- 1Departments of Anesthesiology and Perioperative Medicine and Pharmacology, Penn State College of Medicine, Hershey, PA, United States
- 2Department of Psychiatry and Behavioral Sciences, Stanford University School of Medicine, Stanford, CA, United States
- 3Departments of Neuroscience and Psychiatry, University of Pittsburgh, Pittsburgh, PA, United States
The lateral habenula (LHb) regulates reward learning and controls the updating of reward-related information. Drugs of abuse have the capacity to hijack the cellular and neurocircuit mechanisms mediating reward learning, forming non-adaptable, compulsive behaviors geared toward obtaining illicit substances. Here, we discuss current findings demonstrating how drugs of abuse alter intrinsic and synaptic LHb neuronal function. Additionally, we discuss evidence for how drug-induced LHb alterations may affect the ability to predict reward, potentially facilitating an addiction-like state. Altogether, we combine ex vivo and in vivo results for an overview of how drugs of abuse alter LHb function and how these functional alterations affect the ability to learn and update behavioral responses to hedonic external stimuli.
Introduction
The ability to accurately predict rewarding or aversive outcomes throughout life is a critical adaptive behavior that promotes survival and allows avoidance of threatening or unpleasant confrontations. This adaptive ability is attributed to an evolutionarily conserved hedonic neurocircuit which consists of an interwoven network linking forebrain, midbrain and hindbrain regions, that together systematically regulate the release of monoamines (neuromodulators that mediate reward prediction and motivated behaviors; Kelley, 2005). The lateral habenula (LHb) is centrally located within this hedonic neurocircuit (Figure 1; Aizawa et al., 2011; Shelton et al., 2012) and regulates monoamine release and monoamine controlled behaviors including pain modulation, sleep, stress, motor behaviors and the focus of this review, reward learning (Lecourtier and Kelly, 2007; Hikosaka, 2010; Evely et al., 2016; Boulos et al., 2017).
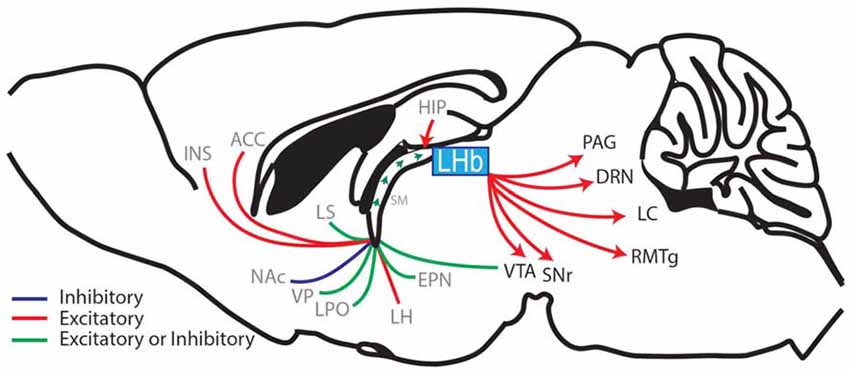
Figure 1. Sagittal rodent brain section illustrating the afferents and efferents of the lateral habenula (LHb). The LHb interconnects the forebrain with the midbrain and hindbrain regions by receiving afferents through the stria medullaris (SM), which contains fibers from the hippocampus (HIP), ventral pallidum (VP), lateral hypothalamus (LH), entopeduncular nucleus (EPN; the EPN is equivalent to the globus pallidus interna in primates and humans and sends a prominent afferent to the LHb (Hong and Hikosaka, 2008; Wallace et al., 2017)) diagonal band of Broca (cholinergic nerve fibers, not shown), lateral septum (LS), lateral preoptic area (LPO), ventral tegmental area (VTA; Stamatakis et al., 2013; Lammel et al., 2015), nucleus accumbens (NAc), anterior cingulate cortex (ACC) and the anterior insula (INS; afferent brain regions labeled in gray). The LHb then relays information via glutamatergic efferents that together form the fasciculus retroflexus. These efferents project to the substantia nigra (SNr), VTA, rostral medial tegmental nucleus (RMTg), the dorsal raphe nuclei (DRN), the locus coeruleus (LC) and the periaqueductal gray (PAG) (LHb-projecting brain regions labeled in black; Aizawa et al., 2011; Shelton et al., 2012; Quina et al., 2015; Yetnikoff et al., 2015).
Reward learning crucially relies on midbrain dopamine neuron activity. This neuronal activity is precisely timed with reward predictions (expected reward) and reward outcomes (Schultz et al., 1997; Steinberg et al., 2013; Chang et al., 2017; Schultz, 2017). If the reward received is better or greater than the predicted reward, it is referred to as a positive reward prediction error (RPE) and is associated with increases in dopamine neuron firing (Schultz et al., 1997). On the other hand, if the reward received is worse or less than the predicted reward, it is referred to as a negative RPE and is associated with decreases in dopamine neuron firing (Schultz et al., 1997). The LHb is linked to this process, as stimulating neurons in the LHb strongly inhibits midbrain dopamine neurons (Christoph et al., 1986; Ji and Shepard, 2007; Matsumoto and Hikosaka, 2007). Additionally, the LHb acts as a center for negative RPEs, as subpopulations of neurons respond to negative prediction errors with neuronal excitation, and to positive prediction errors with neuronal inhibition (Bromberg-Martin and Hikosaka, 2011).
This review discusses current evidence that links the LHb to reward prediction by focusing on the LHb’s activation patterns and regulation of efferent targets during exposure to hedonic stimuli. Additionally, we discuss the underlying mechanisms related to drug-induced changes in LHb functional output, and discuss evidence for how drug-induced LHb alterations may affect the ability to predict reward, potentially facilitating an addiction-like state.
How Does the LHb Regulate Reward Prediction and Reward Learning?
