Corrigendum: Protein Interactors and Trafficking Pathways That Regulate the Cannabinoid Type 1 Receptor (CB1R)
- Centre for Synaptic Plasticity, School of Biochemistry, University of Bristol, Bristol, United Kingdom
The endocannabinoid system (ECS) acts as a negative feedback mechanism to suppress synaptic transmission and plays a major role in a diverse range of brain functions including, for example, the regulation of mood, energy balance, and learning and memory. The function and dysfunction of the ECS are strongly implicated in multiple psychiatric, neurological, and neurodegenerative diseases. Cannabinoid type 1 receptor (CB1R) is the most abundant G protein-coupled receptor (GPCR) expressed in the brain and, as for any synaptic receptor, CB1R needs to be in the right place at the right time to respond appropriately to changing synaptic circumstances. While CB1R is found intracellularly throughout neurons, its surface expression is highly polarized to the axonal membrane, consistent with its functional expression at presynaptic sites. Surprisingly, despite the importance of CB1R, the interacting proteins and molecular mechanisms that regulate the highly polarized distribution and function of CB1R remain relatively poorly understood. Here we set out what is currently known about the trafficking pathways and protein interactions that underpin the surface expression and axonal polarity of CB1R, and highlight key questions that still need to be addressed.
Introduction
Information transfer at synapses is almost always mediated by neurotransmitter released from the presynaptic terminal activating specific postsynaptic receptors. The endocannabinoid system (ECS) is exceptional because it is a retrograde negative feedback system (Kreitzer and Regehr, 2001; Ohno-Shosaku et al., 2001; Wilson and Nicoll, 2001). The predominant endocannabinoid transmitter, 2-arachidonoylglycerol (2-AG), is synthesized in the postsynaptic membrane by diacylglycerol lipase-α (DAGLα). 2-AG then diffuses retrogradely across the synaptic cleft to stimulate cannabinoid type 1 receptors (CB1Rs) at the presynaptic membrane (Wilson and Nicoll, 2002; Castillo et al., 2012; Figure 1A). Activation of presynaptic CB1Rs suppresses neurotransmitter release to dampen down synaptic activity (Mackie and Hille, 1992; Yoshida et al., 2006; Chevaleyre et al., 2007; Jung et al., 2007; Uchigashima et al., 2007; Katona and Freund, 2008; Di Marzo, 2011). This unique architecture underpins the ECS function as a “sensor-feedback machine” that modulates synaptic activity.
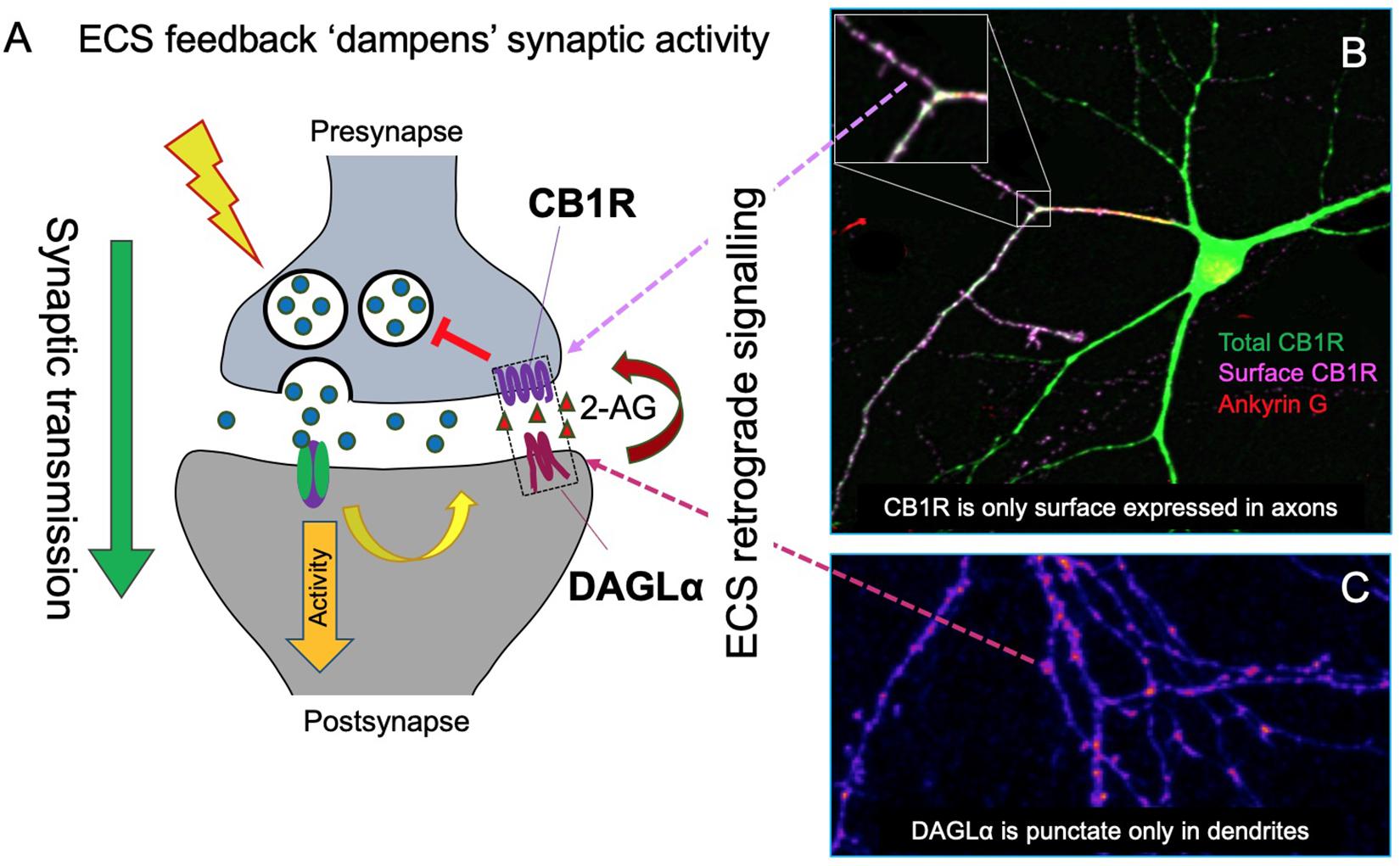
Figure 1. Schematic of the synaptic organization of the ECS. (A) The ECS is highly polarized and unlike nearly all neurotransmitter systems it acts retrogradely. The most abundant endocannabinoid, 2-arachidonoylglycerol (2-AG), is synthesized by the postsynaptic enzyme DAGLα and released to activate presynaptically localized cannabinoid receptors (CB1R) to suppress neurotransmitter release and reduce synaptic activity. (B) Representative image of total and surface expression of N-terminally GFP-tagged CB1R. CB1R is widely distributed in intracellular compartments in neurons, but only stably surface expressed in axons, particularly at the axon terminal. The image shows surface (non-permeabilized, purple) and total (permeabilized, green; colocalization shows as white) CB1R labeled with anti-GFP antibody. The axon was identified by Ankyrin G labeling, red (triple colocalization shows as yellow) (Fletcher-Jones et al. previously unpublished image). (C) Correspondingly, DAGLα is highly localized at the postsynapse. GFP-DAGLα (human) expressed in hippocampal neurons is highly punctate in dendrites consistent with postsynaptic localization (blue = low intensity, purple = high intensity) (Fletcher-Jones et al. previously unpublished image).
The ECS plays key roles in multiple brain functions including synaptic plasticity the cellular basis of learning and memory (Castillo et al., 2012) the central regulation of food intake and energy expenditure (Cardinal et al., 2015) and attenuation of stress-induced glutamate release to counter excitotoxicity (Katona and Freund, 2008). Accordingly, ECS function and dysfunction is strongly implicated in a wide and diverse range of disorders including epilepsy, stroke, dementia, and obesity (Soltesz et al., 2015; Lu and Mackie, 2016; Busquets-Garcia et al., 2018).
Cannabinoid Receptor 1 (Cb1R)
Cannabinoid receptor 1 (CB1R) is a 473 amino acid G protein-coupled receptor (GPCR) highly expressed in the basal ganglia, hippocampus, cerebellum, and cortex (Herkenham et al., 1991). CB1R is a receptor for the endogenous cannabinoids (endocannabinoids) N-arachidonoylethanolamine (anandamide; AEA) and 2-arachidonylglycerol (2-AG) (Kendall and Yudowski, 2016) and mediates most effects of phytocannabinoids, the active components of cannabis, on the CNS.
Cannabinoid type 1 receptor surface expression in neurons is highly polarized to axons and presynaptic sites, where ligand binding results in the downregulation of presynaptic release through coupling to a variety of downstream signaling pathways (Figure 1). In cultured hippocampal neurons, CB1R has a wide intracellular distribution but its plasma membrane surface expression is highly polarized toward the axonal compartment (Figure 1; Irving et al., 2000; Coutts et al., 2001; Leterrier et al., 2006; McDonald et al., 2007a; Fletcher-Jones et al., 2019). CB1R displays a disto-proximal gradient of CB1R expression along the entire axonal plasma membrane (Simon et al., 2013; Wickert et al., 2018) with fine axons reported to exhibit highly punctate surface labeling, often co-localized with sites of synaptic contact (Irving et al., 2000; Coutts et al., 2001). Immunogold electron microscopy analysis of rat brain sections (Katona et al., 1999; Nyiri et al., 2005) and STORM super-resolution imaging (Dudok et al., 2015) detect CB1R predominantly at the presynaptic terminal, concentrated in the plasma membrane at axonal perisynaptic regions of both GABAergic and glutamatergic neurons (Katona et al., 1999, 2006; Kawamura et al., 2006). Notably, small, but functional, sub-populations of CB1R are also observed at the surface of soma and dendrites (Bacci et al., 2004; Marinelli et al., 2009) where they mediate slow self-inhibition, and at mitochondria, where they modulate bioenergetic processes (Benard et al., 2012; Koch et al., 2015). However, how differential targeting of distinct subpopulations to different cellular compartments occurs is unknown, and studies examining the mechanisms that establish and maintain the polarized surface expression of CB1R have provided evidence for contrasting models on how this occurs.
Here we briefly outline CB1R signaling, review the trafficking pathways proposed to mediate CB1R surface expression and axonal polarity, outline the role of the CB1R intracellular C-terminus in mediating these effects, and discuss the known protein interactors of CB1R that contribute to its trafficking and localization.
Cb1R Signaling
Synaptic CB1R Signaling
Endocannabinoid system pharmacology and signaling is complex and is the subject of a number of recent reviews (Araque et al., 2017; Howlett and Abood, 2017; Hunter et al., 2017; Busquets-Garcia et al., 2018). Briefly, CB1R primarily activates Gi proteins, which cause downstream inhibition of cAMP accumulation (Howlett and Fleming, 1984; Howlett, 1987) mediated via the pertussis toxin-sensitive Gi α-subunit inhibition of adenylyl cyclase (Howlett et al., 1986; Howlett, 2004; Figure 2).
