- 1Ottawa Hospital Research Institute (Neuroscience Program), Ottawa, ON, Canada
- 2University of Ottawa Brain and Mind Institute, Ottawa, ON, Canada
- 3Departments of Medicine, Cellular and Molecular Medicine, and Psychiatry, University of Ottawa, Ottawa, ON, Canada
Dopamine, a major neurotransmitter, plays a role in a wide range of brain sensorimotor functions. Parkinson's disease and schizophrenia are two major human neuropsychiatric disorders typically associated with dysfunctional dopamine activity levels, which can be alleviated through the druggability of the dopaminergic systems. Meanwhile, several studies suggest that optimal brain dopamine activity levels are also significantly impacted in other serious neurological conditions, notably stroke, but this has yet to be fully appreciated at both basic and clinical research levels. This is of utmost importance as there is a need for better treatments to improve recovery from stroke. Here, we discuss the state of knowledge regarding the modulation of dopaminergic systems following stroke, and the use of dopamine boosting therapies in animal stroke models to improve stroke recovery. Indeed, studies in animals and humans show stroke leads to changes in dopamine functioning. Moreover, evidence from animal stroke models suggests stimulation of dopamine receptors may be a promising therapeutic approach for enhancing motor recovery from stroke. With respect to the latter, we discuss the evidence for several possible receptor-linked mechanisms by which improved motor recovery may be mediated. One avenue of particular promise is the subtype-selective stimulation of dopamine receptors in conjunction with physical therapy. However, results from clinical trials so far have been more mixed due to a number of potential reasons including, targeting of the wrong patient populations and use of drugs which modulate a wide array of receptors. Notwithstanding these issues, it is hoped that future research endeavors will assist in the development of more refined dopaminergic therapeutic approaches to enhance stroke recovery.
Introduction
Stroke refers to an interruption of blood supply to some regions of the brain. This can be either ischemic (about 85% of strokes) or hemorrhagic (about 15% of strokes) (Auriat and Colbourne, 2008). Stroke is a major cause of disability in adults. In 2008 stroke was the cause of 5.7 million deaths and 46.6 disability-adjusted life years worldwide (Oczkowski, 2013). Stroke survivors are left with damage to their brain resulting in various impaired domains of function. Among the most common of these is motor deficits, affecting up to 80% of patients (Langhorne et al., 2009). Currently available interventions for stroke include clot-busting approaches such as thrombolytic drugs or angioplasty. These interventions are only available in select cases and only in the hyperacute stage of stroke. Once the patient has stabilized, a combination of spontaneous recovery and rehabilitation allow them to regain some function. While rehabilitation methods such as physical therapy have been shown to be more useful than no rehabilitation at all, no one physiotherapy treatment has been shown to be more efficacious than the others (Oczkowski, 2013). Additionally, physical rehabilitation is only available to individuals who are well enough to complete it. Unfortunately, with currently available treatments, 40–60% of stroke patients still present with motor deficits in the chronic stage, after recovery has plateaued (Acler and Manganotti, 2013). There is a need for more strategies to enhance stroke recovery. One approach, pharmacotherapy, uses drugs and physical rehabilitation to boost recovery. Several classes of drugs have been investigated for this purpose, including selective serotonin reuptake inhibitors (SSRIs), cholinergic agents and dopamine (DA)-enhancing drugs (Rösser and Flöel, 2008; Viale et al., 2018). However, none of these therapies have been approved for routine clinical use in stroke recovery. Herein, we argue that interventions targeting the dopaminergic system hold particular promise. Indeed, stroke impacts the dopaminergic system, and this combined with the normal decline in DA function and motor learning abilities with age, makes it a relevant target. There is robust evidence in animal stroke models showing that DA-enhancing drugs can improve motor recovery, through a myriad of potential mechanisms that remain to be fully understood. Lastly, clinical investigations suggest that this may be a valid therapeutic tool for stroke survivors, although better selection of patient groups may be required. This review will focus on interactions between the dopaminergic system and the poststroke brain, specifically as it pertains to motor recovery. To this end, we have excluded evidence relating to therapeutic targeting of dopaminergic system in the facilitation of recovery from traumatic brain injury.
Overview of the Dopaminergic System
The dopaminergic system mostly consists of groups of midbrain DA-synthesizing neurons that enable a wide range of modulatory and neuroendocrine functions in the brain specifically mediated through four major pathways (Björklund and Dunnett, 2007; Brunelin et al., 2013; Grattan, 2015). The nigrostriatal pathway arises from DA neurons originating in the substantia nigra pars compacta (SNc) that primarily project their axons to the striatum to control motor activity. The mesolimbic and mesocortical pathways (a.k.a. the mesocorticolimbic pathway) stem from DA neurons located in the ventral tegmental area (VTA) that send axonal projections to the limbic (e.g., nucleus accumbens, amygdala, hippocampus) and cortical areas (e.g., prefrontal cortex, motor cortex, and somatosensory cortex), respectively. These two dopaminergic pathways play a critical role in reward, memory, learning, cognition as well as planning, control and execution of voluntary movements. The fourth pathway, typically referred to as tuberoinfundibular pathway, arises from DA neurons in the hypothalamic periventricular and arcuate nuclei that display anatomically distinct projections to the medium eminence of the hypothalamus, a region from which DA is released in the hypophyseal portal system to inhibit prolactin secretion from the anterior pituitary gland (Björklund and Dunnett, 2007; Brunelin et al., 2013; Grattan, 2015).
Once released DA interacts with six transmembrane G protein-coupled receptors (GPCRs) separated classically into the D1-class (D1R, D5R) and D2-class (short and long isoforms of D2R, D3R and D4R) subtypes, activating and inhibiting adenylyl cyclase, respectively. Aside from their canonical Gs/olf and Gi/o-linked primary signaling pathways, studies have suggested a number of alternate G protein-dependent and independent signaling routes and downstream effects for these receptors (Beaulieu and Gainetdinov, 2011). There are many drugs which can modulate the activation of these receptors in a nonspecific and class-specific fashion but none exist that can bind to only one subtype. Studies in both animal and human stroke have mostly been done using indirect dopamine agonists, mainly amphetamine (AMPH), methylphenidate (MPH), and the DA precursor levodopa (L-DOPA). AMPH increases the release, and prevents the reuptake of DA, norepinephrine (NE) and to a lesser extent serotonin (Barbay and Nudo, 2009). MPH works by blocking the reuptake and thus increasing extrasynaptic DA and NE levels, although not as dramatically as AMPH (Kuczenski and Segal, 1997; Challman and Lipsky, 2000). Unlike DA, L-DOPA is able to cross the blood brain barrier. It can be given systemically and, once it enters the brain it is converted to DA (Barbeau et al., 1972). The DA molecule is in the synthesis pathway for NE, so L-DOPA can increase NE levels, however it has been reported that only 5% of L-DOPA ends up as NE, although this finding remains to be further validated (Nutt and Fellman, 1984). Notwithstanding this, it is worth mentioning that there is evidence supporting the direct contribution of noradrenergic neurons to dopaminergic neurotransmission through either NE acting as a DA receptor agonist or through co-release with DA (Vanderheyden et al., 1986; Devoto and Flore, 2006; Kubrusly et al., 2007; Smith and Greene, 2012; Root et al., 2015; Kempadoo et al., 2016; Takeuchi et al., 2016). Hence, indirect dopaminergic agonists such as AMPH related drugs may likely also mediate activation of DA receptors through their effects on noradrenergic axonal terminals.
Effect of Stroke on Functioning of the Dopaminergic System
Stroke impacts the DA system in a variety of ways, the implications of which are still somewhat unclear. For obvious reasons this is a difficult topic to study in humans, especially in the acute phase. In animal studies, differences in species and model can shift the timeline and magnitude of results, and this can make it difficult to translate these findings to humans. Add to this the importance of considering not just levels of DA and its metabolites [3,4-dihydroxyphenylacetic acid (DOPAC) and homovanilic acid (HVA)], but also levels of enzymes regulating DA synthesis (tyrosine hydroxylase or TH, and DOPA-decarboxylase) and degradation [monoamine oxidases MAO-A and MAO-B, and catechol-O-methyltransferase (COMT)], DA reuptake (dopamine transporter or DAT) and receptor proteins. Further complicating matters is that in the wake of stroke, any of these factors could be up or downregulated as part of the damage or as part of intrinsic attempts at recovery, making it difficult to determine how best to respond to these natural changes. Nonetheless, the background of the DA system remains an important consideration for those looking to modulate it to promote motor recovery from stroke.
Stroke Triggers Early, Massive Dopamine Release Into the Striatum
A massive DA release into the ipsilateral striatum almost immediately following the onset of ischemic stroke in the middle cerebral artery (MCA) territory has been repeatedly observed in many experimental models (Kogure et al., 1975; Ahagon et al., 1980; Brannan et al., 1987; Globus et al., 1988; Kawano et al., 1988; Slivka et al., 1988; Akiyama et al., 1991; Delbarre et al., 1992; Richards et al., 1993; Hashimoto et al., 1994; Toner and Stamford, 1996). Although this finding has been remarkable consistent, dissenting evidence does exist (Cvejić et al., 1980). The return to DA baseline levels is likely dependent upon the model used and the time that the ischemic period lasts, seeming to range from 30 min after reperfusion in bilateral MCA occlusion (MCAO) models (Akiyama et al., 1991), to several hours after microsphere injection (Kogure et al., 1975), and to take longer in permanent ligation or occlusion methods than in transient methods. All the studies discussed here used stroke models which significantly impact the striatum, so it remains unclear if this is a general reaction to ischemia or only to ischemia affecting the striatum.
Evidence shows that this DA release is not caused by action potentials (Akiyama et al., 1991). It cannot be attenuated with antagonists for D1R, D2R or both (Hashimoto et al., 1994). Prior lesioning of the substantia nigra (SN) on the side of the stroke prevents this massive DA release (Globus et al., 1987, 1988; Hashimoto et al., 1994). There is evidence which links the DA response to ischemia to local release of nitric oxide (Weinberger, 2002). Levels of DA metabolites DOPAC and HVA decrease during ischemia but rise after, which likely indicate efforts to return to baseline DA levels (Globus et al., 1988; Kawano et al., 1988; Slivka et al., 1988). DAT is overwhelmed during this release and drugs targeting it are unable to modulate the level of DA efflux, however it does seem to be active in the return to baseline (Akiyama et al., 1991; Toner and Stamford, 1996). Evidence suggests that the magnitude of the DA efflux correlates with the severity of the ischemia (Richards et al., 1993).
There appears to be differences in the degree of this response between animals of different ages, with aged animals having a more dramatic DA release after infarct and a more severe outcome from stroke (Delbarre et al., 1992). It has been suggested that the massive DA release contributes to neurodegeneration over the course of ischemia, as prior lesions to the SNc were observed to be neuroprotective in the striatum in several studies (Globus et al., 1987, 1988; Buisson et al., 1992; Weinberger, 2002). Additionally, use of D2-class antagonists, though not D1-class antagonists, have been reported to have a protective effect on neurons in the striatum (Hashimoto et al., 1994; Okada et al., 2005; Yulug et al., 2006a,b). In light of the well-established role of excessive glutamate release and sustained activation of extrasynaptic glutamate N-methyl-D-aspartate receptors (NMDAR) in mediating excitotoxicity and cell death during ischemic stroke, it remains unclear how the reported complex functional interactions between NMDARs and DA receptors contribute to either mitigating or exacerbating their deleterious effects (Wang et al., 2012; Brassai et al., 2015; Amantea and Bagetta, 2017).
The massive DA release is followed by a period of reduced DA levels in the hemisphere ipsilateral to the stroke, appearing as early as 2 h after stroke, or as late as 24 h (Zervas et al., 1974; Harrison et al., 1979; Ahagon et al., 1980). Some studies have suggested a loss or inefficiency in DA nerve terminals, with animals with more severe strokes having 30%, and moderately affected animals having 66%, of the number of DA nerve terminals of the contralateral hemisphere (Weinberger et al., 1983). In a rat photothrombosis model employing less intensive but longer duration irradiation to simulate a penumbra zone, levels of MAO-B were increased ~3-fold in the penumbra as compared to contralateral tissue at 4 h poststroke and ~2-fold at 24 h poststroke (Uzdensky et al., 2017). DOPA decarboxylase and TH were down regulated. Additionally, DAT levels were lower than the contralateral side (Uzdensky et al., 2017). These regulatory changes in the penumbra suggest lower DA levels are available after the immediate stroke period. An attenuated DA release response to high K+ stimulation, which was at about 40% of the response of non-ischemic controls, and was not modulated by nomifensine (a DAT inhibitor) treatment, has also been observed. This attenuation lasted at least 48 h after stroke, but was corrected by 98 h poststroke (Akiyama et al., 1991). In the contralateral hemisphere the concentration of DA, but not DA metabolites, has been found to be higher than sham operated animals at 1 week poststroke, but not at 2 weeks after stroke in a mouse photothrombosis model (Obi et al., 2018). It is clear that the massive DA release during the ischemia period leaves the DA system altered, which must be taken into account when considering the use of DA-enhancing therapies following stroke.
There is no data in humans regarding DA levels immediately following stroke, however two studies have used non-human primates. One study injected a shower of microemboli followed by one large embolus into the MCA of baboons. Animals were sacrificed 1 h after injection. DA was found to be increased in the cortical gray matter, significantly so in the frontal and occipital regions, which would be largely spared in this model. DA was found to be decreased in the caudate nucleus (Ishihara et al., 1979). Additionally, in squirrel monkeys subjected to MCA ligation and sacrificed after 3 h, there was a decrease in hemispheric DA on the side ipsilateral to the stroke as compared to the contralateral side (Zervas et al., 1974). It is possible that these decreases came after an initial increase in DA much sooner after stroke, like what is seen in rodent models. In the absence of earlier data, it is difficult to draw a conclusion as to whether primate stroke follows the same pattern as rodents, though it does seem certain that the DA system is modulated in the wake of stroke.
Evidence Indicates a Decrease in DA Receptors Following Stroke
Less information is available regarding the effect of stroke on levels of DA receptors.
Several studies suggest reduced D2R expression in the hemisphere ipsilateral to the stroke around 2–14 days after stroke (Dawson et al., 1994; Martín et al., 2013; Sieber et al., 2014). Less clear are the levels of D1R expression. In two studies employing the MCAO model, D1R expression decreased in the ipsilateral hemisphere, with evidence indicating this was due to down regulation and not simply loss of D1R expressing cells (Abe et al., 2004; Sieber et al., 2014). Meanwhile, a study using a rat photothrombotic model found a decrease in D1R levels in the infarct core but not in the penumbra (Rogozinska and Skangiel-Kramska, 2010). A transcriptional analysis in a transient MCAO model in mice found two subsets of differentially expressed genes responding to stroke in age-independent and dependent manner (Sieber et al., 2014). Furthermore, genes that were regulated differently following stroke in an age-dependent manner showed greater down- (or up-) regulation of gene response to stroke, including genes related to dopaminergic function (Drd1 and Drd2), in younger animals (Sieber et al., 2014).
Striatal Ischemia Leads to Development of Secondary Exofocal Degeneration in the Substantia Nigra Pars Compacta (SNc) and Pars Reticulata (SNr)
Another well documented phenomenon is the delayed degeneration of the ipsilateral SNc following striatal stroke. Studies suggest a progression of events from the time of stroke to the delayed degeneration. Unfortunately, not all animal studies with the transient or permanent MCAO model have reported the percent loss of neurons in SNc and SNr after striatal ischemia. Nonetheless, the few studies reporting this metric have shown a nigral cell loss ranging from 25 to 50% (Zhao et al., 2001; Rodriguez-Grande et al., 2013; Prinz et al., 2015). Intriguingly, the delayed ipsilateral degeneration in rodent models following striatal ischemia systematically happens and is more extensive in SNr relative to SNc; an observation that seems different from antedate postmortem human studies clearly showing delayed nigral cell loss in SNc following a massive stroke in basal ganglia (Forno, 1983; Ohara et al., 1989; Tamura et al., 1990; Yamada et al., 1996). However, these postmortem studies are unclear about whether a cell loss is also a hallmark of human SNr (Forno, 1983; Ohara et al., 1989). Interestingly, a loss of GABAergic inputs to the SNr has been proposed to underlie the exofocal degeneration (Yamada et al., 1996; Zhao et al., 2001). The loss of inhibitory inputs results in “burn out” of the cells in the SN leading to neuronal swelling and the start of apoptosis processes, which are visible in some cells as early as 24 h poststroke (Zhao et al., 2002; Rodriguez-Grande et al., 2013). This development leads to the gathering of microglia and astrocytes in the region (Rodriguez-Grande et al., 2013; Prinz et al., 2015). The cellular edema, due to the increase in inflammatory immune processes and cellular swelling is visible on T2 weighted magnetic resonance imaging (MRI) as early as 4 days poststroke in animal models (Zhao et al., 2001, 2002; Kronenberg et al., 2012; Prinz et al., 2015).
Sometime after the developing degeneration is visible using MRI, a decrease in TH positive cells is observed in the SNc. Some studies have found this decrease to be transient and independent of cell loss (Yamada et al., 1996; Soriano et al., 1997), whereas others have seen it last for the duration of the experiment, and have linked it to loss of SNc neurons (Zhao et al., 2001, 2002; Winter et al., 2005; Kronenberg et al., 2012; Rodriguez-Grande et al., 2013; Prinz et al., 2015). Two studies have linked the exofocal degeneration to a decrease in striatal DA and to changes in behavior (Winter et al., 2005; Kronenberg et al., 2012). Winter et al. (2005) found right side ischemia led to a decrease in DA and HVA in both the left and the right striatum, as well as increased activity in behavioral testing. Left-side infarcted animals showed a reduction in striatal DA on the left side only, and showed increased anxiety-like behaviors. In this study the degeneration occurred at 10 weeks poststroke, much later than what most other animal studies have shown (Winter et al., 2005). Furthermore, Kronenberg et al. (2012) found that left, but not right MCAO led to depression-like behavior at 12 weeks poststroke, unless they were treated with SSRIs starting from 7 days poststroke, when the exofocal degeneration was apparent on MRIs. This study also demonstrated degeneration of VTA (Kronenberg et al., 2012). The fact that both studies indicate differences in the deficit resulting from delayed SNc degeneration depending on the lateralization of the infarct is of note, and fits with the suggestion that left side strokes lead to a higher incidence of poststroke depression in humans (Stanfill et al., 2016). The neuroprotective effect of SSRIs in the study Kronenberg et al. (2012) gives hope to the possibility of preventing this secondary event when it is detected on MRI, although how SSRIs might interact with the proposed loss GABAergic inhibition theory is unclear.
Delayed SNc lesions following striatal infarcts have also been observed in human case studies (Kinoshita et al., 2002), examinations of clinical populations (Nakane et al., 1992; Ogawa et al., 1997) and in postmortem human tissue analysis (Forno, 1983; Ohara et al., 1989; Ogawa et al., 1997; Zhang et al., 2012). In two separate studies of clinical populations looking at 18 and 25 stroke patients, all patients who had striatal damage had the appearance of T2 weighted hyperintensity in the ipsilateral SNc around 1–2 weeks after stroke. Theses hyperintensities were not present earlier after stroke, and became less intense over the ensuing months. No such hyperintensities were seen in patients with purely cortical stroke (Nakane et al., 1992; Ogawa et al., 1997). One of the patients with SNc hyperintensity died of pneumonia during the study period. Postmortem analysis of this individual revealed degeneration of the SNc ipsilateral to the side of stroke with marked neuronal loss, gliosis and a few macrophages, with no reactive neovascularization (Ogawa et al., 1997).
It remains unclear in the aforementioned studies if basal ganglia infarcts and stroke-induced SNc degeneration led to Parkinson's disease (PD)-like symptoms. Movement disorders are an infrequent problem following ischemic and hemorrhagic stroke, and if so progress into either hyperkinetic or hypokinetic conditions (Handley et al., 2009). Typically, when these conditions arise, they stem from neuronal damage in different locations of motor circuitry, but most usually from the basal ganglia and thalamus (Handley et al., 2009). Interestingly, one large observational study has reported an increased risk for a first-time diagnosis of PD following a previous stroke, and also the risk of a first-time ischemic stroke in recently diagnosed PD patients (Becker et al., 2010). Furthermore, striatal silent lacunar infarction may contribute to SNc degeneration and promote progression of PD (Rodriguez-Grande et al., 2013; Zhang et al., 2016). Meanwhile, it remains unclear if the increased risk of PD following stroke is linked, at least in part, to the vascular changes and ischemic neuronal injury brought by the cerebrovascular insult (Becker et al., 2010). Alternatively, coincidence of PD/parkinsonism and cerebrovascular disease in some patients may likely be explained by the higher prevalence of these conditions in aging population (Korczyn, 2015). Likewise, olfactory dysfunction is now recognized as a prodromal sign of many neurodegenerative diseases notably in PD patients, whose olfactory bulbs display a loss of mitral and tufted cells that relay odorant information to brain cortical areas (Doty, 2012; Cave et al., 2016). There are few literature cases of olfactory dysfunction in humans following stroke but none have reported cell loss in olfactory bulb (Rousseaux et al., 1996; Moo and Wityk, 1999; Wehling et al., 2015). Data obtained using transient forebrain ischemia in gerbils point to a differential cell vulnerability to ischemic insult, notably a delayed neuronal loss in glomerular and external plexiform cell layers while mitral cells are spared during the timeline studied (Koh et al., 2004; Her et al., 2007).