The LHb regulates reward prediction and reward learning, in part, through its glutamate projections to the rostromedial tegmental nucleus (RMTg), a brain region that sends inhibitory input to dopamine neurons in the ventral tegmental area (VTA). During negative RPEs, LHb neurons are activated and send excitatory signals to RMTg inhibitory neurons which project to and inhibit dopamine neuron firing (Matsumoto and Hikosaka, 2007; Jhou et al., 2009; Kaufling et al., 2009; Lammel et al., 2012; Figure 1). The LHb’s control over midbrain dopamine neurons is significant because inhibition of dopamine neuron firing is causally associated with negative RPEs and extinction learning (Steinberg et al., 2013; Chang et al., 2016, 2017). Furthermore, LHb activity does not correlate only with negative valence, but also acts as a center for negative RPE (Ullsperger and von Cramon, 2003; Salas et al., 2010; Bromberg-Martin and Hikosaka, 2011), as LHb neurons respond to reward information that is unexpectedly cued, delivered, or denied (Bromberg-Martin and Hikosaka, 2011). Thus, it appears that the LHb receives and processes negative RPE information in much the same way that dopamine neurons process positive RPE signals.
Based on the LHb’s role in processing negative RPE signals, it follows that LHb activity is critically involved in learning and memory processes that are associated with avoidance behaviors (Stamatakis and Stuber, 2012). As discussed earlier, the LHb regulates reward prediction through its glutamate projections to the RMTg. In addition, the LHb sends direct glutamate projections to the VTA, which, when activated, promote avoidance behaviors (Lammel et al., 2012). Light stimulation of LHb neurons that project directly to the VTA causes strong conditioned place aversion, with evidence suggesting that VTA neurons receiving direct excitatory connections from the LHb preferentially project to the medial prefrontal cortex (Lammel et al., 2012). Since a subpopulation of VTA dopamine neurons are activated during exposure to noxious stimuli (Brischoux et al., 2009; Bromberg-Martin et al., 2010), these results suggest that the LHb may promote aversive behaviors through neurocircuit pathways that include specific subpopulations of dopamine neurons.
Examining loss-of-function in the LHb shows that inhibiting LHb function with habenular lesions in rats impairs their ability to recognize or learn newly introduced escape routes in a forced swim test (Thornton et al., 1985), and also impairs their ability to learn to avoid a foot shock during an active avoidance task (Thornton and Bradbury, 1989). Bilateral lesions in rats impair inhibitory avoidance acquisition (latency to leave an enclosed elevated T-maze arm and enter a non-enclosed arm), though panic and escape responses appear to be normal (Pobbe and Zangrossi, 2008). Also, habenula lesions in the rat prevent learned helplessness in a classical foot shock paradigm (Amat et al., 2001). By reducing LHb output to the dorsal raphe, the classically observed increase in serotonin signaling during learned helplessness is attenuated, preventing the formation or expression of learned helplessness behavior (Amat et al., 2001). Lastly, LHb lesions in mice cause dopamine RPE signals to no longer respond specifically to reward omissions, and also cause dopamine RPEs to subsequently lose the ability to signal graded levels of reward reliably, though a significant response still remains (Tian and Uchida, 2015). Together, studies examining loss-of-function in the LHb show evidence of impaired learning, particularly to aversive stimuli. However, work continues to dissect the distinct contribution of the LHb to conditioned-learning processes, as the LHb may participate in aversive learning but not punishment-associated avoidance behaviors (Jean-Richard Dit Bressel and McNally, 2014; Zapata et al., 2017).
Does Localized Drug Administration Alter LHb Neuronal Function?
Subpopulations of neurons in the LHb are differentially regulated by cocaine, a dopamine uptake blocker and one of the commonly abused drugs. LHb neurons that increase their firing during a nociceptive tail pinch or neurons that are non-responsive (non-nociceptive) to a tail pinch have decreases in firing rates during cocaine microiontophoresis application (Dougherty et al., 1990). In contrast, LHb neurons that decrease their firing during a nociceptive tail pinch have increases in firing rates during localized cocaine administration (Dougherty et al., 1990). Cocaine-induced increases in LHb neuronal firing rates are likely due to dopamine-dependent increases in inward currents, dopamine-dependent increases in glutamatergic synaptic transmission, and decreases in GABA presynaptic release (Good et al., 2013; Zuo et al., 2013). In addition to decreasing GABA presynaptic release, localized cocaine administration increases GABA presynaptic release on subpopulations of LHb neurons (Zuo et al., 2013), which may contribute to the above mentioned cocaine-induced decreases in LHb neuronal firing.
Localized ethanol administration, similar to cocaine, increases LHb neuronal firing and enhances presynaptic glutamate release in a process that is regulated through dopamine D1-receptor activation (Zuo et al., 2017a). However, this increase in LHb neuronal firing is limited by ongoing GABAAR-mediated inhibition, which is also enhanced by ethanol and is likely caused by dopamine-dependent pre and postsynaptic modifications (Zuo et al., 2017b). Furthermore, the effects of ethanol on spontaneous excitatory and inhibitory postsynaptic currents (sEPSC and sIPSC, respectively) vary among LHb neurons. Some neurons undergo differential potentiation of sEPSCs and sIPSCs, other neurons show changes in sEPSCs and sIPSCs that are inversely related, and yet different sets of neurons do not undergo any changes in sEPSCs or in sIPSCs (Zuo et al., 2017b).
Finally, localized nicotine administration depolarizes LHb neurons and increases their firing activity (Zuo et al., 2016). At the synapse, nicotine enhances presynaptic glutamate release and enhances GABAergic transmission through pre and postsynaptic modifications (Zuo et al., 2016).
Taken together, these results demonstrate that localized application of addictive substances in the LHb influences a wide array of neuronal properties including firing rates and excitatory and inhibitory transmission, often with diverse effects among subpopulations of neurons. Although these subpopulations have yet to be fully characterized, one potential distinguishing characteristic is the LHb’s projection targets, as data suggest that LHb neurons projecting to specific brain regions have selective responses to drugs of abuse (Maroteaux and Mameli, 2012; Good et al., 2013).
Does Drug Withdrawal Alter LHb Neuronal Function?
LHb neuronal firing rates (measured in vivo) are decreased shortly after cocaine administration (0–10 min post injection), but this transient inhibition progresses to increased firing activity (15–35 min post injection) in specific neuronal populations (~30% of the recorded neurons; Jhou et al., 2013), which parallels cocaine’s immediately rewarding, but later aversive, sensations (Ettenberg et al., 1999). In ex vivo electrophysiological measurements, a subpopulation of LHb neurons shows decreases in excitability during cocaine bath application, which then progresses to increases in excitability above the original baseline after cocaine is washed out (Jhou et al., 2013). These results are in agreement with the observed in vivo biphasic LHb neuronal firing patterns that occur in a subset of neurons during intravenous cocaine administration (Dougherty et al., 1990; Jhou et al., 2013).