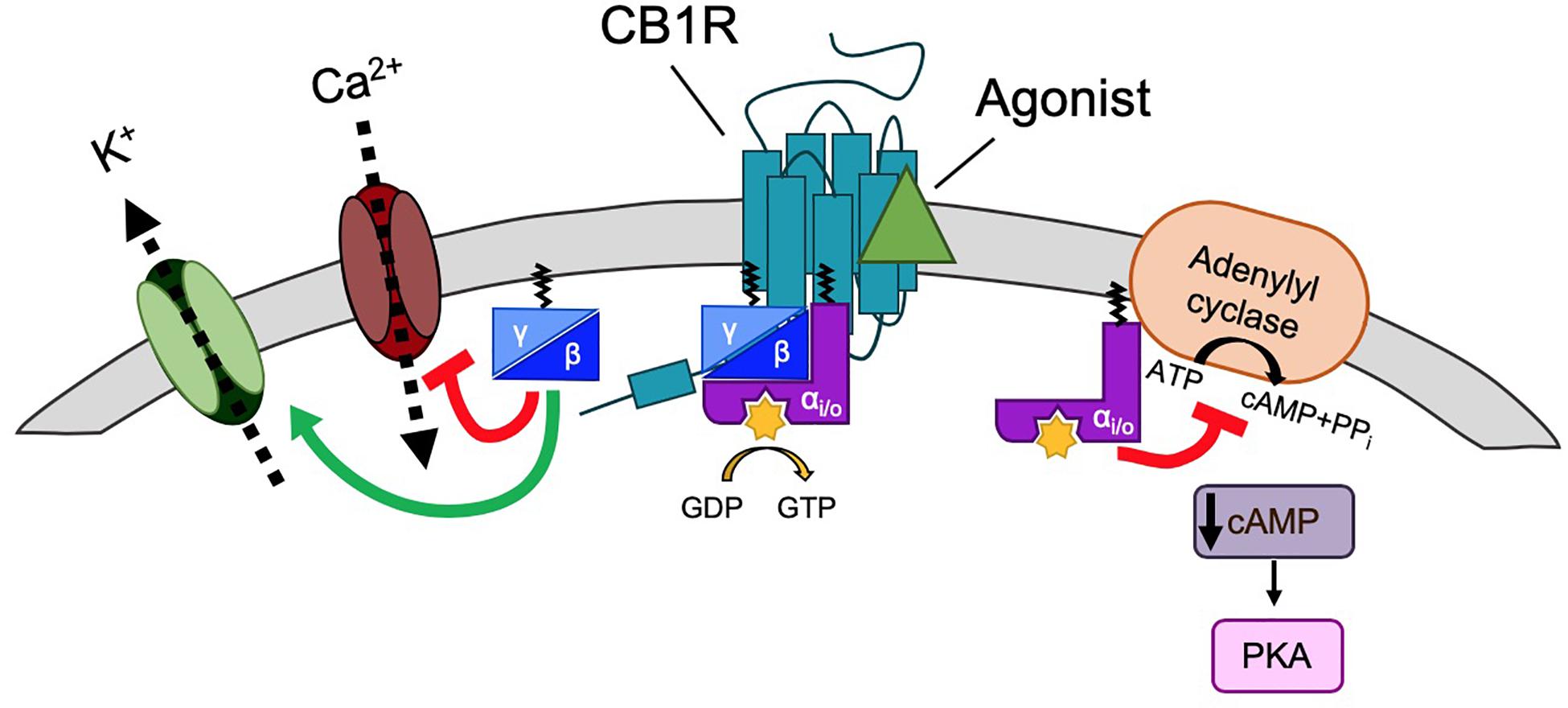
Figure 2. G-protein signaling of CB1R. Classically, CB1R signaling is mediated by Gi/o signaling. CB1R is activated by ligand (green triangle), generating a conformational change that allows for heterotrimeric G-protein (α, β, and γ subunits) binding. The receptor then acts as a guanine nucleotide exchange factor (GEF), activating Gα by GDP-to-GTP exchange. This triggers the dissociation of Gα from Gβγ. Gαi/o inhibits adenylyl cyclase (AC), a membrane protein that catalyzes that conversion of ATP to cAMP+PPi, causing a decrease in cAMP levels and thus inhibiting the PKA phosphorylation pathway. Gβγ inhibits voltage gated Ca2+ channels (VGCCs) and activates G protein-coupled inwardly rectifying K+ channels (GIRKs).
Downstream signaling of CB1R also modulates the activation of a number of kinases. Depletion of cytosolic cAMP levels leads to subsequent inactivation of the protein kinase A (PKA) phosphorylation pathway (Howlett, 2005) whereas the extracellular signal-regulated kinases (ERK), focal adhesion kinases (FAK), c-Jun N-terminal kinase 1/2, and the mitogen-activated protein kinases (MAPK) p42/p44 and p38 are all activated in response to CB1R stimulation (Turu and Hunyady, 2010).
As well as kinase signaling, the classical downstream effects of Gi coupled CB1R activation are increased K+ conductance, through activation of G protein-coupled inward rectifying K+ channels (Kir/GIRK), and decreased Ca2+ conductance, via inhibition of N- and P/Q-type voltage-gated calcium channels (CaV), through interaction with the Gi βγ-subunit (Pan et al., 1996; Twitchell et al., 1997; Figure 2). In addition to Gi/o proteins, CB1R can also couple to Gs, Gq, and G12/13 depending on the cellular/protein context (Busquets-Garcia et al., 2018) for a review. Endocannabinoid activation of astroglial CB1R increases intracellular Ca2+ levels, likely via Gq protein coupling rather than Gi/o (Navarrete and Araque, 2008). Heterodimerisation of CB1R with dopamine D2 receptors can switch the signaling of CB1R and D2 from Gi/o to Gs when co-stimulated, generating the opposing effect: an increase in cAMP levels (Kearn et al., 2005). Different agonists can also elicit different patterns of G-protein coupling, e.g., WIN55212-2, but not Δ9-THC and ACEA, are able to stimulate G12/13 in mouse cortex (Diez-Alarcia et al., 2016).
Endocannabinoid system signaling downstream of CB1R is crucial for both short- and long-term forms of synaptic plasticity. The CB1R-mediated decrease in presynaptic intracellular Ca2+ results in a short-term suppression of inhibition (depolarization-induced suppression of inhibition; DSI) or excitation (DSE), at GABAergic (Wilson and Nicoll, 2001; Ohno-Shosaku et al., 2002) and glutamatergic (Kawamura et al., 2006; Ohno-Shosaku et al., 2007) synapses, respectively, and ECS signaling plays well-established roles in certain forms of long-term depression (LTD) and long-term potentiation (LTP) (Wilson and Nicoll, 2001; Marsicano et al., 2002; Robbe et al., 2002; Chevaleyre and Castillo, 2004; Chevaleyre et al., 2007; Heifets and Castillo, 2009; Puighermanal et al., 2009; Han et al., 2012). Although primarily studied in the hippocampus and cerebellum, ECS signaling has also been implicated in the regulation of cortical, striatal, and limbic circuits (Di Marzo, 2011; Katona and Freund, 2012; Luchicchi and Pistis, 2012).
Signaling From Intracellular CB1R
This review focuses on the trafficking and polarized surface expression of CB1R but, intriguingly, CB1R expressed at the plasma membrane comprises only ∼20% of total CB1R (McIntosh et al., 1998; Leterrier et al., 2006; Rozenfeld and Devi, 2008). Intracellular CB1R is particularly enriched within the endosomal system (McIntosh et al., 1998; Leterrier et al., 2006; Rozenfeld and Devi, 2008). Indeed, since CB1R ligands are lipophilic, and thus membrane permeable, it has been proposed that these endosomal CB1Rs are functional since they associate with Gαi in Rab7-positive late-endosomal fractions (Rozenfeld and Devi, 2008) and the agonist WIN55,212-2 can still induce ERK1/2 phosphorylation even when surface CB1Rs are blocked by the membrane-impermeable antagonist hemopressin (Rozenfeld and Devi, 2008). Consistent with this possibility, intracellular injection of the endocannabinoid anandamide into HEK293 cells expressing CB1R induced Ca2+ release from intracellular stores (Brailoiu et al., 2011). Furthermore, activation of CB1Rs reported to associate with the mitochondrial outer membrane reduce mitochondrial respiration (Benard et al., 2012; Koch et al., 2015) and are implicated in for certain types of memory (Hebert-Chatelain et al., 2016). For recent reviews see Djeungoue-Petga and Hebert-Chatelain (2017), Busquets-Garcia et al. (2018).
Cb1R Traffic Through the Secretory Pathway
ER Membrane Insertion
Cannabinoid type 1 receptor is trafficked to the plasma membrane via the secretory pathway but, like many other GPCRs, CB1R does not have a canonical, cleavable signal peptide (Andersson et al., 2003). Rather, the first transmembrane domain is thought to act as a “reverse anchoring sequence” which is recognized by the signal recognition particle (SRP) Sec61 pathway in the ER membrane (Andersson et al., 2003; Nordstrom and Andersson, 2006). The N-terminal domain is then translocated across the ER membrane in a C-N terminal manner (Rutz et al., 2015) where it is modified by N-linked glycosylation at two sites (N78, N84) [(Song and Howlett, 1995; Nordstrom and Andersson, 2006); unless otherwise stated, all residue numbers used throughout refer to the rat CB1R sequence; Figure 3]. The unusually large size of N-terminal tail of CB1R (117 amino acids) impedes its reverse translocation across the membrane, raising the possibility that a substantial proportion of nascent CB1R could be misfolded and degraded by the proteosome (Andersson et al., 2003; Nordstrom and Andersson, 2006). Indeed, truncation of the large N-terminus, or inclusion of a signal peptide, can greatly enhance receptor stability and cell surface targeting with no effect on agonist binding or signaling [(Andersson et al., 2003; McDonald et al., 2007a) a list of published mutants of CB1R and a brief overview of their effects on trafficking, surface expression and signaling are shown in Table 1]. Endogenous truncation of the N-terminal tail has also been reported, likely occurring during the short amount of time that the N-terminus is exposed to the cytoplasm before translocation (Nordstrom and Andersson, 2006). Interestingly, the first 22 residues of the N-terminus have been reported to constitute a mitochondria targeting sequence (Hebert-Chatelain et al., 2016), suggesting the N-terminus of CB1R represents an important determinant of receptor stability and targeting.
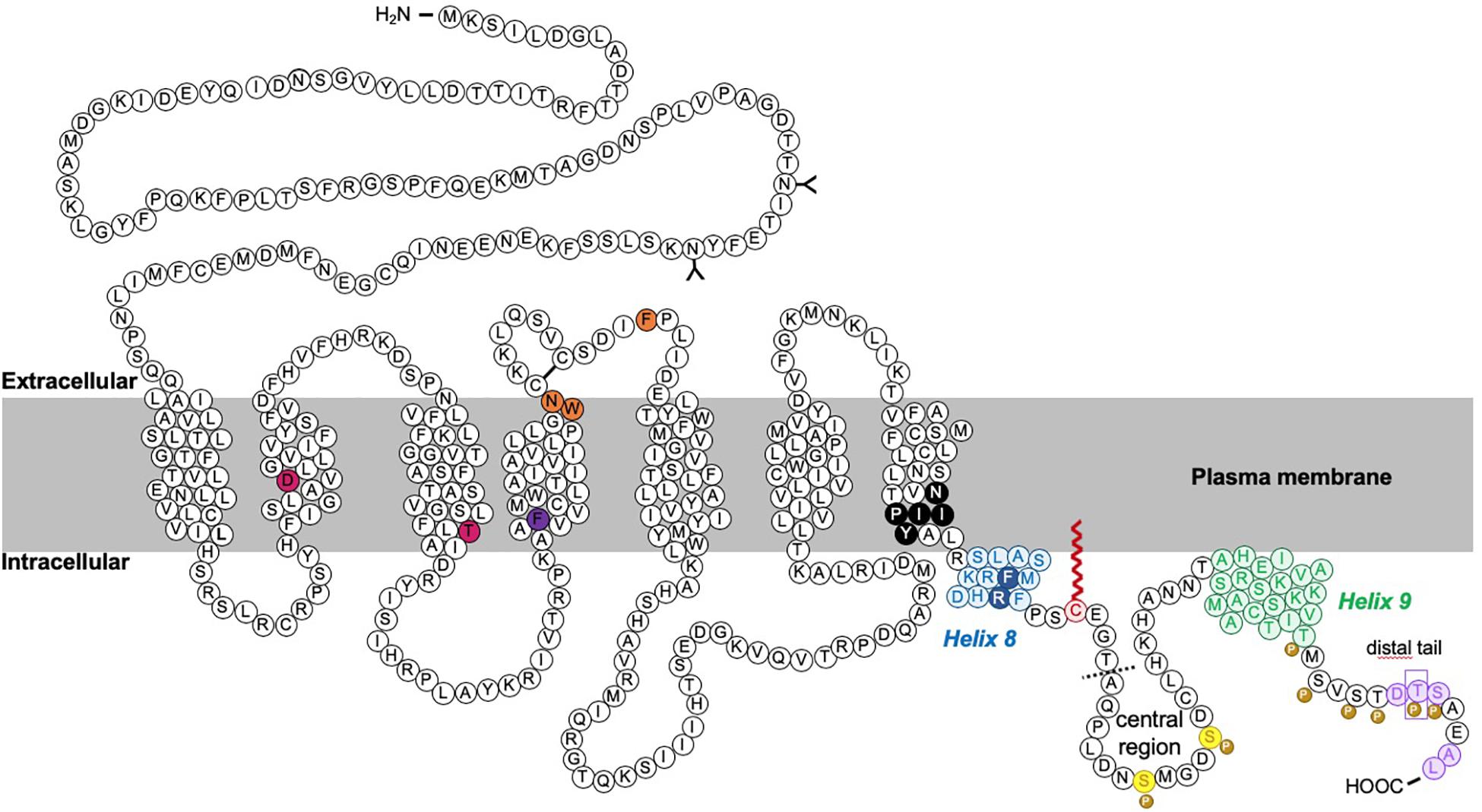
Figure 3. Schematic of rat CB1R. The predicted topology of rat CB1R based on hydrophobicity plots and analysis from crystal structures of human CB1R (97% identity with rat CB1R) and rhodopsin-family GPCRs. Sites of post-translational modification via N-terminal glycosylation (Song and Howlett, 1995) or palmitoylation (Oddi et al., 2018, 2017, 2012) are indicated by the (Y) symbol and a red zigzag line, respectively. Two residues located within the 2nd and 3rd transmembrane domains (TM2/TM3), required for constitutive (Jin et al., 1999) internalization are shown in pink (Roche et al., 1999; D’Antona et al., 2006). Orange residues denote putative amino acids involved in ER exit and surface expression (Ahn et al., 2009a). The F238 residue highlighted in dark purple modulates association with lipid rafts. The black residues indicate the sites of the highly conserved “elbow” shaped NPXXY motif. Within the C-terminal tail, two helical structural motifs, helix 8 (H8) and helix 9 (H9) are highlighted in blue and green, respectively (Ahn et al., 2009b; Stadel et al., 2011). Palmitoylation of C416 (red zigzag line) aids Helix 8 association with the inner leaflet of the plasma membrane. Phosphorylation of two serine residues (yellow) in the central region of the intracellular C-terminus mediate desensitization (Hsieh et al., 1999; Daigle et al., 2008b; Straiker et al., 2012b). However, further phosphorylation of 6 serine/threonine residues in the distal tail (each indicated by the “P” symbol) is required for internalization. GASP1 likely binds the conserved F409/R410 motif in H8, although the distal tail has also been reported to bind GASP1. The motifs required for CRIP1a binding are shown in purple. Phosphorylation of T468 (purple rectangle) decreases affinity for CRIP1a, allowing β-arrestin-2 binding. The exact binding site of SGIP1 is unknown, but it binds downstream of A420 (dotted line). A summary of mutations is provided in Table 1.
