/TH2 response polarization by CeO2 and TiO2 nanoparticles. PLoS ONE (2013) 8:e62816. doi: 10.1371/journal.pone.0062816
129. Hardy CL, LeMasurier JS, Belz GT, Scalzo-Inguanti K, Yao J, Xiang SD, et al. Inert 50-nm polystyrene nanoparticles that modify pulmonary dendritic cell function and inhibit allergic airway inflammation. J Immunol. (2012) 188:1431–41. doi: 10.4049/jimmunol.1100156
130. You R, Lu W, Shan M, Berlin JM, Samuel EL, Marcano DC, et al. Nanoparticulate carbon black in cigarette smoke induces DNA cleavage and Th17-mediated emphysema. eLife (2015) 4:e09623. doi: 10.7554/eLife.09623
131. Keijzer C, Spiering R, Silva AL, van Eden W, Jiskoot W, Vervelde L, et al. PLGA nanoparticles enhance the expression of retinaldehyde dehydrogenase enzymes in dendritic cells and induce FoxP3+ T-cells in vitro. J Control Release (2013) 168:35–40. doi: 10.1016/j.jconrel.2013.02.027
132. Zhang Y, Bai Y, Jia J, Gao N, Li Y, Zhang R, et al. Perturbation of physiological systems by nanoparticles. Chem Soc Rev. (2014) 43:3762–809. doi: 10.1039/C3CS60338E
133. Broos S, Lundberg K, Akagi T, Kadowaki K, Akashi M, Greiff L, et al. Immunomodulatory nanoparticles as adjuvants and allergen-delivery system to human dendritic cells: implications for specific immunotherapy. Vaccine (2010) 28:5075–85. doi: 10.1016/j.vaccine.2010.05.004
134. Hamasaki T, Uto T, Akagi T, Akashi M, Baba M. Modulation of gene expression related to toll-like receptor signaling in dendritic cells by poly(gamma-glutamic acid) nanoparticles. Clin Vaccine Immunol. (2010) 17:748–56. doi: 10.1128/cvi.00505-09
135. Uto T, Akagi T, Hamasaki T, Akashi M, Baba M. Modulation of innate and adaptive immunity by biodegradable nanoparticles. Immunol Lett. (2009) 125:46–52. doi: 10.1016/j.imlet.2009.05.008
136. Zhu M, Li Y, Shi J, Feng W, Nie G, Zhao Y. Exosomes as extrapulmonary signaling conveyors for nanoparticle-induced systemic immune activation. Small (2012) 8:404–12. doi: 10.1002/smll.201101708
137. Mizuashi M, Ohtani T, Nakagawa S, Aiba S. Redox imbalance induced by contact sensitizers triggers the maturation of dendritic cells. J Invest Dermatol (2005) 124:579–86. doi: 10.1111/j.0022-202X.2005.23624.x
Keywords: nanoparticles, physicochemical properties, DC targeting, cancer immunotherapy, DC functions
Citation: Jia J, Zhang Y, Xin Y, Jiang C, Yan B and Zhai S (2018) Interactions Between Nanoparticles and Dendritic Cells: From the Perspective of Cancer Immunotherapy. Front. Oncol. 8:404. doi: 10.3389/fonc.2018.00404
Received: 28 June 2018; Accepted: 04 September 2018;
Published: 25 September 2018.
Edited by:
Giuseppe Giaccone, Georgetown University, United StatesReviewed by:
Lina Ghibelli, Università degli Studi di Roma Tor Vergata, ItalyChao Wang, University of North Carolina at Chapel Hill, United States
Copyright © 2018 Jia, Zhang, Xin, Jiang, Yan and Zhai. This is an open-access article distributed under the terms of the Creative Commons Attribution License (CC BY). The use, distribution or reproduction in other forums is permitted, provided the original author(s) and the copyright owner(s) are credited and that the original publication in this journal is cited, in accordance with accepted academic practice. No use, distribution or reproduction is permitted which does not comply with these terms.
*Correspondence: Bing Yan, ZHJiaW5neWFuQHlhaG9vLmNvbQ==
Shumei Zhai, c216aGFpQHNkdS5lZHUuY24=