Stroke Can Impact the Response of the Dopaminergic System to DA-Modulating Drugs
In a MCAO stroke model in rats 8 weeks or 5 months old at the time of stroke, ischemic animals showed significantly less of a catalepsy response to haloperidol (a non-selective D2R antagonist) as compared to age-matched sham animals. Young ischemic rats had the least catalepsy response at 2 h after drug injection, however they returned to the level of sham animals sooner than aged rats. In a test of locomotor activity following AMPH administration at 5 weeks poststroke, ischemic animals in both age groups reacted with a significantly greater increase in locomotor activity in response to AMPH, despite all groups having similar pre-drug locomotor activity levels. Again, the young ischemic animals had a more dramatic reaction than the older ones, but also returned to baseline levels more quickly, whereas the older ischemic animals were significantly more active than controls at the conclusion of the experiment, 2 h after AMPH administration. The aged and young control animals reacted similarly to AMPH. The authors hypothesize that lower levels of DA receptors due to cell loss in the striatum likely drove the attenuated response to haloperidol based antagonism, whereas a hyperresponsive mesolimbic system due to striatal damage, may have caused the enhanced reaction to AMPH (Borlongan et al., 1995). Interestingly, this pharmacological study shows a differential response of the DA system to stroke based on age, much like the transcriptional study discussed above (Sieber et al., 2014).
A recent study has also shown an altered response to drug manipulation of the dopaminergic system after stroke (Huang et al., 2017). In rats trained to consume alcohol prior to endothelin-1-induced (ET-1) stroke in the MCA territory, there was a greater preference for alcohol than that of sham animals. Huang et al. (2017) reported that spontaneous and stimulated firing of dorsomedial striatum (DMS)-projecting dopaminergic SNc neurons were higher in the side ipsilateral to stroke. They hypothesized that the aberrant behavior was due to a loss of dorsolateral striatal GABAergic projections to the SNc, releasing the inhibition on the DMS-projecting-dopaminergic SNc neurons (Huang et al., 2017). While this study may have many interesting implications for risks of alcoholism after stroke, it is unclear how this may relate to non-alcoholic patients, as the animals were pretrained to drink alcohol prior to stroke and the schedule of exposure to alcohol continued between non-testing days. Thus, these results may reflect the effect of chronic alcohol exposure after ischemia and not the natural condition of the DA system after stroke.
Most of the studies to date have used variations on MCAO in rodent models. This fact could be creating a misleading homogeneity in the results. Although strokes in the territory of the MCA are the most common in humans, many of these vary from the typical MCAO model stroke, and other stroke types do occur in humans. One human study with 48 stroke patients found that lesions in the brainstem correlated to decreases in DA levels and increases in HVA levels (indicating increased DA turnover), whereas cortical and striatal lesions were linked to increases in DA levels and decreases in DA turnover (Hama et al., 2017). These results must be interpreted with caution, as patients were tested at varying time points in the 3 months following stroke, while, as animal studies have shown, a given patient could have vastly different DA system landscape depending on the time point analyzed. DA and HVA levels were determined based on a 24 h urine sample, which does not necessarily perfectly represent brain levels (Hama et al., 2017). Nonetheless it reinforces the idea that different strokes may affect the DA system differently. Therefore, future animal work on the subject using more mobile and adaptable stroke methods such as endothelin-1 (ET-1) or photothrombosis will be required to test this. Lastly, more data regarding the state of the DA system following different strokes would help in deciding when and where therapies modulating DA systems are best applied.
Animal Studies on the Effect of DA-Enhancing Drugs in Poststroke Motor Recovery
A number of tests have been developed to evaluate motor and sensorimotor function in rodents. In many of these tests there is a measurable deficit in response to stroke induction with one or more of the various stroke models. A complete review of these tests is beyond the scope of this paper, and readers are directed toward other reviews (Durukan and Tatlisumak, 2010; Schönfeld et al., 2017). It is not entirely clear whether these behavioral tests are indicative of brain regions controlling specific motor tasks. In fact, it is generally agreed upon that these behavioral tests provide a broad metric of the sensorimotor function, for which the underlying brain connectivity patterns and neuronal circuits have yet to be fully established. Results of studies employing DA-enhancing drugs in poststroke motor recovery are summarized in Table 1.
Behavioral Paradigms Show Rapid Improvement of Motor Skills
Studies have demonstrated effects of drugs which increase DA release, beginning during the period of drug administration. Many of those have explored the effects of AMPH on motor and sensorimotor recovery following brain injury since a beneficial effect of AMPH on righting-attempts in decerebrated cats was first observed in 1946 (Maling and Acheson, 1946).
Beam Walk Test
A large amount of work has been done using the beam walking test to evaluate AMPH facilitated recovery in rats and cats following brain damage/stroke. This finding has been robust, with accelerated recovery being seen in many studies, usually very quickly after AMPH administration (Feeney et al., 1982; Hovda and Feeney, 1984; Sutton et al., 1989; Goldstein and Davis, 1990a,b,c; Boyeson and Feeney, 1991; Schmanke et al., 1996). Feeney et al. (1982) showed that the benefit of AMPH could be blocked by restraining the animals during the active period of the drug, which suggested the importance of experience in conjunction with AMPH. Interestingly, the effect could be blocked by coadministration of haloperidol, indicating an important role for catecholamines, notably DA receptors (Feeney et al., 1982). It should be also noted that the effect of haloperidol alone in this study delayed the spontaneous biological recovery in rats with cortical damage but not in recovered control animals (Feeney et al., 1982).
Meanwhile, several studies did not show a benefit to AMPH treatment on performance on the beam walking task (Boyeson and Feeney, 1991; Brown et al., 2004; Auriat and Colbourne, 2008). Boyeson and Feeney (1991) performed cerebellar ablation lesions and found AMPH made the animals worse than saline controls, although none of the groups showed the same degree of spontaneous recovery seen in cortical ablation studies suggesting that gross motor deficits resulting from cerebellar lesions may have low rates of recovery. Similarly, the striatal lesions in the intracerebral hemorrhage model in the Auriat and Colbourne (2008) study were not responsive to this therapy. It may be that subcortical and cerebellar lesions are not responsive to this treatment; possibly these areas are the sites of action for the early AMPH effect seen in the other beam walking studies. Likewise, no benefit of AMPH on the beam walking task was also reported using a cortical photothrombosis model, which creates a very different injury than an ablation or electrolytic lesion model (Brown et al., 2004). The discrepant results obtained in these two rat studies may be explained in part by the different methodology for training on the beam walk, which did not use aversive stimuli (Brown et al., 2004; Auriat and Colbourne, 2008). Instead of aversive stimuli, as is typically used, the animals were trained by being placed successively farther from the goal box.
Further work in the rat beam walking paradigm shows this effect can be seen with the AMPH related drugs MPH, phentermine and phenylpropanolamine (Feeney et al., 1993). Some studies show enhancement of recovery with NE alone, administered via interventricular infusion or transplant of adrenal medulla cells (Boyeson and Feeney, 1990; Feeney et al., 1993). Similar interventricular infusion experiments, as well as work with the mixed D1R/D2R agonist apomorphine, did not indicate a prominent role for DA in this fast-acting effect on beam walking ability. It seems likely that the role of the DA system becomes more obvious given multiple administrations, and a longer time course. In the rat beam walking paradigm, α1-adrenergic receptor (α1AR) antagonists can inhibit the AMPH mediated enhancement of motor recovery, although α1AR agonists were not found to be sufficient to reproduce this recovery. The α2-adrenergic (α2AR) agonists slowed recovery, while antagonists could facilitate it. These findings seem to contradict the theory that the effect of AMPH is mediated solely through NE. Interestingly, desipramine, which blocks NE reuptake but is not a stimulant, is able to facilitate recovery, while MK-801, which blocks NE reuptake but is also an NMDA receptor antagonist, cannot (Feeney et al., 1993).
Although work in this paradigm has provided valuable insights and directed more recent work, it should be noted that results on this test are reported using an ordinal scale, which spans a wide range of capabilities in relatively few categories. On the two lowest levels of the scale the rat does not cross the beam at all. Performing the task with aversive stimuli increases the stress of the task for the animal. The aversive stimuli and AMPH may intersect to affect the way the animal experiences practice sessions, perhaps inducing animals to be more active and to respond more often to the stimuli and perform the test, getting more practice on the task. It should also be noted that prodding rats forward during the beam walking task was seen to improve performance in lesioned rats during both massed and spaced trials, indicating that motivational factors may be important on this task (Goldstein and Davis, 1990c). Furthermore, attempts to replicate positive effects of AMPH on beam walking in more modern stroke models which employ ischemia or hemorrhage, have not been successful.
Tactile Placing in Cats
Another classic paradigm for testing the effect of AMPH on recovery from stroke is testing tactile placing after stimulation of the dorsal paw surface in cats with sensorimotor cortex ablations. Recovery and reaction to AMPH seems to be determined by the lesion location. Large prefrontal cortex lesions never show recovery regardless of AMPH administration (Hovda and Feeney, 1984). Lesions affecting primarily the visual cortex, showed early and lasting complete restoration after injection with AMPH. Like in beam walking this effect could be blocked with haloperidol (Hovda et al., 1987). A study affecting the motor cortex led to permanent deficits in paw placing in most animals, but exposure to AMPH could temporarily restore tactile placing. Animals tested at 4 days post lesion had very minimal and short-lived recovery of the response at 3 h after the drug. Animals tested at 9 and 15 days after lesioning had much more robust responses that lasted about 12 h after administration of AMPH. In partly recovered cats, but not unlesioned cats, this recovery could be blocked with haloperidol (Feeney and Hovda, 1983). Due to the early onset of recovery, and the ability for the animal to respond positively to AMPH at 4 days postop, a time thought to be too soon for many plasticity and sprouting processes, these results have been taken to indicate that a rapid response to the drug is involved. However, the weakness of the response at 4 days postop may suggest that while AMPH may be of some benefit that early, it becomes more powerful at later time points when slower acting recovery mechanisms may have come into play. Again, haloperidol was able to block the benefit of AMPH. Interestingly, a weaker and more transient, but still statistically significant, increase in tactile placing was seen in cats given 0.25 and 0.5 mg/kg apomorphine. The use of higher and lower doses showed no effect (Feeney and Hovda, 1983).
Behavioral Paradigms Demonstrate Recovery After a Delay
In several motor behavioral tasks showing recovery mediated by AMPH, L-DOPA or VTA stimulation, recovery was shown not during the first period of administration, but after a delay. In these studies repeated administration was used.
Tactile Placing in Response to Vibrissae or Forelimb Stimulation in Rats
A paradigm similar to tactile placing in cats has been used in rats, this time with more lasting recovery. The paw placing response to vibrissae stimulation or forelimb stimulation can recover in rats with small electrolytic lesions of the motor cortex, given three administrations of AMPH. Rats recover sooner and more completely on placing in response to vibrissae stimulation, than they do on forelimb stimulus-forelimb placing (Schmanke et al., 1996; Schmanke and Barth, 1997). In both vibrissae > forelimb and forelimb > forelimb paradigms performance that is significantly greater than vehicle controls appears after a delay (10 and 35 days, respectively) (Schmanke et al., 1996; Schmanke and Barth, 1997).
The Foot Fault Test and the Horizontal Ladder
The foot fault test and the horizontal ladder test both involve an evaluation of the percentage of missteps an animal makes while traversing an elevated grid or ladder surface. While not a fine motor task, this task requires more refined motor control than the beam walking task (Barbay and Nudo, 2009). Among studies showing a benefit of AMPH on this test, all gave AMPH at least twice, indicating that more chronic stimulation may be more effective in this paradigm (Stroemer et al., 1998; Ramic et al., 2006; Papadopoulos et al., 2009; Wolf et al., 2014).
Skilled Reaching Tests
Many studies have also been done using skilled pellet reaching tasks in rats. Several studies have had success demonstrating accelerated or superior recovery on pellet reaching tasks when multiple AMPH administrations are paired with either training or focussed activity, although AMPH alone or paired with environmental enrichment also showed recovery above the level of controls in some studies (Adkins and Jones, 2005; Gilmour et al., 2005; Ramic et al., 2006; Papadopoulos et al., 2009; Wolf et al., 2014). In non-human primates following cauterization of surface vasculature in the hand representation, one dose of 0.25 mg/kg AMPH and 14 days of training initiated 10 days postop was able to significantly improve performance on a skilled reaching task as compared to controls on days 4, 5, and 8–13 of training. AMPH-treated monkeys were still significantly better than rehab only controls at 9 weeks postop (Barbay et al., 2006). The efficacy of a single dose of AMPH in a non-human primate model, when multiple doses are typically required in rodent models may be due to the differences in lesion type.
Among the studies that did not demonstrate a benefit of AMPH on skilled reaching recovery there are several possible factors (Rasmussen et al., 2006, 2011; Alaverdashvili et al., 2007; Auriat and Colbourne, 2008). Alaverdashvili et al. (2007) used an oral administration route, and used all female mice, which may have affected the drug bioavailability (Barbay and Nudo, 2009). Additionally, studies looking at recovery of the skill reaching task in rats with striatal damage showed no benefit to AMPH (Rasmussen et al., 2006, 2011; Auriat and Colbourne, 2008). These studies suggest that benefits gained from AMPH administration potentially depend on the location of stroke lesion.
Other Behavioral Tests
A single study, using long-term administration of 2 mg/kg AMPH in rats with MCAO lesions, saw reduced infarct size in the AMPH group and improvements in turning asymmetry and body posture in the body swing test (Liu et al., 2011).
Only one study in animals has demonstrated improved motor recovery on behavioral tests using L-DOPA treatment after stroke. In a MCAO stroke model, rats exhibited significantly better recovery on the rotating pole test at various speeds, the cylinder test and a neuroscore, when animals were given a high dose (20 mg/kg) of L-DOPA. On some tests improved recovery could be seen at the 5 mg/kg dose (Ruscher et al., 2012). As 5% or less of L-DOPA is converted to NE, this effect is very likely mediated by DA (Nutt and Fellman, 1984). In lesion studies on rats, VTA stimulation led to recovery of pre-lesion performance levels on a lever pressing to stop aversive stimuli task within 4 days, vs. no recovery seen in controls (Castro-Alamancos et al., 1992; Castro-Alamancos and Borrell, 1995). Data obtained with L-DOPA and VTA stimulation, in which the mechanism of action is most likely linked to the dopaminergic system, suggest that DA plays also a role in AMPH-triggered effects reported in aforementioned studies.
Potential Benefit of Pairing Forced Use of the Impaired Limb and DA-Enhancing Pharmacotherapy
Forced use of the impaired limb by immobilizing the non-affected limb as part of training or during exposure to environmental enrichment, in conjunction with AMPH, showed improvement on the cylinder test, but not on the speed of beam crossing (Goldstein, 2009). In skilled reaching tests, animals were prevented from using their unimpaired limb during training and testing either by the setup of the apparatus, with success (Barbay et al., 2006) or via application of a tape-bracelet to prevent reaching through narrow slots (Alaverdashvili et al., 2007) without seeing recovery and by bandaging the ipsilateral limb during testing (Gilmour et al., 2005). These approaches lead to skilled reaching improvement but not improved recovery on the foot fault test. Overall, these studies suggest that there may be some benefit in combining constraint-induced movement therapy (CIMT) with DA-enhancing approaches.
Potential Mechanisms of Action of DA-Enhancing Drugs
Reversal of Catecholamine Diaschisis
Due to the rapid time course of response to AMPH on the beam walking paradigm, and the tactile placing test in cats, the dominant theory regarding the mechanism by which AMPH evokes benefits on these tasks is a resolution of diaschisis in depressed regions remote to the injury site. In cats given bilateral lesions of varying sizes, this improved recovery is still observed on the beam walking task. This demonstrates that the rapid improvement on beam walking is not dependent on the corresponding contralateral region, nor on the adjacent regions (Sutton et al., 1989). The resolution of diaschisis theory is supported by the finding that cytochrome oxidase activity, which provides a readout of cellular energy production, is supressed in rats with cortical ablations in a number of regions important for motor performance, but this suppression can be reversed with a single injection of 2 mg/kg AMPH 24 h after ablation (Sutton et al., 2000). Additionally, it has been shown that AMPH can attenuate traumatic brain injury-triggered remote reductions in cerebral metabolic glucose utilization (Queen et al., 1997). It may be that this rapid effect is more strongly mediated by NE than by DA, which would fit well with the findings mentioned above showing NE is more effective in the beam walking paradigm, and the weaker effect of apomorphine on tactile placing in cats. It is possible that a DA-mediated augmentation of recovery requires a longer time course to work, or perhaps requires repeated stimulation of the dopaminergic system to be fully apparent.
Enhanced Synaptic Plasticity, Axonal Sprouting, and Cortical Map Reorganization
Synaptic Plasticity
The results implicating NMDA receptors in the early recovery response to AMPH indicate a role for long-term potentiation (LTP) processes in this rapid AMPH mediated recovery. AMPH has been shown to increase LTP in the hippocampus and the prefrontal cortex in non-injured rodents (Delanoy et al., 1983; Xu et al., 2010). Interestingly, in the prefrontal cortex AMPH-driven augmentation of LTP was primarily mediated by D1R, except in the case of hyperdopaminergic mice, where AMPH reinstated LTP via a recruitment of β-adrenergic receptors. Both of these receptors are coupled to the cyclic AMP (cAMP)-protein kinase A pathway (Xu et al., 2010). DA receptors have been shown to be important modulators of LTP in many parts of the brain, including PFC (Gurden et al., 1999, 2000; Huang et al., 2004; Wirkner et al., 2004; Hotte et al., 2005, 2006; Ruan et al., 2014), hippocampus (Otmakhova and Lisman, 1996; Lemon and Manahan-Vaughan, 2006) and striatum (Calabresi et al., 1997, 2000, 2007; Centonze et al., 2001, 2003; Dudman et al., 2003; Wolf et al., 2003; Shen et al., 2008). Most relevantly, DA receptors, in particular D1-class subtypes, have been heavily implicated in motor learning processes in both striatum and primary motor cortex (Willuhn et al., 2003; Luft et al., 2004; Willuhn and Steiner, 2006, 2008; Molina-Luna et al., 2009; Hosp et al., 2011; Rioult-Pedotti et al., 2015). Evidence from human studies of patients with Korsakoff's syndrome (McEntee et al., 1987), and in healthy human patients using transcranial magnetic stimulation (TMS) suggest that DA receptors play an important role in these processes in humans (Floel et al., 2005, 2008; Breitenstein et al., 2006; Meintzschel and Ziemann, 2006; Nitsche et al., 2006, 2009; Kesar et al., 2017).
Axonal Sprouting
There is evidence implicating longer term processes for delayed recovery. Several studies which showed improvement on the foot fault and horizontal ladder test as well as skilled reaching tasks, saw improvement was associated with indicators of axonal sprouting and neurite growth. Stroemer et al. (1998) reported significant increases in growth associated protein 43 (GAP-43), a synaptogenesis marker, at 3 and 7 days relative to saline-injected animals. From day 14 postop to 2 month postop, AMPH-treated animals had increased distribution of synaptophysin, which also indicates synaptogenesis, on the side contralateral to the infarct (Stroemer et al., 1998). Biotinylated dextran amine neuroanatomical tracing was used to demonstrate significant increases in fibers projecting across the midline from the uninjured cortex at the level of the basilar pontine nuclei, red nucleus and cervical spinal cord in AMPH-treated animals (Ramic et al., 2006; Papadopoulos et al., 2009; Wolf et al., 2014).
Many of the studies discussed herein have shown recovery mediated by enhanced sprouting in the contralateral hemisphere (Ramic et al., 2006; Papadopoulos et al., 2009; Wolf et al., 2014) while there is evidence for recovery mediated by the infarcted hemisphere (Liu et al., 2011). However, the role of the contralateral hemisphere in motor recovery is a controversial one. Although the assumption of lost motor function by the intact hemisphere has been shown to improve outcome, this recovery mechanism is correlated with worse outcomes than the resumption motor control by the affected side (Boyd et al., 2017). It has been suggested that perhaps those who are more seriously afflicted may be more likely to undergo contralateral side-based recovery because their affected hemisphere is unable to recover. In such cases sprouting from the contralateral hemisphere may be the most powerful recovery mechanism available (Hallett, 2001; Jang, 2013).
Cortical Map Reorganization
In motor cortex ablation studies, immunostaining for c-Fos, a marker of neuronal activity and plasticity, indicated that the forelimb representations associated with lever pressing ability had relocated to the hindlimb after forelimb lesions, but only in rats receiving VTA stimulation during lever pressing (Castro-Alamancos et al., 1992). Lesions to the hindlimb area in recovered animals reinstated the post-ablation deficit on the forelimb lever pressing task (Castro-Alamancos et al., 1992). In a follow-up study, the forelimb motor area of rats was mapped before lesion and only the forelimb area targeted was in the lesion operation (Castro-Alamancos and Borrell, 1995). Again, better motor recovery was seen in rats subjected to VTA stimulation. Post-injury mapping of this group showed the forelimb motor area had moved to an area caudal of the lesion. Little to no forelimb area reorganization was seen in unstimulated animals. Thus, results obtained with VTA stimulation suggest a role for DA in cortical remapping processes.
An important issue with the use of lesioned animals in the above-mentioned studies is that they are not the best experimental models to study recovery following ischemic stroke. Interestingly, a study on somatosensory recovery following photothrombotic stroke found that poststroke administration of haloperidol impaired the rate of spontaneous recovery of the somatosensory system as compared to controls (Obi et al., 2018). The spontaneous recovery was linked to increased DA levels and astrocytic activity in the contralateral hemisphere. While mice in this model regain sensation spontaneously, recovery processes also lead to maladaptive plasticity, in the form of a shift to single neurons receiving stimulation from more than one limb, visible as early as 1 week after stroke, and fully developed by 4 weeks poststroke. Administration of partial D2-class agonist aripiprazole starting 2 weeks after stroke halted this process and resulted in significantly less neurons receiving input from all four limbs (Obi et al., 2018). These findings suggest that D2R may play a role in the prevention of maladaptive cortical map reorganization.
Modulation of Growth Factor Expression
Fibroblast Growth Factor 2 (FGF-2)
In addition to being a neuroprotective agent in stroke, FGF-2 (a.k.a. basic fibroblast growth factor) can act as an angiogenic factor to promote sprouting and neurogenesis in stroke models (Lin and Finklestein, 1997; Wada et al., 2003; Issa et al., 2005; Slevin et al., 2006; Paciaroni and Bogousslavsky, 2011). FGF-2 expression in VTA is augmented following AMPH administration (Mueller et al., 2006), which may play a role in poststroke recovery. Indeed, a study using the transient MCAO stroke model showed that AMPH-enhanced poststroke motor performance of rats was accompanied with a transient increase in FGF-2 expression in layer V sensorimotor neurons in the unlesioned cortex at 2 weeks poststroke, along with enhanced axonal sprouting across the midline from the unlesioned cortex (Wolf et al., 2014). Additionally, the study reported that media from FGF-2 conditioned astrocytes were able to stimulate neurite outgrowth in primary cultured neurons, which was linked to activation of β- and α1-adrenergic receptors. However, the role of DA receptors was not tested (Wolf et al., 2014). This issue is of potential clinical relevance for dopaminergic pharmacotherapy in poststroke motor recovery as DA receptor activation increases FGF-2 levels (Reuss et al., 2000; Roceri et al., 2001; Fumagalli et al., 2003, 2006a; Li et al., 2006; He et al., 2008; Zhang et al., 2009).
Brain-Derived Neurotrophic Factor (BDNF)
BDNF has been linked to enhanced motor recovery following stroke via increased sprouting, neuronal plasticity and neurogenesis, and has thus been implicated as a factor in the beneficial effects of rehabilitation and exercise (Kurozumi et al., 2004; Schäbitz et al., 2007; Ploughman et al., 2009; Mang et al., 2013; Berretta et al., 2014; Livingston-Thomas et al., 2016; Cook et al., 2017). AMPH administration during poststroke recovery in a transient MCAO rat stroke model leads to an increase in the levels of axonal fiber and white matter growth (ipsilateral side), neurofilament (ipsilateral and contralateral side), synaptophysin (ipsilateral side), matrix metalloproteinase 2 activity (ipsilateral side cortex), and mRNA for BDNF (ipsilateral side cortex) (Liu et al., 2011). These findings suggest an increase in sprouting processes in the infarcted cortex modulated by an AMPH-dependent BDNF expression. Interestingly, the infarct-induced reduction of BDNF mRNA levels in the lesioned cortex was attenuated by the poststroke AMPH treatment (Liu et al., 2011). Likewise, DA-enhancing drugs used in the treatment of Parkinson's disease (e.g., L-DOPA and MAO-B inhibitors) are also known to increase BDNF levels (Fumagalli et al., 2006b). Meanwhile, the mechanism underlying AMPH-induced BDNF increase during poststroke recovery is unclear, but likely involved the dopaminergic system. In fact, DA receptors can increase BDNF protein levels through D1R, D5R and D1R-D2R heterodimers or through D1R-mediated transactivation of tropomyosin receptor kinase B (TrkB), the BDNF receptor (Küppers and Beyer, 2001; Iwakura et al., 2008; Hasbi et al., 2009; Perreault et al., 2012; Xing et al., 2012).
Glial Cell-Derived Neurotrophic Factor (GDNF)
GDNF has been shown to promote neurogenesis and has been associated with the beneficial effects of exercise in stroke recovery (Kobayashi et al., 2006; Ohwatashi et al., 2013). As far as we know, whether GDNF expression can be modulated by AMPH administration during poststroke recovery remains to be firmly established. Meanwhile, studies have reported that DA receptor stimulation by direct agonists can augment GDNF levels (Ohta et al., 2004, 2010; Du et al., 2005; Carnicella et al., 2009; Ahmadiantehrani and Ron, 2013), suggesting that DA-enhancing drugs such as AMPH and L-DOPA mediate their beneficial effects on poststroke recovery in part through a modulation of GDNF expression. Indeed, Kuric et al. (2013) showed that L-DOPA treatment caused an increase in GDNF in the infarct core and peri-infarct region in a rat MCAO stroke model. The GDNF increase was attributed to D1R-expressing reactive astrocytes which appear in the peri-infarct region around 7 days following stroke. Interestingly, in cell culture, this relationship was only seen following oxygen-glucose deprivation experiments (Ruscher et al., 2012; Kuric et al., 2013).
Potential Recruitment of Cocaine- and Amphetamine-Regulated Transcript
Cocaine- and amphetamine-regulated transcript (CART) has been marked as a potential neuroprotective agent, however some data suggests it may be able to mediate poststroke recovery after the acute stage as well (Xu et al., 2006; Jia et al., 2008). CART was found to be lower in the ipsilateral hemisphere of stroke animals following stroke and slowly returned to control levels in a manner that was not modulated by AMPH (Liu et al., 2011). In a MCAO model, mice receiving intranasal administration of CART peptide 3 days poststroke showed improved motor performance, and a number of markers indicating enhanced sprouting of neurons, increased neurogenesis and better vascular recovery (Luo et al., 2013; Liu et al., 2016). CART is unlikely to be the major mediator of the effects of AMPH following stroke (Rogge et al., 2008; Luo et al., 2013). Nonetheless, with its strong interaction with the reward system and the co-localization of CART with DA receptors in some neurons receiving dopaminergic projections, it seems very likely that CART is able to respond to the effects of dopaminergic stimulation (Hubert et al., 2008; Rogge et al., 2008; Hu et al., 2015; Borkar et al., 2018).
Modulation of Immune and Inflammatory Responses
L-DOPA treatment in the MCAO paradigm was also linked to modulation of the immune and inflammatory response to stroke. The immune response to stroke is complicated, and still poorly understood. It doubtlessly includes elements that are part of endogenous recovery processes, and elements that are maladaptive in overall recovery. Cytotoxic T-cells may be one of these detrimental immune elements, and could contribute to blood-brain barrier permeability, increase inflammation and release of cytotoxins. Animals treated with L-DOPA had lower levels of cytotoxic T-cells (CD3+CD8+ T-cells), lower levels of pro-inflammatory cytokines IL-5, IFN-⋎, IL-4, and TFN-α in the infarcted hemisphere and lower level of major histocompatibility complex class II (MHC II) cells in the infarct zone (Kuric and Ruscher, 2014a,b). These MHC II cells expressed D1R. Meanwhile, L-DOPA treatment was able to ameliorate ischemia-induced lymphocytopenia and increase levels of CD3+CD4+ T-helper cells in the blood (Kuric and Ruscher, 2014c). Similar to the findings of de novo L-DOPA responsiveness in reactive astrocytes, de novo expression of D2R has been observed on microglia following stroke. These microglia were observed in the infarct area and were responsive to a D2R/D3R agonist (Huck et al., 2015). In an intracerebral hemorrhage stroke (ICH) model, D2R expression in microglia, astrocytes and neurons increased in the perihemorrhage region around 24 h after ICH and stayed high for 7 days poststroke. D2R knockdown led to increases in pro-inflammatory cytokines and chemokines in the infarct region. This D2R-mediated effect was linked to levels of the anti-inflammatory protein αB-crystallin, which was expressed in a similar pattern as D2R following ICH (Zhang et al., 2015). A further study, in a mouse MCAO model, found that the anti-inflammatory bioactive alkaloid sinomenine, was able to reduce inflammatory processes after the stroke, and that this was mediated through an increase in D2R expression on astrocytes, as well as an increase in αB-crystallin levels (Qiu et al., 2016).
Vascular Recovery
One region of stroke recovery which is often overlooked is vascular recovery. While endogenous recovery processes do promote angiogenesis, there is evidence that these processes can be modulated. In the wake of stroke it is important to re-establish blood flow, and appropriate blood flow can help support recovery processes (Arai et al., 2009; Liu et al., 2014). As mentioned above, DA has been linked to molecules which show angiogenic effects following stroke, such as FGF-2 and CART. DA receptors are also capable of increasing cerebral blood flow, possibly through a D1R mediated pathway, and D1R/D5R are found on the microvasculature (von Essen, 1974; Krimer et al., 1998; Choi et al., 2006; Tan, 2009; Ohlin et al., 2012). These findings suggest that DA may be able to modulate this aspect of recovery. Indeed, the D1-class agonist dihydrexidine has been shown to enhance cerebral perfusion in humans (Mu et al., 2007).
A Pleiotropic Role of DA in Stroke Recovery
Given the diverse findings surrounding possible mechanisms of a dopaminergic system-mediated enhancement of recovery following stroke, it seems likely that DA plays a pleiotropic role in stroke recovery processes (Figure 1). While DA may not be the driving force behind reversal of diaschisis, it is implicated in plasticity processes such LTP and long-term depression (LTD), which lead to motor-map reorganization. Recently, an elegant study has demonstrated that activation of the cyclic adenosine monophosphate (cAMP) response element binding (CREB) protein plays an important role in poststroke recovery from motor deficits (Caracciolo et al., 2018). This might also be of functional relevance for a role of DA in recovery from stroke as it can regulate CREB activity (Hyman et al., 1995; Gershon et al., 2007; Belgacem and Borodinsky, 2017). Additionally, DA appears to play a role in sprouting of spared neurons, and support of new born neurons through potentially modulation of growth factor expression. DA is also implicated in the modulation of immune and inflammatory processes and may play a role in promoting angiogenesis. Recovery from stroke is a complicated process, and a pharmacological treatment which is able to modulate multiple aspects of recovery may be beneficial to stroke sufferers.
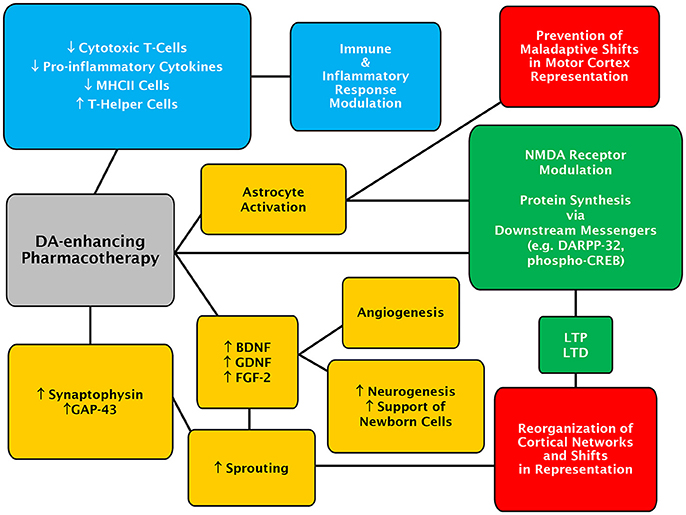
Figure 1. Concept map of the potential mechanisms of action for DA-enhancing pharmacotherapies in poststroke recovery. Mechanisms of actions and effects evoked by DA-enhancing drugs, which are likely to be primarily mediated through D1- and D2-class receptors, are depicted in colored boxes. The DA-enhancing actions culminating in astrocytic-dependent regulatory processes are in yellow while those involving the recruitment of neuronal signaling partners and modulation of synaptic adaptation are in green. Immune and inflammatory-linked events are in blue. Lastly, changes at the level of cerebral cortex are shown in red.
Clinical Evidence for the Use of DA-Enhancing Drugs in Motor Recovery From Stroke
Results of clinical studies employing DA-boosting drugs to enhance motor recovery following stroke are summarized in Table 2.
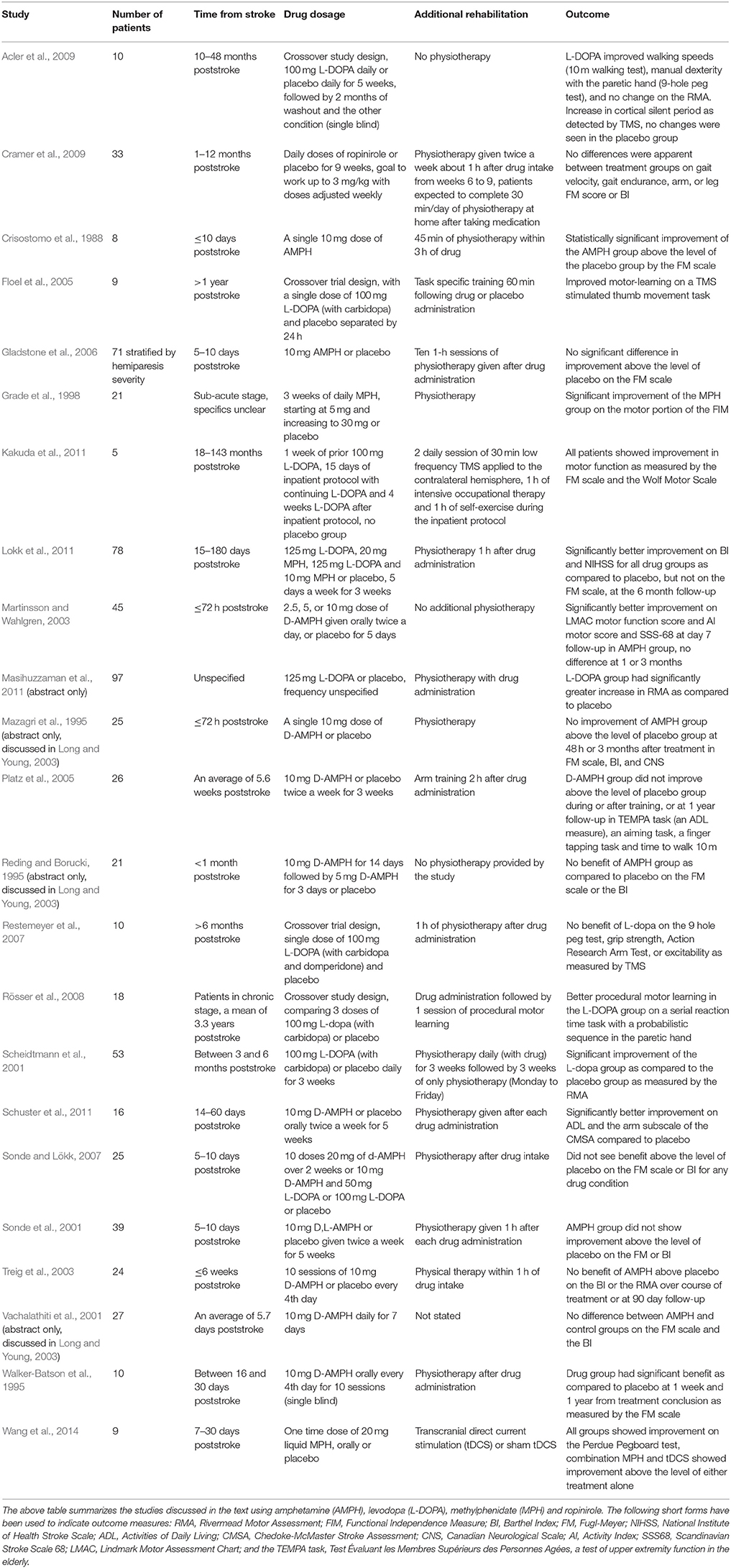
Table 2. Summary of clinical trials using indirect and direct dopaminergic agonists to improve poststroke motor recovery.
Amphetamine Studies
The successful transfer of the beneficial effect of AMPH observed in animal stroke models into medical practice have been hampered by the mixed results obtained in clinical trials. Indeed, an early study with eight stroke patients showed that a single dose of AMPH followed by physical therapy could significantly increase motor function improvement above the level attained with placebo and physical therapy (Crisostomo et al., 1988). Meanwhile, another preliminary study in 25 patients found a single dose of AMPH was not sufficient to improve motor function recovery above the level of placebo treatment (Long and Young, 2003). Some studies employing repeated dosing and physiotherapy, usually at an interval of several days, have shown a significant benefit of AMPH on motor recovery and/or activities of daily living, particularly in the hemiparetic arm (Walker-Batson et al., 1995; Martinsson and Wahlgren, 2003; Gladstone et al., 2006; Schuster et al., 2011). Other trials using similar dosing patterns have found no benefit (Sonde et al., 2001; Treig et al., 2003; Platz et al., 2005; Gladstone et al., 2006; Sonde and Lökk, 2007). More aggressive dosing approaches, with daily AMPH, have yet to produce positive results (Long and Young, 2003). Unfortunately, studies supporting the use of AMPH for motor recovery often have low enrollment. Experimental group dissimilarity is also a concern, with many studies showing differences in mean patient age or in level of motor function after stroke, across both successful and unsuccessful trials. A 2007 meta-analysis of six studies (176 patients) that looked at motor recovery saw evidence of better relative change from poststroke baseline in patients given AMPH and a nearly significant effect of AMPH on activities of daily living score (Martinsson et al., 2007). A later meta-analysis did not observe a significant improvement of motor scores in patients given AMPH, although the trend for an increased motor benefit was still present (Sprigg and Bath, 2009).
L-DOPA Studies
Studies employing L-DOPA for motor recovery from stroke have had somewhat more success, although results are still mixed. In the sub-acute phase several studies showed L-DOPA and physical therapy improve recovery above the level of placebo and physical therapy (Scheidtmann et al., 2001; Lokk et al., 2011; Masihuzzaman et al., 2011). One study was only able to find a trend toward improvement with L-DOPA, although this group had only 4 patients in the L-DOPA group, and 7 patients in a combination L-DOPA and AMPH group, as well as a high degree of initial variability and an uneven distribution of deficits on the Fugl-Meyer arm portion (Sonde and Lökk, 2007). The study by Masihuzzaman et al. (2011) reported that both ischemic and hemorrhagic strokes responded well to the L-DOPA treatment. In the chronic phase a single dose of L-DOPA was sufficient to significantly boost motor learning on a TMS-induced thumb movement paradigm (Floel et al., 2005), but a single dose could not boost performance on the Nine-hole Peg Test, the Action Research Arm Test or on grip strength (Restemeyer et al., 2007). The study by Floel et al. (2005) trained participants on the test task, whereas, the Restemeyer et al. (2007) study employed a physiotherapy session with an emphasis on dexterity. These studies possibly suggest that a single dose is not enough to lead to generalizable motor improvement in a chronic stroke population, although it should also be noted that there was substantial variability in scores for the Nine-hole Peg test, and that performance on the Action Research Arm test (a test which is prone to ceiling effects) was high before intervention (Restemeyer et al., 2007; Duncan, 2013). In support of multiple doses being required for enhancing stroke recovery, a group of patients received three doses of L-DOPA over 2 days which led to increased procedural motor learning in the paretic hand without changes to motor arousal or response style (Rösser et al., 2008). Moreover, 5 weeks of L-DOPA without physiotherapy ameliorated scores on the Nine-hole Peg test and augmented speed of 10-meter walking, as well as lengthening the cortical silent period, while no change was seen in placebo group. Although the study was not able to show improvement on the Rivermead Motor Assessment, these findings indicate that L-DOPA alone could be sufficient to improve motor recovery and modulate cortical excitability (Acler et al., 2009). While the above findings do suggest that there is a beneficial role for L-DOPA in poststroke motor recovery, the trials performed thus far are not without flaws. Several of the trials have involved few patients or have used a crossover design, and one study only employed single blinding. Two large scale clinical trials called “Dopamine Augmented Rehabilitation in Stroke” (DARS) (Bhakta et al., 2014) and “Effect of Serotonin and Levodopa in Ischemic Stroke” (SELEIS, ClinicalTrials.gov identifier: NCT02386475), using L-DOPA and physical therapy in stroke patients are underway and perhaps will be able to give further insight into the efficacy of this treatment approach.
There has also been interest in combining L-DOPA pharmacotherapy with other emerging recovery strategies. One group tested an aggressive treatment plan involving a total of 7 weeks of daily L-DOPA, and 15 days of inpatient care that involved 20 min of low frequency TMS applied to the contralateral hemisphere, 1 h of one-on-one occupational therapy and 1 h of self-exercise, repeated twice daily, on chronic stroke sufferers. All patients in the small cohort showed improved motor function in the paretic arm, most of which was maintained at the 4 week follow up, however the absence of any control groups makes drawing any conclusions difficult (Kakuda et al., 2011). Lastly, the use of L-DOPA to facilitate robot-assisted motor therapy following stroke has also been proposed (Tran et al., 2016).
Methylphenidate (MPH) Studies
The use of MPH to enhance stroke recovery has also been tested in clinical studies. One to three weeks of MPH combined with physical therapy significantly increased scores on the modified functional independence measure (FIM) and nearly significantly increased performance on the Fugl-Meyer scale in post-acute stroke patients (Grade et al., 1998). Moreover, Lokk et al. (2011) found that MPH and MPH + L-DOPA were able to improve scores on the Barthel Index and National Institute of Health Stroke Scale, but not on the Fugl-Meyer Scale, as compared to placebo treatment. Further, another study reported a single dose of 20 mg of MPH was able to improve performance on a finger tapping task, though not performance of grip strength or a target pursuit task (Tardy et al., 2006). The improvement in finger tapping was correlated to hyperactivity of the ipsilateral primary sensorimotor cortex and the contralateral premotor region, as measured by functional MRI during the drug's active period. A hypoactivity of the anterior cingulum was also observed under the MPH condition (Tardy et al., 2006). Interestingly, the combination of MPH and TMS led to significant motor benefits, which were above the level of either treatment alone on the Perdue Peg Board test (Wang et al., 2014). However, as no placebo group was included in this study, it is unclear if the modest improvement seen in peg board performance in the MPH alone group is of any potential clinical relevance. Likewise, while this study did not show changes in cortical excitability in any of their MPH or TMS groups, it is worth mentioning that MPH has been shown to regulate cortical excitability during a motor task in healthy patients (Kratz et al., 2009; Wang et al., 2014).
Direct Dopamine Agonists
To date only one study tested a direct dopamine agonist, the D2-class drug ropinirole, along with physical therapy to facilitate motor recovery from stroke (Cramer et al., 2009). In spite of all groups showing significant improvement over the study period, there was no benefit of ropinirole treatment in either recent (earlier than 3 months from onset) or later (between 3 and 12 months from onset) stroke cases. Importantly, ropinirole showed no serious adverse effects, and medication compliance was high, yet the majority of patients in the drug group never reached the planned dose (3 mg daily) for the study. Additionally, the drug group had a higher rate of comorbidities such as diabetes and depression, and the placebo group received a higher level of physiotherapy outside of the parameters of the study (Cramer et al., 2009).
Further studies in human stroke patients, examined drugs given to stroke patients for co-morbidities, classifying them as “potentially deleterious” or not, based on preclinical findings. The “potentially deleterious” group, which included dopamine antagonists, were found to worsen motor outcome (Goldstein and Davis, 1990a; Goldstein, 1995, 1998).
Why Have Clinical Results Been So Mixed?
There are many challenges inherent in translating results from animal studies into human patients, particularly in the stroke field (Corbett et al., 2017). Animal studies are typically done in young, otherwise healthy animals without comorbidities or other medications. Animal models have the benefit of producing extremely similar strokes in animals with identical genetic backgrounds. In clinical stroke studies factors such as age or stroke severity can have a greater impact on outcome than the expected benefit of a given treatment, so groups must be well balanced, or analysis should take co-variants into account (Bath et al., 2012). Furthermore, appropriately scaling factors such as dosage, timing and rehab intensity from animal to human models is likely to be of critical importance, but may not be straightforward (Adkins et al., 2009). Consider the issue of timing after stroke. While humans typically show spontaneous recovery over the 6 months following stroke, rodents see spontaneous recovery plateauing at about 4 weeks following stroke, however it is difficult to determine what the timing of the physiological recovery processes observed in animals would look like in humans (Chollet et al., 2014).
Stroke Lesion Location
Aside from the intricacies of dose, timing and rehab type and intensity, one potential problem as to why studies have had such mixed results is that studies may not be targeting the responsive patient populations (Chollet et al., 2014), nor are the studies large enough to allow for stratification of patient populations. In terms of characteristics of the stroke, responsiveness to pharmacotherapy could depend on time from onset of stroke, severity of deficit or stroke location. Stroke location should be considered carefully. One animal study showed that AMPH worsened outcome in rats given unilateral nigrostriatal pathway lesions (Mintz and Tomer, 1986). This may have relevance in patients who present with delayed substantia nigra degeneration ipsilateral to the infarct. For these patients an approach using direct agonists may be better than indirect agonists which could potentially culminate in higher risk of DA depletion. One study has reported that patients whose stroke occurs on their dominant side respond better to dopaminergic pharmacotherapy. This is believed to occur because the dominant hemisphere, equally boosted by the pharmacotherapy, may overpower and inhibit the infarcted, non-dominant hemisphere. As discussed in section Cortical Map Reorganization, increased assumption of function by the contralateral hemisphere is considered undesirable recovery strategy (Rösser and Flöel, 2008; Boyd et al., 2017). That is not to say that such patients cannot benefit from pharmacotherapy, however, they may also need to engage other strategies, such a cortical inhibition of the unaffected hemisphere using TMS, or CIMT to ensure optimal recovery.
Genetic Polymorphisms
Another factor that may be a source of a lot of variation between studies is the genetics of the individuals involved. Research suggests that polymorphisms in genes such as BDNF and apolipoprotein E may influence stroke recovery, and specifically may interact with treatments (Stewart and Cramer, 2017). Genetic polymorphisms potentially impacting dopaminergic response exist at varying rates in the population notably for COMT, DAT, D1R, D2R and D3R (Pearson-Fuhrhop et al., 2013). Taking into account the well-established inverted U-shaped dose-effect relationship to DA levels on a variety of behavioral outputs, the response of a patient to DA modulating treatments may differ greatly based on where their genetics place them on the curve (Cools and D'Esposito, 2011; Thirugnanasambandam et al., 2011; Floresco, 2013; Vaillancourt et al., 2013; Arnsten et al., 2015). Evidence linking variations in the gene for COMT to differences in motor skill outcome in stroke patients, given regular physical therapy treatment, suggests that baseline “dopaminergic tone” is one factor in recovery (Liepert et al., 2013; Kim et al., 2016). Thus, the genetics of the individual may also interact with DA-enhancing treatments. DAT polymorphisms have been shown to impact the response of children with attention deficit hyperactivity disorder (ADHD) to MPH in both behavioral and neurophysiological measures (Stein et al., 2005; Gilbert et al., 2006). Several studies in healthy adults have shown that use of drugs increasing DA levels are beneficial on motor learning, impulse control, and working memory tasks in participants whose genetics make them prone to a lower dopaminergic tone (Mattay et al., 2003; Pearson-Fuhrhop et al., 2013; MacDonald et al., 2016). In contrast, participants with a higher dopaminergic tone perform better at baseline, but see their performance worsen under the influence of DA-enhancing drugs (Mattay et al., 2003; Pearson-Fuhrhop et al., 2013; MacDonald et al., 2016). While most studies examine their results by single genetic factors, an emerging and promising approach is the use of a gene score, which assigns a direction of impact on the level of dopaminergic system activity to each polymorphism and evaluates participants by where they fall on a combined score (Pearson-Fuhrhop et al., 2013). This approach allows one to account for the combined effects of various polymorphisms. However, the direction of the predicted effect of the polymorphism on dopaminergic tone should be carefully investigated for all the genes involved, and the role of the gene in the specific process under investigation must be considered. Recently the COMT gene rs4680 single nucleotide polymorphism that changes the wild type G allele (Val) to an A allele (Met) at amino acid 158 (Vat158Met) has been assessed with respect to motor learning under L-DOPA using this gene scoring approach (Pearson-Fuhrhop et al., 2013). Pearson-Fuhrhop et al. (2013) assigned individuals who are homozygous for the G allele (Val) a score of 0 for that gene, and individuals with the A allele a score of 1, because the Met variant of COMT has a lower activity and therefore people with this gene will have greater extra-synaptic levels of DA. Interestingly, they found that their gene-score model fit better to the motor learning under L-DOPA results obtained, when the score for the COMT gene was taken out of account (Pearson-Fuhrhop et al., 2013). The logic behind this interpretation of the COMT polymorphism seems sound when you consider that the A allele of the COMT gene (Met variant) was associated with improved baseline performance, and worse drug-influenced performance on pre-frontal cortex based tasks, however, in stroke patients not given drugs, better motor performance was seen in patients with the COMT Val/Val or Val/Met genotypes (Mattay et al., 2003; Liepert et al., 2013; Kim et al., 2016). Levels of COMT are higher in the prefrontal cortex, and lower in the striatum, while the opposite is true of DAT (Mattay et al., 2003). As a result, higher prefrontal DA levels may lead to increased prefrontal cortex inhibition of striatal activity, without concomitant increases in striatal DA, so in motor learning based tasks, which require striatal and motor cortical activity, the Met allele of the COMT gene may not be beneficial. Adding to the complexity of considering whole-network ramifications of the genes governing the dopaminergic system, there is evidence that polymorphisms in the BDNF gene may interact with polymorphisms for DA-linked genes and affect plasticity processes (Witte et al., 2012). A great deal of work remains to be done on the pharmacogenetics of stroke recovery, particularly as it pertains to the dopaminergic system.
Spectrum of Actions of DA-Enhancing Drugs
Studies thus far have mostly used drugs that act upon a wide variety of targets. By virtue of a modulation of the DAT and NE transporter function, AMPH and MPH can potentially activate numerous adrenergic (α1AR, α2AR, β1AR, β2AR) and dopaminergic (D1R, D2R, D3R, D4R, and D5R) receptor subtypes. Another confounding factor with respect to the usage of these drugs is the potential recruitment of the trace amine-associated receptor 1 (TAAR1), a Gs-linked GPCR widely expressed in the central nervous system (CNS). Notably, TAAR1, which chiefly responds to endogenous trace amines (β-phenylethylamine, tyramine, and octopamine), can also bind to and be activated by DA, NE and AMPH-like compounds (Pei et al., 2016). Likewise, administration of L-DOPA, the precursor to DA and NE, possibly leads to a non-specific activation of a large number of CNS dopaminergic and adrenergic receptors. Consequently, the potential recruitment of a large spectrum of molecular targets by these drugs may ultimately mitigate their therapeutic effects. For instance, D1 and D2-class dopaminergic receptors act in opposing directions in their classical pathways. Previous pharmacological manipulations of the dopaminergic system, for example the use of D2-class receptor antagonists in the treatment of psychosis, have shown that drugs preferentially targeting a given receptor or receptor-class are often more efficacious to treat symptoms without causing unwanted side effects (Beaulieu et al., 2015). It is likely that the best dopaminergic facilitation of stroke recovery comes from some optimal balance between D1 and D2-class receptor activation, and it is possible that a non-specific increase in DA receptor stimulation may not allow reaching that optimal balance. Several of the likely mechanisms of action for a DA-driven facilitation of stroke recovery could be particularly associated with D1-class dopaminergic receptors, including the modulation of motor learning, augmentation of BDNF and GDNF levels and increase in cerebral blood flow and neurovascular coupling (Luft et al., 2004; Choi et al., 2006; Nitsche et al., 2009; Tan, 2009; Perreault et al., 2012; Xing et al., 2012; Kuric et al., 2013). Specific activation of D1-class receptors would be unlikely to have great effects on endogenous DA levels, as feedback inhibition is the domain of D2-class receptors, particularly presynaptic D2R (Boyar and Altar, 1987; Beaulieu et al., 2015). Conversely, D2-class receptors seem to be linked to modulation of immune responses (Huck et al., 2015; Zhang et al., 2015; Qiu et al., 2016), LTP/LTD in concert with D1-class receptors (Calabresi et al., 2007; Shen et al., 2008; Molina-Luna et al., 2009) and it has been suggested that these receptors can also modulate relevant growth factors, BDNF (Takeuchi et al., 2002; Ahmadiantehrani and Ron, 2013; Rioult-Pedotti et al., 2015; Adachi et al., 2018), GNDF (Bozzi and Borrelli, 1999; Ohta et al., 2010) and FGF-2 (Roceri et al., 2001; Fumagalli et al., 2003, 2006b; Li et al., 2006; Mueller et al., 2006). Notwithstanding these findings, the intricate role of biogenic amines in the facilitation of poststroke recovery, notably that of DA and its receptors, remains to be experimentally tested in a more systematic fashion. This is likely to improve our understanding of the mechanistic underpinnings that underlie stroke recovery, which in turn may lead to better treatment in the future.
Potential Complications to DA-Enhancing Therapies in Stroke Patients
As mentioned previously, following stroke there is a massive release of DA, which is thought to be a source of excitotoxicity, and to exacerbate cell damage. As such, it is likely that dopaminergic therapies should be avoided in the acute stages of stroke and are best applied once the stroke has stabilized (Goldstein, 1998). As alluded above, to improve stroke recovery these therapies may be best in the context of personalized medicine, as patients with the wrong genotype or stroke locations may not benefit from these therapies. Another group of patients who may be harmed from a dopaminergic therapy are the rare individuals who develop poststroke movement disorders and may not respond well to DA-enhancing therapy (Handley et al., 2009; Siniscalchi et al., 2012; Ruppert et al., 2017).
Additionally, administration of AMPH and the related methamphetamine can lead to degeneration of DA cells and terminals in a variety of rodent brain regions including the striatum and the olfactory bulb (Deng et al., 2007; Atianjoh et al., 2008). In fact, use of high doses of AMPH and derivatives has been shown to lead to neurotoxicity in rodent models, culminating in a decrease in striatal DA, which can last for years after the cessation of the drug (Berman et al., 2008, 2009; Yamamoto et al., 2010). Interestingly, nonhuman primates administered with lower and more clinically relevant AMPH doses display the neurotoxicity hallmarks seen in rodents given high doses, suggesting humans may be more susceptible to this phenomenon than rodents (Ricaurte et al., 2005; Berman et al., 2009). Further, it has been suggested that elderly individuals may be more vulnerable to this effect, which perhaps emphasizes the non-ideal nature of AMPH treatment, should other agents prove suitable for stroke recovery (Berman et al., 2009). However, the clinical relevance of this potential neurodegeneration induced by AMPH derivatives in the context of poststroke recovery has yet to be confirmed as per the dose regimens (AMPH) and type of drugs used (therapeutic usefulness of methamphetamine, if any, is probably limited). In the following sub-sections we discuss the more potentially clinically relevant unwanted effects of drugs utilized in the context of improving poststroke recovery. The reader should bear in mind that most dopaminergic drugs referred herein have been also used to treat some neuropsychiatric disorders (e.g., PD, ADHD). Importantly, whether some of undesirable effects (e.g., impulse-control disorders) reported for instance in PD (Weintraub et al., 2010; Mestre et al., 2013; Maloney et al., 2017; Voon et al., 2017) could also be observed with “sub-chronic” time course employed in clinical stroke recovery studies (see Table 2 for drug scheduling used with approved dosage by US Food Drug Administration), is unclear.
AMPH and MPH
The use of AMPH has its own particular risks, most notably cardiovascular side effects, nervousness, insomnia, and loss of appetite, and is not considered an ideal therapy for these reasons (Martinsson and Wahlgren, 2003; Floel and Cohen, 2010; Engelter, 2013). Likewise, although MPH is typically well tolerated, common side effects include hypertension, insomnia and anorexia. Meta-analysis of the clinical stroke data indicates a trend toward increased risk of death in AMPH-treated patients (Martinsson et al., 2007; Sprigg and Bath, 2009). However, no single study reported an increased risk of death, nor any significant adverse effects attributed to AMPH (Martinsson et al., 2007). Several studies did show increases in heart rate and hypertension under the effects of the drug. Considering that these trials used careful screening to avoid treating patients with any additional cardiovascular risk factors, this may mean that AMPH treatment is likely to be unsafe in the broader population of stroke patients (Martinsson et al., 2007; Sprigg and Bath, 2009; Engelter et al., 2010). While studies using MPH after stroke have not shown increased risk of death or side effects under the drug, few trials have been done. MPH studies have had very low ratios of enrollment: screening, indicating strict screening measures are being employed, and again suggesting a limited applicability notably due to cardiovascular risks in older poststroke patients (Engelter, 2013). In support of higher incidence of cardiovascular risks in stroke patients, a recent histological study reported heart and kidney damages in adolescent rats receiving 2 daily administration of the equivalent clinical dose of MPH for 7 days (Loureiro-Vieira et al., 2018).
L-DOPA
Side effects of L-DOPA are generally milder. L-DOPA is typically given with dopa-decarboxylase inhibitors to maximize the amount of L-DOPA available in the brain, which may also help to prevent the development of side effects in the peripheral system (Barbeau et al., 1972; Politi et al., 2018). The most common side effect of L-DOPA treatment is nausea, which can be treated with domperidone (Restemeyer et al., 2007; Engelter, 2013). While L-DOPA treatment for Parkinson's disease can lead to dyskinesia, this complication appears to be an interaction with the parkinsonian state, and there has been no reports of dyskinesia developing in stroke patients, even with up to 7 weeks administration, suggesting that dyskinesia is not likely to occur in stroke sufferers unless they also have a Parkinson's disease-like condition (Kakuda et al., 2011). An observational study of the use of drugs for pharmacological augmentation (PA) of stroke recovery found that in one Swiss stroke rehab ward, 39% of patients were being given drugs for PA, of those 65% received L-DOPA. These patients taking PA agents had only mild and transient side effects and the use of PA agents was associated with a larger change in FIM score (Engelter et al., 2010). The fact that L-DOPA is being given quite frequently off label to stroke patients should further spur on studies in this area to confirm efficacy and safety. Overall L-DOPA appears to be relatively safe, with side effects being mild and transient.
Ropinirole
Tolerability of ropinirole is less clear. Although it was seemingly safe in the Cramer et al. (2009) study reported above, it did lead to higher levels of side-effects (dizziness, sleepiness, fatigue), and patients were not able to reach the planned dose for the study. Additionally, medication effects overcame the blinding (Cramer et al., 2009).
Drug Abuse and Impulse-Control Disorders (ICDs)
In studies using ropinirole, L-DOPA or amphetamine derivatives, there has not been any specific observation of DA dysregulation syndrome (a form of ICD) in patients taking dopaminergic drugs during stroke recovery. Meanwhile, some studies have reported that some impulse control disorders (e.g., compulsive shopping or gambling) are possible in non-PD patients (e.g., restless leg syndrome, fibromyalgia, prolactinomas) taking DA agonists (Mestre et al., 2013; Maloney et al., 2017). However, as far as we know, there are no reports of ICDs in non-PD patients taking L-DOPA. With regards to the use of AMPH derivatives, there are no comprehensive studies published specifically about the prevalence of drug abuse or ICDs among patients prescribed with AMPH derivatives to treat neuropsychiatric disorders such as ADHD, for instance. Yet, addiction may be a particular concern, as some evidence suggests that following stroke, there may be an increased vulnerability to addiction, although it is unclear if this is caused by the stroke itself or by an interaction of stroke and prior experience with addictive substances (Huang et al., 2017). Abuse of stimulants is fairly common with non-ADHD adolescents and while rare, there is a risk with ADHD adolescents (Jaffe, 2002). MPH has also been abused, and may be addictive, particularly when administration strays from the prescribed routes and dosages (Challman and Lipsky, 2000; Morton and Stockton, 2000). In adults treated for ADHD (>25-year old), co-medication with other CNS drugs is frequent, and hence will likely confound, if any, the prevalence of drug abuse (Pauly et al., 2018). The incidence of unwanted effects (e.g., drug abuse) with the use of DA-enhancing drugs will also likely depend on demographic, premorbid personality type (e.g., impaired executive function) and/or genetic/epigenetic risk factors (Mestre et al., 2013; Maloney et al., 2017; Voon et al., 2017). Importantly, as discussed in section Genetic Polymorphisms, genetic risk factors associated with DA-related genes have been implicated in the extent of dopaminergic stimulation.
Conclusions
The interplay of stroke and the dopaminergic system is deeply complex. Strokes can exert significant effects on the DA system, which may require appropriate treatment. An understanding of these effects can set the stage for a better understanding of the effects of dopaminergic treatment following stroke. Results in humans have been mixed but, given to the responsive patient populations, this may be a powerful adjunct to physical therapy. Although beyond the scope of this review, there is also evidence that DA-based pharmacotherapy may be beneficial in aphasia (Gill and Leff, 2012), hemi-spatial neglect (van der Kemp et al., 2017), and poststroke depression (Kohno et al., 2010; Delbari et al., 2011; Adam et al., 2013; Spiegel and Chatterjee, 2014; Stanfill et al., 2016). Meanwhile, like the findings for DA-based pharmacotherapies in motor recovery, results in these areas are quite mixed. The current body of literature provides hope for the efficacy of DA-enhancing drugs for motor recovery from stroke. Studies performed using animal stroke models and in clinical stroke patients seem to suggest an approach pairing multiple administrations of DA-enhancing therapies and intensive physical rehabilitation is the most efficacious. This should be seen as the jumping off point for further studies, at both the preclinical and clinical levels, to optimize issues such as timing and the most responsive patient populations, which will maximize the chances of successful translation. We have identified several possible mediators of this DA system driven effect, including modulation of long-term potentiation and depression, motor map reorganization, growth factors such as BDNF, GDNF, and FGF-2, increased cortical sprouting and interactions with astrocytes and glial cells. Understanding of the underlying mechanisms will allow the field to better choose and design pharmaceutical treatments. Indeed, specific targeting of receptor subtypes may allow for better outcomes, by allowing for manipulation of the most powerful recovery mechanisms, while avoiding undesirable side-effects. More work remains to be done at both the basic science level, to illuminate mechanisms and clarify best practices, and at the clinical level, to solidify the best interpretation of the results in human patients.
Author Contributions
AG and MT contributed to the conception, writing and editing of the manuscript. AG and MT approved the manuscript for publication. AG designed the figure and tables.
Conflict of Interest Statement
The authors declare that the research was conducted in the absence of any commercial or financial relationships that could be construed as a potential conflict of interest.
Acknowledgments
Research in the Tiberi laboratory is supported by grants from the Canadian Partnership for Stroke Recovery (CPSR), Canadian Institutes of Health Research (FRN-125878 and FRN-153320) and the Natural Sciences and Engineering Research Council of Canada (RGPIN-2017-05019). AG is the recipient of scholarships from CPSR and Ontario Graduate Scholarship Program.
References
Abe, K., Kashiwagi, Y., Tokumura, M., Hosoi, R., Hatazawa, J., and Inoue, O. (2004). Discrepancy between cell injury and benzodiazepine receptor binding after transient middle cerebral artery occlusion in rats. Synapse 53, 234–239. doi: 10.1002/syn.20057
Acler, M., and Manganotti, P. (2013). Role, indications and controversies of levodopa administration in chronic stroke patients. Eur. J. Phys. Rehabil. Med. 49, 243–249.
Acler, M., Fiaschi, A., and Manganotti, P. (2009). Long-term levodopa administration in chronic stroke patients. A clinical and neurophysiologic single-blind placebo-controlled cross-over pilot study. Restor. Neurol. Neurosci 27, 277–283. doi: 10.3233/RNN-2009-0477
Adachi, N., Yoshimura, A., Chiba, S., Ogawa, S., and Kunugi, H. (2018). Rotigotine, a dopamine receptor agonist, increased BDNF protein levels in the rat cortex and hippocampus. Neurosci. Lett. 662, 44–50. doi: 10.1016/j.neulet.2017.10.006
Adam, R., Leff, A., Sinha, N., Turner, C., Bays, P., Draganski, B., et al. (2013). Dopamine reverses reward insensitivity in apathy following globus pallidus lesions. Cortex 49, 1292–1303. doi: 10.1016/j.cortex.2012.04.013
Adkins, D. L., and Jones, T. A. (2005). D-Amphetamine enhances skilled reaching after ischemic cortical lesions in rats. Neurosci. Lett. 380, 214–218. doi: 10.1016/j.neulet.2005.01.036
Adkins, D. L., Schallert, T., and Goldstein, L. B. (2009). Poststroke treatment: lost in translation. Stroke 40, 8–9. doi: 10.1161/STROKEAHA.108.534248
Ahagon, A., Ishikawa, M., and Handa, H. (1980). Histochemical changes of brain dopamine in an acute stage of cerebral ischemia in gerbils. Stroke 11, 622–628. doi: 10.1161/01.STR.11.6.622
Ahmadiantehrani, S., and Ron, D. (2013). Dopamine D2 receptor activation leads to an up-regulation of glial cell line-derived neurotrophic factor via Gbetagamma-Erk1/2-dependent induction of Zif268. J. Neurochem. 125, 193–204. doi: 10.1111/jnc.12178
Akiyama, Y., Ito, A., Koshimura, K., Ohue, T., Yamagata, S., Miwa, S., et al. (1991). Effects of transient forebrain ischemia and reperfusion on function of dopaminergic neurons and dopamine reuptake in vivo in rat striatum. Brain Res. 561, 120–127. doi: 10.1016/0006-8993(91)90756-L
Alaverdashvili, M., Lim, D. H., and Whishaw, I. Q. (2007). No improvement by amphetamine on learned non-use, attempts, success or movement in skilled reaching by the rat after motor cortex stroke. Eur. J. Neurosci. 25, 3442–3452. doi: 10.1111/j.1460-9568.2007.05594.x
Amantea, D., and Bagetta, G. (2017). Excitatory and inhibitory amino acid neurotransmitters in stroke: from neurotoxicity to ischemic tolerance. Curr. Opin. Pharmacol. 35, 111–119. doi: 10.1016/j.coph.2017.07.014
Arai, K., Jin, G., Navaratna, D., and Lo, E. H. (2009). Brain angiogenesis in developmental and pathological processes: neurovascular injury and angiogenic recovery after stroke. FEBS J. 276, 4644–4652. doi: 10.1111/j.1742-4658.2009.07176.x
Arnsten, A. F., Wang, M., and Paspalas, C. D. (2015). Dopamine's actions in primate prefrontal cortex: challenges for treating cognitive disorders. Pharmacol. Rev. 67, 681–696. doi: 10.1124/pr.115.010512
Atianjoh, F. E., Ladenheim, B., Krasnova, I. N., and Cadet, J. L. (2008). Amphetamine causes dopamine depletion and cell death in the mouse olfactory bulb. Eur. J. Pharmacol. 589, 94–97. doi: 10.1016/j.ejphar.2008.05.001
Auriat, A. M., and Colbourne, F. (2008). Influence of amphetamine on recovery after intracerebral hemorrhage in rats. Behav. Brain Res. 186, 222–229. doi: 10.1016/j.bbr.2007.08.010
Barbay, S., and Nudo, R. J. (2009). The effects of amphetamine on recovery of function in animal models of cerebral injury: a critical appraisal. Neurorehabilitation 25, 5–17. doi: 10.3233/NRE-2009-0495
Barbay, S., Zoubina, E. V., Dancause, N., Frost, S. B., Eisner-Janowicz, I., Stowe, A. M., et al. (2006). A single injection of D-amphetamine facilitates improvements in motor training following a focal cortical infarct in squirrel monkeys. Neurorehabil. Neural Repair 20, 455–458. doi: 10.1177/1545968306290773
Barbeau, A., Mars, H., Botez, M. I., and Joubert, M. (1972). Levodopa combined with peripheral decarboxylase inhibition in Parkinson's disease. Can. Med. Assoc. J. 106, 1169–1174.
Bath, P. M., Lees, K. R., Schellinger, P. D., Altman, H., Bland, M., Hogg, C., et al. (2012). Statistical analysis of the primary outcome in acute stroke trials. Stroke 43, 1171–1178. doi: 10.1161/STROKEAHA.111.641456
Beaulieu, J. M., and Gainetdinov, R. R. (2011). The physiology, signaling, and pharmacology of dopamine receptors. Pharmacol. Rev. 63, 182–217. doi: 10.1124/pr.110.002642
Beaulieu, J. M., Espinoza, S., and Gainetdinov, R. R. (2015). Dopamine receptors - IUPHAR Review 13. Br. J. Pharmacol. 172, 1–23. doi: 10.1111/bph.12906
Becker, C., Jick, S. S., and Meier, C. R. (2010). Risk of stroke in patients with idiopathic Parkinson disease. Parkinsonism Relat. Disord. 16, 31–35. doi: 10.1016/j.parkreldis.2009.06.005
Belgacem, Y. H., and Borodinsky, L. N. (2017). CREB at the crossroads of activity-dependent regulation of nervous system development and function. Adv. Exp. Med. Biol. 1015, 19–39. doi: 10.1007/978-3-319-62817-2_2
Berman, S. M., Kuczenski, R., McCracken, J. T., and London, E. D. (2009). Potential adverse effects of amphetamine treatment on brain and behavior: a review. Mol. Psychiatry 14, 123–142. doi: 10.1038/mp.2008.90
Berman, S., O'Neill, J., Fears, S., Bartzokis, G., and London, E. D. (2008). Abuse of amphetamines and structural abnormalities in the brain. Ann. N. Y. Acad. Sci. 1141, 195–220. doi: 10.1196/annals.1441.031
Berretta, A., Tzeng, Y. C., and Clarkson, A. N. (2014). Post-stroke recovery: the role of activity-dependent release of brain-derived neurotrophic factor. Expert Rev. Neurother. 14, 1335–1344. doi: 10.1586/14737175.2014.969242
Bhakta, B. B., Hartley, S., Holloway, I., Couzens, J. A., Ford, G. A., Meads, D., et al. (2014). The DARS (Dopamine Augmented Rehabilitation in Stroke) trial: protocol for a randomised controlled trial of Co-careldopa treatment in addition to routine NHS occupational and physical therapy after stroke. Trials 15:316. doi: 10.1186/1745-6215-15-316
Björklund, A., and Dunnett, S. B. (2007). Dopamine neuron systems in the brain: an update. Trends Neurosci. 30, 194–202. doi: 10.1016/j.tins.2007.03.006
Borkar, C. D., Bharne, A. P., Nagalakshmi, B., Sakharkar, A. J., Subhedar, N. K., and Kokare, D. M. (2018). Cocaine- and Amphetamine-Regulated Transcript Peptide (CART) alleviates MK-801-induced schizophrenic dementia-like symptoms. Neuroscience 375, 94–107. doi: 10.1016/j.neuroscience.2018.01.056
Borlongan, C. V., Martinez, R., Shytle, R. D., Freeman, T. B., Cahill, D. W., and Sanberg, P. R. (1995). Striatal dopamine-mediated motor behavior is altered following occlusion of the middle cerebral artery. Pharmacol. Biochem. Behav. 52, 225–229. doi: 10.1016/0091-3057(95)00108-9
Boyar, W. C., and Altar, C. A. (1987). Modulation of in vivo dopamine release by D2 but not D1 receptor agonists and antagonists. J. Neurochem. 48, 824–831. doi: 10.1111/j.1471-4159.1987.tb05591.x
Boyd, L. A., Hayward, K. S., Ward, N. S., Stinear, C. M., Rosso, C., Fisher, R. J., et al. (2017). Biomarkers of stroke recovery: consensus-based core recommendations from the stroke recovery and rehabilitation roundtable. Int. J. Stroke 12, 480–493. doi: 10.1177/1747493017714176
Boyeson, M. G., and Feeney, D. M. (1990). Intraventricular norepinephrine facilitates motor recovery following sensorimotor cortex injury. Pharmacol. Biochem. Behav. 35, 497–501. doi: 10.1016/0091-3057(90)90279-Q
Boyeson, M. G., and Feeney, D. M. (1991). Adverse effects of catecholaminergic drugs following unilateral cerebellar ablations. Restor. Neurol. Neurosci. 3, 227–233.
Bozzi, Y., and Borrelli, E. (1999). Absence of the dopamine D2 receptor leads to a decreased expression of GDNF and NT-4 mRNAs in restricted brain areas. Eur. J. Neurosci. 11, 1275–1284. doi: 10.1046/j.1460-9568.1999.00541.x
Brannan, T., Weinberger, J., Knott, P., Taff, I., Kaufmann, H., Togasaki, D., et al. (1987). Direct evidence of acute, massive striatal dopamine release in gerbils with unilateral strokes. Stroke 18, 108–110. doi: 10.1161/01.STR.18.1.108
Brassai, A., Suvanjeiev, R. G., Ban, E. G., and Lakatos, M. (2015). Role of synaptic and nonsynaptic glutamate receptors in ischaemia induced neurotoxicity. Brain Res. Bull. 112, 1–6. doi: 10.1016/j.brainresbull.2014.12.007
Breitenstein, C., Flöel, A., Korsukewitz, C., Wailke, S., Bushuven, S., and Knecht, S. (2006). A shift of paradigm: from noradrenergic to dopaminergic modulation of learning? J. Neurol. Sci. 248, 42–47. doi: 10.1016/j.jns.2006.05.012
Brown, A. W., Bjelke, B., and Fuxe, K. (2004). Motor response to amphetamine treatment, task-specific training, and limited motor experience in a postacute animal stroke model. Exp. Neurol. 190, 102–108. doi: 10.1016/j.expneurol.2004.07.005
Brunelin, J., Fecteau, S., and Suaud-Chagny, M. F. (2013). Abnormal striatal dopamine transmission in schizophrenia. Curr. Med. Chem. 20, 397–404. doi: 10.2174/0929867311320030011
Buisson, A., Callebert, J., Mathieu, E., Plotkine, M., and Boulu, R. G. (1992). Striatal protection induced by lesioning the substantia nigra of rats subjected to focal ischemia. J. Neurochem. 59, 1153–1157. doi: 10.1111/j.1471-4159.1992.tb08358.x
Calabresi, P., Gubellini, P., Centonze, D., Picconi, B., Bernardi, G., Chergui, K., et al. (2000). Dopamine and cAMP-regulated phosphoprotein 32 kDa controls both striatal long-term depression and long-term potentiation, opposing forms of synaptic plasticity. J. Neurosci. 20, 8443–8451. doi: 10.1523/JNEUROSCI.20-22-08443.2000
Calabresi, P., Picconi, B., Tozzi, A., and Di Filippo, M. (2007). Dopamine-mediated regulation of corticostriatal synaptic plasticity. Trends Neurosci. 30, 211–219. doi: 10.1016/j.tins.2007.03.001
Calabresi, P., Saiardi, A., Pisani, A., Baik, J. H., Centonze, D., Mercuri, N. B., et al. (1997). Abnormal synaptic plasticity in the striatum of mice lacking dopamine D2 receptors. J. Neurosci. 17, 4536–4544. doi: 10.1523/JNEUROSCI.17-12-04536.1997
Caracciolo, L., Marosi, M., Mazzitelli, J., Latifi, S., Sano, Y., Galvan, L., et al. (2018). CREB controls cortical circuit plasticity and functional recovery after stroke. Nat. Commun. 9:2250. doi: 10.1038/s41467-018-04445-9
Carnicella, S., Ahmadiantehrani, S., He, D. Y., Nielsen, C. K., Bartlett, S. E., Janak, P. H., et al. (2009). Cabergoline decreases alcohol drinking and seeking behaviors via glial cell line-derived neurotrophic factor. Biol. Psychiatry 66, 146–153. doi: 10.1016/j.biopsych.2008.12.022
Castro-Alamancos, M. A., and Borrell, J. (1995). Functional recovery of forelimb response capacity after forelimb primary motor cortex damage in the rat is due to the reorganization of adjacent areas of cortex. Neuroscience 68, 793–805. doi: 10.1016/0306-4522(95)00178-L
Castro-Alamancos, M. A., Garcia-Segura, L. M., and Borrell, J. (1992). Transfer of function to a specific area of the cortex after induced recovery from brain damage. Eur. J. Neurosci. 4, 853–863. doi: 10.1111/j.1460-9568.1992.tb00195.x
Cave, J. W., Fujiwara, N., Weibman, A. R., and Baker, H. (2016). Cytoarchitectural changes in the olfactory bulb of Parkinson's disease patients. NPJ Parkinsons Dis. 2:16011. doi: 10.1038/npjparkd.2016.11
Centonze, D., Grande, C., Saulle, E., Martin, A. B., Gubellini, P., Pavon, N., et al. (2003). Distinct roles of D1 and D5 dopamine receptors in motor activity and striatal synaptic plasticity. J. Neurosci. 23, 8506–8512. doi: 10.1523/JNEUROSCI.23-24-08506.2003
Centonze, D., Picconi, B., Gubellini, P., Bernardi, G., and Calabresi, P. (2001). Dopaminergic control of synaptic plasticity in the dorsal striatum. Eur. J. Neurosci. 13, 1071–1077. doi: 10.1046/j.0953-816x.2001.01485.x
Challman, T. D., and Lipsky, J. J. (2000). Methylphenidate: its pharmacology and uses. Mayo Clin. Proc. 75, 711–721. doi: 10.1016/S0025-6196(11)64618-1
Choi, J. K., Chen, Y. I., Hamel, E., and Jenkins, B. G. (2006). Brain hemodynamic changes mediated by dopamine receptors: role of the cerebral microvasculature in dopamine-mediated neurovascular coupling. Neuroimage 30, 700–712. doi: 10.1016/j.neuroimage.2005.10.029
Chollet, F., Cramer, S. C., Stinear, C., Kappelle, L. J., Baron, J. C., Weiller, C., et al. (2014). Pharmacological therapies in post stroke recovery: recommendations for future clinical trials. J. Neurol. 261, 1461–1468. doi: 10.1007/s00415-013-7172-z
Cook, D. J., Nguyen, C., Chun, H. N., Chiu, A. S., Machnicki, M., et al. (2017). Hydrogel-delivered brain-derived neurotrophic factor promotes tissue repair and recovery after stroke. J. Cereb. Blood Flow Metab. 37, 1030–1045. doi: 10.1177/0271678X16649964
Cools, R., and D'Esposito, M. (2011). Inverted-U-shaped dopamine actions on human working memory and cognitive control. Biol. Psychiatry 69, e113–e125. doi: 10.1016/j.biopsych.2011.03.028
Corbett, D., Carmichael, S. T., Murphy, T. H., Jones, T. A., Schwab, M. E., Jolkkonen, J., et al. (2017). Enhancing the alignment of the preclinical and clinical stroke recovery research pipeline: consensus-based core recommendations from the Stroke Recovery and Rehabilitation Roundtable Translational Working Group. Neurorehabil. Neural Repair 31, 699–707. doi: 10.1177/1545968317724285
Cramer, S. C., Dobkin, B. H., Noser, E. A., Rodriguez, R. W., and Enney, L. A. (2009). Randomized, placebo-controlled, double-blind study of ropinirole in chronic stroke. Stroke 40, 3034–3038. doi: 10.1161/STROKEAHA.109.552075
Crisostomo, E. A., Duncan, P. W., Propst, M., Dawson, D. V., and Davis, J. N. (1988). Evidence that amphetamine with physical therapy promotes recovery of motor function in stroke patients. Ann. Neurol. 23, 94–97. doi: 10.1002/ana.410230117
Cvejić, V., Micic, D. V., Djuricic, B. M., Mrsulja, B. J., and Mrsulja, B. B. (1980). Monoamines and related enzymes in cerebral cortex and basal ganglia following transient ischemia in gerbils. Acta Neuropathol. 51, 71–77. doi: 10.1007/BF00688852
Dawson, V. L., Hsu, C. Y., Liu, T. H., Dawson, T. M., and Wamsley, J. K. (1994). Receptor alterations in subcortical structures after bilateral middle cerebral artery infarction of the cerebral cortex. Exp. Neurol. 128, 88–96. doi: 10.1006/exnr.1994.1115
Delanoy, R. L., Tucci, D. L., and Gold, P. E. (1983). Amphetamine effects on long term potentiation in dentate granule cells. Pharmacol. Biochem. Behav. 18, 137–139. doi: 10.1016/0091-3057(83)90263-0
Delbari, A., Salman-Roghani, R., and Lokk, J. (2011). Effect of methylphenidate and/or levodopa combined with physiotherapy on mood and cognition after stroke: a randomized, double-blind, placebo-controlled trial. Eur. Neurol. 66, 7–13. doi: 10.1159/000329275
Delbarre, B., Delbarre, G., and Calinon, F. (1992). Free radicals and neurotransmitters in gerbil brain. Influence of age and ischemia reperfusion insult. EXS 62, 199–212. doi: 10.1007/978-3-0348-7460-1_20
Deng, X., Ladenheim, B., Jayanthi, S., and Cadet, J. L. (2007). Methamphetamine administration causes death of dopaminergic neurons in the mouse olfactory bulb. Biol. Psychiatry 61, 1235–1243. doi: 10.1016/j.biopsych.2006.09.010
Devoto, P., and Flore, G. (2006). On the origin of cortical dopamine: is it a co-transmitter in noradrenergic neurons? Curr. Neuropharmacol. 4, 115–125. doi: 10.2174/157015906776359559
Doty, R. L. (2012). Olfaction in Parkinson's disease and related disorders. Neurobiol. Dis. 46, 527–552. doi: 10.1016/j.nbd.2011.10.026
Du, F., Li, R., Huang, Y., Li, X., and Le, W. (2005). Dopamine D3 receptor-preferring agonists induce neurotrophic effects on mesencephalic dopamine neurons. Eur. J. Neurosci. 22, 2422–2430. doi: 10.1111/j.1460-9568.2005.04438.x
Dudman, J. T., Eaton, M. E., Rajadhyaksha, A., Macias, W., Taher, M., Barczak, A., et al. (2003). Dopamine D1 receptors mediate CREB phosphorylation via phosphorylation of the NMDA receptor at Ser897-NR1. J. Neurochem. 87, 922–934. doi: 10.1046/j.1471-4159.2003.02067.x
Duncan, P. W. (2013). Outcome measures in stroke rehabilitation. Handb. Clin. Neurol. 110, 105–111. doi: 10.1016/B978-0-444-52901-5.00009-5
Durukan, A., and Tatlisumak, T. (2010). Preconditioning-induced ischemic tolerance: a window into endogenous gearing for cerebroprotection. Exp. Transl. Stroke Med. 2, 2. doi: 10.1186/2040-7378-2-2
Engelter, S. T. (2013). Safety in pharmacological enhancement of stroke rehabilitation. Eur. J. Phys. Rehabil. Med. 49, 261–267.
Engelter, S. T., Frank, M., Lyrer, P. A., and Conzelmann, M. (2010). Safety of pharmacological augmentation of stroke rehabilitation. Eur. Neurol. 64, 325–330. doi: 10.1159/000322134
Feeney, D. M., and Hovda, D. A. (1983). Amphetamine and apomorphine restore tactile placing after motor cortex injury in the cat. Psychopharmacology 79, 67–71. doi: 10.1007/BF00433018
Feeney, D. M., Gonzalez, A., and Law, W. A. (1982). Amphetamine, haloperidol, and experience interact to affect rate of recovery after motor cortex injury. Science 217, 855–857. doi: 10.1126/science.7100929
Feeney, D. M., Weisend, M. P., and Kline, A. E. (1993). Noradrenergic pharmacotherapy, intracerebral infusion and adrenal transplantation promote functional recovery after cortical damage. J. Neural Transplant. Plast. 4, 199–213. doi: 10.1155/NP.1993.199
Floel, A., and Cohen, L. G. (2010). Recovery of function in humans: cortical stimulation and pharmacological treatments after stroke. Neurobiol. Dis. 37, 243–251. doi: 10.1016/j.nbd.2009.05.027
Floel, A., Hummel, F., Breitenstein, C., Knecht, S., and Cohen, L. G. (2005). Dopaminergic effects on encoding of a motor memory in chronic stroke. Neurology 65, 472–474. doi: 10.1212/01.wnl.0000172340.56307.5e
Floel, A., Vomhof, P., Lorenzen, A., Roesser, N., Breitenstein, C., and Knecht, S. (2008). Levodopa improves skilled hand functions in the elderly. Eur. J. Neurosci. 27, 1301–1307. doi: 10.1111/j.1460-9568.2008.06079.x
Floresco, S. B. (2013). Prefrontal dopamine and behavioral flexibility: shifting from an “inverted-U” toward a family of functions. Front. Neurosci. 7:62. doi: 10.3389/fnins.2013.00062
Forno, L. S. (1983). Reaction of the substantia nigra to massive basal ganglia infarction. Acta Neuropathol. 62, 96–102.
Fumagalli, F., Bedogni, F., Maragnoli, M. E., Gennarelli, M., Perez, J., Racagni, G., et al. (2003). Dopaminergic D2 receptor activation modulates FGF-2 gene expression in rat prefrontal cortex and hippocampus. J. Neurosci. Res. 74, 74–80. doi: 10.1002/jnr.10733
Fumagalli, F., Pasquale, L., Racagni, G., and Riva, M. A. (2006a). Dynamic regulation of fibroblast growth factor 2 (FGF-2) gene expression in the rat brain following single and repeated cocaine administration. J. Neurochem. 96, 996–1004. doi: 10.1111/j.1471-4159.2005.03627.x
Fumagalli, F., Racagni, G., and Riva, M. A. (2006b). Shedding light into the role of BDNF in the pharmacotherapy of Parkinson's disease. Pharmacogenomics J. 6, 95–104. doi: 10.1038/sj.tpj.6500360
Gershon, A. A., Vishne, T., and Grunhaus, L. (2007). Dopamine D2-like receptors and the antidepressant response. Biol. Psychiatry 61, 145–153. doi: 10.1016/j.biopsych.2006.05.031
Gilbert, D. L., Wang, Z., Sallee, F. R., Ridel, K. R., Merhar, S., Zhang, J., et al. (2006). Dopamine transporter genotype influences the physiological response to medication in ADHD. Brain 129(Pt 8), 2038–2046. doi: 10.1093/brain/awl147
Gill, S. K., and Leff, A. P. (2012). Dopaminergic therapy in aphasia. Aphasiology 28, 155–170. doi: 10.1080/02687038.2013.802286
Gilmour, G., Iversen, S. D., O'Neill, M. F., O'Neill, M. J., Ward, M. A., and Bannerman, D. M. (2005). Amphetamine promotes task-dependent recovery following focal cortical ischaemic lesions in the rat. Behav. Brain Res. 165, 98–109. doi: 10.1016/j.bbr.2005.06.027
Gladstone, D. J., Danells, C. J., Armesto, A., McIlroy, W. E., Staines, W. R., Graham, S. J., et al. (2006). Physiotherapy coupled with dextroamphetamine for rehabilitation after hemiparetic stroke: a randomized, double-blind, placebo-controlled trial. Stroke 37, 179–185. doi: 10.1161/01.STR.0000195169.42447.78
Globus, M. Y., Busto, R., Dietrich, W. D., Martinez, E., Valdes, I., and Ginsberg, M. D. (1988). Effect of ischemia on the in vivo release of striatal dopamine, glutamate, and gamma-aminobutyric acid studied by intracerebral microdialysis. J. Neurochem. 51, 1455–1464. doi: 10.1111/j.1471-4159.1988.tb01111.x
Globus, M. Y., Ginsberg, M. D., Dietrich, W. D., Busto, R., and Scheinberg, P. (1987). Substantia nigra lesion protects against ischemic damage in the striatum. Neurosci. Lett. 80, 251–256. doi: 10.1016/0304-3940(87)90463-0
Goldstein, L. B. (1995). Common drugs may influence motor recovery after stroke. The sygen in acute stroke study investigators. Neurology 45, 865–871. doi: 10.1212/WNL.45.5.865
Goldstein, L. B. (1998). Potential effects of common drugs on stroke recovery. Arch. Neurol. 55, 454–456. doi: 10.1001/archneur.55.4.454
Goldstein, L. B. (2009). Amphetamine trials and tribulations. Stroke 40, S133–135. doi: 10.1161/STROKEAHA.108.533703
Goldstein, L. B., and Davis, J. N. (1990a). Beam-walking in rats: studies towards developing an animal model of functional recovery after brain injury. J. Neurosci. Methods 31, 101–107. doi: 10.1016/0165-0270(90)90154-8
Goldstein, L. B., and Davis, J. N. (1990b). Influence of lesion size and location on amphetamine-facilitated recovery of beam-walking in rats. Behav. Neurosci. 104, 320–327. doi: 10.1037/0735-7044.104.2.320
Goldstein, L. B., and Davis, J. N. (1990c). Post-lesion practice and amphetamine-facilitated recovery of beam-walking in the rat. Restor. Neurol. Neurosci. 1, 311–314.
Grade, C., Redford, B., Chrostowski, J., Toussaint, L., and Blackwell, B. (1998). Methylphenidate in early poststroke recovery: a double-blind, placebo-controlled study. Arch. Phys. Med. Rehabil. 79, 1047–1050. doi: 10.1016/S0003-9993(98)90169-1
Grattan, D. R. (2015). 60 YEARS OF NEUROENDOCRINOLOGY: The hypothalamo-prolactin axis. J. Endocrinol. 226, T101–122. doi: 10.1530/JOE-15-0213
Gurden, H., Takita, M., and Jay, T. M. (2000). Essential role of D1 but not D2 receptors in the NMDA receptor-dependent long-term potentiation at hippocampal-prefrontal cortex synapses in vivo. J. Neurosci. 20:RC106. doi: 10.1523/JNEUROSCI.20-22-j0003.2000
Gurden, H., Tassin, J. P., and Jay, T. M. (1999). Integrity of the mesocortical dopaminergic system is necessary for complete expression of in vivo hippocampal-prefrontal cortex long-term potentiation. Neuroscience 94, 1019–1027. doi: 10.1016/S0306-4522(99)00395-4
Hallett, M. (2001). Plasticity of the human motor cortex and recovery from stroke. Brain Res. Brain Res. Rev. 36, 169–174. doi: 10.1016/S0165-0173(01)00092-3
Hama, S., Murakami, T., Yamashita, H., Onoda, K., Yamawaki, S., and Kurisu, K. (2017). Neuroanatomic pathways associated with monoaminergic dysregulation after stroke. Int. J. Geriatr. Psychiatry 32, 633–642. doi: 10.1002/gps.4503
Handley, A., Medcalf, P., Hellier, K., and Dutta, D. (2009). Movement disorders after stroke. Age Ageing 38, 260–266. doi: 10.1093/ageing/afp020
Harrison, M. J., Marsden, C. D., and Jenner, P. (1979). Effect of experimental ischemia on neurotransmitter amines in the gerbil brain. Stroke 10, 165–168. doi: 10.1161/01.STR.10.2.165
Hasbi, A., Fan, T., Alijaniaram, M., Nguyen, T., Perreault, M. L., O'Dowd, B. F., et al. (2009). Calcium signaling cascade links dopamine D1-D2 receptor heteromer to striatal BDNF production and neuronal growth. Proc. Natl. Acad. Sci. U.S.A. 106, 21377–21382. doi: 10.1073/pnas.0903676106
Hashimoto, N., Matsumoto, T., Mabe, H., Hashitani, T., and Nishino, H. (1994). Dopamine has inhibitory and accelerating effects on ischemia-induced neuronal cell damage in the rat striatum. Brain Res. Bull. 33, 281–288. doi: 10.1016/0361-9230(94)90195-3
He, X., Wang, D., Zhang, X., Li, A., Gu, X., Ding, F., et al. (2008). Prolonged modulation of FGF-2 expression in astrocytic cultures induced by O,O'-diacetyl-apomorphine. Biochem. Biophys. Res. Commun. 369, 824–829. doi: 10.1016/j.bbrc.2008.02.082
Her, Y., Yoo, K. Y., Hwang, I. K., Lee, J. S., Kang, T. C., Lee, B. H., et al. (2007). N-methyl-D-aspartate receptor type 1 immunoreactivity and protein level in the gerbil main olfactory bulb after transient forebrain ischemia. Neurochem. Res. 32, 125–131. doi: 10.1007/s11064-006-9237-1
Hosp, J. A., Pekanovic, A., Rioult-Pedotti, M. S., and Luft, A. R. (2011). Dopaminergic projections from midbrain to primary motor cortex mediate motor skill learning. J. Neurosci. 31, 2481–2487. doi: 10.1523/JNEUROSCI.5411-10.2011
Hotte, M., Naudon, L., and Jay, T. M. (2005). Modulation of recognition and temporal order memory retrieval by dopamine D1 receptor in rats. Neurobiol. Learn. Mem. 84, 85–92. doi: 10.1016/j.nlm.2005.04.002
Hotte, M., Thuault, S., Lachaise, F., Dineley, K. T., Hemmings, H. C., Nairn, A. C., et al. (2006). D1 receptor modulation of memory retrieval performance is associated with changes in pCREB and pDARPP-32 in rat prefrontal cortex. Behav. Brain Res. 171, 127–133. doi: 10.1016/j.bbr.2006.03.026
Hovda, D. A., and Feeney, D. M. (1984). Amphetamine with experience promotes recovery of locomotor function after unilateral frontal cortex injury in the cat. Brain Res. 298, 358–361. doi: 10.1016/0006-8993(84)91437-9
Hovda, D. A., Sutton, R. L., and Feeney, D. M. (1987). Recovery of tactile placing after visual cortex ablation in cat: a behavioral and metabolic study of diaschisis. Exp. Neurol. 97, 391–402. doi: 10.1016/0014-4886(87)90099-9
Hu, Z., Oh, E. H., Chung, Y. B., Hong, J. T., and Oh, K. W. (2015). Predominant D1 receptors involvement in the over-expression of CART peptides after repeated cocaine administration. Korean J. Physiol. Pharmacol. 19, 89–97. doi: 10.4196/kjpp.2015.19.2.89
Huang, C. C. Y., Ma, T., Roltsch Hellard, E. A., Wang, X., Selvamani, A., Lu, J., et al. (2017). Stroke triggers nigrostriatal plasticity and increases alcohol consumption in rats. Sci. Rep. 7:2501. doi: 10.1038/s41598-017-02714-z
Huang, Y. Y., Simpson, E., Kellendonk, C., and Kandel, E. R. (2004). Genetic evidence for the bidirectional modulation of synaptic plasticity in the prefrontal cortex by D1 receptors. Proc. Natl. Acad. Sci. U.S.A. 101, 3236–3241. doi: 10.1073/pnas.0308280101
Hubert, G. W., Jones, D. C., Moffett, M. C., Rogge, G., and Kuhar, M. J. (2008). CART peptides as modulators of dopamine and psychostimulants and interactions with the mesolimbic dopaminergic system. Biochem. Pharmacol. 75, 57–62. doi: 10.1016/j.bcp.2007.07.028
Huck, J. H., Freyer, D., Bottcher, C., Mladinov, M., Muselmann-Genschow, C., Thielke, M., et al. (2015). De novo expression of dopamine D2 receptors on microglia after stroke. J. Cereb. Blood Flow Metab. 35, 1804–1811. doi: 10.1038/jcbfm.2015.128
Hyman, S. E., Cole, R. L., Konradi, C., and Kosofsky, B. E. (1995). Dopamine regulation of transcription factor-target interactions in rat striatum. Chem. Senses 20, 257–260.
Ishihara, N., Welch, K. M., Meyer, J. S., Chabi, E., Naritomi, H., Wang, T. P., et al. (1979). Influence of cerebral embolism on brain monoamines. J. Neurol. Neurosurg. Psychiatr. 42, 847–853. doi: 10.1136/jnnp.42.9.847
Issa, R., AlQteishat, A., Mitsios, N., Saka, M., Krupinski, J., Tarkowski, E., et al. (2005). Expression of basic fibroblast growth factor mRNA and protein in the human brain following ischaemic stroke. Angiogenesis 8, 53–62. doi: 10.1007/s10456-005-5613-8
Iwakura, Y., Nawa, H., Sora, I., and Chao, M. V. (2008). Dopamine D1 receptor-induced signaling through TrkB receptors in striatal neurons. J. Biol. Chem. 283, 15799–15806. doi: 10.1074/jbc.M801553200
Jaffe, S. L. (2002). Failed attempts at intranasal abuse of Concerta. J. Am. Acad. Child Adolesc. Psychiatry 41:5. doi: 10.1097/00004583-200201000-00003
Jang, S. H. (2013). Motor function-related maladaptive plasticity in stroke: a review. Neurorehabilitation 32, 311–316. doi: 10.3233/NRE-130849
Jia, J., Chen, X., Zhu, W., Luo, Y., Hua, Z., and Xu, Y. (2008). CART protects brain from damage through ERK activation in ischemic stroke. Neuropeptides 42, 653–661. doi: 10.1016/j.npep.2008.05.006
Kakuda, W., Abo, M., Kobayashi, K., Momosaki, R., Yokoi, A., Fukuda, A., et al. (2011). Combination treatment of low-frequency rTMS and occupational therapy with levodopa administration: an intensive neurorehabilitative approach for upper limb hemiparesis after stroke. Int. J. Neurosci. 121, 373–378. doi: 10.3109/00207454.2011.560314
Kawano, T., Tsutsumi, K., Miyake, H., and Mori, K. (1988). Striatal dopamine in acute cerebral ischemia of stroke-resistant rats. Stroke 19, 1540–1543. doi: 10.1161/01.STR.19.12.1540
Kempadoo, K. A., Mosharov, E. V., Choi, S. J., Sulzer, D., and Kandel, E. R. (2016). Dopamine release from the locus coeruleus to the dorsal hippocampus promotes spatial learning and memory. Proc. Natl. Acad. Sci. U.S.A. 113, 14835–14840. doi: 10.1073/pnas.1616515114
Kesar, T. M., Belagaje, S. R., Pergami, P., Haut, M. W., Hobbs, G., and Buetefisch, C. M. (2017). Effects of monoaminergic drugs on training-induced motor cortex plasticity in older adults. Brain Res. 1670, 106–117. doi: 10.1016/j.brainres.2017.06.015
Kim, B. R., Kim, H. Y., Chun, Y. I., Yun, Y. M., Kim, H., Choi, D. H., et al. (2016). Association between genetic variation in the dopamine system and motor recovery after stroke. Restor. Neurol. Neurosci. 34, 925–934. doi: 10.3233/RNN-160667
Kinoshita, T., Moritani, T., Shrier, D. A., Wang, H. Z., Hiwatashi, A., Numaguchi, Y., et al. (2002). Secondary degeneration of the substantia nigra and corticospinal tract after hemorrhagic middle cerebral artery infarction: diffusion-weighted MR findings. Magn. Reson. Med. Sci. 1, 175–178. doi: 10.2463/mrms.1.175
Kobayashi, T., Ahlenius, H., Thored, P., Kobayashi, R., Kokaia, Z., and Lindvall, O. (2006). Intracerebral infusion of glial cell line-derived neurotrophic factor promotes striatal neurogenesis after stroke in adult rats. Stroke 37, 2361–2367. doi: 10.1161/01.STR.0000236025.44089.e1
Kogure, K., Scheinberg, P., Matsumoto, A., Busto, R., and Reinmuth, O. M. (1975). Catecholamines in experimental brain ischemia. Arch. Neurol. 32, 21–24. doi: 10.1001/archneur.1975.00490430043005
Koh, U. S., Hwang, I. K., Lee, J. C., Lee, H. Y., Seong, N. S., Chung, H. G., et al. (2004). Histochemical study on neurodegeneration in the olfactory bulb after transient forebrain ischaemia in the Mongolian gerbil. Anat. Histol. Embryol. 33, 208–211. doi: 10.1111/j.1439-0264.2004.00538.x
Kohno, N., Abe, S., Toyoda, G., Oguro, H., Bokura, H., and Yamaguchi, S. (2010). Successful treatment of post-stroke apathy by the dopamine receptor agonist ropinirole. J. Clin. Neurosci. 17, 804–806. doi: 10.1016/j.jocn.2009.09.043
Korczyn, A. D. (2015). Vascular parkinsonism–characteristics, pathogenesis and treatment. Nat. Rev. Neurol. 11, 319–326. doi: 10.1038/nrneurol.2015.61
Kratz, O., Diruf, M. S., Studer, P., Gierow, W., Buchmann, J., Moll, G. H., et al. (2009). Effects of methylphenidate on motor system excitability in a response inhibition task. Behav. Brain Funct. 5, 12. doi: 10.1186/1744-9081-5-12
Krimer, L. S., Muly, E. C. III., Williams, G. V., and Goldman-Rakic, P. S. (1998). Dopaminergic regulation of cerebral cortical microcirculation. Nat. Neurosci. 1, 286–289. doi: 10.1038/1099
Kronenberg, G., Balkaya, M., Prinz, V., Gertz, K., Ji, S., Kirste, I., et al. (2012). Exofocal dopaminergic degeneration as antidepressant target in mouse model of poststroke depression. Biol. Psychiatry 72, 273–281. doi: 10.1016/j.biopsych.2012.02.026
Kubrusly, R. C., Ventura, A. L., de Melo Reis, R. A., Serra, G. C., Yamasaki, E. N., Gardino, P. F., et al. (2007). Norepinephrine acts as D1-dopaminergic agonist in the embryonic avian retina: late expression of beta1-adrenergic receptor shifts norepinephrine specificity in the adult tissue. Neurochem. Int. 50, 211–218. doi: 10.1016/j.neuint.2006.08.004
Kuczenski, R., and Segal, D. S. (1997). Effects of methylphenidate on extracellular dopamine, serotonin, and norepinephrine: comparison with amphetamine. J. Neurochem. 68, 2032–2037. doi: 10.1046/j.1471-4159.1997.68052032.x
Küppers, E., and Beyer, C. (2001). Dopamine regulates brain-derived neurotrophic factor (BDNF) expression in cultured embryonic mouse striatal cells. Neuroreport 12, 1175–1179. doi: 10.1097/00001756-200105080-00025
Kuric, E., and Ruscher, K. (2014a). Dynamics of major histocompatibility complex class II-positive cells in the postischemic brain–influence of levodopa treatment. J. Neuroinflammation 11, 145. doi: 10.1186/s12974-014-0145-z
Kuric, E., and Ruscher, K. (2014b). Reduction of rat brain CD8+ T-cells by levodopa/benserazide treatment after experimental stroke. Eur. J. Neurosci. 40, 2463–2470. doi: 10.1111/ejn.12598
Kuric, E., and Ruscher, K. (2014c). Reversal of stroke induced lymphocytopenia by levodopa/benserazide treatment. J. Neuroimmunol. 269, 94–97. doi: 10.1016/j.jneuroim.2014.02.009
Kuric, E., Wieloch, T., and Ruscher, K. (2013). Dopamine receptor activation increases glial cell line-derived neurotrophic factor in experimental stroke. Exp. Neurol. 247, 202–208. doi: 10.1016/j.expneurol.2013.04.016
Kurozumi, K., Nakamura, K., Tamiya, T., Kawano, Y., Kobune, M., Hirai, S., et al. (2004). BDNF gene-modified mesenchymal stem cells promote functional recovery and reduce infarct size in the rat middle cerebral artery occlusion model. Mol. Ther. 9, 189–197. doi: 10.1016/j.ymthe.2003.10.012
Langhorne, P., Coupar, F., and Pollock, A. (2009). Motor recovery after stroke: a systematic review. Lancet Neurol. 8, 741–754. doi: 10.1016/S1474-4422(09)70150-4
Lemon, N., and Manahan-Vaughan, D. (2006). Dopamine D1/D5 receptors gate the acquisition of novel information through hippocampal long-term potentiation and long-term depression. J. Neurosci. 26, 7723–7729. doi: 10.1523/JNEUROSCI.1454-06.2006
Li, A., Guo, H., Luo, X., Sheng, J., Yang, S., Yin, Y., et al. (2006). Apomorphine-induced activation of dopamine receptors modulates FGF-2 expression in astrocytic cultures and promotes survival of dopaminergic neurons. FASEB J. 20, 1263–1265. doi: 10.1096/fj.05-5510fje
Liepert, J., Heller, A., Behnisch, G., and Schoenfeld, A. (2013). Catechol-O-methyltransferase polymorphism influences outcome after ischemic stroke: a prospective double-blind study. Neurorehabil. Neural Repair 27, 491–496. doi: 10.1177/1545968313481282
Lin, D. A., and Finklestein, S. P. (1997). REVIEW: basic fibroblast growth factor: a Treatment for stroke? Neuroscientist 3, 247–250. doi: 10.1177/107385849700300412
Liu, H. S., Shen, H., Harvey, B. K., Castillo, P., Lu, H., Yang, Y., et al. (2011). Post-treatment with amphetamine enhances reinnervation of the ipsilateral side cortex in stroke rats. Neuroimage 56, 280–289. doi: 10.1016/j.neuroimage.2011.02.049
Liu, H. S., Shen, H., Luo, Y., Hoffer, B. J., Wang, Y., and Yang, Y. (2016). Post-treatment with cocaine- and amphetamine-regulated transcript enhances infarct resolution, reinnervation, and angiogenesis in stroke rats - an MRI study. NMR Biomed. 29, 361–370. doi: 10.1002/nbm.3461
Liu, J., Wang, Y., Akamatsu, Y., Lee, C. C., Stetler, R. A., Lawton, M. T., et al. (2014). Vascular remodeling after ischemic stroke: mechanisms and therapeutic potentials. Prog. Neurobiol. 115, 138–156. doi: 10.1016/j.pneurobio.2013.11.004
Livingston-Thomas, J., Nelson, P., Karthikeyan, S., Antonescu, S., Jeffers, M. S., Marzolini, S., et al. (2016). Exercise and environmental enrichment as enablers of task-specific neuroplasticity and stroke recovery. Neurotherapeutics 13, 395–402. doi: 10.1007/s13311-016-0423-9
Lokk, J., Salman Roghani, R., and Delbari, A. (2011). Effect of methylphenidate and/or levodopa coupled with physiotherapy on functional and motor recovery after stroke–a randomized, double-blind, placebo-controlled trial. Acta Neurol. Scand. 123, 266–273. doi: 10.1111/j.1600-0404.2010.01395.x
Long, D., and Young, J. (2003). Dexamphetamine treatment in stroke. QJM 96, 673–685. doi: 10.1093/qjmed/hcg113
Loureiro-Vieira, S., Costa, V. M., Duarte, J. A., Duarte-Araujo, M., Goncalves-Monteiro, S., Maria de Lourdes, B., et al. (2018). Methylphenidate clinically oral doses improved brain and heart glutathione redox status and evoked renal and cardiac tissue injury in rats. Biomed. Pharmacother. 100, 551–563. doi: 10.1016/j.biopha.2018.02.017
Luft, A. R., Buitrago, M. M., Ringer, T., Dichgans, J., and Schulz, J. B. (2004). Motor skill learning depends on protein synthesis in motor cortex after training. J. Neurosci. 24, 6515–6520. doi: 10.1523/JNEUROSCI.1034-04.2004
Luo, Y., Shen, H., Liu, H. S., Yu, S. J., Reiner, D. J., Harvey, B. K., et al. (2013). CART peptide induces neuroregeneration in stroke rats. J. Cereb. Blood Flow Metab. 33, 300–310. doi: 10.1038/jcbfm.2012.172
MacDonald, H. J., Stinear, C. M., Ren, A., Coxon, J. P., Kao, J., Macdonald, L., et al. (2016). Dopamine gene profiling to predict impulse control and effects of dopamine agonist ropinirole. J. Cogn. Neurosci. 28, 909–919. doi: 10.1162/jocn_a_00946
Maling, H. M., and Acheson, G. H. (1946). Righting and other postural activity in low-decerebrate and in spinal cats after d-amphetamine. J. Neurophysiol. 9, 379–386. doi: 10.1152/jn.1946.9.5.379
Maloney, E. M., Djamshidian, A., and O'Sullivan, S. S. (2017). Phenomenology and epidemiology of impulsive-compulsive behaviours in Parkinson's disease, atypical Parkinsonian disorders and non-Parkinsonian populations. J. Neurol. Sci. 374, 47–52. doi: 10.1016/j.jns.2016.12.058
Mang, C. S., Campbell, K. L., Ross, C. J., and Boyd, L. A. (2013). Promoting neuroplasticity for motor rehabilitation after stroke: considering the effects of aerobic exercise and genetic variation on brain-derived neurotrophic factor. Phys. Ther. 93, 1707–1716. doi: 10.2522/ptj.20130053
Martín, A., Gomez-Vallejo, V., San Sebastian, E., Padro, D., Markuerkiaga, I., Llarena, I., et al. (2013). In vivo imaging of dopaminergic neurotransmission after transient focal ischemia in rats. J. Cereb. Blood Flow Metab. 33, 244–252. doi: 10.1038/jcbfm.2012.162
Martinsson, L., and Wahlgren, N. G. (2003). Safety of dexamphetamine in acute ischemic stroke: a randomized, double-blind, controlled dose-escalation trial. Stroke 34, 475–481. doi: 10.1161/01.STR.0000050161.38263.AE
Martinsson, L., Hardemark, H., and Eksborg, S. (2007). Amphetamines for improving recovery after stroke. Cochrane Database Syst. Rev. CD002090. doi: 10.1002/14651858.CD002090.pub2
Masihuzzaman, A. M., Uddin, M. J., Majumder, S., Barman, K. K., and Ullah, M. A. (2011). Effect of low dose levodopa on motor outcome of different types of stroke. Mymensingh Med. J. 20, 689–693.
Mattay, V. S., Goldberg, T. E., Fera, F., Hariri, A. R., Tessitore, A., Egan, M. F., et al. (2003). Catechol O-methyltransferase val158-met genotype and individual variation in the brain response to amphetamine. Proc. Natl. Acad. Sci. U.S.A. 100, 6186–6191. doi: 10.1073/pnas.0931309100
Mazagri, R., Shuaib, A., McPherson, M., and Deighton, M. (1995). Amphetamine failed to improve motor function in acute stroke [abstract]. Can. J. Neurol. Sci. 22:S25.
McEntee, W. J., Mair, R. G., and Langlais, P. J. (1987). Neurochemical specificity of learning: dopamine and motor learning. Yale J. Biol. Med. 60, 187–193.
Meintzschel, F., and Ziemann, U. (2006). Modification of practice-dependent plasticity in human motor cortex by neuromodulators. Cereb. Cortex 16, 1106–1115. doi: 10.1093/cercor/bhj052
Mestre, T. A., Strafella, A. P., Thomsen, T., Voon, V., and Miyasaki, J. (2013). Diagnosis and treatment of impulse control disorders in patients with movement disorders. Ther. Adv. Neurol. Disord. 6, 175–188. doi: 10.1177/1756285613476127
Mintz, M., and Tomer, R. (1986). Exposure to amphetamine after substantia nigra lesion interferes with the process of behavioral recovery. Pharmacol. Biochem. Behav. 25, 1307–1311. doi: 10.1016/0091-3057(86)90127-9
Molina-Luna, K., Pekanovic, A., Rohrich, S., Hertler, B., Schubring-Giese, M., Rioult-Pedotti, M. S., et al. (2009). Dopamine in motor cortex is necessary for skill learning and synaptic plasticity. PLoS ONE 4:e7082. doi: 10.1371/journal.pone.0007082
Moo, L., and Wityk, R. J. (1999). Olfactory and taste dysfunction after bilateral middle cerebral artery stroke. J. Stroke Cerebrovasc. Dis. 8, 353–354. doi: 10.1016/S1052-3057(99)80011-1
Morton, W. A., and Stockton, G. G. (2000). Methylphenidate Abuse and Psychiatric Side Effects. Prim. Care Companion J. Clin. Psychiatry 2, 159–164. doi: 10.4088/PCC.v02n0502
Mu, Q., Johnson, K., Morgan, P. S., Grenesko, E. L., Molnar, C. E., Anderson, B., et al. (2007). A single 20 mg dose of the full D1 dopamine agonist dihydrexidine (DAR-0100) increases prefrontal perfusion in schizophrenia. Schizophr. Res. 94, 332–341. doi: 10.1016/j.schres.2007.03.033
Mueller, D., Chapman, C. A., and Stewart, J. (2006). Amphetamine induces dendritic growth in ventral tegmental area dopaminergic neurons in vivo via basic fibroblast growth factor. Neuroscience 137, 727–735. doi: 10.1016/j.neuroscience.2005.09.038
Nakane, M., Teraoka, A., Asato, R., and Tamura, A. (1992). Degeneration of the ipsilateral substantia nigra following cerebral infarction in the striatum. Stroke 23, 328–332. doi: 10.1161/01.STR.23.3.328
Nitsche, M. A., Kuo, M. F., Grosch, J., Bergner, C., Monte-Silva, K., and Paulus, W. (2009). D1-receptor impact on neuroplasticity in humans. J. Neurosci. 29, 2648–2653. doi: 10.1523/JNEUROSCI.5366-08.2009
Nitsche, M. A., Lampe, C., Antal, A., Liebetanz, D., Lang, N., Tergau, F., et al. (2006). Dopaminergic modulation of long-lasting direct current-induced cortical excitability changes in the human motor cortex. Eur. J. Neurosci. 23, 1651–1657. doi: 10.1111/j.1460-9568.2006.04676.x
Nutt, J. G., and Fellman, J. H. (1984). Pharmacokinetics of levodopa. Clin. Neuropharmacol. 7, 35–49. doi: 10.1097/00002826-198403000-00002
Obi, K., Amano, I., and Takatsuru, Y. (2018). Role of dopamine on functional recovery in the contralateral hemisphere after focal stroke in the somatosensory cortex. Brain Res. 1678, 146–152. doi: 10.1016/j.brainres.2017.10.022
Oczkowski, W. (2013). Pharmacological therapies to enhance motor recovery and walking after stroke: emerging strategies. Expert Rev. Neurother. 13, 903–909. doi: 10.1586/14737175.2013.814940
Ogawa, T., Okudera, T., Inugami, A., Noguchi, K., Kado, H., Yoshida, Y., et al. (1997). Degeneration of the ipsilateral substantia nigra after striatal infarction: evaluation with MR imaging. Radiology 204, 847–851. doi: 10.1148/radiology.204.3.9280270
Ohara, S., Kondo, K., Kagoshima, M., and Yanagisawa, N. (1989). [Secondary degeneration of substantia nigra following massive basal ganglia infarction]. Rinsho Shinkeigaku 29, 1352–1356.
Ohlin, K. E., Sebastianutto, I., Adkins, C. E., Lundblad, C., Lockman, P. R., and Cenci, M. A. (2012). Impact of L-DOPA treatment on regional cerebral blood flow and metabolism in the basal ganglia in a rat model of Parkinson's disease. Neuroimage 61, 228–239. doi: 10.1016/j.neuroimage.2012.02.066
Ohta, K., Fujinami, A., Kuno, S., Sakakimoto, A., Matsui, H., Kawahara, Y., et al. (2004). Cabergoline stimulates synthesis and secretion of nerve growth factor, brain-derived neurotrophic factor and glial cell line-derived neurotrophic factor by mouse astrocytes in primary culture. Pharmacology 71, 162–168. doi: 10.1159/000077451
Ohta, K., Kuno, S., Inoue, S., Ikeda, E., Fujinami, A., and Ohta, M. (2010). The effect of dopamine agonists: the expression of GDNF, NGF, and BDNF in cultured mouse astrocytes. J. Neurol. Sci. 291, 12–16. doi: 10.1016/j.jns.2010.01.013
Ohwatashi, A., Ikeda, S., Harada, K., Kamikawa, Y., and Yoshida, A. (2013). Exercise enhanced functional recovery and expression of GDNF after photochemically induced cerebral infarction in the rat. EXCLI J. 12, 693–700.
Okada, Y., Sakai, H., Kohiki, E., Suga, E., Yanagisawa, Y., Tanaka, K., et al. (2005). A dopamine D4 receptor antagonist attenuates ischemia-induced neuronal cell damage via upregulation of neuronal apoptosis inhibitory protein. J. Cereb. Blood Flow Metab. 25, 794–806. doi: 10.1038/sj.jcbfm.9600078
Otmakhova, N. A., and Lisman, J. E. (1996). D1/D5 dopamine receptor activation increases the magnitude of early long-term potentiation at CA1 hippocampal synapses. J. Neurosci. 16, 7478–7486. doi: 10.1523/JNEUROSCI.16-23-07478.1996
Paciaroni, M., and Bogousslavsky, J. (2011). Trafermin for stroke recovery: is it time for another randomized clinical trial? Expert Opin. Biol. Ther. 11, 1533–1541. doi: 10.1517/14712598.2011.616888
Papadopoulos, C. M., Tsai, S. Y., Guillen, V., Ortega, J., Kartje, G. L., and Wolf, W. A. (2009). Motor recovery and axonal plasticity with short-term amphetamine after stroke. Stroke 40, 294–302. doi: 10.1161/STROKEAHA.108.519769
Pauly, V., Frauger, E., Lepelley, M., Mallaret, M., Boucherie, Q., and Micallef, J. (2018). Patterns and profiles of methylphenidate use both in children and adults. Br. J. Clin. Pharmacol. 84, 1215–1227. doi: 10.1111/bcp.13544
Pearson-Fuhrhop, K. M., Minton, B., Acevedo, D., Shahbaba, B., and Cramer, S. C. (2013). Genetic variation in the human brain dopamine system influences motor learning and its modulation by L-Dopa. PLoS ONE 8:e61197. doi: 10.1371/journal.pone.0061197
Pei, Y., Asif-Malik, A., and Canales, J. J. (2016). Trace amines and the trace amine-associated receptor 1: pharmacology, neurochemistry, and clinical implications. Front. Neurosci. 10:148. doi: 10.3389/fnins.2016.00148
Perreault, M. L., Fan, T., Alijaniaram, M., O'Dowd, B. F., and George, S. R. (2012). Dopamine D1-D2 receptor heteromer in dual phenotype GABA/glutamate-coexpressing striatal medium spiny neurons: regulation of BDNF, GAD67 and VGLUT1/2. PLoS ONE 7:e33348. doi: 10.1371/journal.pone.0033348
Platz, T., Kim, I. H., Engel, U., Pinkowski, C., Eickhof, C., and Kutzner, M. (2005). Amphetamine fails to facilitate motor performance and to enhance motor recovery among stroke patients with mild arm paresis: interim analysis and termination of a double blind, randomised, placebo-controlled trial. Restor. Neurol. Neurosci. 23, 271–280.
Ploughman, M., Windle, V., MacLellan, C. L., White, N., Dore, J. J., and Corbett, D. (2009). Brain-derived neurotrophic factor contributes to recovery of skilled reaching after focal ischemia in rats. Stroke 40, 1490–1495. doi: 10.1161/STROKEAHA.108.531806
Politi, C., Ciccacci, C., Novelli, G., and Borgiani, P. (2018). Genetics and treatment response in Parkinson's disease: an update on pharmacogenetic studies. Neuromolecular Med. 20, 1–17. doi: 10.1007/s12017-017-8473-7
Prinz, V., Hetzer, A. M., Muller, S., Balkaya, M., Leithner, C., Kronenberg, G., et al. (2015). MRI heralds secondary nigral lesion after brain ischemia in mice: a secondary time window for neuroprotection. J. Cereb. Blood Flow Metab. 35, 1903–1909. doi: 10.1038/jcbfm.2015.153
Qiu, J., Yan, Z., Tao, K., Li, Y., Li, Y., Li, J., et al. (2016). Sinomenine activates astrocytic dopamine D2 receptors and alleviates neuroinflammatory injury via the CRYAB/STAT3 pathway after ischemic stroke in mice. J. Neuroinflammation 13:263. doi: 10.1186/s12974-016-0739-8
Queen, S. A., Chen, M. J., and Feeney, D. M. (1997). d-Amphetamine attenuates decreased cerebral glucose utilization after unilateral sensorimotor cortex contusion in rats. Brain Res. 777, 42–50. doi: 10.1016/S0006-8993(97)00717-8
Ramic, M., Emerick, A. J., Bollnow, M. R., O'Brien, T. E., Tsai, S. Y., and Kartje, G. L. (2006). Axonal plasticity is associated with motor recovery following amphetamine treatment combined with rehabilitation after brain injury in the adult rat. Brain Res. 1111, 176–186. doi: 10.1016/j.brainres.2006.06.063
Rasmussen, R. S., Overgaard, K., Hildebrandt-Eriksen, E. S., and Boysen, G. (2006). D-amphetamine improves cognitive deficits and physical therapy promotes fine motor rehabilitation in a rat embolic stroke model. Acta Neurol. Scand. 113, 189–198. doi: 10.1111/j.1600-0404.2005.00547.x
Rasmussen, R. S., Overgaard, K., Kristiansen, U., and Johansen, F. F. (2011). Acute but not delayed amphetamine treatment improves behavioral outcome in a rat embolic stroke model. Neurol. Res. 33, 774–782. doi: 10.1179/1743132811Y.0000000009
Reding, M. J., and Borucki, S. (1995). Effect of Dextroamphetamine on motor recovery after stroke [abstract]. Neurology 45(Suppl. 4):A222.
Restemeyer, C., Weiller, C., and Liepert, J. (2007). No effect of a levodopa single dose on motor performance and motor excitability in chronic stroke. A double-blind placebo-controlled cross-over pilot study. Restor. Neurol. Neurosci. 25, 143–150.
Reuss, B., Leung, D. S., Ohlemeyer, C., Kettenmann, H., and Unsicker, K. (2000). Regionally distinct regulation of astroglial neurotransmitter receptors by fibroblast growth factor-2. Mol. Cell. Neurosci. 16, 42–58. doi: 10.1006/mcne.2000.0857
Ricaurte, G. A., Mechan, A. O., Yuan, J., Hatzidimitriou, G., Xie, T., Mayne, A. H., et al. (2005). Amphetamine treatment similar to that used in the treatment of adult attention-deficit/hyperactivity disorder damages dopaminergic nerve endings in the striatum of adult nonhuman primates. J. Pharmacol. Exp. Ther. 315, 91–98. doi: 10.1124/jpet.105.087916
Richards, D. A., Obrenovitch, T. P., Symon, L., and Curzon, G. (1993). Extracellular dopamine and serotonin in the rat striatum during transient ischaemia of different severities: a microdialysis study. J. Neurochem. 60, 128–136. doi: 10.1111/j.1471-4159.1993.tb05830.x
Rioult-Pedotti, M. S., Pekanovic, A., Atiemo, C. O., Marshall, J., and Luft, A. R. (2015). Dopamine promotes motor cortex plasticity and motor skill learning via PLC activation. PLoS ONE 10:e0124986. doi: 10.1371/journal.pone.0124986
Roceri, M., Molteni, R., Fumagalli, F., Racagni, G., Gennarelli, M., Corsini, G., et al. (2001). Stimulatory role of dopamine on fibroblast growth factor-2 expression in rat striatum. J. Neurochem. 76, 990–997. doi: 10.1046/j.1471-4159.2001.00088.x
Rodriguez-Grande, B., Blackabey, V., Gittens, B., Pinteaux, E., and Denes, A. (2013). Loss of substance P and inflammation precede delayed neurodegeneration in the substantia nigra after cerebral ischemia. Brain Behav. Immun. 29, 51–61. doi: 10.1016/j.bbi.2012.11.017
Rogge, G., Jones, D., Hubert, G. W., Lin, Y., and Kuhar, M. J. (2008). CART peptides: regulators of body weight, reward and other functions. Nat. Rev. Neurosci. 9, 747–758. doi: 10.1038/nrn2493
Rogozinska, K., and Skangiel-Kramska, J. (2010). Effect of focal cerebral ischaemia on modulatory neurotransmitter receptors in the rat brain: an autoradiographic study. J. Chem. Neuroanat. 40, 232–238. doi: 10.1016/j.jchemneu.2010.06.004
Root, D. H., Hoffman, A. F., Good, C. H., Zhang, S., Gigante, E., Lupica, C. R., et al. (2015). Norepinephrine activates dopamine D4 receptors in the rat lateral habenula. J. Neurosci. 35, 3460–3469. doi: 10.1523/JNEUROSCI.4525-13.2015
Rösser, N., and Flöel, A. (2008). Pharmacological enhancement of motor recovery in subacute and chronic stroke. Neurorehabilitation 23, 95–103.
Rösser, N., Heuschmann, P., Wersching, H., Breitenstein, C., Knecht, S., and Flöel, A. (2008). Levodopa improves procedural motor learning in chronic stroke patients. Arch. Phys. Med. Rehabil. 89, 1633–1641. doi: 10.1016/j.apmr.2008.02.030
Rousseaux, M., Muller, P., Gahide, I., Mottin, Y., and Romon, M. (1996). Disorders of smell, taste, and food intake in a patient with a dorsomedial thalamic infarct. Stroke 27, 2328–2330. doi: 10.1161/01.STR.27.12.2328
Ruan, H., Saur, T., and Yao, W. D. (2014). Dopamine-enabled anti-Hebbian timing-dependent plasticity in prefrontal circuitry. Front. Neural Circuits 8:38. doi: 10.3389/fncir.2014.00038
Ruppert, E., Bataillard, M., Namer, I. J., Tatu, L., Hacquard, A., Hugueny, L., et al. (2017). Hyperdopaminergism in lenticulostriate stroke-related restless legs syndrome: an imaging study. Sleep Med. 30, 136–138. doi: 10.1016/j.sleep.2016.02.011
Ruscher, K., Kuric, E., and Wieloch, T. (2012). Levodopa treatment improves functional recovery after experimental stroke. Stroke 43, 507–513. doi: 10.1161/STROKEAHA.111.638767
Schäbitz, W. R., Steigleder, T., Cooper-Kuhn, C. M., Schwab, S., Sommer, C., Schneider, A., et al. (2007). Intravenous brain-derived neurotrophic factor enhances poststroke sensorimotor recovery and stimulates neurogenesis. Stroke 38, 2165–2172. doi: 10.1161/STROKEAHA.106.477331
Scheidtmann, K., Fries, W., Muller, F., and Koenig, E. (2001). Effect of levodopa in combination with physiotherapy on functional motor recovery after stroke: a prospective, randomised, double-blind study. Lancet 358, 787–790. doi: 10.1016/S0140-6736(01)05966-9
Schmanke, T. D., Avery, R. A., and Barth, T. M. (1996). The effects of amphetamine on recovery of function after cortical damage in the rat depend on the behavioral requirements of the task. J. Neurotrauma 13, 293–307.
Schmanke, T., and Barth, T. M. (1997). Amphetamine and task-specific practice augment recovery of vibrissae-evoked forelimb placing after unilateral sensorimotor cortical injury in the rat. J. Neurotrauma 14, 459–468. doi: 10.1089/neu.1997.14.459
Schönfeld, L. M., Dooley, D., Jahanshahi, A., Temel, Y., and Hendrix, S. (2017). Evaluating rodent motor functions: which tests to choose? Neurosci. Biobehav. Rev. 83, 298–312. doi: 10.1016/j.neubiorev.2017.10.021
Schuster, C., Maunz, G., Lutz, K., Kischka, U., Sturzenegger, R., and Ettlin, T. (2011). Dexamphetamine improves upper extremity outcome during rehabilitation after stroke: a pilot randomized controlled trial. Neurorehabil. Neural Repair 25, 749–755. doi: 10.1177/1545968311405674
Shen, W., Flajolet, M., Greengard, P., and Surmeier, D. J. (2008). Dichotomous dopaminergic control of striatal synaptic plasticity. Science 321, 848–851. doi: 10.1126/science.1160575
Sieber, M. W., Guenther, M., Jaenisch, N., Albrecht-Eckardt, D., Kohl, M., Witte, O. W., et al. (2014). Age-specific transcriptional response to stroke. Neurobiol. Aging 35, 1744–1754. doi: 10.1016/j.neurobiolaging.2014.01.012
Siniscalchi, A., Gallelli, L., Labate, A., Malferrari, G., Palleria, C., and Sarro, G. D. (2012). Post-stroke movement disorders: clinical manifestations and pharmacological management. Curr. Neuropharmacol. 10, 254–262. doi: 10.2174/157015912803217341
Slevin, M., Kumar, P., Gaffney, J., Kumar, S., and Krupinski, J. (2006). Can angiogenesis be exploited to improve stroke outcome? Mechanisms and therapeutic potential. Clin. Sci. 111, 171–183. doi: 10.1042/CS20060049
Slivka, A., Brannan, T. S., Weinberger, J., Knott, P. J., and Cohen, G. (1988). Increase in extracellular dopamine in the striatum during cerebral ischemia: a study utilizing cerebral microdialysis. J. Neurochem. 50, 1714–1718. doi: 10.1111/j.1471-4159.1988.tb02468.x
Smith, C. C., and Greene, R. W. (2012). CNS dopamine transmission mediated by noradrenergic innervation. J. Neurosci. 32, 6072–6080. doi: 10.1523/JNEUROSCI.6486-11.2012
Sonde, L., and Lökk, J. (2007). Effects of amphetamine and/or L-dopa and physiotherapy after stroke - a blinded randomized study. Acta Neurol. Scand. 115, 55–59. doi: 10.1111/j.1600-0404.2006.00728.x
Sonde, L., Nordstrom, M., Nilsson, C. G., Lökk, J., and Viitanen, M. (2001). A double-blind placebo-controlled study of the effects of amphetamine and physiotherapy after stroke. Cerebrovasc. Dis. 12, 253–257. doi: 10.1159/000047712
Soriano, M. A., Justicia, C., Ferrer, I., Rodriguez-Farre, E., and Planas, A. M. (1997). Striatal infarction in the rat causes a transient reduction of tyrosine hydroxylase immunoreactivity in the ipsilateral substantia nigra. Neurobiol. Dis. 4, 376–385. doi: 10.1006/nbdi.1997.0166
Spiegel, D. R., and Chatterjee, A. (2014). A case of abulia, status/post right middle cerebral artery territory infarct, treated successfully with olanzapine. Clin. Neuropharmacol. 37, 186–189. doi: 10.1097/WNF.0000000000000053
Sprigg, N., and Bath, P. M. (2009). Speeding stroke recovery? A systematic review of amphetamine after stroke. J. Neurol. Sci. 285, 3–9. doi: 10.1016/j.jns.2009.04.040
Stanfill, A., Elijovich, L., Baughman, B., and Conley, Y. (2016). A review and conceptual model of dopaminergic contributions to poststroke depression. J. Neurosci. Nurs. 48, 242–246. doi: 10.1097/JNN.0000000000000240
Stein, M. A., Waldman, I. D., Sarampote, C. S., Seymour, K. E., Robb, A. S., Conlon, C., et al. (2005). Dopamine transporter genotype and methylphenidate dose response in children with ADHD. Neuropsychopharmacology 30, 1374–1382. doi: 10.1038/sj.npp.1300718
Stewart, J. C., and Cramer, S. C. (2017). Genetic variation and neuroplasticity: role in rehabilitation after stroke. J. Neurol. Phys. Ther 41(Suppl. 3), S17–S23. doi: 10.1097/NPT.0000000000000180
Stroemer, R. P., Kent, T. A., and Hulsebosch, C. E. (1998). Enhanced neocortical neural sprouting, synaptogenesis, and behavioral recovery with D-amphetamine therapy after neocortical infarction in rats. Stroke 29, 2381–2393; discussion: 2393–2395. doi: 10.1161/01.STR.29.11.2381
Sutton, R. L., Hovda, D. A., and Feeney, D. M. (1989). Amphetamine accelerates recovery of locomotor function following bilateral frontal cortex ablation in cats. Behav. Neurosci. 103, 837–841. doi: 10.1037/0735-7044.103.4.837
Sutton, R. L., Hovda, D. A., Chen, M. J., and Feeney, D. M. (2000). Alleviation of brain injury-induced cerebral metabolic depression by amphetamine: a cytochrome oxidase histochemistry study. Neural Plast. 7, 109–125. doi: 10.1155/NP.2000.109
Takeuchi, T., Duszkiewicz, A. J., Sonneborn, A., Spooner, P. A., Yamasaki, M., Watanabe, M., et al. (2016). Locus coeruleus and dopaminergic consolidation of everyday memory. Nature 537, 357–362. doi: 10.1038/nature19325
Takeuchi, Y., Fukunaga, K., and Miyamoto, E. (2002). Activation of nuclear Ca(2+)/calmodulin-dependent protein kinase II and brain-derived neurotrophic factor gene expression by stimulation of dopamine D2 receptor in transfected NG108-15 cells. J. Neurochem. 82, 316–328. doi: 10.1046/j.1471-4159.2002.00967.x
Tamura, A., Kirino, T., Sano, K., Takagi, K., and Oka, H. (1990). Atrophy of the ipsilateral substantia nigra following middle cerebral artery occlusion in the rat. Brain Res. 510, 154–157
Tan, C. O. (2009). Anticipatory changes in regional cerebral hemodynamics: a new role for dopamine? J. Neurophysiol. 101, 2738–2740. doi: 10.1152/jn.00141.2009
Tardy, J., Pariente, J., Leger, A., Dechaumont-Palacin, S., Gerdelat, A., Guiraud, V., et al. (2006). Methylphenidate modulates cerebral post-stroke reorganization. Neuroimage 33, 913–922. doi: 10.1016/j.neuroimage.2006.07.014
Thirugnanasambandam, N., Grundey, J., Paulus, W., and Nitsche, M. A. (2011). Dose-dependent nonlinear effect of L-DOPA on paired associative stimulation-induced neuroplasticity in humans. J. Neurosci. 31, 5294–5299. doi: 10.1523/JNEUROSCI.6258-10.2011
Toner, C. C., and Stamford, J. A. (1996). 'Real time' measurement of dopamine release in an in vitro model of neostriatal ischaemia. J. Neurosci. Methods 67, 133–140.
Tran, D. A., Pajaro-Blazquez, M., Daneault, J. F., Gallegos, J. G., Pons, J., Fregni, F., et al. (2016). Combining Dopaminergic facilitation with robot-assisted upper limb therapy in stroke survivors: a focused review. Am. J. Phys. Med. Rehabil. 95, 459–474. doi: 10.1097/PHM.0000000000000438
Treig, T., Werner, C., Sachse, M., and Hesse, S. (2003). No benefit from D-amphetamine when added to physiotherapy after stroke: a randomized, placebo-controlled study. Clin. Rehabil. 17, 590–599. doi: 10.1191/0269215503cr653oa
Uzdensky, A., Demyanenko, S., Fedorenko, G., Lapteva, T., and Fedorenko, A. (2017). Protein profile and morphological alterations in penumbra after focal photothrombotic infarction in the rat cerebral cortex. Mol. Neurobiol. 54, 4172–4188. doi: 10.1007/s12035-016-9964-5
Vachalathiti, R., Asavavallobh, C., Nilanont, Y., and Poungvarin, N. (2001). Comparison of physical therapy and physical therapy with amphetamine in sensorimotor recovery of acute stroke patients: randomised controlled trial. J. Neurol. Sci. 187(Suppl. 1):S253.
Vaillancourt, D. E., Schonfeld, D., Kwak, Y., Bohnen, N. I., and Seidler, R. (2013). Dopamine overdose hypothesis: evidence and clinical implications. Mov. Disord. 28, 1920–1929. doi: 10.1002/mds.25687
van der Kemp, J., Dorresteijn, M., Ten Brink, A. F., Nijboer, T. C., and Visser-Meily, J. M. (2017). Pharmacological treatment of visuospatial neglect: a systematic review. J. Stroke Cerebrovasc. Dis. 26, 686–700. doi: 10.1016/j.jstrokecerebrovasdis.2017.02.012
Vanderheyden, P., Ebinger, G., Kanarek, L., and Vauquelin, G. (1986). Epinephrine and norepinephrine stimulation of adenylate cyclase in bovine retina homogenate: evidence for interaction with the dopamine D1 receptor. Life Sci. 38, 1221–1227. doi: 10.1016/0024-3205(86)90177-3
Viale, L., Catoira, N. P., Di Girolamo, G., and Gonzalez, C. D. (2018). Pharmacotherapy and motor recovery after stroke. Expert Rev. Neurother. 18, 65–82. doi: 10.1080/14737175.2018.1400910
von Essen, C. (1974). Effects of dopamine on the cerebral blood flow in the dog. Acta Neurol. Scand. 50, 39–52. doi: 10.1111/j.1600-0404.1974.tb01345.x
Voon, V., Napier, T. C., Frank, M. J., Sgambato-Faure, V., Grace, A. A., Rodriguez-Oroz, M., et al. (2017). Impulse control disorders and levodopa-induced dyskinesias in Parkinson's disease: an update. Lancet Neurol. 16, 238–250. doi: 10.1016/S1474-4422(17)30004-2
Wada, K., Sugimori, H., Bhide, P. G., Moskowitz, M. A., and Finklestein, S. P. (2003). Effect of basic fibroblast growth factor treatment on brain progenitor cells after permanent focal ischemia in rats. Stroke 34, 2722–2728. doi: 10.1161/01.STR.0000094421.61917.71
Walker-Batson, D., Smith, P., Curtis, S., Unwin, H., and Greenlee, R. (1995). Amphetamine paired with physical therapy accelerates motor recovery after stroke. Further evidence. Stroke 26, 2254–2259. doi: 10.1161/01.STR.26.12.2254
Wang, M., Wong, A. H., and Liu, F. (2012). Interactions between NMDA and dopamine receptors: a potential therapeutic target. Brain Res. 1476, 154–163. doi: 10.1016/j.brainres.2012.03.029
Wang, Q. M., Cui, H., Han, S. J., Black-Schaffer, R., Volz, M. S., Lee, Y. T., et al. (2014). Combination of transcranial direct current stimulation and methylphenidate in subacute stroke. Neurosci. Lett. 569, 6–11. doi: 10.1016/j.neulet.2014.03.011
Wehling, E., Naess, H., Wollschlaeger, D., Hofstad, H., Bramerson, A., Bende, M., et al. (2015). Olfactory dysfunction in chronic stroke patients. BMC Neurol. 15:199. doi: 10.1186/s12883-015-0463-5
Weinberger, J. (2002). The role of dopamine in cerebral ischemic damage: a review of studies with Gerald Cohen. Parkinsonism Relat. Disord. 8, 413–416. doi: 10.1016/S1353-8020(02)00023-8
Weinberger, J., Cohen, G., and Nieves-Rosa, J. (1983). Nerve terminal damage in cerebral ischemia: greater susceptibility of catecholamine nerve terminals relative to serotonin nerve terminals. Stroke 14, 986–989. doi: 10.1161/01.STR.14.6.986
Weintraub, D., Koester, J., Potenza, M. N., Siderowf, A. D., Stacy, M., Voon, V., et al. (2010). Impulse control disorders in Parkinson disease: a cross-sectional study of 3090 patients. Arch. Neurol. 67, 589–595. doi: 10.1001/archneurol.2010.65
Willuhn, I., and Steiner, H. (2006). Motor-skill learning-associated gene regulation in the striatum: effects of cocaine. Neuropsychopharmacology 31, 2669–2682. doi: 10.1038/sj.npp.1300995
Willuhn, I., and Steiner, H. (2008). Motor-skill learning in a novel running-wheel task is dependent on D1 dopamine receptors in the striatum. Neuroscience 153, 249–258. doi: 10.1016/j.neuroscience.2008.01.041
Willuhn, I., Sun, W., and Steiner, H. (2003). Topography of cocaine-induced gene regulation in the rat striatum: relationship to cortical inputs and role of behavioural context. Eur. J. Neurosci. 17, 1053–1066. doi: 10.1046/j.1460-9568.2003.02525.x
Winter, B., Juckel, G., Viktorov, I., Katchanov, J., Gietz, A., Sohr, R., et al. (2005). Anxious and hyperactive phenotype following brief ischemic episodes in mice. Biol. Psychiatry 57, 1166–1175. doi: 10.1016/j.biopsych.2005.02.010
Wirkner, K., Krause, T., Koles, L., Thummler, S., Al-Khrasani, M., and Illes, P. (2004). D1 but not D2 dopamine receptors or adrenoceptors mediate dopamine-induced potentiation of N-methyl-d-aspartate currents in the rat prefrontal cortex. Neurosci. Lett. 372, 89–93. doi: 10.1016/j.neulet.2004.09.015
Witte, A. V., Kurten, J., Jansen, S., Schirmacher, A., Brand, E., Sommer, J., et al. (2012). Interaction of BDNF and COMT polymorphisms on paired-associative stimulation-induced cortical plasticity. J. Neurosci. 32, 4553–4561. doi: 10.1523/JNEUROSCI.6010-11.2012
Wolf, M. E., Mangiavacchi, S., and Sun, X. (2003). Mechanisms by which dopamine receptors may influence synaptic plasticity. Ann. N. Y. Acad. Sci. 1003, 241–249. doi: 10.1196/annals.1300.015
Wolf, W. A., Martin, J. L., Kartje, G. L., and Farrer, R. G. (2014). Evidence for fibroblast growth factor-2 as a mediator of amphetamine-enhanced motor improvement following stroke. PLoS ONE 9:e108031. doi: 10.1371/journal.pone.0108031
Xing, B., Guo, J., Meng, X., Wei, S. G., and Li, S. B. (2012). The dopamine D1 but not D3 receptor plays a fundamental role in spatial working memory and BDNF expression in prefrontal cortex of mice. Behav. Brain Res. 235, 36–41. doi: 10.1016/j.bbr.2012.06.035
Xu, T. X., Ma, Q., Spealman, R. D., and Yao, W. D. (2010). Amphetamine modulation of long-term potentiation in the prefrontal cortex: dose dependency, monoaminergic contributions, and paradoxical rescue in hyperdopaminergic mutant. J. Neurochem. 115, 1643–1654. doi: 10.1111/j.1471-4159.2010.07073.x
Xu, Y., Zhang, W., Klaus, J., Young, J., Koerner, I., Sheldahl, L. C., et al. (2006). Role of cocaine- and amphetamine-regulated transcript in estradiol-mediated neuroprotection. Proc. Natl. Acad. Sci. U.S.A. 103, 14489–14494. doi: 10.1073/pnas.0602932103
Yamada, K., Goto, S., Yoshikawa, M., and Ushio, Y. (1996). Gabaergic transmission and tyrosine hydroxylase expression in the nigral dopaminergic neurons: an in vivo study using a reversible ischemia model of rats. Neuroscience 73, 783–789. doi: 10.1016/0306-4522(96)00041-3
Yamamoto, B. K., Moszczynska, A., and Gudelsky, G. A. (2010). Amphetamine toxicities: classical and emerging mechanisms. Ann. N. Y. Acad. Sci. 1187, 101–121. doi: 10.1111/j.1749-6632.2009.05141.x
Yulug, B., Yildiz, A., Guzel, O., Kilic, E., Schabitz, W. R., and Kilic, E. (2006a). Risperidone attenuates brain damage after focal cerebral ischemia in vivo. Brain Res. Bull. 69, 656–659. doi: 10.1016/j.brainresbull.2006.03.017
Yulug, B., Yildiz, A., Hudaoglu, O., Kilic, E., Cam, E., and Schabitz, W. R. (2006b). Olanzapine attenuates brain damage after focal cerebral ischemia in vivo. Brain Res. Bull. 71, 296–300. doi: 10.1016/j.brainresbull.2006.09.018
Zervas, N. T., Hori, H., Negora, M., Wurtman, R. J., Larin, F., and Lavyne, M. H. (1974). Reduction in brain dopamine following experimental cerebral ischaemia. Nature 247, 283–284. doi: 10.1038/247283a0
Zhang, G., Zhang, Y., Zhang, C., Wang, Y., Ma, G., Nie, K., et al. (2016). Striatal silent lacunar infarction is associated with changes to the substantia nigra in patients with early-stage Parkinson's disease: a diffusion kurtosis imaging study. J. Clin. Neurosci. 33, 138–141. doi: 10.1016/j.jocn.2016.03.032
Zhang, J., Zhang, Y., Xing, S., Liang, Z., and Zeng, J. (2012). Secondary neurodegeneration in remote regions after focal cerebral infarction: a new target for stroke management? Stroke 43, 1700–1705. doi: 10.1161/STROKEAHA.111.632448
Zhang, X., Zhou, Z., Wang, D., Li, A., Yin, Y., Gu, X., et al. (2009). Activation of phosphatidylinositol-linked D1-like receptor modulates FGF-2 expression in astrocytes via IP3-dependent Ca2+ signaling. J. Neurosci. 29, 7766–7775. doi: 10.1523/JNEUROSCI.0389-09.2009
Zhang, Y., Chen, Y., Wu, J., Manaenko, A., Yang, P., Tang, J., et al. (2015). Activation of dopamine D2 receptor suppresses neuroinflammation through alphab-crystalline by inhibition of NF-kappaB nuclear translocation in experimental ICH mice model. Stroke 46, 2637–2646. doi: 10.1161/STROKEAHA.115.009792
Zhao, F., Kuroiwa, T., Miyasaka, N., Nagaoka, T., Nakane, M., Tamura, A., et al. (2001). Characteristic changes in T(2)-value, apparent diffusion coefficient, and ultrastructure of substantia nigra evolving exofocal postischemic neuronal death in rats. Brain Res. 895, 238–244. doi: 10.1016/S0006-8993(00)03281-9
Keywords: dopamine receptors, amphetamine, L-DOPA, stroke recovery, pharmacotherapy, animal stroke models, clinical trials, motor recovery
Citation: Gower A and Tiberi M (2018) The Intersection of Central Dopamine System and Stroke: Potential Avenues Aiming at Enhancement of Motor Recovery. Front. Synaptic Neurosci. 10:18. doi: 10.3389/fnsyn.2018.00018
Received: 16 March 2018; Accepted: 13 June 2018;
Published: 06 July 2018.
Edited by:
Fang Liu, Centre for Addiction and Mental Health, CanadaReviewed by:
John N. J. Reynolds, University of Otago, New ZealandMohamed Jaber, University of Poitiers, France
Copyright © 2018 Gower and Tiberi. This is an open-access article distributed under the terms of the Creative Commons Attribution License (CC BY). The use, distribution or reproduction in other forums is permitted, provided the original author(s) and the copyright owner(s) are credited and that the original publication in this journal is cited, in accordance with accepted academic practice. No use, distribution or reproduction is permitted which does not comply with these terms.
*Correspondence: Mario Tiberi, bXRpYmVyaUB1b3R0YXdhLmNh