TGN Sorting
Selective sorting of proteins to the correct subcellular domain is crucial but, conceptually, establishing axonal polarity is more complex than establishing dendritic polarity since both dendritic and axonal cargos are synthesized in the somatodendritic compartment [although see (Gonzalez et al., 2018; Luarte et al., 2018) regarding the possibility of an axonal local secretory pathway]. The TGN represents an important “hub” in determining polarized protein trafficking, with the target domain of the TGN-derived vesicles acting as a major determinant of the route taken by the cargo to the axonal surface. While several sorting signals and adaptors have been described for dendritic cargo, the mechanisms underpinning polarized sorting of proteins to axons are less well defined (Lasiecka and Winckler, 2011; Bentley and Banker, 2016).
Evidence for Non-polarized Delivery of CB1R From the TGN
Brefeldin A (BFA) reversibly prevents secretory pathway trafficking by disrupting the Golgi (Hunziker et al., 1991; Graham et al., 1993). Using a BFA block and release protocol in cultured neurons to monitor CB1R forward traffic, it has been reported that CB1R is non-discriminately delivered to both the somatodendritic and axonal membrane (Leterrier et al., 2006). Following BFA washout to allow synchronous release of receptors from the Golgi, within 4–8 h more than 50% of neurons exhibited surface expressed CB1R either uniformly throughout the neuron, or on the somatodendritic membrane, suggesting that receptors are sorted from the TGN in a non-polarized manner (Leterrier et al., 2006). 24 h after BFA washout, two-thirds of neurons displayed the expected axonally polarized surface expression. These data were interpreted to indicate that differential rates of constitutive endocytosis in axons versus dendrites lead to the establishment of axonal polarity (see “Differential Internalization of CB1R” section below), rather than polarized trafficking through the secretory pathway (Leterrier et al., 2006; Figure 4A).
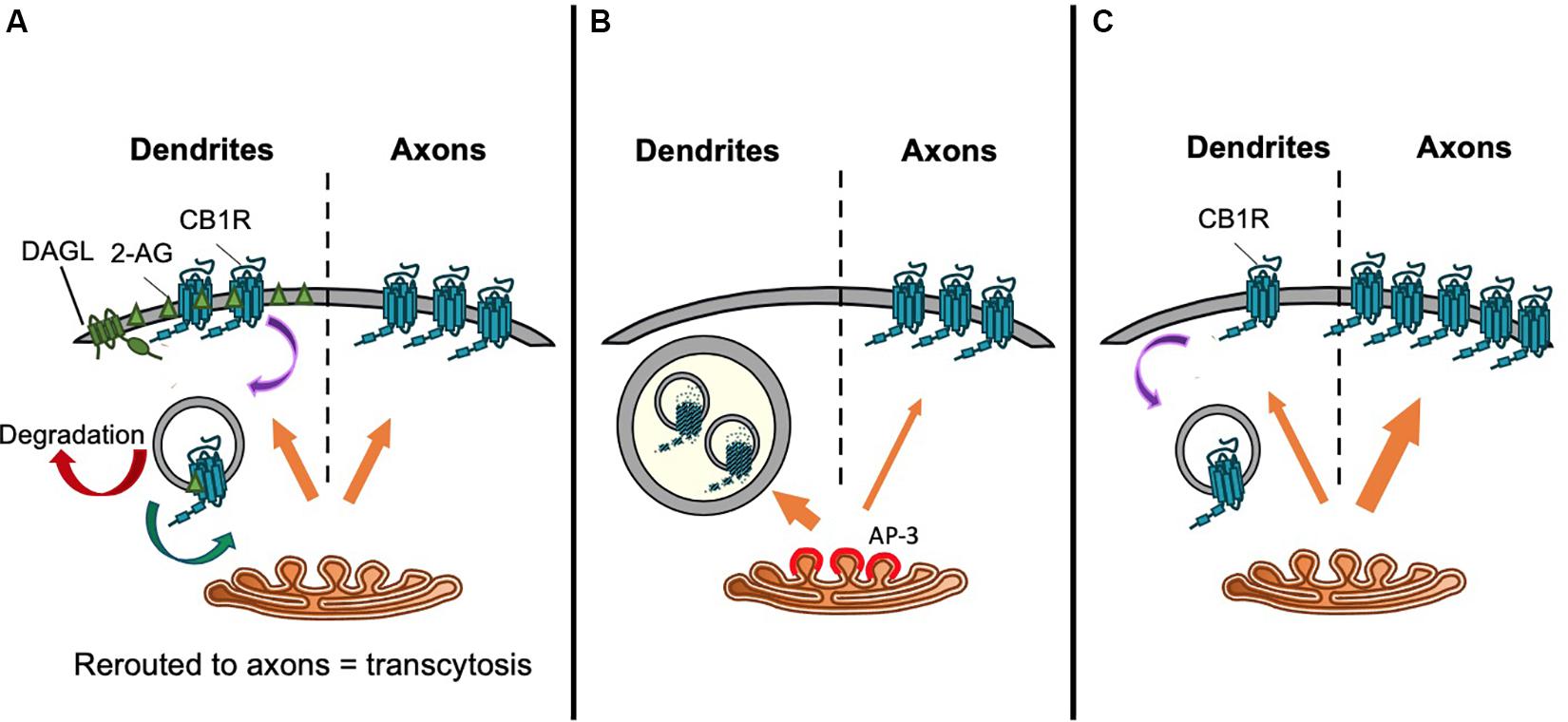
Figure 4. Proposed trafficking pathways that establish CB1R axonal polarization. Schematics representing (A) Model 1: CB1R is delivered to the dendritic and axonal surface indiscriminately. The presence of DAGLα exclusively on the dendritic surface causes a polarized production of 2-AG with high levels in the dendritic membrane. Therefore, de novo CB1R delivered to the dendritic membrane is immediately activated and internalized. Internalized CB1R is either degraded or rerouted to distal end of axons, a process referred to as transcytosis. On the other hand, the absence of DAGLα in the axonal compartment means that newly delivered CB1R is retained on the axonal surface. This results in the axonally polarized distribution, where it is available to be bound by retrogradely crossing endocannabinoids produced under specific synaptic conditions (Leterrier et al., 2006). (B) Model 2: Constitutive sorting to somatodendritic lysosomes. CB1R is constitutively sorted by AP-3 from the TGN to somatodendritic lysosomes. By an unknown mechanism, CB1R can be rescued from degradation and rerouted to axons. It is possible that this mechanism is also AP-3-dependent, since in AP-3 sorts cargo from the TGN to axons in C. elegans (Rozenfeld and Devi, 2008; Li et al., 2016). (C) Model 3. Secretory pathway bias. CB1R is preferentially targeted via the secretory pathway to the axonal membrane. Polarity is enhanced by immediate removal of de novo CB1R from dendritic surface (Fletcher-Jones et al., 2019).
Targeting to Late Endosomes/Lysosomes From the TGN
An alternative suggestion is that CB1R does not reach the somatodendritic surface after egress from the TGN. Rather, CB1R is constitutively targeted primarily to late endosomes/lysosomes (Figure 4B). In the N18TG2 neuroblastoma cell line, newly synthesized CB1R is rapidly degraded without reaching the membrane, with most intracellular CB1R having a half-life of less than 5 h (McIntosh et al., 1998). Moreover, in primary neurons, a significant proportion of endogenous CB1R colocalises with late endosomal/lysosomal markers (Rozenfeld and Devi, 2008). Interestingly, the delta subunit of AP-3, an adaptor complex that mediates trafficking between the TGN and lysosomes (Odorizzi et al., 1998; Scales and Scheller, 1999; Guardia et al., 2018) immunoprecipitates with CB1R, suggesting that CB1R associates with AP-3 (Rozenfeld and Devi, 2008). Indeed, AP-3δ knockdown increased the surface expression of CB1R in both Neuro2A cells and in primary hippocampal neurons (Rozenfeld and Devi, 2008) suggesting that interaction with AP-3δ acts to localize CB1R to late endosomes/lysosomes and restrict somatodendritic surface expression.
AP-3 isoforms have been proposed to play a number of specialized roles in neurons including biogenesis of synaptic vesicles and sorting of transmembrane proteins from endosomes to synaptic vesicles (Nakatsu et al., 2004; Salazar et al., 2004). Moreover, AP-3 may mediate axonal cargo secretory vesicle budding from the TGN, analogous to the better understood role of AP-1 and AP-4 in budding of dendritically destined secretory vesicles (Danglot and Galli, 2007; Li et al., 2016; Guardia et al., 2018), raising the, as yet unconfirmed, possibility that AP-3 may play a role in directing axonal delivery of CB1R, in addition to its role in mediating late endosomal/lysosomal targeting.
Direct Preferential Trafficking of CB1R to the Axon
While these proposed non-polarized and AP-3δ-based targeting mechanisms are of undoubted interest, they are limited by the fact that they are based on experiments that block the transit of multiple proteins through the secretory pathway. BFA blocks any cargo from trafficking and, furthermore, has been shown to also affect endocytosis and recycling (Miller et al., 1992; Wood and Brown, 1992), and AP-3δ knockdown affects any cargo sorted by AP-3.
Most recently, a targeted, retention using selective hooks (RUSH) approach indicated that CB1R is preferentially delivered to the axonal membrane by the secretory pathway (Fletcher-Jones et al., 2019; Figure 4C). CB1R was engineered to incorporate an N-terminal fluorescent protein and streptavidin binding peptide (SBP) tag which was anchored in the ER by the expression of an ER-localized streptavidin-KDEL “hook” (Boncompain et al., 2012; Evans et al., 2017). The addition of biotin, which has a greater affinity for streptavidin than SBP (Keefe et al., 2001), outcompetes the hook-reporter interaction and the reporter is synchronously released and trafficked by bulk flow through the secretory system to the plasma membrane. The progress of the reporter can be monitored by live imaging of the fluorescent protein or by fixed immunostaining for SBP and/or the fluorescent protein (Evans et al., 2017). These experiments revealed that after release from the ER, intracellular CB1R is detected in the axon as soon as 25 min after release, and significantly more de novo CB1R is delivered by the secretory pathway to the axonal membrane than the somatodendritic membrane (Fletcher-Jones et al., 2019). These data indicate that CB1R polarity is established early in the secretory pathway by directed trafficking of CB1R-containing vesicles to axons.
Cb1R and the Endosomal System
While polarity can be established by directed trafficking from the TGN, receptor polarity can be maintained by differential rates of endocytosis in different neuronal compartments. CB1R undergoes both agonist-dependent and constitutive endocytosis, and differences in the rate of endocytosis between axons and dendrites have been proposed to maintain the axonally polarized surface expression of CB1R.
Mechanisms of Agonist-Induced Endocytosis
Like other GPCRs, internalization of CB1R is dynamically regulated by receptor stimulation. Agonist profiling studies of CB1R in cell lines indicate that potent agonists (e.g., WIN55,212-2, CP55,940 and HU 210) cause more rapid internalization than Δ9-THC, the psychoactive component of cannabis, or endogenous cannabinoid analogs, such as methanandamide (Hsieh et al., 1999). It is important to note, however, that the pattern and rate of endocytosis varies according to cell type and, in particular, endocytosis proceeds more slowly in neurons than in clonal cell lines (Coutts et al., 2001; Leterrier et al., 2006). This likely reflects differences in the molecular mechanisms which direct receptor trafficking according to the cell type or membrane subdomain. For example, truncation of last 14 amino acids of CB1R markedly reduces activity-dependent internalization in AtT20 cells but does not affect rates of internalization in HEK293 cells (Hsieh et al., 1999; Daigle et al., 2008a), suggesting cellular context represents an important determinant of CB1R endocytosis. Indeed, given that difference in the rate of constitutive endocytosis between different neuronal compartments has been proposed to contribute to the polarized expression of CB1R, it appears that context, even within the same cell, plays an important role in CB1R behavior.
Agonist-induced internalization of GPCRs, including of CB1R, generally occurs as a result of receptor phosphorylation and β-arrestin binding (see “β-Arrestin” section below), followed by clathrin-mediated endocytosis (CME) (Ahn et al., 2013b; Blume et al., 2016; Figure 5). However, blocking CME only partially impairs CB1R internalization (Keren and Sarne, 2003; Wu et al., 2008). An alternative and/or parallel mechanism is caveolin-mediated endocytosis of proteins localized to lipid rafts (Razani et al., 2002). Lipid rafts are specialized microdomains of plasma membrane rich in sphingolipids and cholesterol (Allen et al., 2007) and plasma membrane expressed CB1R has been reported to reside in lipid rafts (Sarnataro et al., 2005; Asimaki and Mangoura, 2011). Indeed, membrane cholesterol depletion using methyl-β-cyclodextrin (MβCD), which disrupts lipid rafts, is an effective way of preventing CB1R endocytosis (Keren and Sarne, 2003; Leterrier et al., 2004, 2006) and a mutation that increases lipid raft association of CB1R (CB1R-F238L) increases constitutive endocytosis of CB1R (Wickert et al., 2018). Association with lipid raft domains has also been proposed to influence receptor signaling and ligand binding (Keren and Sarne, 2003; Bari et al., 2005, 2008; Wu et al., 2008) and this association has been reported to rely on palmitoylation of cysteine residue C416 in rat CB1R (see “Palmitoylation of ctCB1R” section below).
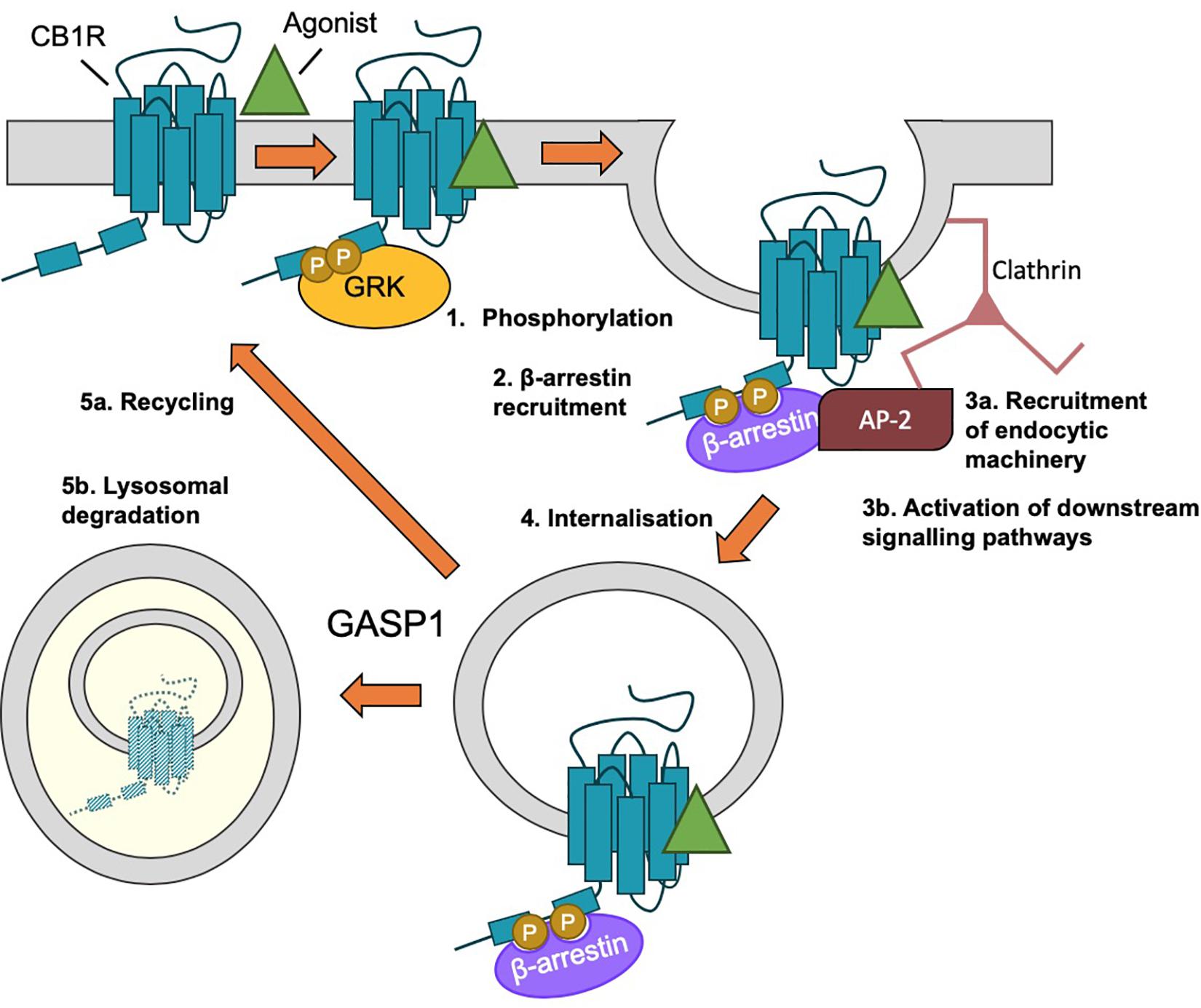
Figure 5. β-arrestin-mediated internalization of CB1R. (1) Following agonist binding, GPCR kinases (GRKs) phosphorylate the C-terminal tail of CB1R. (2) Phosphorylation of ctCB1R leads to recruitment of β-arrestins. (3) β-arrestin recruitment (a) acts as scaffold for recruitment of AP-2 and clathrin and (b) elicits the activation of downstream kinase cascades, most classically, the activation of ERK1/2. (4) The desensitized CB1R is internalized by clathrin-mediated endocytosis (CME). (5) Endosomal CB1R is either recycled (a) or sorted by GASP1 binding for lysosomal degradation (b), depending on the concentration and duration of agonist.
Differential Internalization of CB1R – A Mechanism for Maintaining Polarity?
Cannabinoid type 1 receptor displays high levels of constitutive activity due to significant endocannabinoid tone in brain and other tissues (Nie and Lewis, 2001a; Pertwee, 2005; Howlett et al., 2011) that is sensitive to the application of antagonists such as SR 141716A (Bouaboula et al., 1997; Pan et al., 1998) or AM251/AM281 (Leterrier et al., 2004, 2006). Consequently, CB1R undergoes constitutive internalization, and this has been proposed as a mechanism for the polarized surface distribution of CB1R in neurons, since it has been reported that rapid rates of constitutive endocytosis in somatodendritic compartments leads to an accumulation of CB1R at the axonal plasma membrane (Leterrier et al., 2006; McDonald et al., 2007a; Simon et al., 2013). More specifically, monitoring internalization of CB1R in cultured hippocampal neurons by live incubation with an antibody recognizing an extracellular epitope (antibody feeding), followed by stripping remaining surface antibody with a low pH wash, demonstrated an enhanced rate of receptor endocytosis in the somatodendritic compartment compared to axons (Leterrier et al., 2006). Furthermore, blockade of receptor endocytosis by overexpression of a dominant-negative dynamin 1 disrupts CB1R polarity and increases its somatodendritic surface expression (Leterrier et al., 2006; Bohn, 2007), suggesting these differential rates of endocytosis are capable of supporting polarized surface expression.
Whether this enhanced dendritic internalization is dependent on receptor activity, or on the conformational state of the receptor, has produced conflicting results. It has been reported that preventing the receptor adopting an active conformation using the inverse agonist AM281 leads to an increase in somatodendritic surface expression of CB1R and therefore a loss of axonal polarity (Leterrier et al., 2006). Furthermore, “locking” the receptor in a hypoactive conformation by incorporation of a T211A mutation (D’Antona et al., 2006) resulted in a loss of axonal polarity (Simon et al., 2013). Indeed, somatodendritic CB1R exhibits a high level of constitutive activity as a result of locally produced 2-AG (Ladarre et al., 2014) and reducing constitutive activity in response to endogenously produced 2-AG using the DAGLα inhibitor THL results in an upregulation of CB1R on the somatodendritic surface (Turu et al., 2007). Together, these studies support a role for enhanced endocytosis from the somatodendritic compartment occurring in response to high levels of constitutive activity of the receptor (Figure 4A). In contrast, other studies detected no effect of inverse agonists on CB1R axonal polarity (Coutts et al., 2001; McDonald et al., 2007a), and reported that CB1R mutants that display defective constitutive activity [CB1R-D164N; (Nie and Lewis, 2001a)] or that lack agonist-induced endocytosis in heterologous cells [a mutant lacking the last 14 amino acids (Hsieh et al., 1999)] exhibit normal axonal polarity (McDonald et al., 2007a).
Using antibody feeding to monitor surface expression during the RUSH forward trafficking assay, Fletcher-Jones et al. (2019) found that newly delivered axonal CB1R exhibited relatively stable surface expression, whereas what little CB1R was delivered to the dendritic plasma membrane was rapidly removed by endocytosis (Fletcher-Jones et al., 2019; Figure 4C). Notably, however, there was only a small, non-significant trend toward increased dendritic surface expression following treatment with the inverse agonist AM281. This study therefore supports a model whereby the main driving force for axonal polarity is preferential delivery to the axonal plasma membrane, whereas endocytosis, constitutive or agonist-induced, acts to maintain and enhance polarity.
Thus, an emerging consensus in the field is that differential rates of endocytosis between axons and dendrites play an important role in maintaining CB1R polarity. Furthermore, there is accumulating evidence for a role of constitutive receptor activity in this process, although the findings are conflicting, potentially due to the different experimental systems (for example, the age and culturing methods of primary neurons) and CB1R constructs used (for example the use of N- versus C-terminally tagged receptors). Clearly, further work will be required to reconcile these differences. Nonetheless, how the different rates of endocytosis between neuronal compartments occurs mechanistically is an important outstanding question in the field. It remains possible that compartment-specific interactions that stabilize receptor surface expression, or enhance endocytosis, in distinct compartments may underlie the differential endocytosis of CB1R in axons versus dendrites.
Post-Endocytic Sorting of Cb1R
Degradation
After activation, most GPCRs are endocytosed from the cell surface into low pH endosomes that facilitate ligand detachment, before the receptor is recycled back to the surface or trafficked for degradation (Ferguson, 2001; Figure 5). Several studies have examined CB1R recycling following agonist-induced internalization, using immunocytochemistry and live antibody feeding protocols, to selectively label surface expressed exogenous CB1R in cell lines to follow their endocytic fate (Hsieh et al., 1999; Sim-Selley et al., 2006; Martini et al., 2007, 2010; Tappe-Theodor et al., 2007; Grimsey et al., 2010; Blume et al., 2016). Together, these studies indicate that relatively short agonist exposure (<20 min) and/or low agonist concentrations favor recycling. Increasing the concentration or duration of agonist application promotes receptor degradation, resulting in recovery by newly synthesized receptors, reduced surface levels, and accumulation within lysosomal compartments. Indeed, prolonged agonist exposure and the subsequent down-regulation and desensitization of CB1R, in combination with changes in receptor G protein coupling and endocannabinoid content, has been implicated in the tolerance and dependence associated with prolonged cannabis use (Mato et al., 2005; Martini et al., 2010).
Transcytosis
What happens to CB1R following internalization from the somatodendritic membrane is unclear. However, the Lenkei group have presented evidence that CB1R may traffic in a transcytotic manner, with endosomal somatodendritic CB1R being sorted and sent to the axonal membrane, particularly to more distal areas (Simon et al., 2013). To investigate this, they grew primary neurons in microfluidic chambers, which allow for the separation of the somatodendritic and axonal domains. Interestingly, a small amount of N-terminal antibody fed into the somatodendritic compartment of the chamber was detected after 4 h on the axonal membrane, especially in the very distal end of the axon (Simon et al., 2013) suggesting that receptors that briefly reach the somatodendritic surface are subsequently rerouted to the axonal membrane. However, the pathways that mediate this transcytotic sorting have yet to be established.
Roles of the Cb1R C-Terminal Domain
In common with many other neurotransmitter receptors, multiple protein interactions and post-translational modifications occur at the intracellular C-terminal region of CB1R (ctCB1R; Figure 3). NMR spectroscopy and circular dichroism analysis has revealed that ctCB1R contains two α-helical domains, known as Helix 8 (H8) and Helix 9 (H9), respectively (Ahn et al., 2009b). These helices are amphipathic and likely associate with the plasma membrane along their non-polar faces (Ahn et al., 2009b; Stadel et al., 2011).
A recent report highlighted the importance of the CB1R C-terminal domain in polarized trafficking and surface expression in cultured neurons (Fletcher-Jones et al., 2019). The single-pass membrane protein CD4 has no intrinsic localization signals and is normally surface expressed in a non-polarized manner (Garrido et al., 2001; Fache et al., 2004). However, a CD4-ctCB1R chimera displays a markedly more axonally polarized surface distribution than CD4 alone. Furthermore, CD4-ctCB1R was internalized more compared to CD4 in dendrites but not in axons, indicating ctCB1R plays a role in establishing the differential endocytosis described previously (Fletcher-Jones et al., 2019). Importantly, however, axonal surface expression of CD4-ctCB1R was not completely polarized, suggesting that other localization motifs or signals potentially induced by agonist binding are required for full polarization.
Helix 8 (H8)
Helix 8 is a conserved feature of class A GPCR C-termini (Han et al., 2001) and has been identified in crystal structures across a range of GPCR subtypes. NMR studies demonstrate that, in a variety of GPCRs, H8 forms an amphipathic helix that associates with the plasma membrane via its hydrophobic face, and may also contact intracellular loops in an activity-dependent manner (Murakami and Kouyama, 2008; Ahn et al., 2010; Stadel et al., 2011). It is positioned immediately downstream of another highly conserved motif, the membrane imbedded NPXXY motif located at the end of TM7. In rhodopsin, the NPXXY motif forms a bending “elbow region,” allowing H8 to contact the plasma membrane, providing structural constraints on H8, and mediating structural rearrangements during receptor conformational switches from active to inactive states (Fritze et al., 2003).
In various GPCRs, H8 has been implicated in receptor homodimerization (Salahpour et al., 2004; Knepp et al., 2012; Parmar et al., 2017), ER exit (Salahpour et al., 2004; Parmar et al., 2017), surface expression (Feierler et al., 2011; Spomer et al., 2014; Pandey et al., 2019), as a site of G protein coupling (Delos Santos et al., 2006; Kaye et al., 2011; Kuramasu et al., 2011; Kawasaki et al., 2015; Markx et al., 2019), and in β-arrestin binding (Feierler et al., 2011; Kirchberg et al., 2011; Kang et al., 2015; Yang et al., 2019) and subsequent internalization (Faussner et al., 2005; Aratake et al., 2012). Specifically in CB1R, disruption of H8 helicity and hydrophobicity impairs CB1R trafficking, causing it to accumulate in the ER (Ahn et al., 2010). However, the other roles H8 plays in the trafficking, signaling and protein interaction profile of CB1R are currently unknown.
Helix 9 (H9)
Until recently, the functional significance of H9 has been enigmatic, with only two other GPCRs, squid rhodopsin (Murakami and Kouyama, 2008) and the bradykinin receptor (Piserchio et al., 2005) reported to have an analogous structural domain (Stadel et al., 2011). However, studies of squid rhodopsin suggest an association of H9 with the plasma membrane via interactions with other cytoplasmic regions including H8 (Shimamura et al., 2008). For CB1R, evidence from NMR spectroscopy suggests that H9, like H8, lies along the inner-membrane surface, associating with the lipid bilayer via a cluster of hydrophobic residues on the non-polar face of the helix (Ahn et al., 2009b, 2010). As suggested for squid rhodopsin (Murakami and Kouyama, 2008) and the bradykinin receptor (Piserchio et al., 2005) the polar face of H9 may further serve as a Gαq-binding site.
Most recently, evidence has shown that H9 plays multifaceted roles in the polarized delivery and membrane retention of CB1R, and in the downstream activation of ERK1/2 following stimulation with the CB1R agonist arachidonyl-2′-chloroethylamide (ACEA) (Fletcher-Jones et al., 2019). A CB1R mutant lacking H9 (CB1RΔH9) displayed increased secretory pathway delivery to dendrites, indicating that H9 contributes to polarized delivery by restricting sorting to dendrites. Furthermore, CB1RΔH9 was less surface expressed and endocytosed more, in both axons and dendrites, than CB1RWT. Application of the inverse agonist AM281 reversed the increased internalization, suggesting that H9 stabilizes CB1R against agonist-induced internalization. Furthermore, deletion of H9 also reduced ERK1/2 activation in response to ACEA, suggesting H9 is required for the full signaling capacity of the receptor (Fletcher-Jones et al., 2019). However, future work is needed to define the structural contribution of H9, or H9 interacting proteins, that mediate these effects.
Palmitoylation of ctCB1R
The presence of a palmitoylation site immediately downstream of H8 (C416 in rat CB1R) is conserved amongst class A GPCRs (Sensoy and Weinstein, 2015) and computational modeling of CB1R, as well as the dopamine D2 receptor and the β2-adrenergic receptor, suggests that palmitoylation of this conserved residue enhances the stability and membrane/lipid raft association of the amphipathic H8 (Oddi et al., 2017). Mutation of the corresponding cysteine in human CB1R to an alanine residue (C415A) prevented palmitoylation of CB1R in HEK293 cells (Oddi et al., 2012). Moreover, analysis of the palmitoylation state of CB1R isolated from plasma membrane-enriched P2 membrane fractions derived from rat forebrain indicated that the majority of plasma membrane CB1R is palmitoylated (Oddi et al., 2012). When this residue is de-palmitoylated, membrane association of H8 ceases to be energetically favorable, so the helix unravels, causing some interaction sites to be lost and exposing other interaction sites to the aqueous domain (Sensoy and Weinstein, 2015). Overall, characterization of the non-palmitoylatable CB1R mutant suggests that palmitoylation at this site affects CB1R trafficking, localization, and signaling (Oddi et al., 2012, 2017, 2018). Specifically, blocking palmitoylation reduced plasma membrane expression, increased diffusional mobility, and prevented agonist-induced internalization of the receptor in SH-SY5Y and HEK293 cells, effects attributed to decreased association of CB1R with lipid rafts and caveolin-1 (Oddi et al., 2012, 2017). Furthermore, CB1R signaling was also diminished in the C416A CB1R mutant due to the instability of H8, which prevented the interaction of CB1R with G proteins and β-arrestins (Oddi et al., 2012, 2017, 2018).
Proteins That Interact With ctCB1R
Although not as extensively characterized as many other neurotransmitter receptors, there have been a number of studies investigating the proteins that interact with the C-terminal domain of CB1R. The best characterized of the currently identified interacting proteins are discussed below. Of these, β-arrestins and GASP1 are relatively generic in that they play important roles in pathways common to the regulation of multiple GPCRs. CRIP1 and SGIP1, however, are more selective for CB1R, suggesting that these could be suitable candidates for manipulation to specifically intervene in CB1R localization and function. Importantly, however, the proteins already identified are certainly not a comprehensive list and future studies to identify and validate novel protein-protein interactions will provide important information about the molecular mechanisms of CB1R trafficking and polarity.
β-Arrestins
β-arrestins 1 and 2 regulate agonist-induced internalization and desensitization of multiple GPCRs (Moore et al., 2007) and CB1Rs have been reported to undergo β-arrestin-mediated agonist-dependent desensitization and internalization (Daigle et al., 2008a; Wickert et al., 2018; Al-Zoubi et al., 2019; Figure 5).
Several in vitro studies have examined the coupling of CB1R with β-arrestin 1 and/or 2, although results differ widely depending on cell type and agonist used (Jin et al., 1999; Daigle et al., 2008a, b; Gyombolai et al., 2013, 2015; Laprairie et al., 2014, 2016; Delgado-Peraza et al., 2016). Most recently, a study using bioluminescence resonance energy transfer (BRET) to measure β-arrestin translocation to the membrane following untagged CB1R activation in HEK293 cells showed stronger β-arrestin 2 membrane translocation than β-arrestin 1, although β-arrestin 2 coupling was, again, highly dependent on the specific ligand (Ibsen et al., 2019).
Functional in vivo studies using β-arrestin 1 or β-arrestin 2 knockout mice show that their roles in CB1R desensitization and tolerance are highly dependent on brain region and agonist type (Breivogel et al., 2008, 2013; Nguyen et al., 2012; Breivogel and Vaghela, 2015). Deletion of β-arrestin 1 modulates the effects of acute synthetic agonist CP55940, but not THC, on cannabinoid-mediated behaviors such as antinociception and hypothermia, but does not affect the development of tolerance to either CP55940 or THC (Breivogel and Vaghela, 2015). A similar agonist-dependent effect is seen in β-arrestin 2 knockout mice (Breivogel et al., 2008). Deletion of β-arrestin 2 enhances acute THC-mediated antinociception and hypothermia, but not catalepsy (Nguyen et al., 2012). Furthermore, with repeated administration of Δ9-THC, β-arrestin 2 knockout mice displayed reduced desensitization in cerebellum, caudal periaqueductal gray and spinal cord, along with attenuated tolerance to Δ9-THC-mediated antinocioception. However, β-arrestin 2 knockout mice showed increased desensitization in the hypothalamus, cortex, globus pallidus and substantia nigra, along with greater tolerance to THC-induced catalepsy (Nguyen et al., 2012). These data indicate that the role of β-arrestin 1 and 2 in CB1R desensitization and internalization is highly agonist and region specific (see Al-Zoubi et al., 2019 for a recent review).
β-arrestin binding sites usually include a cluster of at least 2 phosphorylated residues and they are typically recruited to GPCRs through GPCR kinase (GRK)-mediated phosphorylation (Moore et al., 2007). CB1R desensitization involves GPCR kinase 2/3 (GRK2/3)-mediated phosphorylation of two serine residues (S426 and S430), which recruit β-arrestin 1/2 and prevent G protein coupling (Jin et al., 1999; Kouznetsova et al., 2002; Daigle et al., 2008a; Morgan et al., 2014). Similar to deletion of β-arrestin 2, S426A/S430A knock in mice display reduced tolerance to cannabinoid-mediated pain for some agonists, but not others (Morgan et al., 2014; Nealon et al., 2019).
However, S426 and S430 phosphorylation are not sufficient for agonist-induced CB1R internalization, which requires phosphorylation of combinations of six serines and threonines at the extreme C-terminus that modulate β-arrestin 1/2 recruitment (Moore et al., 2007; Daigle et al., 2008b; Straiker et al., 2012b). In addition, pharmacological analysis has suggested that β-arrestin 1 and 2 may play distinct roles in CB1R signaling and internalization, respectively (Ahn et al., 2013a). Indeed, the cortex of β-arrestin 2 knockout mice contain more synaptosomal CB1R compared to WT mice (Breivogel et al., 2013) while β-arrestin 1 knockout mice show no difference in CB1R density in brain membranes compared to WT controls (Breivogel and Vaghela, 2015).
While a role for β-arrestins in CB1R function is well-established, the only studies to report a direct association of β-arrestins with CB1R used NMR spectroscopy to examine the interaction of purified β-arrestin 1 with phosphorylated ctCB1R peptides (Bakshi et al., 2007; Singh et al., 2011), and a complication that currently hampers definitive conclusions is that the effects of modifying S426 and S430, and other phosphorylation sites in ctCB1R, appear to be highly dependent on the cell type studied (Straiker et al., 2012b). Therefore, while it is clear that arrestins play an important role in the modulation of CB1R surface expression and signaling, the exact details of how and where on CB1R binding occurs remain to be determined.
G Protein-Coupled Receptor Associated Protein 1 (GASP1)
G protein-coupled receptor associated protein 1 has been linked to the intracellular sorting of CB1R to lysosomes following chronic exposure to agonist (Simonin et al., 2004). Members of the family of GASP proteins (GASP1-10) bind the C-terminal tails of multiple GPCRs, including the D4 dopaminergic receptor, the β2 adrenergic receptor, and the δ opioid receptor, where they play roles in post-endocytic sorting to lysosomes for degradation (Whistler et al., 2002; Heydorn et al., 2004; Simonin et al., 2004; Moser et al., 2010). A screen of a range of GPCRs has identified two conserved residues in the H8 motif of CB1R (F409/R410) that are required for high-affinity GASP1 interactions (Simonin et al., 2004). Despite the fact that GASP1 was originally isolated as a CB1R interactor from a GST pulldown using only the extreme distal portion of ctCB1R [the last 14 amino acids; (Martini et al., 2007)], CB1R truncations lacking the last 13 amino acids of the C-terminal tail were still able to co-immunoprecipitate GASP1 when co-expressed in HEK293 cells (Tappe-Theodor et al., 2007), potentially via binding to H8. Thus, the precise binding domain for GASP1 on CB1R has not yet been identified and it is possible that there may be multiple sites for GASP1 interaction in ctCB1R.
Consistent with its known function, expression of a dominant negative GASP1 attenuated downregulation of surface CB1R induced by chronic (24 h) exposure to the agonist WIN55,212-2 in both HEK293 cells stably expressing N-terminally FLAG-tagged CB1R and in primary neurons (Martini et al., 2007; Tappe-Theodor et al., 2007). Importantly, this process has been implicated in the development of tolerance to cannabinoids in habitual cannabis users since, unlike wild-type mice, GASP1 knockout mice do not develop tolerance to repeated administration of WIN55,212-2 in 3 components of the classic tetrad of cannabinoid-mediated behaviors (antinociception, hypolocomotion, and catalepsy) (Martini et al., 2010). However, WT and GASP1 knockout mice both developed tolerance to WIN55,21202-mediated hypothermia, potentially due to differences in overlapping expression of CB1R and GASP1 in different brain regions driving diverse mechanisms of tolerance (Martini et al., 2010). However, one limitation of this study when extrapolating the results habitual cannabis users is the use of WIN55,212-2 rather than Δ9-THC since desensitization, internalization, recycling, and tolerance are highly agonist-specific (Hsieh et al., 1999; Wu et al., 2008; Martini et al., 2007; Morgan et al., 2014; Nealon et al., 2019). Indeed, a recent study reported that Δ9-THC- but not WIN55,212-2-mediated tolerance is dependent on c-Jun N-terminal Kinase (JNK) signaling (Henderson-Redmond et al., 2020).
Cannabinoid Receptor Interacting Protein 1a and 1b (CRIP1a/b)
Cannabinoid receptor interacting protein 1a (CRIP1a), and its primate-specific splice variant CRIP1b, represent a novel class of proteins that were identified by yeast-two hybrid screens against a human cDNA library using the last 55 amino acids of CB1R as a bait (Niehaus et al., 2007). Interaction with ctCB1R was validated in vitro using GST pulldowns of purified CRIP1a/b protein and co-immunoprecipitations from rat brain lysates (for CRIP1a only, as CRIP1b is primate specific), further suggesting that these proteins interact in vivo (Niehaus et al., 2007). Furthermore, in mice, CRIP1a co-localizes with CB1R at presynaptic boutons in both pyramidal neurons and interneurons of the hippocampus, and in situ hybridization analysis shows that CRIP1a and CB1R expression overlap, especially in glutamatergic and GABAergic neurons in the hippocampus (Guggenhuber et al., 2016). However, CRIP1a is also abundant in cells that express little or no CB1R (e.g., dentate granule cells), indicating that CRIP1a likely has other functions independent of CB1R (Guggenhuber et al., 2016).
Five amino acids in the very distal carboxy terminus of CB1R are necessary for CRIP1a binding: D467, T468, S469, A472, and L473 (Mascia et al., 2017) and, interestingly, a similar motif present in the metabotropic glutamate receptor mGlu8a also binds CRIP1a (Mascia et al., 2017).
Cannabinoid receptor interacting protein 1a (but not 1b) contains a Class I PDZ ligand at its C-terminus (Niehaus et al., 2007) indicating a possible interaction with PDZ domain-containing proteins. Furthermore, CRIP1a (but not 1b) contains a palmitoylation site that may facilitate partitioning to the membrane and thus association with CB1R (Niehaus et al., 2007; Booth et al., 2019).
It has been reported recently that CRIP1a overexpression suppresses agonist-induced internalization of CB1R, but not desensitization, by competing with β-arrestin-2 for binding to the distal tail of ctCB1R (Smith et al., 2015; Blume et al., 2017). Phosphorylation of ctCB1R at T468 reduced binding to CRIP1a, allowing for β-arrestin-2 binding (Blume et al., 2017). This is consistent with the requirement for phosphorylation of residues in the central region of ctCB1R for desensitization, but not internalization, which requires phosphorylation of the distal tail (Jin et al., 1999; Kouznetsova et al., 2002; Daigle et al., 2008b; Straiker et al., 2012a; Morgan et al., 2014). Accordingly, overexpression of CRIP1a attenuates CB1R G protein signaling in HEK293 cells, N18TG2 cells, and autaptic neuronal cultures, reducing the downstream inhibition of N-type VGCCs and activation of ERK (Niehaus et al., 2007; Blume et al., 2015, 2017; Smith et al., 2015).
Src Homology 3-Domain Growth Factor Receptor-Bound 2-Like (endophilin) Interacting Protein 1 (SGIP1)
SGIP1 was first identified as a novel transcript in a screen of hypothalamic mRNA in the obesity model of the fat sand rat (Psammomys obesus) that is markedly upregulated in comparison to lean counterparts (Trevaskis et al., 2005). Both SGIP1 and CB1R are strongly associated with diet-induced obesity [DIO; (Trevaskis et al., 2005; Soria-Gomez et al., 2014; Cardinal et al., 2015)] and siRNA-mediated knockdown of hypothalamic SGIP1 inhibited food intake, suggesting that SGIP1 in the hypothalamus plays a role in energy expenditure (Trevaskis et al., 2005).
Since its identification, accumulating evidence has identified SGIP1 as an endocytic protein. SGIP1 is capable of binding liposomes (Uezu et al., 2007), and also interacts with a number of adaptor proteins with roles in CME, including endophilin 3 (Trevaskis et al., 2005) the AP-2 complex (Hollopeter et al., 2014) intersectin 1 (ITSN1) (Dergai et al., 2010) amphiphysin, (Daumke et al., 2014) and Eps15 (Uezu et al., 2007).
SGIP1 was identified as a CB1R interactor by yeast two-hybrid assays using ctCB1R as a bait, and the interaction confirmed using coimmunoprecipitation assays (Hajkova et al., 2016). Moreover, SGIP1α, a brain-specific isoform of SGIP1, is enriched in presynaptic boutons (Wilhelm et al., 2014), and co-localizes with CB1R, bassoon, and synaptotagmin 1 (Hajkova et al., 2016; Lee et al., 2019).
Importantly, co-expression of SGIP1 with CB1R in HEK293 cells interfered with agonist-induced internalization of CB1R compared to cells expressing CB1R alone (Hajkova et al., 2016). This reduction in agonist-induced internalization was almost the same as the reduction in internalization that occurred when CME was blocked by expression of a dominant negative dynamin. A smaller, but significant, reduction in constitutive internalization was also observed in CB1R/SGIP1 co-expressing cells compared to HEK293 cells expressing CB1R alone. Furthermore, SGIP1 enhanced β-arrestin-2 association with activated CB1R and reduced CB1R agonist-induced ERK1/2 activation. However, Gi/o-protein activation and downstream Ca2+ mobilization were unaffected (Hajkova et al., 2016).
Since SGIP1 is part of the CME complex it is initially counter-intuitive that its overexpression prevents CB1R internalization. An explanation for this is that SGIP1 competes for binding with FCHo1/2 proteins (Hajkova et al., 2016). Both SGIP1 and FCHo1/2 are members of the muniscin family of cargo adapters, which contain an N-terminal membrane biding domain, an AP-2 activator domain, a proline rich domain, and a C-terminal μ homology domain (Dergai et al., 2010; Hollopeter et al., 2014). However, the membrane binding domain of FCHo1/2 is an F-BAR domain capable of plasma membrane shaping during pit formation, while the membrane binding domain of SGIP1 has no sequence similarity. Thus, differential membrane binding may allow SGIP1 to act as “dominant negative” to inhibit FCHo1/2-dependent CB1R internalization. Furthermore, SGIP1 interacts with endophilin 3, which has also been reported to inhibit endocytosis (Sugiura et al., 2004; Zhang et al., 2015). It should be noted, however, that SGIP1 knockdown has been reported to selectively impair internalization of the pre-synaptic protein synaptotagmin 1 during synaptic vesicle recycling, although other synaptic vesicle proteins including synaptophysin and VAMP2 were not affected by SGIP1 knockdown (Lee et al., 2019). SGIP1 also reportedly activates AP-2 (Hollopeter et al., 2014) and initiates membrane tubulation, and both SGIP1 overexpression and knockdown reduce transferrin (Tfn) uptake (Uezu et al., 2007).
Thus, SGIP1α is an endocytic adaptor protein that appears to play complex, and potentially target specific, roles in plasma membrane protein internalization. Nonetheless, it selectively reduces CB1R internalization and since both CB1R (Mazier et al., 2015) and SGIP1α (Trevaskis et al., 2005; Cummings et al., 2012) play key roles in food intake and energy expenditure (Hajkova et al., 2016), it is interesting to speculate that targeting the interaction between these two proteins could be a useful therapeutic approach to combat obesity.
Overview and Perspectives
There is burgeoning medical and societal interest in the recreational and therapeutic use of cannabinoid drugs. In addition, there is intense scientific interest in, and appreciation of, the pervasive influence and importance of ECS neuromodulatory feedback in almost all aspects of synaptic transmission and plasticity. However, although the complex pharmacology has been the subject of concerted research for decades, our knowledge of the protein interactions and synaptic dynamics of cannabinoid receptors lags far behind that of many other neurotransmitter receptors, and there remain many outstanding questions.
Indeed, the question of whether CB1R is directly axonally targeted from the secretory pathway has produced confounding results, with some studies favoring a direct route, and others observing non-targeted CB1R sorting at the TGN. While the differences between these studies may result from different methodological approaches, ages of neurons used and differently tagged receptors, further work will be required to resolve these discrepancies. Similarly, while there is a broad agreement that differential endocytosis rates between axons and the somatodendritic compartment contributes to the axonally polarized surface expression of CB1R, there is conflicting evidence regarding the role of receptor conformation and basal activity in these processes. Moreover, while the protein interactions and post-translational modifications that control CB1R signaling, internalization and sorting have begun to be addressed, these studies have utilized a number of different experimental systems that have made full interpretation of these findings difficult. Undoubtedly, further studies on these issues, using standardized approaches where possible and focusing on these pathways in neuronal cells, will benefit and add clarity to the field. Finally, while a number of CB1R interactors have been identified, there almost certainly exist a variety of others than are currently unknown. Future studies using both targeted and mass spectrometry approaches will likely address these exciting possibilities directly.
Given the importance of the ECS in a wide variety of brain functions, and its implication in a number of disease states, we anticipate that this fundamental “nuts and bolts” knowledge will both provide important information about the function and organization of the ECS, and potentially uncover novel therapeutic targets for beneficially manipulating CB1R function in a number of contexts.
Author Contributions
All authors listed have made a substantial, direct and intellectual contribution to the work, and approved it for publication.
Funding
We are grateful to the BBSRC (BB/R00787X/1) to JH and KW, Leverhulme Trust, and Wellcome Trust (105384/Z/14/A) to JH and AE for financial support. AF-J was funded by a University of Bristol Ph.D. Scholarship.
Conflict of Interest
The authors declare that the research was conducted in the absence of any commercial or financial relationships that could be construed as a potential conflict of interest.
References
Ahn, K. H., Bertalovitz, A. C., Mierke, D. F., and Kendall, D. A. (2009a). Dual role of the second extracellular loop of the cannabinoid receptor 1: ligand binding and receptor localization. Mol. Pharmacol. 76, 833–842. doi: 10.1124/mol.109.057356
Ahn, K. H., Pellegrini, M., Tsomaia, N., Yatawara, A. K., Kendall, D. A., and Mierke, D. F. (2009b). Structural analysis of the human cannabinoid receptor one carboxyl-terminus identifies two amphipathic helices. Biopolymers 91, 565–573. doi: 10.1002/bip.21179
Ahn, K. H., Mahmoud, M. M., Samala, S., Lu, D., and Kendall, D. A. (2013a). Profiling two indole-2-carboxamides for allosteric modulation of the CB1 receptor. J. Neurochem. 124, 584–589. doi: 10.1111/jnc.12115
Ahn, K. H., Mahmoud, M. M., Shim, J. Y., and Kendall, D. A. (2013b). Distinct roles of beta-arrestin 1 and beta-arrestin 2 in ORG27569-induced biased signaling and internalization of the cannabinoid receptor 1 (CB1). J. Biol. Chem. 288, 9790–9800. doi: 10.1074/jbc.m112.438804
Ahn, K. H., Nishiyama, A., Mierke, D. F., and Kendall, D. A. (2010). Hydrophobic residues in helix 8 of cannabinoid receptor 1 are critical for structural and functional properties. Biochemistry 49, 502–511. doi: 10.1021/bi901619r
Allen, J. A., Halverson-Tamboli, R. A., and Rasenick, M. M. (2007). Lipid raft microdomains and neurotransmitter signalling. Nat. Rev. Neurosci. 8, 128–140. doi: 10.1038/nrn2059
Al-Zoubi, R., Morales, P., and Reggio, P. H. (2019). Structural insights into CB1 receptor biased signaling. Int. J. Mol. Sci. 20:1837. doi: 10.3390/ijms20081837
Anavi-Goffer, S., Fleischer, D., Hurst, D. P., Lynch, D. L., Barnett-Norris, J., Shi, S., et al. (2007). Helix 8 Leu in the CB1 cannabinoid receptor contributes to selective signal transduction mechanisms. J. Biol. Chem. 282, 25100–25113. doi: 10.1074/jbc.m703388200
Andersson, H., D’antona, A. M., Kendall, D. A., Von Heijne, G., and Chin, C. N. (2003). Membrane assembly of the cannabinoid receptor 1: impact of a long N-terminal tail. Mol. Pharmacol. 64, 570–577. doi: 10.1124/mol.64.3.570
Araque, A., Castillo, P. E., Manzoni, O. J., and Tonini, R. (2017). Synaptic functions of endocannabinoid signaling in health and disease. Neuropharmacology 124, 13–24. doi: 10.1016/j.neuropharm.2017.06.017
Aratake, Y., Okuno, T., Matsunobu, T., Saeki, K., Takayanagi, R., Furuya, S., et al. (2012). Helix 8 of leukotriene B4 receptor 1 inhibits ligand-induced internalization. FASEB J. 26, 4068–4078. doi: 10.1096/fj.12-212050
Asimaki, O., and Mangoura, D. (2011). Cannabinoid receptor 1 induces a biphasic ERK activation via multiprotein signaling complex formation of proximal kinases PKCepsilon, Src, and Fyn in primary neurons. Neurochem. Int. 58, 135–144. doi: 10.1016/j.neuint.2010.11.002
Bacci, A., Huguenard, J. R., and Prince, D. A. (2004). Long-lasting self-inhibition of neocortical interneurons mediated by endocannabinoids. Nature 431, 312–316. doi: 10.1038/nature02913
Bakshi, K., Mercier, R. W., and Pavlopoulos, S. (2007). Interaction of a fragment of the cannabinoid CB1 receptor C-terminus with arrestin-2. FEBS Lett. 581, 5009–5016. doi: 10.1016/j.febslet.2007.09.030
Bari, M., Battista, N., Fezza, F., Finazzi-Agro, A., and Maccarrone, M. (2005). Lipid rafts control signaling of type-1 cannabinoid receptors in neuronal cells. Implications for anandamide-induced apoptosis. J. Biol. Chem. 280, 12212–12220. doi: 10.1074/jbc.m411642200
Bari, M., Oddi, S., De Simone, C., Spagnolo, P., Gasperi, V., Battista, N., et al. (2008). Type-1 cannabinoid receptors colocalize with caveolin-1 in neuronal cells. Neuropharmacology 54, 45–50. doi: 10.1016/j.neuropharm.2007.06.030
Benard, G., Massa, F., Puente, N., Lourenco, J., Bellocchio, L., Soria-Gomez, E., et al. (2012). Mitochondrial CB(1) receptors regulate neuronal energy metabolism. Nat. Neurosci. 15, 558–564. doi: 10.1038/nn.3053
Bentley, M., and Banker, G. (2016). The cellular mechanisms that maintain neuronal polarity. Nat. Rev. Neurosci. 17, 611–622. doi: 10.1038/nrn.2016.100
Blume, L. C., Eldeeb, K., Bass, C. E., Selley, D. E., and Howlett, A. C. (2015). Cannabinoid receptor interacting protein (CRIP1a) attenuates CB1R signaling in neuronal cells. Cell. Signal. 27, 716–726. doi: 10.1016/j.cellsig.2014.11.006
Blume, L. C., Leone-Kabler, S., Luessen, D. J., Marrs, G. S., Lyons, E., Bass, C. E., et al. (2016). Cannabinoid receptor interacting protein suppresses agonist-driven CB1 receptor internalization and regulates receptor replenishment in an agonist-biased manner. J. Neurochem. 139, 396–407. doi: 10.1111/jnc.13767
Blume, L. C., Patten, T., Eldeeb, K., Leone-Kabler, S., Ilyasov, A. A., Keegan, B. M., et al. (2017). Cannabinoid receptor interacting protein 1a competition with beta-Arrestin for cb1 receptor binding sites. Mol. Pharmacol. 91, 75–86. doi: 10.1124/mol.116.104638
Bohn, L. M. (2007). Constitutive trafficking–more than just running in circles? Mol. Pharmacol. 71, 957–958. doi: 10.1124/mol.107.034223
Boncompain, G., Divoux, S., Gareil, N., De Forges, H., Lescure, A., Latreche, L., et al. (2012). Synchronization of secretory protein traffic in populations of cells. Nat. Methods 9, 493–498. doi: 10.1038/nmeth.1928
Booth, W. T., Walker, N. B., Lowther, W. T., and Howlett, A. C. (2019). Cannabinoid receptor interacting protein 1a (CRIP1a): function and structure. Molecules 24:672.
Bouaboula, M., Perrachon, S., Milligan, L., Canat, X., Rinaldi-Carmona, M., Portier, M., et al. (1997). A selective inverse agonist for central cannabinoid receptor inhibits mitogen-activated protein kinase activation stimulated by insulin or insulin-like growth factor 1. Evidence for a new model of receptor/ligand interactions. J. Biol. Chem. 272, 22330–22339. doi: 10.1074/jbc.272.35.22330
Brailoiu, G. C., Oprea, T. I., Zhao, P., Abood, M. E., and Brailoiu, E. (2011). Intracellular cannabinoid type 1 (CB1) receptors are activated by anandamide. J. Biol. Chem. 286, 29166–29174. doi: 10.1074/jbc.m110.217463
Breivogel, C. S., Lambert, J. M., Gerfin, S., Huffman, J. W., and Razdan, R. K. (2008). Sensitivity to delta9-tetrahydrocannabinol is selectively enhanced in beta-arrestin2 -/- mice. Behav. Pharmacol. 19, 298–307. doi: 10.1097/fbp.0b013e328308f1e6
Breivogel, C. S., Puri, V., Lambert, J. M., Hill, D. K., Huffman, J. W., and Razdan, R. K. (2013). The influence of beta-arrestin2 on cannabinoid CB1 receptor coupling to G-proteins and subcellular localization and relative levels of beta-arrestin1 and 2 in mouse brain. J. Recept. Signal Transduct. Res. 33, 367–379. doi: 10.3109/10799893.2013.838787
Breivogel, C. S., and Vaghela, M. S. (2015). The effects of beta-arrestin1 deletion on acute cannabinoid activity, brain cannabinoid receptors and tolerance to cannabinoids in mice. J. Recept. Signal Transduct. Res. 35, 98–106. doi: 10.3109/10799893.2014.1003659
Busquets-Garcia, A., Bains, J., and Marsicano, G. (2018). CB1 receptor signaling in the brain: extracting specificity from ubiquity. Neuropsychopharmacology 43, 4–20. doi: 10.1038/npp.2017.206
Cardinal, P., Bellocchio, L., Guzman-Quevedo, O., Andre, C., Clark, S., Elie, M., et al. (2015). Cannabinoid type 1 (CB1) receptors on Sim1-expressing neurons regulate energy expenditure in male mice. Endocrinology 156, 411–418. doi: 10.1210/en.2014-1437
Castillo, P. E., Younts, T. J., Chavez, A. E., and Hashimotodani, Y. (2012). Endocannabinoid signaling and synaptic function. Neuron 76, 70–81. doi: 10.1016/j.neuron.2012.09.020
Chevaleyre, V., and Castillo, P. E. (2004). Endocannabinoid-mediated metaplasticity in the hippocampus. Neuron 43, 871–881. doi: 10.1016/j.neuron.2004.08.036
Chevaleyre, V., Heifets, B. D., Kaeser, P. S., Sudhof, T. C., and Castillo, P. E. (2007). Endocannabinoid-mediated long-term plasticity requires cAMP/PKA signaling and RIM1alpha. Neuron 54, 801–812. doi: 10.1016/j.neuron.2007.05.020
Coutts, A. A., Anavi-Goffer, S., Ross, R. A., Macewan, D. J., Mackie, K., Pertwee, R. G., et al. (2001). Agonist-induced internalization and trafficking of cannabinoid CB1 receptors in hippocampal neurons. J. Neurosci. 21, 2425–2433. doi: 10.1523/jneurosci.21-07-02425.2001
Cummings, N., Shields, K. A., Curran, J. E., Bozaoglu, K., Trevaskis, J., Gluschenko, K., et al. (2012). Genetic variation in SH3-domain GRB2-like (endophilin)-interacting protein 1 has a major impact on fat mass. Int. J. Obes 36, 201–206. doi: 10.1038/ijo.2011.67
Daigle, T. L., Kearn, C. S., and Mackie, K. (2008a). Rapid CB1 cannabinoid receptor desensitization defines the time course of ERK1/2 MAP kinase signaling. Neuropharmacology 54, 36–44. doi: 10.1016/j.neuropharm.2007.06.005
Daigle, T. L., Kwok, M. L., and Mackie, K. (2008b). Regulation of CB1 cannabinoid receptor internalization by a promiscuous phosphorylation-dependent mechanism. J. Neurochem. 106, 70–82. doi: 10.1111/j.1471-4159.2008.05336.x
Danglot, L., and Galli, T. (2007). What is the function of neuronal AP-3? Biol Cell 99, 349–361. doi: 10.1042/bc20070029
D’Antona, A. M., Ahn, K. H., and Kendall, D. A. (2006). Mutations of CB1 T210 produce active and inactive receptor forms: correlations with ligand affinity, receptor stability, and cellular localization. Biochemistry 45, 5606–5617. doi: 10.1021/bi060067k
Daumke, O., Roux, A., and Haucke, V. (2014). BAR domain scaffolds in dynamin-mediated membrane fission. Cell 156, 882–892. doi: 10.1016/j.cell.2014.02.017
Delgado-Peraza, F., Ahn, K. H., Nogueras-Ortiz, C., Mungrue, I. N., Mackie, K., Kendall, D. A., et al. (2016). Mechanisms of biased beta-arrestin-mediated signaling downstream from the cannabinoid 1 receptor. Mol. Pharmacol. 89, 618–629. doi: 10.1124/mol.115.103176
Delos Santos, N. M., Gardner, L. A., White, S. W., and Bahouth, S. W. (2006). Characterization of the residues in helix 8 of the human beta1-adrenergic receptor that are involved in coupling the receptor to G proteins. J. Biol. Chem. 281, 12896–12907. doi: 10.1074/jbc.m508500200
Dergai, O., Novokhatska, O., Dergai, M., Skrypkina, I., Tsyba, L., Moreau, J., et al. (2010). Intersectin 1 forms complexes with SGIP1 and Reps1 in clathrin-coated pits. Biochem. Biophys. Res. Commun. 402, 408–413. doi: 10.1016/j.bbrc.2010.10.045
Di Marzo, V. (2011). Endocannabinoid signaling in the brain: biosynthetic mechanisms in the limelight. Nat. Neurosci. 14, 9–15. doi: 10.1038/nn.2720
Diez-Alarcia, R., Ibarra-Lecue, I., Lopez-Cardona, A. P., Meana, J., Gutierrez-Adan, A., Callado, L. F., et al. (2016). Biased agonism of three different cannabinoid receptor agonists in mouse brain cortex. Front. Pharmacol. 7:415. doi: 10.3389/fphar.2016.00415
Djeungoue-Petga, M. A., and Hebert-Chatelain, E. (2017). Linking mitochondria and synaptic transmission: the CB1 receptor. Bioessays 39:1700126. doi: 10.1002/bies.201700126
Dudok, B., Barna, L., Ledri, M., Szabo, S. I., Szabadits, E., Pinter, B., et al. (2015). Cell-specific STORM super-resolution imaging reveals nanoscale organization of cannabinoid signaling. Nat. Neurosci. 18, 75–86. doi: 10.1038/nn.3892
Evans, A. J., Gurung, S., Wilkinson, K. A., Stephens, D. J., and Henley, J. M. (2017). Assembly, secretory pathway trafficking, and surface delivery of kainate receptors is regulated by neuronal activity. Cell Rep. 19, 2613–2626. doi: 10.1016/j.celrep.2017.06.001
Fache, M. P., Moussif, A., Fernandes, F., Giraud, P., Garrido, J. J., and Dargent, B. (2004). Endocytotic elimination and domain-selective tethering constitute a potential mechanism of protein segregation at the axonal initial segment. J. Cell Biol. 166, 571–578. doi: 10.1083/jcb.200312155
Faussner, A., Bauer, A., Kalatskaya, I., Schussler, S., Seidl, C., Proud, D., et al. (2005). The role of helix 8 and of the cytosolic C-termini in the internalization and signal transduction of B(1) and B(2) bradykinin receptors. FEBS J. 272, 129–140. doi: 10.1111/j.1432-1033.2004.04390.x
Feierler, J., Wirth, M., Welte, B., Schussler, S., Jochum, M., and Faussner, A. (2011). Helix 8 plays a crucial role in bradykinin B(2) receptor trafficking and signaling. J. Biol. Chem. 286, 43282–43293. doi: 10.1074/jbc.m111.256909
Ferguson, S. S. (2001). Evolving concepts in G protein-coupled receptor endocytosis: the role in receptor desensitization and signaling. Pharmacol. Rev. 53, 1–24.
Fletcher-Jones, A., Hildick, K. L., Evans, A. J., Nakamura, Y., Wilkinson, K. A., and Henley, J. M. (2019). The C-terminal helix 9 motif in rat cannabinoid receptor type 1 regulates axonal trafficking and surface expression. eLife 8:e44252.
Fritze, O., Filipek, S., Kuksa, V., Palczewski, K., Hofmann, K. P., and Ernst, O. P. (2003). Role of the conserved NPxxY(x)5,6F motif in the rhodopsin ground state and during activation. Proc. Natl. Acad. Sci. U.S.A. 100, 2290–2295. doi: 10.1073/pnas.0435715100
Garrido, E., Tato, P., and Molinari, J. L. (2001). A factor isolated from Taenia solium metacestodes stimulates T lymphocytes to proliferate and produce gamma interferon. Parasitol. Res. 87, 956–962. doi: 10.1007/s004360100484
Gonzalez, C., Cornejo, V. H., and Couve, A. (2018). Golgi bypass for local delivery of axonal proteins, fact or fiction? Curr. Opin. Cell Biol. 53, 9–14. doi: 10.1016/j.ceb.2018.03.010
Graham, T. R., Scott, P. A., and Emr, S. D. (1993). Brefeldin A reversibly blocks early but not late protein transport steps in the yeast secretory pathway. EMBO J. 12, 869–877. doi: 10.1002/j.1460-2075.1993.tb05727.x
Grimsey, N. L., Graham, E. S., Dragunow, M., and Glass, M. (2010). Cannabinoid receptor 1 trafficking and the role of the intracellular pool: implications for therapeutics. Biochem. Pharmacol. 80, 1050–1062. doi: 10.1016/j.bcp.2010.06.007
Guardia, C. M., De Pace, R., Mattera, R., and Bonifacino, J. S. (2018). Neuronal functions of adaptor complexes involved in protein sorting. Curr. Opin. Neurobiol 51, 103–110. doi: 10.1016/j.conb.2018.02.021
Guggenhuber, S., Alpar, A., Chen, R., Schmitz, N., Wickert, M., Mattheus, T., et al. (2016). Cannabinoid receptor-interacting protein Crip1a modulates CB1 receptor signaling in mouse hippocampus. Brain Struct. Funct. 221, 2061–2074. doi: 10.1007/s00429-015-1027-6
Gyombolai, P., Boros, E., Hunyady, L., and Turu, G. (2013). Differential beta-arrestin2 requirements for constitutive and agonist-induced internalization of the CB1 cannabinoid receptor. Mol. Cell. Endocrinol. 372, 116–127. doi: 10.1016/j.mce.2013.03.013
Gyombolai, P., Toth, A. D., Timar, D., Turu, G., and Hunyady, L. (2015). Mutations in the ’DRY’ motif of the CB1 cannabinoid receptor result in biased receptor variants. J. Mol. Endocrinol. 54, 75–89. doi: 10.1530/jme-14-0219
Hajkova, A., Techlovska, S., Dvorakova, M., Chambers, J. N., Kumpost, J., Hubalkova, P., et al. (2016). SGIP1 alters internalization and modulates signaling of activated cannabinoid receptor 1 in a biased manner. Neuropharmacology 107, 201–214. doi: 10.1016/j.neuropharm.2016.03.008
Han, J., Kesner, P., Metna-Laurent, M., Duan, T., Xu, L., Georges, F., et al. (2012). Acute cannabinoids impair working memory through astroglial CB1 receptor modulation of hippocampal LTD. Cell 148, 1039–1050. doi: 10.1016/j.cell.2012.01.037
Han, M., Gurevich, V. V., Vishnivetskiy, S. A., Sigler, P. B., and Schubert, C. (2001). Crystal structure of beta-arrestin at 1.9 A: possible mechanism of receptor binding and membrane Translocation. Structure 9, 869–880.
Hebert-Chatelain, E., Desprez, T., Serrat, R., Bellocchio, L., Soria-Gomez, E., Busquets-Garcia, A., et al. (2016). A cannabinoid link between mitochondria and memory. Nature 539, 555–559.
Heifets, B. D., and Castillo, P. E. (2009). Endocannabinoid signaling and long-term synaptic plasticity. Annu. Rev. Physiol. 71, 283–306. doi: 10.1146/annurev.physiol.010908.163149
Henderson-Redmond, A. N., Nealon, C. M., Davis, B. J., Yuill, M. B., Sepulveda, D. E., Blanton, H. L., et al. (2020). c-Jun N terminal kinase signaling pathways mediate cannabinoid tolerance in an agonist-specific manner. Neuropharmacology 164:107847. doi: 10.1016/j.neuropharm.2019.107847
Herkenham, M., Lynn, A. B., Johnson, M. R., Melvin, L. S., De Costa, B. R., and Rice, K. C. (1991). Characterization and localization of cannabinoid receptors in rat brain: a quantitative in vitro autoradiographic study. J. Neurosci. 11, 563–583. doi: 10.1523/jneurosci.11-02-00563.1991
Heydorn, A., Sondergaard, B. P., Ersboll, B., Holst, B., Nielsen, F. C., Haft, C. R., et al. (2004). A library of 7TM receptor C-terminal tails. Interactions with the proposed post-endocytic sorting proteins ERM-binding phosphoprotein 50 (EBP50), N-ethylmaleimide-sensitive factor (NSF), sorting nexin 1 (SNX1), and G protein-coupled receptor-associated sorting protein (GASP). J. Biol. Chem. 279, 54291–54303. doi: 10.1074/jbc.m406169200
Hollopeter, G., Lange, J. J., Zhang, Y., Vu, T. N., Gu, M., Ailion, M., et al. (2014). The membrane-associated proteins FCHo and SGIP are allosteric activators of the AP2 clathrin adaptor complex. eLife 3:e03648.
Howlett, A. C. (1987). Cannabinoid inhibition of adenylate cyclase: relative activity of constituents and metabolites of marihuana. Neuropharmacology 26, 507–512. doi: 10.1016/0028-3908(87)90035-9
Howlett, A. C. (2004). Efficacy in CB1 receptor-mediated signal transduction. Br. J. Pharmacol. 142, 1209–1218.
Howlett, A. C. (2005). Cannabinoid receptor signaling. Handb. Exp. Pharmacol. 2005, 53–79. doi: 10.1007/3-540-26573-2_2
Howlett, A. C., and Abood, M. E. (2017). CB1 and CB2 Receptor Pharmacology. Adv. Pharmacol. 80, 169–206.
Howlett, A. C., and Fleming, R. M. (1984). Cannabinoid inhibition of adenylate cyclase. Pharmacology of the response in neuroblastoma cell membranes. Mol. Pharmacol. 26, 532–538.
Howlett, A. C., Qualy, J. M., and Khachatrian, L. L. (1986). Involvement of Gi in the inhibition of adenylate cyclase by cannabimimetic drugs. Mol. Pharmacol. 29, 307–313.
Howlett, A. C., Reggio, P. H., Childers, S. R., Hampson, R. E., Ulloa, N. M., and Deutsch, D. G. (2011). Endocannabinoid tone versus constitutive activity of cannabinoid receptors. Br. J. Pharmacol. 163, 1329–1343. doi: 10.1111/j.1476-5381.2011.01364.x
Hsieh, C., Brown, S., Derleth, C., and Mackie, K. (1999). Internalization and recycling of the CB1 cannabinoid receptor. J. Neurochem. 73, 493–501. doi: 10.1046/j.1471-4159.1999.0730493.x
Hunter, M. R., Finlay, D. B., Macdonald, C. E., Cawston, E. E., Grimsey, N. L., and Glass, M. (2017). Real-Time Measurement of Cannabinoid Receptor-Mediated cAMP Signaling. Methods Enzymol. 593, 43–59. doi: 10.1016/bs.mie.2017.05.001
Hunziker, W., Whitney, J. A., and Mellman, I. (1991). Selective inhibition of transcytosis by brefeldin A in MDCK cells. Cell 67, 617–627. doi: 10.1016/0092-8674(91)90535-7
Ibsen, M. S., Finlay, D. B., Patel, M., Javitch, J. A., Glass, M., and Grimsey, N. L. (2019). Cannabinoid CB1 and CB2 receptor-mediated arrestin translocation: species, subtype, and agonist-dependence. Front. Pharmacol. 10:350. doi: 10.3389/fphar.2019.00350
Irving, A. J., Coutts, A. A., Harvey, J., Rae, M. G., Mackie, K., Bewick, G. S., et al. (2000). Functional expression of cell surface cannabinoid CB(1) receptors on presynaptic inhibitory terminals in cultured rat hippocampal neurons. Neuroscience 98, 253–262. doi: 10.1016/s0306-4522(00)00120-2
Jin, W., Brown, S., Roche, J. P., Hsieh, C., Celver, J. P., Kovoor, A., et al. (1999). Distinct domains of the CB1 cannabinoid receptor mediate desensitization and internalization. J. Neurosci. 19, 3773–3780. doi: 10.1523/jneurosci.19-10-03773.1999
Jung, K. M., Astarita, G., Zhu, C., Wallace, M., Mackie, K., and Piomelli, D. (2007). A key role for diacylglycerol lipase-alpha in metabotropic glutamate receptor-dependent endocannabinoid mobilization. Mol. Pharmacol. 72, 612–621. doi: 10.1124/mol.107.037796
Kang, Y., Zhou, X. E., Gao, X., He, Y., Liu, W., Ishchenko, A., et al. (2015). Crystal structure of rhodopsin bound to arrestin by femtosecond X-ray laser. Nature 523, 561–567.
Katona, I., and Freund, T. F. (2008). Endocannabinoid signaling as a synaptic circuit breaker in neurological disease. Nat. Med. 14, 923–930. doi: 10.1038/nm.f.1869
Katona, I., and Freund, T. F. (2012). Multiple functions of endocannabinoid signaling in the brain. Annu. Rev. Neurosci. 35, 529–558. doi: 10.1146/annurev-neuro-062111-150420
Katona, I., Sperlagh, B., Sik, A., Kafalvi, A., Vizi, E. S., Mackie, K., et al. (1999). Presynaptically located CB1 cannabinoid receptors regulate GABA release from axon terminals of specific hippocampal interneurons. J. Neurosci. 19, 4544–4558. doi: 10.1523/jneurosci.19-11-04544.1999
Katona, I., Urban, G. M., Wallace, M., Ledent, C., Jung, K. M., Piomelli, D., et al. (2006). Molecular composition of the endocannabinoid system at glutamatergic synapses. J. Neurosci. 26, 5628–5637. doi: 10.1523/jneurosci.0309-06.2006
Kawamura, Y., Fukaya, M., Maejima, T., Yoshida, T., Miura, E., Watanabe, M., et al. (2006). The CB1 cannabinoid receptor is the major cannabinoid receptor at excitatory presynaptic sites in the hippocampus and cerebellum. J. Neurosci. 26, 2991–3001. doi: 10.1523/jneurosci.4872-05.2006
Kawasaki, T., Saka, T., Mine, S., Mizohata, E., Inoue, T., Matsumura, H., et al. (2015). The N-terminal acidic residue of the cytosolic helix 8 of an odorant receptor is responsible for different response dynamics via G-protein. FEBS Lett. 589, 1136–1142. doi: 10.1016/j.febslet.2015.03.025
Kaye, R. G., Saldanha, J. W., Lu, Z. L., and Hulme, E. C. (2011). Helix 8 of the M1 muscarinic acetylcholine receptor: scanning mutagenesis delineates a G protein recognition site. Mol. Pharmacol. 79, 701–709. doi: 10.1124/mol.110.070177
Kearn, C. S., Blake-Palmer, K., Daniel, E., Mackie, K., and Glass, M. (2005). Concurrent stimulation of cannabinoid CB1 and dopamine D2 receptors enhances heterodimer formation: a mechanism for receptor cross-talk? Mol. Pharmacol. 67, 1697–1704. doi: 10.1124/mol.104.006882
Keefe, A. D., Wilson, D. S., Seelig, B., and Szostak, J. W. (2001). One-step purification of recombinant proteins using a nanomolar-affinity streptavidin-binding peptide, the SBP-Tag. Protein Exp. Purif. 23, 440–446. doi: 10.1006/prep.2001.1515
Kendall, D. A., and Yudowski, G. A. (2016). Cannabinoid receptors in the central nervous system: their signaling and roles in disease. Front. Cell Neurosci. 10:294. doi: 10.3389/fncel.2016.00294
Keren, O., and Sarne, Y. (2003). Multiple mechanisms of CB1 cannabinoid receptors regulation. Brain Res. 980, 197–205. doi: 10.1016/s0006-8993(03)02970-6
Kirchberg, K., Kim, T. Y., Moller, M., Skegro, D., Dasara Raju, G., Granzin, J., et al. (2011). Conformational dynamics of helix 8 in the GPCR rhodopsin controls arrestin activation in the desensitization process. Proc. Natl. Acad. Sci. U.S.A. 108, 18690–18695. doi: 10.1073/pnas.1015461108
Knepp, A. M., Periole, X., Marrink, S. J., Sakmar, T. P., and Huber, T. (2012). Rhodopsin forms a dimer with cytoplasmic helix 8 contacts in native membranes. Biochemistry 51, 1819–1821. doi: 10.1021/bi3001598
Koch, M., Varela, L., Kim, J. G., Kim, J. D., Hernandez-Nuno, F., Simonds, S. E., et al. (2015). Hypothalamic POMC neurons promote cannabinoid-induced feeding. Nature 519, 45–50. doi: 10.1038/nature14260
Kouznetsova, M., Kelley, B., Shen, M., and Thayer, S. A. (2002). Desensitization of cannabinoid-mediated presynaptic inhibition of neurotransmission between rat hippocampal neurons in culture. Mol. Pharmacol. 61, 477–485. doi: 10.1124/mol.61.3.477
Kreitzer, A. C., and Regehr, W. G. (2001). Retrograde inhibition of presynaptic calcium influx by endogenous cannabinoids at excitatory synapses onto Purkinje cells. