- 1Department of Clinical and Molecular Medicine, Faculty of Medicine and Health Sciences, Norwegian University of Science and Technology, Trondheim, Norway
- 2Center for Computational Neuroscience, Egil and Pauline Braathen and Fred Kavli Center for Cortical Microcircuits, Kavli Institute for Systems Neuroscience, NTNU Norwegian University of Science and Technology, Trondheim, Norway
Layer II of the medial entorhinal cortex (MEC LII) contains the largest number of spatially modulated grid cells and is one of the first regions in the brain to express Alzheimer’s disease (AD)-related pathology. The most common principal cell type in MEC LII, reelin-expressing stellate cells, are grid cell candidates. Recently we found evidence that γ-aminobutyric acid (GABA)A receptor subunits show a specific distribution in MEC LII, in which GABAA α3 is selectively associated with reelin-positive neurons, with limited association with the other principal cell type, calbindin (CB)-positive pyramidal neurons. Furthermore, the expression of α3 subunit decreases in mice between P15 and P25, which coincides with the emergence of stable grid cell activity. It has been shown that the α3 subunit undergoes specific developmental changes and that it may exert pro-inflammatory actions if improperly regulated. In this review article, we evaluate the changing kinetics of α3-GABAA receptors (GABAARs). during development in relation to α3-subunit expression pattern in MEC LII and conclude that α3 could be closely related to the stabilization of grid cell activity and theta oscillations. We further conclude that dysregulated α3 may be a driving factor in early AD pathology.
Introduction
Most inhibitory signaling involves transmission of γ-aminobutyric acid (GABA) between neurons, and most of GABAergic signaling is mediated by ionotropic GABAA receptors (GABAARs). These receptors are heteropentameric, consisting of five subunit proteins that together form a central chloride permeable pore. The subunit composition of GABAARs shows great variation throughout the brain. A total of 19 candidate subunit proteins can form a receptor: α1–6, β1–3, γ1–3, δ, ε, θ, π and ρ1–3 (Farrant and Nusser, 2005). The composition of a receptor often involves 2α, 2β and 1γ or 1δ subunits (Tretter et al., 1997; Nakamura et al., 2015). Different receptor compositions have different distributions on the postsynaptic cell membrane, along with different pharmacological properties and efficiency in mediating GABAergic neurotransmission.
One brain region, which shows a striking inhibition dominated local network, is layer II of the medial entorhinal cortex (MEC LII). With strong reciprocal connections to the hippocampus, the MEC is a major hub for the generation of an internal representation of space (Hafting et al., 2005; Sargolini et al., 2006; Solstad et al., 2008; Kropff et al., 2015). Principal cells of MEC LII can be classified into stellate and pyramidal cells, which largely express reelin (RE) and calbindin (CB), respectively, although intermediate cell types have also been described (Fuchs et al., 2015; Witter et al., 2017). MEC LII principal cells play essential roles in brain function. First, it has recently been found that RE+ cell activity drives the maturation of the entire entorhinal-hippocampal circuit (Donato et al., 2017). Moreover, both RE+ and CB+ cells encompass spatially modulated grid cells, which have a hexagonally arranged activity pattern spanning the explored environment (Domnisoru et al., 2013; Schmidt-Hieber and Häusser, 2013; Tang et al., 2014; Sun et al., 2015; Rowland et al., 2018). Most MEC LII principal cells are connected through fast-spiking perisomatic GABAergic parvalbumin-expressing (PV+) interneurons (Couey et al., 2013; Fuchs et al., 2015), which have been shown to be crucial for the emergence of grid cell activity (Buetfering et al., 2014; Miao et al., 2017). However, until recently, cell type-specific expression of GABAAR subunits has not been reported.
Previously we discovered that RE+ cells rather prominently express the GABAAR subunit α3 (α3-GABAARs; Figure 1; Berggaard et al., 2018b). Studies have shown that α3 may play important roles in neuronal development (Ohlson et al., 2007; Daniel et al., 2011), as well as in regulating anxiety and stress (Dias et al., 2005). Furthermore, emerging evidence suggests dysregulated activity by α3-GABAARs causes the subunit to become pro-inflammatory and to play important roles in the onset and progression of pathologies such as cancers and colon inflammation (Gumireddy et al., 2016; Liu et al., 2016; Long et al., 2017; Seifi et al., 2018). It is therefore possible the α3 subunit is involved in various processes in MEC LII besides mediating inhibitory neurotransmission.
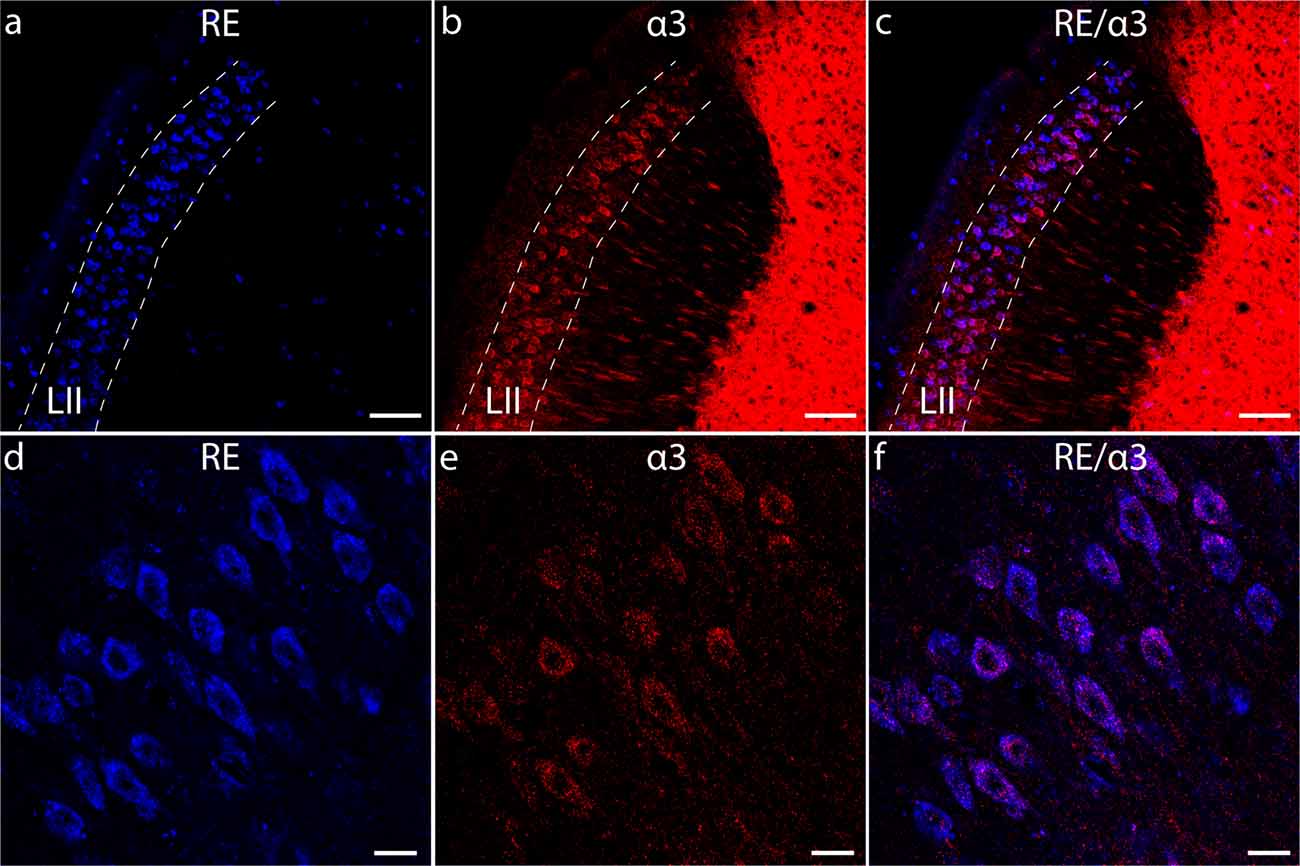
Figure 1. RE+ cells of layer II of the medial entorhinal cortex (MEC) LII express the GABAAR α3 subunit. (A–C) Overview of the dorsal portion of the adult mouse MEC from a sagittal section, stained for RE (A) and α3 (B). Panel (C) shows an overlay of (A,B). (D–F) High magnification images of RE (D) and α3 (E) immunoreactive cells in dorsal MEC LII. Panel (F) shows an overlay of (D,E). Note the strong association between RE and α3 immunoreactivity. Dashed lines delineate LII. Scale bars: (A–C), 100 μm; (D–F), 20 μm.
In this review article, we will highlight the known involvement of α3 in physiological and pathological processes. We will further discuss the possible implications of the strong presence of α3 in RE+ cells of MEC LII, focusing particularly on early postnatal development, kinetics of GABAergic inhibition, and the possible role of this subunit in Alzheimer’s disease (AD). We will hereafter refer to the subunit protein and RNA as α3 and Gabra3, respectively.
Kinetics of GABAAR Mediated Inhibition in MEC LII
In the mouse MEC LII, we found RE+ and CB+ principal cells to express distinct GABAAR subunits during a period implicated in grid cell maturation. Both cell types showed weak and strong expression of α1 and γ2, respectively, and no somato-dendritic expression of α2 and α4. The subunits α3 and α5 were instead largely specific to RE+ and CB+ cells, respectively, apart from a subset of CB+ cells expressing α3 (Berggaard et al., 2018b). Since the location and subunit composition of receptors on the postsynaptic cell membrane are important for determining the effect of inhibition, it is likely that GABAergic inhibition of the two cell types has different effects. GABAARs containing α1 and/or α3 in combination with γ2 subunits are predominantly located in the synapse where they mediate phasic inhibition in response to GABA release from the presynaptic terminal. In contrast, α5-GABAARs are expressed in extrasynaptic spaces where they mediate tonic inhibition (Brünig et al., 2002; Farrant and Nusser, 2005).
How fast GABAARs bind to, and dissociate from, GABA, is an important influencer of for instance oscillation frequency. Although α3-GABAARs predominantly mediate phasic inhibition, their activation and deactivation kinetics are in general slower than of α1-GABAARs. The activation kinetics of a receptor partly depends on its sensitivity to GABA. This can be found by measuring the concentration of GABA needed to elicit the half-maximal response (EC50; Farrant and Nusser, 2005). When comparing the EC50 values of receptors containing the αxβ3γ2 combination, it was found that α3β3γ2, and in one study also α2β3γ2, exhibit the highest EC50 values, and thereby lowest sensitivity to GABA compared to all other α subunits (Böhme et al., 2004; Mortensen et al., 2011). α3 subunits may, however, also exist in combination with ε and/or θ subunits, and it has been found that receptors with the α3β1ε combination are nearly 100-fold more sensitive to GABA compared to α3β1γ2 (Ranna et al., 2006). Furthermore, while open, the maximum GABA currents obtained with α3-GABAARs are larger than those obtained with α1- and α5-GABAARs (Mortensen et al., 2011; Table 1).
The deactivation kinetics are also much slower for α3-GABAARs compared to α1-GABAARs (Table 2), and are associated with slow desensitization rates of α3-GABAARs (Barberis et al., 2007; Mortensen et al., 2011; Eyre et al., 2012). This is in contrast to the extrasynaptic α5-GABAARs, which exhibit faster desensitization, but slower deactivation, such that the strength of GABAergic transmission decreases rapidly but lasts longer than in case of α3-GABAARs (Mortensen et al., 2011).
Our previous study focused on the presence of α3 and not that of ε and θ subunits in MEC LII, thus it is unknown whether α3 combines with these subunits in RE+ cells. However, the strong expression of γ2 in RE+ cells (Berggaard et al., 2018b) renders it likely that at least a subset of α3-GABAARs in RE+ cells contain γ2. Although the sensitivity of these receptors to GABA is low, the high concentration of GABA following presynaptic release would still allow for fast activation, albeit slightly slower than for α1-GABAARs. Once α3-GABAARs are activated, their responsiveness to GABA remains high for a prolonged time until the receptors deactivate rather sharply. One of the key features of RE+ cells, apart from low input resistance and sag potential, includes high-frequency burst firing at the beginning of a spike train (Canto and Witter, 2012; Couey et al., 2013). Based on these observations, one can predict that the strong and prolonged inhibitory currents, followed by rather sharp deactivation of α3-GABAARs on the postsynaptic membrane, are among the factors that enable RE+ cells to enter into bursting mode.
α3 Subunit in Development
Throughout development, there are alterations in subunit composition, distribution and kinetics of GABAARs. Laurie et al. (1992) applied in situ hybridization to demonstrate age-related changes in expression of 13 GABAAR subunits. They report changes in expression pattern of each individual GABAAR subunit mRNA during early development that coincide with the shift in GABA’s role from excitatory, neurotrophic factor to an inhibitory transmitter. During embryonic and early postnatal period of the rat, α3 and α2 subunits are the most widespread among the α subunits in the brain, followed by the α5 subunit. Moreover, α3 displays the highest mRNA levels among α subunits in the neocortex until postnatal day P6–12, after which it is largely replaced by the α1 subunit and restricted to the deeper cortical layers (Laurie et al., 1992; Wisden et al., 1992).
Simultaneous to a decrease in α3 protein expression during development, an increasing amount of Gabra3, its RNA counterpart, undergoes so-called adenosine-to-inosine (A-to-I) editing (Ohlson et al., 2007). A-to-I editing is catalyzed by Adenosine Deaminases that act on RNA (ADAR) enzymes and is a type of post-transcriptional processing of double-stranded RNA especially common in the human brain (Bass, 2002). Ever since A-to-I editing was discovered, tens of thousands of editing sites have been revealed. In addition, knowledge about the important roles of A-to-I editing in brain development and the involvement of improperly or unedited RNA in brain diseases has increased (Khermesh et al., 2016; Bajad et al., 2017). During editing of Gabra3, an isoleucine molecule is recoded into methionine by ADAR1 and ADAR2 of the ADAR family, at a highly evolutionary conserved genomic region (Ohlson et al., 2007; Daniel et al., 2011). The percentage of A-to-I edited Gabra3 displays a gradual increase from approximately 4%–7% at E15 to 53%–54% and 90%–93% at P2 and P7, respectively, which persists into adulthood (Rula et al., 2008; Wahlstedt et al., 2009; Ensterö et al., 2010). Slightly lower levels of edited Gabra3 at P7 (78%) with a subsequent increase to 92% at P21 were also observed (Daniel et al., 2011). In contrast, total levels of Gabra3 mRNA showed a sharp increase between mouse embryonic day E15 to E19, followed by a gradual decrease between postnatal day P7 and adulthood. High levels of edited Gabra3 were observed in all brain areas investigated, apart from the hippocampus, where only about 70% of Gabra3 was edited in the adult (Rula et al., 2008). In addition, the striatum has been found to have significantly lower levels of edited Gabra3 compared to the cortex (O’Neil et al., 2017).
A possible role of α3 is to maintain synapses. In a study on mice exhibiting targeted deletion of the α3 subunit, the GABAergic synapses in the reticular nucleus of thalamus were fewer and larger compared to wild type mice (Studer et al., 2006).
α3 Subunit and GABA-Shift
There are indications that A-to-I editing of Gabra3 could facilitate the transition of GABA from excitatory to inhibitory. In the immature brain, GABAergic synapses mature prior to glutamatergic synapses (Tyzio et al., 1999; Khazipov et al., 2001; Ben-Ari et al., 2007). However, due to high intracellular concentration of Cl−, GABAergic activation of GABAARs at this stage results in an excitatory effect through an efflux of Cl− ions. The shift results from an increase in the expression of potassium chloride channels (KCCs). During development there is an increase in expression of Cl− extruding K+/Cl− co-transporter KCC2, which causes reduced levels of intracellular Cl− such that GABAAR-mediated signaling becomes hyperpolarizing (Rivera et al., 1999). This “GABA switch” occurs at slightly different time points in different brain regions and is dependent on GABAergic activation of GABAARs, as perturbed activation of GABAARs directly affects the mRNA levels of KCC2 as well as the timing of the switch (Ganguly et al., 2001; Leitch et al., 2005). In the chick retina, increased levels of A-to-I edited Gabra3 were found to go hand in hand with an increased expression of KCC2, which could imply that A-to-I editing of Gabra3 is an important component for the GABA switch (Ring et al., 2010).
Furthermore, editing of Gabra3 changes the kinetics of α3-GABAAR mediated inhibition. Electrophysiological recordings in human embryonic kidney cells have revealed that non-edited α3, in combination with β3 and γ2, have an average EC50 value of nearly half of that of edited α3, implying that the sensitivity to GABA is much higher for isoleucine-containing subunits compared to the ones containing methionine. Furthermore, the decay rate was found to be slower for unedited α3 compared to edited α3, suggesting that GABAergic transmission through α3-GABAARs is more effective in immature brain compared to the adult. The editing position in Gabra3 is in the third transmembrane region, which is a region known for regulating trafficking of α subunits of GABAARs. Thus, the expression of α3-GABAARs is directly affected by the isoleucine to methionine change. Confirmation of this notion was obtained in a study which showed that edited α3 displayed a 60% reduction in cell surface expression and approximately 40% reduction in total protein levels compared to non-edited α3, irrespective of subunit composition (Nimmich et al., 2009; Daniel et al., 2011).
α3 Subunit: Possible Role in RE+ and Grid Cell Development?
In MEC LII, RE+ cells drive the development of the entorhinal-hippocampal circuit, including CB+ and PV+ cells in MEC LII. It was found that the maturation of RE+ cells is independent of any input from excitatory neurons, and instead depends on RE+ cell birth date, which suggests cell autonomous pathways being responsible for their maturation (Donato et al., 2017). The effect of input from GABAergic terminals on RE+ cells was, however, not investigated. This could be of interest considering these synapses are established prior to glutamatergic synapses. Since RE+ and CB+ cells largely express α3- and α5-GABAARs, respectively (Berggaard et al., 2018b), the cell type-specific instructive signal from GABAergic interneurons might promote development of RE+ cells following a dorsoventral gradient. Interestingly, in the early postnatal period of the rat, mRNA levels of most GABAAR subunits in the EC are higher than in the neighboring cortex (Laurie et al., 1992), which could imply increased GABAergic activity in this region compared to the surround. Moreover, ultrastructural investigations on the development of PV+ terminal input on rat MEC LII cell somata revealed that, at P10, close to all somata in the dorsal portion form synaptic contacts with PV+ terminals. Somata in the ventral portion, however, showed significantly less PV+ terminal apposition (Berggaard et al., 2018a).
In rodents, the emergence and stabilization of grid cell activity has been reported to happen after eye opening, from approximately P20, with the number of grid cells reaching adult levels around P22 (Wills et al., 2010, 2012; Tan et al., 2017). During this time, we noticed a significant decrease in both protein and mRNA levels of the α3 subunit, whereas the levels of other subunits measured were unchanged (Berggaard et al., 2018b). This decrease occurs in the same time frame with data on the change of Gabra3 levels in the brain during development, and may therefore serve a more widespread function, such as decreased α3/α1 ratio and consequently more rapid GABAergic transmission. However, since decreasing levels of Gabra3 have been directly associated with A-to-I editing of the subunit, the decrease seen in α3 levels between P15 and P25 may reflect α3-GABAARs in RE+ and a subset of CB+ cells undergoing A-to-I editing. This could be important, since at least a subset of grid cells likely contain α3-GABAARs. In case α3-GABAARs in immature grid cells undergo editing during the period that grid cell activity emerges, this would cause the kinetics of GABAergic inhibition in these cells to alter. A possible outcome of such an event is that the new kinetics of GABAergic transmission allow grid cell activity to stabilize.
α3 Subunit Is Regulated by Sex Hormones
While most of the GABAAR receptor subunit genes appear in small clusters on autosomes, the α3, ε and θ subunits are the only known subunit genes that are positioned on the X chromosome. More specifically, they are located in close approximation to each other at the Xq28 position in humans, which is a candidate region for X-linked disorders such as early onset parkinsonism (Bell et al., 1989; Garret et al., 1997; Korpi et al., 2002; Kolb-Kokocinski et al., 2006). They might regulate the turnover of noradrenaline, dopamine and 5-HT (McKernan and Whiting, 1996).
Emerging evidence suggests that ovarian hormones regulate Gabra3. In a study on gonadotropin-releasing hormone neurons, which regulate reproduction, there were among several genes increased levels of Gabra3 mRNA in proestrous compared to metestrous female mice. This was in contrast to Gabra1-2 and Gabra5 mRNA levels, which were unchanged between the two groups (Vastagh et al., 2016). In addition, mRNA levels of Gabra3 were higher in male mice compared to metestrous female mice (Vastagh et al., 2015). In the dorsal raphe nucleus of female rhesus monkeys, there was a significant increase in Gabra3 RNA upon treatment with a combination of progesterone and estradiol, but not after estradiol treatment alone. Since progesterone and estradiol treatment also gave a reduction in RNA of JNK-1 and kynurenine 3-hydroxylase, which are pro-apoptotic and generate neurotoxic quinolones, this could imply that an upregulation of Gabra3 is neuroprotective in the serotonergic population (Reddy and Bethea, 2005). In addition, MEC LII is closely regulated by treatment with ovarian hormones, as shown in a study on ovariectomized rats with unilateral lesion of the perforant path. This ultimately causes ipsilateral degeneration of the RE+ cell population of EC LII. Here it was found that treatment with a combination of estradiol + discontinuous application of progesterone significantly improved neuronal survival and neurite outgrowth compared to estradiol + continuous progesterone administration or estradiol alone (Barron et al., 2015). The mechanisms underlying this neuroprotection could therefore in part be due to treatment-induced increased levels of Gabra3 in RE+ cells.
α3-Subunit: Possible Role in Alzheimer’s Disease?
While the importance of A-to-I editing of Gabra3 is still being investigated, it becomes increasingly clear that a failure of proper A-to-I editing can cause various diseases (for review see Bajad et al., 2017). For example, studies on different types of cancers, including breast, pancreatic and lung, have implicated Gabra3 in the disease progression. Specifically, Gabra3 was found to promote cancer cell invasion and migration by activating various inflammatory pathways such as AKT/mTOR and JNK (Gumireddy et al., 2016; Liu et al., 2016; Long et al., 2017). In one of the studies, it was discovered that only the unedited form of Gabra3 had metastatic properties, while A-to-I edited Gabra3 was found to suppress cancer progression (Gumireddy et al., 2016). This could imply that dysregulated A-to-I editing of Gabra3 in the brain may also be pathological. With respect to MEC LII, there is a close link to AD, since it is one of the first regions in the brain to exhibit pathological changes associated with the neurodegenerative disease (Braak and Braak, 1991; Kobro-Flatmoen et al., 2016). AD has previously been associated with a reduction in A-to-I editing (Khermesh et al., 2016). In the frontal cortex of humans aged 22–102 years who had mostly died of heart failure, nearly all subjects had editing levels of Gabra3 of at least 90%, apart from two subjects who had died of skin cancer and hypoxia ischemia and whose editing levels were only 60% (Nicholas et al., 2010). Overall, these results suggest Gabra3 remains edited throughout life in the healthy brain, and that high levels of unedited Gabra3 are most likely pathological. It is therefore possible that the ratio of edited to unedited Gabra3 is lower in MEC LII of AD patients. In this regard, it is worth noting that many of the cell types which show increased vulnerability to AD pathology express α3, including strong expression on cholinergic, noradrenergic, dopaminergic and serotonergic systems (Gao et al., 1995; Rodríguez-Pallares et al., 2001; Corteen et al., 2015). Furthermore, early AD is associated with decreased power and frequency of gamma oscillations (Klein et al., 2016), which could be related to a change in activity of α3-GABAARs. The regulation of Gabra3 by ovarian hormones may thus be of relevance for AD, considering the typical AD patient is a postmenopausal woman.
Conclusion
The GABAAR α3 subunit is strongly expressed in MEC LII, predominantly in RE+ stellate cells. In this review, we have discussed some potential consequences that the distribution of α3-GABAARs may have in refining GABAergic activity and strength in specific neurons and synaptic circuits in navigation. The α3 subunit undergoes an isoleucine-to-methionine change during early postnatal development, which significantly alters the kinetics of α3-GABAAR mediated inhibition. This editing event may coincide with, and be important for, the development of MEC LII cells, such as grid cells. Theta oscillation activity of grid cells matures during early developmental stages and shows a similar temporal pattern for the α3 subunit. Furthermore, the fact that α3 is regulated by ovarian hormones, and that a failure to properly edit α3 is likely pathological, could be a factor in AD.
Data Availability
The datasets for this manuscript are not publicly available because the data are available upon request. Requests to access the datasets should be directed to am9oYW5uZXMud2FudEBudG51Lm5v.
Author Contributions
NB, MW and JW wrote the article.
Funding
This work was sponsored by Norwegian University of Science and Technology (Norges Teknisk-Naturvitenskapelige Universitet).
Conflict of Interest Statement
The authors declare that the research was conducted in the absence of any commercial or financial relationships that could be construed as a potential conflict of interest.
References
Bajad, P., Jantsch, M. F., Keegan, L., and O’Connell, M. (2017). A to I editing in disease is not fake news. RNA Biol. 14, 1223–1231. doi: 10.1080/15476286.2017.1306173
Barberis, A., Mozrzymas, J. W., Ortinski, P. I., and Vicini, S. (2007). Desensitization and binding properties determine distinct α1β2γ2 and α3β2γ2 GABAA receptor-channel kinetic behavior. Eur. J. Neurosci. 25, 2726–2740. doi: 10.1111/j.1460-9568.2007.05530.x
Barron, A. M., Brown, M. A., Morgan, T. E., and Pike, C. J. (2015). Impact of continuous versus discontinuous progesterone on estradiol regulation of neuron viability and sprouting after entorhinal cortex lesion in female rats. Endocrinology 156, 1091–1099. doi: 10.1210/en.2014-1216
Bass, B. L. (2002). RNA editing by adenosine deaminases that act on RNA. Annu. Rev. Biochem. 71, 817–846. doi: 10.1146/annurev.biochem.71.110601.135501
Bell, M. V., Bloomfield, J., McKinleyj, M., Patterson, M. N., Darlison, M. G., Barnard, E. A., et al. (1989). Physical linkage of a GABAA receptor subunit gene to the DXS374 locus in human Xq28. Am. J. Hum. Genet. 45, 883–888.
Ben-Ari, Y., Gaiarsa, J.-L., Tyzio, R., and Khazipov, R. (2007). GABA: a pioneer transmitter that excites immature neurons and generates primitive oscillations. Physiol. Rev. 87, 1215–1284. doi: 10.1152/physrev.00017.2006
Berggaard, N., Bjerke, I. E., B Paulsen, A. E., Hoang, L., T Skogaker, N. E., Witter, M. P., et al. (2018a). Development of parvalbumin-expressing basket terminals in layer II of the rat medial entorhinal cortex. eNeuro 5:ENEURO.0438-17.2018. doi: 10.1523/ENEURO.0438-17.2018
Berggaard, N., Seifi, M., van der Want, J. J. L., and Swinny, J. D. (2018b). Spatiotemporal distribution of GABAA receptor subunits within layer II of mouse medial entorhinal cortex: implications for grid cell excitability. Front. Neuroanat. 12:46. doi: 10.3389/fnana.2018.00046
Böhme, I., Rabe, H., and Lüddens, H. (2004). Four amino acids in the α subunits determine the γ-aminobutyric acid sensitivities of GABAA receptor subtypes. J. Biol. Chem. 279, 35193–35200. doi: 10.1074/jbc.M405653200
Braak, H., and Braak, E. (1991). Acta neuropathologica Alzheimer’s disease affects limbic nuclei of the thalamus. Acta Neuropathol. 81, 261–268. doi: 10.1007/bf00305867
Brünig, I., Scotti, E., Sidler, C., and Fritschy, J.-M. (2002). Intact sorting, targeting, and clustering of γ-aminobutyric acid A receptor subtypes in hippocampal neurons in vitro. J. Comp. Neurol. 443, 43–55. doi: 10.1002/cne.10102
Buetfering, C., Allen, K., and Monyer, H. (2014). Parvalbumin interneurons provide grid cell-driven recurrent inhibition in the medial entorhinal cortex. Nat. Neurosci. 17, 710–718. doi: 10.1038/nn.3696
Canto, C. B., and Witter, M. P. (2012). Cellular properties of principal neurons in the rat entorhinal cortex. II. The medial entorhinal cortex. Hippocampus 22, 1277–1299. doi: 10.1002/hipo.20993
Corteen, N. L., Carter, J. A., Rudolph, U., Belelli, D., Lambert, J. J., and Swinny, J. D. (2015). Localisation and stress-induced plasticity of GABAA receptor subunits within the cellular networks of the mouse dorsal raphe nucleus. Brain Struct. Funct. 220, 2739–2763. doi: 10.1007/s00429-014-0824-7
Couey, J. J., Witoelar, A., Zhang, S.-J., Zheng, K., Ye, J., Dunn, B., et al. (2013). Recurrent inhibitory circuitry as a mechanism for grid formation. Nat. Neurosci. 16, 318–324. doi: 10.1038/nn.3310
Daniel, C., Wahlstedt, H., Ohlson, J., Björk, P., and Ohman, M. (2011). Adenosine-to-inosine RNA editing affects trafficking of the γ-aminobutyric acid type A (GABAA) receptor. J. Biol. Chem. 286, 2031–2040. doi: 10.1074/jbc.M110.130096
Dias, R., Sheppard, W. F. A., Fradley, R. L., Garrett, E. M., Stanley, J. L., Tye, S. J., et al. (2005). Evidence for a significant role of α 3-containing GABAA receptors in mediating the anxiolytic effects of benzodiazepines. J. Neurosci. 25, 10682–10688. doi: 10.1523/JNEUROSCI.1166-05.2005
Domnisoru, C., Kinkhabwala, A. A., and Tank, D. W. (2013). Membrane potential dynamics of grid cells. Nature 495, 199–204. doi: 10.1038/nature11973
Donato, F., Jacobsen, R. I., Moser, M. B., and Moser, E. I. (2017). Stellate cells drive maturation of the entorhinal-hippocampal circuit. Science 355:eaai8178. doi: 10.1126/science.aai8178
Ensterö, M., Åkerborg, Ö., Lundin, D., Wang, B., Furey, T. S., Öhman, M., et al. (2010). A computational screen for site selective A-to-I editing detects novel sites in neuron specific Hu proteins. BMC Bioinformatics 11:6. doi: 10.1186/1471-2105-11-6
Eyre, M. D., Renzi, M., Farrant, M., and Nusser, Z. (2012). Setting the time course of inhibitory synaptic currents by mixing multiple GABAA receptor α subunit isoforms. J. Neurosci. 32, 5853–5867. doi: 10.1523/JNEUROSCI.6495-11.2012
Farrant, M., and Nusser, Z. (2005). Variations on an inhibitory theme: phasic and tonic activation of GABAA receptors. Nat. Rev. Neurosci. 6, 215–229. doi: 10.1038/nrn1625
Fuchs, E. C., Neitz, A., Pinna, R., Melzer, S., Caputi, A., and Monyer, H. (2015). Local and distant input controlling excitation in layer II of the medial entorhinal cortex. Neuron 89, 194–208. doi: 10.1016/j.neuron.2015.11.029
Ganguly, K., Schinder, A. F., Wong, S. T., and Poo, M. (2001). GABA itself promotes the developmental switch of neuronal GABAergic responses from excitation to inhibition. Cell 105, 521–532. doi: 10.1016/s0092-8674(01)00341-5
Gao, B., Hornung, J.-P., and Fritschy, J.-M. (1995). Identification of distinct GABAA-receptor subtypes in cholinergic and parvalbumin-positive neurons of the rat and marmoset medial septum—diagonal band complex. Neuroscience 65, 101–117. doi: 10.1016/0306-4522(94)00480-s
Garret, K. M., Haque, D., Berry, D., Niekrasz, I., Gan, J., Rotter, A., et al. (1997). The GABAA receptor α6 subunit gene (Gabra6) is tightly linked to the α1-γ2 subunit cluster on mouse chromosome 11. Mol. Brain Res. 45, 133–137. doi: 10.1016/s0169-328x(96)00290-2
Gumireddy, K., Li, A., Kossenkov, A. V., Sakurai, M., Yan, J., Li, Y., et al. (2016). The mRNA-edited form of GABRA3 suppresses GABRA3-mediated Akt activation and breast cancer metastasis. Nat. Commun. 7:10715. doi: 10.1038/ncomms10715
Hafting, T., Fyhn, M., Molden, S., Moser, M.-B., and Moser, E. I. (2005). Microstructure of a spatial map in the entorhinal cortex. Nature 436, 801–806. doi: 10.1038/nature03721
Khazipov, R., Esclapez, M., Caillard, O., Bernard, C., Khalilov, I., Tyzio, R., et al. (2001). Early development of neuronal activity in the primate hippocampus in utero. J. Neurosci. 21, 9770–9781. doi: 10.1523/JNEUROSCI.21-24-09770.2001
Khermesh, K., D’Erchia, A. M., Barak, M., Annese, A., Wachtel, C., Levanon, E. Y., et al. (2016). Reduced levels of protein recoding by A-to-I RNA editing in Alzheimer’s disease. RNA 22, 290–302. doi: 10.1261/rna.054627.115
Klein, A. S., Donoso, J. R., Kempter, R., Schmitz, D., and Beed, P. (2016). Early cortical changes in γ oscillations in Alzheimer’s disease. Front. Syst. Neurosci. 10:83. doi: 10.3389/fnsys.2016.00083
Kobro-Flatmoen, A., Nagelhus, A., and Witter, M. P. (2016). Reelin-immunoreactive neurons in entorhinal cortex layer II selectively express intracellular amyloid in early Alzheimer’s disease. Neurobiol. Dis. 93, 172–183. doi: 10.1016/j.nbd.2016.05.012
Kolb-Kokocinski, A., Mehrle, A., Bechtel, S., Simpson, J., Kioschis, P., Wiemann, S., et al. (2006). The systematic functional characterisation of Xq28 genes prioritises candidate disease genes. BMC Genomics 7:29. doi: 10.1186/1471-2164-7-29
Korpi, E. R., Gründer, G., and Lüddens, H. (2002). Drug interactions at GABAA receptors. Prog. Neurobiol. 67, 113–159. doi: 10.1016/s0301-0082(02)00013-8
Kropff, E., Carmichael, J. E., Moser, M.-B., and Moser, E. I. (2015). Speed cells in the medial entorhinal cortex. Nature 523, 419–424. doi: 10.1038/nature14622
Laurie, D. J., Wisden, W., and Seeburg, P. H. (1992). The distribution of thirteen GABAA receptor subunit mRNAs in the rat brain. III. Embryonic and postnatal development. J. Neurosci. 12, 4151–4172. doi: 10.1523/JNEUROSCI.12-11-04151.1992
Leitch, E., Coaker, J., Young, C., Mehta, V., and Sernagor, E. (2005). GABA type-A activity controls its own developmental polarity switch in the maturing retina. J. Neurosci. 25, 4801–4805. doi: 10.1523/JNEUROSCI.0172-05.2005
Liu, L., Yang, C., Shen, J., Huang, L., Lin, W., Tang, H., et al. (2016). GABRA3 promotes lymphatic metastasis in lung adenocarcinoma by mediating upregulation of matrix metalloproteinases. Oncotarget 7, 32341–32350. doi: 10.18632/oncotarget.8700
Long, M., Zhan, M., Xu, S., Yang, R., Chen, W., Zhang, S., et al. (2017). miR-92b-3p acts as a tumor suppressor by targeting Gabra3 in pancreatic cancer. Mol. Cancer 16:167. doi: 10.1186/s12943-017-0723-7
McKernan, R. M., and Whiting, P. J. (1996). Which GABA,-receptor subtypes really occur in the brain? Trends Nurosci. 19, 139–143. doi: 10.1016/S0166-2236(96)80023-3
Miao, C., Cao, Q., Moser, M.-B., and Moser, E. I. (2017). Parvalbumin and somatostatin interneurons control different space-coding networks in the medial entorhinal cortex. Cell 171, 507.e17–521.e17. doi: 10.1016/j.cell.2017.08.050
Mortensen, M., Patel, B., and Smart, T. G. (2011). GABA potency at GABAA receptors found in synaptic and extrasynaptic zones. Front. Cell. Neurosci. 6:1. doi: 10.3389/fncel.2012.00001
Nakamura, Y., Darnieder, L. M., Deeb, T. Z., and Moss, S. J. (2015). Regulation of GABAARs by phosphorylation. Adv. Pharmacol. 72, 97–146. doi: 10.1016/bs.apha.2014.11.008
Nicholas, A., de Magalhaes, J. P., Kraytsberg, Y., Richfield, E. K., Levanon, E. Y., and Khrapko, K. (2010). Age-related gene-specific changes of A-to-I mRNA editing in the human brain. Mech. Ageing Dev. 131, 445–447. doi: 10.1016/j.mad.2010.06.001
Nimmich, M. L., Heidelberg, L. S., and Fisher, J. L. (2009). RNA editing of the GABAA receptor α3 subunit alters the functional properties of recombinant receptors. Neurosci. Res. 63, 288–293. doi: 10.1016/j.neures.2009.01.003
O’Neil, R. T., Wang, X., Morabito, M. V., and Emeson, R. B. (2017). Comparative analysis of A-to-I editing in human and non-human primate brains reveals conserved patterns and context-dependent regulation of RNA editing. Mol. Brain Res. 10:11. doi: 10.1186/s13041-017-0291-1
Ohlson, J., Pedersen, J. S., Haussler, D., and Ohman, M. (2007). Editing modifies the GABAA receptor subunit α3. RNA 13, 698–703. doi: 10.1261/rna.349107
Ranna, M., Sinkkonen, S., Möykkynen, T., Uusi-Oukari, M., and Korpi, E. (2006). Impact of ε and θ subunits on pharmacological properties of α3β1 GABA A receptors expressed in Xenopus oocytes. BMC Pharmacol. 6:1. doi: 10.1186/1471-2210-6-1
Reddy, A. P., and Bethea, C. L. (2005). Preliminary array analysis reveals novel genes regulated by ovarian steroids in the monkey raphe region. Psychopharmacology 180, 125–140. doi: 10.1007/s00213-005-2154-1
Ring, H., Boije, H., Daniel, C., Ohlson, J., Öhman, M., and Hallböök, F. (2010). Increased A-to-I RNA editing of the transcript for GABAA receptor subunit α3 during chick retinal development. Vis. Neurosci. 27, 149–157. doi: 10.1017/S0952523810000180
Rivera, C., Voipio, J., Payne, J. A., Ruusuvuori, E., Lahtinen, H., Lamsa, K., et al. (1999). The K+/Cl− co-transporter KCC2 renders GABA hyperpolarizing during neuronal maturation. Nature 397, 251–255. doi: 10.1038/16697
Rodríguez-Pallares, J., Caruncho, H. J., López-Real, A., Wójcik, S., Guerra, M. J., and Labandeira-García, J. L. (2001). Rat brain cholinergic, dopaminergic, noradrenergic and serotonergic neurons express GABAA receptors derived from the α3 subunit. Receptors Channels 7, 471–478. doi: 10.1016/s0166-4328(01)00208-x
Rowland, D. C., Obenhaus, H. A., Skytøen, E. R., Zhang, Q., Kentros, C. G., Moser, E. I., et al. (2018). Functional properties of stellate cells in medial entorhinal cortex layer II. Elife 7:e36664. doi: 10.7554/eLife.36664
Rula, E. Y., Lagrange, A. H., Jacobs, M. M., Hu, N., Macdonald, R. L., and Emeson, R. B. (2008). Developmental modulation of GABAA receptor function by RNA editing. J. Neurosci. 28, 6196–6201. doi: 10.1523/JNEUROSCI.0443-08.2008
Sargolini, F., Fyhn, M., Hafting, T., McNaughton, B. L., Witter, M. P., Moser, M.-B., et al. (2006). Conjunctive representation of position, direction, and velocity in entorhinal cortex. Science 312, 758–762. doi: 10.1126/science.1125572
Schmidt-Hieber, C., and Häusser, M. (2013). Cellular mechanisms of spatial navigation in the medial entorhinal cortex. Nat. Neurosci. 16, 325–331. doi: 10.1038/nn.3340
Seifi, M., Rodaway, S., Rudolph, U., and Swinny, J. D. (2018). GABAA receptor subtypes regulate stress-induced colon inflammation in mice. Gastroenterology 155, 852.e3–864.e3. doi: 10.1053/j.gastro.2018.05.033
Solstad, T., Boccara, C. N., Kropff, E., Moser, M.-B., and Moser, E. I. (2008). Representation of geometric borders in the entorhinal cortex. Science 322, 1865–1868. doi: 10.1126/science.1166466
Studer, R., von Boehmer, L., Haenggi, T., Schweizer, C., Benke, D., Rudolph, U., et al. (2006). Alteration of GABAergic synapses and gephyrin clusters in the thalamic reticular nucleus of GABA A receptor α3 subunit-null mice. Eur. J. Neurosci. 24, 1307–1315. doi: 10.1111/j.1460-9568.2006.05006.x
Sun, C., Kitamura, T., Yamamoto, J., Martin, J., Pignatelli, M., Kitch, L. J., et al. (2015). Distinct speed dependence of entorhinal island ocean cells, including respective grid cells. Proc. Natl. Acad. Sci. U S A 112, 9466–9471. doi: 10.1073/pnas.1511668112
Tan, H. M., Wills, T. J., and Cacucci, F. (2017). The development of spatial and memory circuits in the rat. Wiley Interdiscip. Rev. Cogn. Sci. 8:e1424. doi: 10.1002/wcs.1424
Tang, Q., Burgalossi, A., Ebbesen, C. L., Ray, S., Naumann, R., Schmidt, H., et al. (2014). Pyramidal and stellate cell specificity of grid and border representations in layer 2 of medial entorhinal cortex. Neuron 84, 1191–1197. doi: 10.1016/j.neuron.2014.11.009
Tretter, V., Ehya, N., Fuchs, K., and Sieghart, W. (1997). Stoichiometry and assembly of a recombinant GABAA receptor subtype. J. Neurosci. 17, 2728–2737. doi: 10.1523/JNEUROSCI.17-08-02728.1997
Tyzio, R., Represa, A., Jorquera, I., Ben-Ari, Y., Gozlan, H., and Aniksztejn, L. (1999). The establishment of GABAergic and glutamatergic synapses on CA1 pyramidal neurons is sequential and correlates with the development of the apical dendrite. J. Neurosci. 19, 10372–10382. doi: 10.1523/JNEUROSCI.19-23-10372.1999
Vastagh, C., Rodolosse, A., Solymosi, N., Farkas, I., Auer, H., Sárvári, M., et al. (2015). Differential gene expression in gonadotropin-releasing hormone neurons of male and metestrous female mice. Neuroendocrinology 102, 44–59. doi: 10.1159/000430818
Vastagh, C., Rodolosse, A., Solymosi, N., and Liposits, Z. (2016). Altered expression of genes encoding neurotransmitter receptors in GnRH neurons of proestrous mice. Front. Cell. Neurosci. 10:230. doi: 10.3389/fncel.2016.00230
Wahlstedt, H., Daniel, C., Ensterö, M., and Ohman, M. (2009). Large-scale mRNA sequencing determines global regulation of RNA editing during brain development. Genome Res. 19, 978–986. doi: 10.1101/gr.089409.108
Wills, T. J., Barry, C., and Cacucci, F. (2012). The abrupt development of adult-like grid cell firing in the medial entorhinal cortex. Front. Neural Circuits 6:21. doi: 10.3389/fncir.2012.00021
Wills, T. J., Cacucci, F., Burgess, N., and O’Keefe, J. (2010). Development of the hippocampal cognitive map in preweanling rats. Science 328, 1573–1576. doi: 10.1126/science.1188224
Wisden, W., Laurie, D. J., Monyer, H., and Seeburg, P. H. (1992). The distribution of 13 GABAA receptor subunit mRNAs in the rat brain. I. Telencephalon, diencephalon, mesencephalon. J. Neurosci. 12, 1040–1062. doi: 10.1523/JNEUROSCI.12-03-01040.1992
Keywords: GABAA receptor subunit α3, medial entorhinal cortex, development, A-to-I editing, grid cells, Alzheimer’s disease
Citation: Berggaard N, Witter MP and van der Want JJL (2019) GABAA Receptor Subunit α3 in Network Dynamics in the Medial Entorhinal Cortex. Front. Syst. Neurosci. 13:10. doi: 10.3389/fnsys.2019.00010
Received: 27 November 2018; Accepted: 25 February 2019;
Published: 15 March 2019.
Edited by:
James W. Grau, Texas A&M University, United StatesReviewed by:
Ehren Lee Newman, Indiana University Bloomington, United StatesHenry J. Waldvogel, The University of Auckland, New Zealand
Copyright © 2019 Berggaard, Witter and van der Want. This is an open-access article distributed under the terms of the Creative Commons Attribution License (CC BY). The use, distribution or reproduction in other forums is permitted, provided the original author(s) and the copyright owner(s) are credited and that the original publication in this journal is cited, in accordance with accepted academic practice. No use, distribution or reproduction is permitted which does not comply with these terms.
*Correspondence: Johannes J. L. van der Want, am9oYW5uZXMud2FudEBudG51Lm5v