In addition to the effects on LHb neurons following acute cocaine exposure, withdrawal from short-access (2 h/day) cocaine self-administration causes increases in LHb neuron excitability as measured using ex vivo electrophysiology (Neumann et al., 2014). In animals after 5–7 days of withdrawal from cocaine self-administration, regular spiking LHb neurons have a significantly higher membrane excitability compared to saline-exposed controls (Neumann et al., 2014), and the increased membrane excitability returns to basal levels by withdrawal day 45 (Neumann et al., 2014).
In addition to changes in LHb neuronal firing induced by withdrawal from drugs of abuse, synaptic changes at inhibitory and excitatory synapses are also detected. On withdrawal day 2 or 14 following 5 days of passive cocaine administration, GABAAR-mediated inhibition is decreased at entopeduncular nucleus (EPN)-to-LHb neuronal synapses (measured in brain slices) (Ishikawa and Kenny, 2016; Meye et al., 2016; Figure 1). Furthermore, these EPN-targeted LHb neurons preferentially innervate midbrain (VTA or substantia nigra (SNr)) GABAergic neurons that encode aversion, and normalizing the GABAergic neurotransmission at EPN-to-LHb synapses prevents stress-induced reinstatement to cocaine-associated contexts (Meye et al., 2016).
At excitatory synapses, increases in miniature EPSC (mEPSC) amplitude are observed along with increases in the ratio of currents mediated by AMPA- and NMDA-type glutamate receptors (AMPAR/NMDAR) onto LHb neurons that specifically project to the RMTg (but not the VTA; Maroteaux and Mameli, 2012). This increase in mEPSC amplitude and in AMPAR/NMDAR ratio is likely due to increases in the number of synaptically-expressed AMPARs at this cocaine-withdrawal time point (24 h after two consecutive cocaine intraperitoneal injections; Maroteaux and Mameli, 2012). With cocaine-induced increases in AMPAR levels, the subpopulation of LHb neurons projecting to the RMTg becomes sensitized to synaptic activity and undergoes long-term synaptic potentiation (Maroteaux and Mameli, 2012). These results suggest that specific subpopulations of LHb neurons, with respect to their projection targets, are more sensitive to incoming signals, potentially amplifying select neuronal outputs during cocaine withdrawal.
Increases in LHb neuronal activity are not uniquely correlated with cocaine-induced withdrawal, but are also observed following exposure to ethanol. Withdrawal from ethanol (20% ethanol in a water bottle given intermittently 24 times over 8 weeks) increases spontaneous action potential firing and membrane excitability in LHb neurons measured using ex vivo brain slices (Li et al., 2016, 2017). At the synaptic level, the AMPAR/NMDAR ratio and the frequency of sEPSCs are increased during ethanol withdrawal (Li et al., 2016, 2017). Additionally, in an ethanol-induced conditioned taste aversion (CTA) paradigm, the basal firing in LHb neurons is increased in vivo when compared to neuronal firing pre-CTA (Tandon et al., 2017).
These results suggest that the increased LHb activity may serve as a neural mechanism to facilitate drug-induced negative affect. Therefore, inhibiting LHb neuronal output could potentially prevent drug-induced anhedonia. To this end, low dose (0.25 g/kg) ethanol-induced conditioned place aversion is reversed to conditioned place preference (CPP) following lidocaine-induced LHb inhibition (Zuo et al., 2017a). In addition, ethanol-induced CTA is attenuated in animals with LHb lesions (Haack et al., 2014; Tandon et al., 2017). Furthermore, cocaine-induced avoidance behaviors in a runway operant paradigm are abolished in animals with lesioned LHb efferents (Jhou et al., 2013).
In summary, withdrawal from drugs of abuse facilitates a correlative response between the intrinsic and synaptic properties of LHb neurons, in that increases in action potential firing and membrane excitability are associated with enhanced glutamatergic transmission and a reduced GABAergic influence. Based on the responses of LHb neurons during localized drug application and during withdrawal, LHb neurons are responsive to drugs of abuse. How these drugs of abuse affect reward learning via the LHb becomes a potentially critical topic to explore.
How Do Drugs of Abuse Alter LHb-Regulated Reward Prediction or Reward Learning?
The LHb is necessary for updating drug-induced learned behaviors as well as discriminating between behaviors that elicit reward. For example, lesions in the LHb prior to extinction training prevent extinction learning in animals trained to self-administer cocaine (Friedman et al., 2010). Additionally, LHb lesions block an animal’s ability to discriminate between rewarding and non-rewarding predictive cues. This was shown in animals that learned to press an active lever during cocaine availability (signaled by a light cue; Go) and to abstain from lever pressing when cocaine was not available (signaled by the absence of a light cue; No Go; Zapata et al., 2017). Animals with inhibited LHb function showed an inability to discriminate between the predictive cues and continued to press the lever during the No Go cue despite the absence of available reward (Zapata et al., 2017). Furthermore, pharmacologically inhibiting LHb function had no effect on the motivation to seek cocaine, which, in addition to the impaired predictive-cue discrimination mentioned above, led the authors to interpret that the LHb may not only mediate reward updating, but also facilitate suppression of impulsive behaviors (Zapata et al., 2017).
Clinical studies show that patients suffering from cocaine-use disorder have impaired reward learning, compared to healthy controls (Parvaz et al., 2015; Ersche et al., 2016), which can be mediated by changes in the neurocircuits underlying reward prediction (Wrase et al., 2007; Sjoerds et al., 2013; Rose et al., 2014; Deserno et al., 2015). These reward-learning deficits are also observed in preclinical models of addiction which show that reward learning is impaired by drugs of abuse following non-contingent drug administration (Groman et al., 2018) and, as discussed below, contingent drug administration.
In the extinction phase of drug self-administration, the drug reward is withheld after performing the learned behavioral response (a negative RPE), likely invoking negative RPE signaling in the LHb and subsequent LHb-induced dopamine neuron inhibition (Figure 2). Therefore, if exposure to drugs of abuse alters LHb function, negative RPE signaling in the LHb would be impaired, thus affecting extinction learning. Animals trained to self-administer drugs of abuse, including psychostimulants, opioids and ethanol, are able to extinguish this learned response (Grimm and See, 2000; Shalev et al., 2001; Richards et al., 2008). This conclusion is not drawn from direct statistical measures, but rather on the clear separation of data points observed between the acquisition phase and the extinction phase of drug self-administration. However, the ability to extinguish a learned response does not imply that extinction learning is fully intact. There are clear time-dependent changes in extinction behavior following withdrawal from heroin and cocaine (this effect is also seen following extinction of sucrose self-administration; Shalev et al., 2001; Grimm et al., 2002), which may rely on changes in neuronal activity of LHb afferents targeting the VTA (Mahler and Aston-Jones, 2012). Future experiments that directly link LHb function and drug-induced extinction learning are still needed in order to demonstrate that the LHb is altered following drug exposure, and that these drug-induced modifications in the LHb are causally associated with reward-learning impairments. Doing so may provide direct evidence that drug-induced modifications in the LHb impair extinction learning and promote relapse to drugs of abuse.
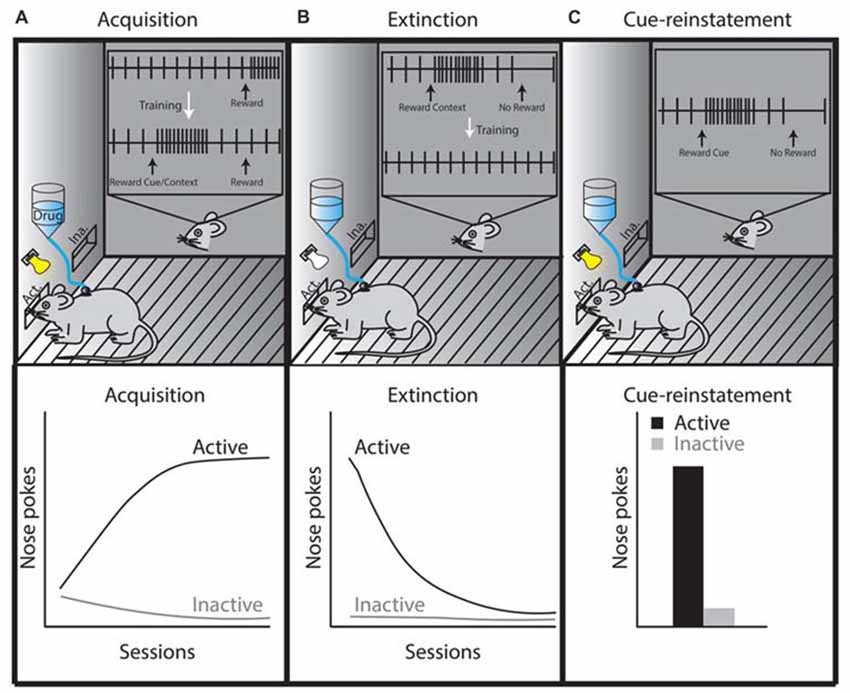
Figure 2. Theoretical illustration of dopamine neuron activity during three different self-administration phases, which is based on phasic dopamine release that occurs in the NAc during drug self-administration, extinction, or reinstatement (Phillips et al., 2003; Stuber et al., 2005). (A) In the acquisition or maintenance phases, animals are placed in a specific context in which they learn that active lever presses or nose pokes elicit intravenous drug administration (this is sometimes paired with a cue, which becomes associated with the rewarding properties of the drug). Once acquired, as shown by steady increases in daily active lever or nose pokes (lower left graph, black line), the maintenance phase is initiated and the animals’ active lever presses or nose pokes reach a plateau. The inset in panel (A) shows the activity of a midbrain dopamine neuron during drug self-administration. The firing of the dopamine neuron is precisely timed with reward prediction errors (RPEs) such that an unexpected delivery of drug elicits phasic dopamine neuron firing. When these rewards are repeatedly preceded by a cue, phasic dopamine neuron firing shifts from occurring at the time of reward to occurring at the time of exposure to the reward-predicting cue. (B) The extinction phase refers to extinguishing the behavior learned during the acquisition/maintenance phase (lower middle graph, black line), which occurs by eliminating drug delivery after the animal completes a learned behavioral response to obtain the drug (e.g., an active lever press or nose poke). This behavior is performed in the absence of a cue in tests requiring cue-reinstatement. The inset in panel (B) shows that omitting the expected reward (negative RPE) inhibits dopamine neuron firing. However, drug-associated contexts (i.e., house light, lever or nose poke hole) are likely to induce phasic firing of dopamine neurons until these learned associations are updated during extinction training (McFarland and Ettenberg, 1997; Crombag and Shaham, 2002; Stuber et al., 2005). (C) Drug-associated cues reinstate the learned behavior of active nose pokes (lower right graph, panel C). If the reward is omitted following the cue presentation (negative RPE), the tonic firing of dopamine-neurons would be inhibited at the time the reward is expected (inset, panel C). If this reward omission is repeated, dopamine neurons no longer show phasic firing patterns during reward-predicting cue re-exposure (Schultz et al., 1997; Schultz, 2016). The precise timing of dopamine-neuron firing controls cue-reward learning making the phasic firing of dopamine neurons an important neural mechanism responsible for the adaptability of reward-seeking behaviors.
Given that positive RPE is associated with the phasic firing of dopamine neurons, and that negative RPE is associated with LHb signaling, one would expect that the acquisition or maintenance phase of drug self-administration (a positive RPE paradigm) might be independent of LHb control. In agreement with this, it has been shown that LHb lesions do not alter heroin or cocaine self-administration administration during the acquisition or maintenance phases, as LHb lesioned animals seek and obtain similar amounts of drug compared to non-lesioned animals (Wang et al., 2009; Friedman et al., 2010).
Concluding Remarks
The LHb regulates dopamine neuron firing during negative RPE signaling and provides instructive signals for reward-seeking behaviors (Matsumoto and Hikosaka, 2007; Friedman et al., 2010; Bromberg-Martin and Hikosaka, 2011; Zapata et al., 2017). It is hypothesized that drugs of abuse alter positive RPE signals through drug-induced modifications in midbrain dopamine neurons (Redish, 2004; Keiflin and Janak, 2015). In parallel, drugs of abuse may impact negative RPE signals through drug-induced adaptations in the LHb. Evidence suggests that patients suffering from cocaine-use disorder have impaired negative RPE signaling (Parvaz et al., 2015), which is potentially due to the drug-induced modifications in LHb function (based on preclinical findings; Lecca et al., 2014). These drug-induced modifications include changes in membrane excitability, increases or decreases in excitatory transmission, and increases or decreases in inhibitory transmission, which have lasting impacts on LHb neuronal functions during drug abstinence. Since the LHb is important for encoding negative RPE, and drugs of abuse alter LHb functions, it is possible that negative RPEs are compromised in drug-exposed animals. Although there is some circumstantial evidence that drugs of abuse may affect extinction learning in preclinical models of addiction, direct causal associations between drug-induced LHb modifications and LHb-regulated negative RPE signaling are still lacking.
In addition to regulating negative RPE, the LHb is critically involved in learning and memory processes that are associated with avoidance behaviors (Stamatakis and Stuber, 2012), which are also disrupted in patients suffering from cocaine-use disorder (Ersche et al., 2016). The neuronal mechanisms underlying the impairment of avoidance behaviors are unknown. Preclinical models have shown that psychostimulants facilitate axonal degeneration of the fasciculus retroflexus (a collection of efferent LHb fibers; Carlson et al., 2001; Ellison, 2002; Ciani et al., 2005; Lax et al., 2013), limiting the LHb’s regulation over downstream targets. However, this axonal degeneration has only been detected in animals exposed to psychostimulants; it is unclear whether axonal degeneration of the fasciculus retroflexus is common among other abused substances. Additionally, these avoidance behaviors may be linked to the LHb’s regulation of anxiety disorders through its efferent connections to serotonin neurons in the dorsal raphe nucleus, another brain region signaling reward-context information with implications in addiction (Nakamura, 2013; Müller and Homberg, 2015).
In conclusion, we reviewed evidence supporting the importance of the LHb in regulating reward learning and updating of reward-related information. In preclinical experiments, lesioning the LHb or inhibiting LHb function elicits similar impairments in reward prediction when compared to human patients suffering from drug abuse. However, more work is needed in animal models of drug abuse to understand whether and how drugs of abuse facilitate reward-related learning impairments mediated by LHb function.
Author Contributions
NG, PN and YD developed the focus of this review. NG and PN wrote the manuscript.
Conflict of Interest Statement
The authors declare that the research was conducted in the absence of any commercial or financial relationships that could be construed as a potential conflict of interest.
Acknowledgments
We would like to thank Dr. Diane McCloskey for her thoughtful input provided during the writing of this manuscript.
References
Aizawa, H., Amo, R., and Okamoto, H. (2011). Phylogeny and ontogeny of the habenular structure. Front. Neurosci. 5:138. doi: 10.3389/fnins.2011.00138
Amat, J., Sparks, P. D., Matus-Amat, P., Griggs, J., Watkins, L. R., and Maier, S. F. (2001). The role of the habenular complex in the elevation of dorsal raphe nucleus serotonin and the changes in the behavioral responses produced by uncontrollable stress. Brain Res. 917, 118–126. doi: 10.1016/s0006-8993(01)02934-1
Boulos, L. J., Darcq, E., and Kieffer, B. L. (2017). Translating the habenula-from rodents to humans. Biol. Psychiatry 81, 296–305. doi: 10.1016/j.biopsych.2016.06.003
Brischoux, F., Chakraborty, S., Brierley, D. I., and Ungless, M. A. (2009). Phasic excitation of dopamine neurons in ventral VTA by noxious stimuli. Proc. Natl. Acad. Sci. U S A 106, 4894–4899. doi: 10.1073/pnas.0811507106
Bromberg-Martin, E. S., and Hikosaka, O. (2011). Lateral habenula neurons signal errors in the prediction of reward information. Nat. Neurosci. 14, 1209–1216. doi: 10.1038/nn.2902
Bromberg-Martin, E. S., Matsumoto, M., and Hikosaka, O. (2010). Dopamine in motivational control: rewarding, aversive, and alerting. Neuron 68, 815–834. doi: 10.1016/j.neuron.2010.11.022
Carlson, J., Noguchi, K., and Ellison, G. (2001). Nicotine produces selective degeneration in the medial habenula and fasciculus retroflexus. Brain Res. 906, 127–134. doi: 10.1016/s0006-8993(01)02570-7
Chang, C. Y., Esber, G. R., Marrero-Garcia, Y., Yau, H. J., Bonci, A., and Schoenbaum, G. (2016). Brief optogenetic inhibition of dopamine neurons mimics endogenous negative reward prediction errors. Nat. Neurosci. 19, 111–116. doi: 10.1038/nn.4191
Chang, C. Y., Gardner, M., Di Tillio, M. G., and Schoenbaum, G. (2017). Optogenetic blockade of dopamine transients prevents learning induced by changes in reward features. Curr. Biol. 27, 3480.e3–3486.e3. doi: 10.1016/j.cub.2017.09.049
Christoph, G. R., Leonzio, R. J., and Wilcox, K. S. (1986). Stimulation of the lateral habenula inhibits dopamine-containing neurons in the substantia nigra and ventral tegmental area of the rat. J. Neurosci. 6, 613–619. doi: 10.1523/JNEUROSCI.06-03-00613.1986
Ciani, E., Severi, S., Bartesaghi, R., and Contestabile, A. (2005). Neurochemical correlates of nicotine neurotoxicity on rat habenulo-interpeduncular cholinergic neurons. Neurotoxicology 26, 467–474. doi: 10.1016/j.neuro.2005.04.001
Crombag, H. S., and Shaham, Y. (2002). Renewal of drug seeking by contextual cues after prolonged extinction in rats. Behav. Neurosci. 116, 169–173. doi: 10.1037//0735-7044.116.1.169
Deserno, L., Beck, A., Huys, Q. J., Lorenz, R. C., Buchert, R., Buchholz, H. G., et al. (2015). Chronic alcohol intake abolishes the relationship between dopamine synthesis capacity and learning signals in the ventral striatum. Eur. J. Neurosci. 41, 477–486. doi: 10.1111/ejn.12802
Dougherty, P. M., Qiao, J. T., Wiggins, R. C., and Dafny, N. (1990). Microiontophoresis of cocaine, desipramine, sulpiride, methysergide, and naloxone in habenula and parafasciculus. Exp. Neurol. 108, 241–246. doi: 10.1016/0014-4886(90)90129-g
Ellison, G. (2002). Neural degeneration following chronic stimulant abuse reveals a weak link in brain, fasciculus retroflexus, implying the loss of forebrain control circuitry. Eur. Neuropsychopharmacol. 12, 287–297. doi: 10.1016/s0924-977x(02)00020-2
Ersche, K. D., Gillan, C. M., Jones, P. S., Williams, G. B., Ward, L. H., Luijten, M., et al. (2016). Carrots and sticks fail to change behavior in cocaine addiction. Science 352, 1468–1471. doi: 10.1126/science.aaf3700
Ettenberg, A., Raven, M. A., Danluck, D. A., and Necessary, B. D. (1999). Evidence for opponent-process actions of intravenous cocaine. Pharmacol. Biochem. Behav. 64, 507–512. doi: 10.1016/s0091-3057(99)00109-4
Evely, K. M., Hudson, R. L., Dubocovich, M. L., and Haj-Dahmane, S. (2016). Melatonin receptor activation increases glutamatergic synaptic transmission in the rat medial lateral habenula. Synapse 70, 181–186. doi: 10.1002/syn.21892
Friedman, A., Lax, E., Dikshtein, Y., Abraham, L., Flaumenhaft, Y., Sudai, E., et al. (2010). Electrical stimulation of the lateral habenula produces enduring inhibitory effect on cocaine seeking behavior. Neuropharmacology 59, 452–459. doi: 10.1016/j.neuropharm.2010.06.008
Good, C. H., Wang, H., Chen, Y. H., Mejias-Aponte, C. A., Hoffman, A. F., and Lupica, C. R. (2013). Dopamine D4 receptor excitation of lateral habenula neurons via multiple cellular mechanisms. J. Neurosci. 33, 16853–16864. doi: 10.1523/JNEUROSCI.1844-13.2013
Grimm, J. W., and See, R. E. (2000). Dissociation of primary and secondary reward-relevant limbic nuclei in an animal model of relapse. Neuropsychopharmacology 22, 473–479. doi: 10.1016/s0893-133x(99)00157-8
Grimm, J. W., Shaham, Y., and Hope, B. T. (2002). Effect of cocaine and sucrose withdrawal period on extinction behavior, cue-induced reinstatement, and protein levels of the dopamine transporter and tyrosine hydroxylase in limbic and cortical areas in rats. Behav. Pharmacol. 13, 379–388. doi: 10.1097/00008877-200209000-00011
Groman, S. M., Rich, K. M., Smith, N. J., Lee, D., and Taylor, J. R. (2018). Chronic exposure to methamphetamine disrupts reinforcement-based decision-making in rats. Neuropsychopharmacology 43, 770–780. doi: 10.1038/npp.2017.159
Haack, A. K., Sheth, C., Schwager, A. L., Sinclair, M. S., Tandon, S., and Taha, S. A. (2014). Lesions of the lateral habenula increase voluntary ethanol consumption and operant self-administration, block yohimbine-induced reinstatement of ethanol seeking and attenuate ethanol-induced conditioned taste aversion. PLoS One 9:e92701. doi: 10.1371/journal.pone.0092701
Hikosaka, O. (2010). The habenula: from stress evasion to value-based decision-making. Nat. Rev. Neurosci. 11, 503–513. doi: 10.1038/nrn2866
Hong, S., and Hikosaka, O. (2008). The globus pallidus sends reward-related signals to the lateral habenula. Neuron 60, 720–729. doi: 10.1016/j.neuron.2008.09.035
Ishikawa, M., and Kenny, P. J. (2016). Crash course in pallidus-habenula signaling. Nat. Neurosci. 19, 981–983. doi: 10.1038/nn.4349
Jean-Richard Dit Bressel, P., and McNally, G. P. (2014). The role of the lateral habenula in punishment. PLoS One 9:e111699. doi: 10.1371/journal.pone.0111699
Jhou, T. C., Geisler, S., Marinelli, M., Degarmo, B. A., and Zahm, D. S. (2009). The mesopontine rostromedial tegmental nucleus: a structure targeted by the lateral habenula that projects to the ventral tegmental area of Tsai and substantia nigra compacta. J. Comp. Neurol. 513, 566–596. doi: 10.1002/cne.21891
Jhou, T. C., Good, C. H., Rowley, C. S., Xu, S. P., Wang, H., Burnham, N. W., et al. (2013). Cocaine drives aversive conditioning via delayed activation of dopamine-responsive habenular and midbrain pathways. J. Neurosci. 33, 7501–7512. doi: 10.1523/JNEUROSCI.3634-12.2013
Ji, H., and Shepard, P. D. (2007). Lateral habenula stimulation inhibits rat midbrain dopamine neurons through a GABAA receptor-mediated mechanism. J. Neurosci. 27, 6923–6930. doi: 10.1523/JNEUROSCI.0958-07.2007
Kaufling, J., Veinante, P., Pawlowski, S. A., Freund-Mercier, M. J., and Barrot, M. (2009). Afferents to the GABAergic tail of the ventral tegmental area in the rat. J. Comp. Neurol. 513, 597–621. doi: 10.1002/cne.21983
Keiflin, R., and Janak, P. H. (2015). Dopamine prediction errors in reward learning and addiction: from theory to neural circuitry. Neuron 88, 247–263. doi: 10.1016/j.neuron.2015.08.037
Kelley, A. E. (2005). “Neurochemical networks encoding emotion and motivation: an evolutionary perspective,” in Who Needs Emotions?: The Brain Meets the Robot, eds J.-M. Fellous and M. A. Arbib (New York, NY: Oxford University Press), 29–77.
Lammel, S., Lim, B. K., Ran, C., Huang, K. W., Betley, M. J., Tye, K. M., et al. (2012). Input-specific control of reward and aversion in the ventral tegmental area. Nature 491, 212–217. doi: 10.1038/nature11527
Lammel, S., Steinberg, E. E., Földy, C., Wall, N. R., Beier, K., Luo, L., et al. (2015). Diversity of transgenic mouse models for selective targeting of midbrain dopamine neurons. Neuron 85, 429–438. doi: 10.1016/j.neuron.2014.12.036
Lax, E., Friedman, A., Croitoru, O., Sudai, E., Ben-Moshe, H., Redlus, L., et al. (2013). Neurodegeneration of lateral habenula efferent fibers after intermittent cocaine administration: implications for deep brain stimulation. Neuropharmacology 75, 246–254. doi: 10.1016/j.neuropharm.2013.06.034
Lecca, S., Meye, F. J., and Mameli, M. (2014). The lateral habenula in addiction and depression: an anatomical, synaptic and behavioral overview. Eur. J. Neurosci. 39, 1170–1178. doi: 10.1111/ejn.12480
Lecourtier, L., and Kelly, P. H. (2007). A conductor hidden in the orchestra? Role of the habenular complex in monoamine transmission and cognition. Neurosci. Biobehav. Rev. 31, 658–672. doi: 10.1016/j.neubiorev.2007.01.004
Li, J., Kang, S., Fu, R., Wu, L., Wu, W., Liu, H., et al. (2017). Inhibition of AMPA receptor and CaMKII activity in the lateral habenula reduces depressive-like behavior and alcohol intake in rats. Neuropharmacology 126, 108–120. doi: 10.1016/j.neuropharm.2017.08.035
Li, J., Zuo, W., Fu, R., Xie, G., Kaur, A., Bekker, A., et al. (2016). High frequency electrical stimulation of lateral habenula reduces voluntary ethanol consumption in rats. Int. J. Neuropsychopharmacol. 19:pyw050. doi: 10.1093/ijnp/pyw050
Mahler, S. V., and Aston-Jones, G. S. (2012). Fos activation of selective afferents to ventral tegmental area during cue-induced reinstatement of cocaine seeking in rats. J. Neurosci. 32, 13309–13326. doi: 10.1523/JNEUROSCI.2277-12.2012
Maroteaux, M., and Mameli, M. (2012). Cocaine evokes projection-specific synaptic plasticity of lateral habenula neurons. J. Neurosci. 32, 12641–12646. doi: 10.1523/JNEUROSCI.2405-12.2012
Matsumoto, M., and Hikosaka, O. (2007). Lateral habenula as a source of negative reward signals in dopamine neurons. Nature 447, 1111–1115. doi: 10.1038/nature05860
McFarland, K., and Ettenberg, A. (1997). Reinstatement of drug-seeking behavior produced by heroin-predictive environmental stimuli. Psychopharmacology 131, 86–92. doi: 10.1007/s002130050269
Meye, F. J., Soiza-Reilly, M., Smit, T., Diana, M. A., Schwarz, M. K., and Mameli, M. (2016). Shifted pallidal co-release of GABA and glutamate in habenula drives cocaine withdrawal and relapse. Nat. Neurosci. 19, 1019–1024. doi: 10.1038/nn.4334
Müller, C. P., and Homberg, J. R. (2015). The role of serotonin in drug use and addiction. Behav. Brain Res. 277, 146–192. doi: 10.1016/j.bbr.2014.04.007
Nakamura, K. (2013). The role of the dorsal raphe nucleus in reward-seeking behavior. Front. Integr. Neurosci. 7:60. doi: 10.3389/fnint.2013.00060
Neumann, P. A., Ishikawa, M., Otaka, M., Huang, Y. H., Schlüter, O. M., and Dong, Y. (2014). Increased excitability of lateral habenula neurons in adolescent rats following cocaine self-administration. Int. J. Neuropsychopharmacol. 18:pyu109. doi: 10.1093/ijnp/pyu109
Parvaz, M. A., Konova, A. B., Proudfit, G. H., Dunning, J. P., Malaker, P., Moeller, S. J., et al. (2015). Impaired neural response to negative prediction errors in cocaine addiction. J. Neurosci. 35, 1872–1879. doi: 10.1523/JNEUROSCI.2777-14.2015
Phillips, P. E., Stuber, G. D., Heien, M. L., Wightman, R. M., and Carelli, R. M. (2003). Subsecond dopamine release promotes cocaine seeking. Nature 422, 614–618. doi: 10.1038/nature01476
Pobbe, R. L., and Zangrossi, H. Jr. (2008). Involvement of the lateral habenula in the regulation of generalized anxiety- and panic-related defensive responses in rats. Life Sci. 82, 1256–1261. doi: 10.1016/j.lfs.2008.04.012
Quina, L. A., Tempest, L., Ng, L., Harris, J. A., Ferguson, S., Jhou, T. C., et al. (2015). Efferent pathways of the mouse lateral habenula. J. Comp. Neurol. 523, 32–60. doi: 10.1002/cne.23662
Redish, A. D. (2004). Addiction as a computational process gone awry. Science 306, 1944–1947. doi: 10.1126/science.1102384
Richards, J. K., Simms, J. A., Steensland, P., Taha, S. A., Borgland, S. L., Bonci, A., et al. (2008). Inhibition of orexin-1/hypocretin-1 receptors inhibits yohimbine-induced reinstatement of ethanol and sucrose seeking in Long-Evans rats. Psychopharmacology 199, 109–117. doi: 10.1007/s00213-008-1136-5
Rose, E. J., Salmeron, B. J., Ross, T. J., Waltz, J., Schweitzer, J. B., McClure, S. M., et al. (2014). Temporal difference error prediction signal dysregulation in cocaine dependence. Neuropsychopharmacology 39, 1732–1742. doi: 10.1038/npp.2014.21
Salas, R., Baldwin, P., de Biasi, M., and Montague, P. R. (2010). BOLD responses to negative reward prediction errors in human habenula. Front. Hum. Neurosci. 4:36. doi: 10.3389/fnhum.2010.00036
Schultz, W. (2017). Reward prediction error. Curr. Biol. 27, R369–r371. doi: 10.1016/j.cub.2017.02.064
Schultz, W., Dayan, P., and Montague, P. R. (1997). A neural substrate of prediction and reward. Science 275, 1593–1599. doi: 10.1126/science.275.5306.1593
Shalev, U., Morales, M., Hope, B., Yap, J., and Shaham, Y. (2001). Time-dependent changes in extinction behavior and stress-induced reinstatement of drug seeking following withdrawal from heroin in rats. Psychopharmacology 156, 98–107. doi: 10.1007/s002130100748
Shelton, L., Becerra, L., and Borsook, D. (2012). Unmasking the mysteries of the habenula in pain and analgesia. Prog. Neurobiol. 96, 208–219. doi: 10.1016/j.pneurobio.2012.01.004
Sjoerds, Z., de Wit, S., van den Brink, W., Robbins, T. W., Beekman, A. T., Penninx, B. W., et al. (2013). Behavioral and neuroimaging evidence for overreliance on habit learning in alcohol-dependent patients. Transl. Psychiatry 3:e337. doi: 10.1038/tp.2013.107
Stamatakis, A. M., Jennings, J. H., Ung, R. L., Blair, G. A., Weinberg, R. J., Neve, R. L., et al. (2013). A unique population of ventral tegmental area neurons inhibits the lateral habenula to promote reward. Neuron 80, 1039–1053. doi: 10.1016/j.neuron.2013.08.023
Stamatakis, A. M., and Stuber, G. D. (2012). Activation of lateral habenula inputs to the ventral midbrain promotes behavioral avoidance. Nat. Neurosci. 15, 1105–1107. doi: 10.1038/nn.3145
Steinberg, E. E., Keiflin, R., Boivin, J. R., Witten, I. B., Deisseroth, K., and Janak, P. H. (2013). A causal link between prediction errors, dopamine neurons and learning. Nat. Neurosci. 16, 966–973. doi: 10.1038/nn.3413
Stuber, G. D., Wightman, R. M., and Carelli, R. M. (2005). Extinction of cocaine self-administration reveals functionally and temporally distinct dopaminergic signals in the nucleus accumbens. Neuron 46, 661–669. doi: 10.1016/j.neuron.2005.04.036
Tandon, S., Keefe, K. A., and Taha, S. A. (2017). Excitation of lateral habenula neurons as a neural mechanism underlying ethanol-induced conditioned taste aversion. J. Physiol. 595, 1393–1412. doi: 10.1113/JP272994
Thornton, E. W., and Bradbury, G. E. (1989). Effort and stress influence the effect of lesion of the habenula complex in one-way active avoidance learning. Physiol. Behav. 45, 929–935. doi: 10.1016/0031-9384(89)90217-5
Thornton, E. W., Evans, J. A., and Harris, C. (1985). Attenuated response to nomifensine in rats during a swim test following lesion of the habenula complex. Psychopharmacology 87, 81–85. doi: 10.1007/bf00431783
Tian, J., and Uchida, N. (2015). Habenula lesions reveal that multiple mechanisms underlie dopamine prediction errors. Neuron 87, 1304–1316. doi: 10.1016/j.neuron.2015.08.028
Ullsperger, M., and von Cramon, D. Y. (2003). Error monitoring using external feedback: specific roles of the habenular complex, the reward system, and the cingulate motor area revealed by functional magnetic resonance imaging. J. Neurosci. 23, 4308–4314. doi: 10.1523/JNEUROSCI.23-10-04308.2003
Wallace, M. L., Saunders, A., Huang, K. W., Philson, A. C., Goldman, M., Macosko, E. Z., et al. (2017). Genetically distinct parallel pathways in the entopeduncular nucleus for limbic and sensorimotor output of the basal ganglia. Neuron 94, 138.e5–152.e5. doi: 10.1016/j.neuron.2017.03.017
Wang, Y., Zhang, F., Tang, S., Lai, M., Hao, W., Zhang, Y., et al. (2009). Lack of effect of habenula lesion on heroin self-administration in rats. Neurosci. Lett. 461, 167–171. doi: 10.1016/j.neulet.2009.06.029
Wrase, J., Schlagenhauf, F., Kienast, T., Wüstenberg, T., Bermpohl, F., Kahnt, T., et al. (2007). Dysfunction of reward processing correlates with alcohol craving in detoxified alcoholics. Neuroimage 35, 787–794. doi: 10.1016/j.neuroimage.2006.11.043
Yetnikoff, L., Cheng, A. Y., Lavezzi, H. N., Parsley, K. P., and Zahm, D. S. (2015). Sources of input to the rostromedial tegmental nucleus, ventral tegmental area, and lateral habenula compared: a study in rat. J. Comp. Neurol. 523, 2426–2456. doi: 10.1002/cne.23797
Zapata, A., Hwang, E. K., and Lupica, C. R. (2017). Lateral habenula involvement in impulsive cocaine seeking. Neuropsychopharmacology 42, 1103–1112. doi: 10.1038/npp.2016.286
Zuo, W., Chen, L., Wang, L., and Ye, J. H. (2013). Cocaine facilitates glutamatergic transmission and activates lateral habenular neurons. Neuropharmacology 70, 180–189. doi: 10.1016/j.neuropharm.2013.01.008
Zuo, W., Fu, R., Hopf, F. W., Xie, G., Krnjevic, K., Li, J., et al. (2017a). Ethanol drives aversive conditioning through dopamine 1 receptor and glutamate receptor-mediated activation of lateral habenula neurons. Addict. Biol. 22, 103–116. doi: 10.1111/adb.12298
Zuo, W., Wang, L., Chen, L., Krnjevic, K., Fu, R., Feng, X., et al. (2017b). Ethanol potentiates both GABAergic and glutamatergic signaling in the lateral habenula. Neuropharmacology 113, 178–187. doi: 10.1016/j.neuropharm.2016.09.026
Keywords: lateral habenula, addiction, reward, learning, drugs of abuse, neurocircuits
Citation: Graziane NM, Neumann PA and Dong Y (2018) A Focus on Reward Prediction and the Lateral Habenula: Functional Alterations and the Behavioral Outcomes Induced by Drugs of Abuse. Front. Synaptic Neurosci. 10:12. doi: 10.3389/fnsyn.2018.00012
Received: 29 March 2018; Accepted: 09 May 2018;
Published: 29 May 2018.
Edited by:
Fereshteh S. Nugent, F. Edward Hebert School of Medicine, Uniformed Services University, United StatesReviewed by:
Carl Richard Lupica, National Institute on Drug Abuse (NIDA), United StatesHoward Fields, University of California, San Francisco, United States
Copyright © 2018 Graziane, Neumann and Dong. This is an open-access article distributed under the terms of the Creative Commons Attribution License (CC BY). The use, distribution or reproduction in other forums is permitted, provided the original author(s) and the copyright owner are credited and that the original publication in this journal is cited, in accordance with accepted academic practice. No use, distribution or reproduction is permitted which does not comply with these terms.
*Correspondence: Nicholas M. Graziane, bmdyYXppYW5lQHBlbm5zdGF0ZWhlYWx0aC5wc3UuZWR1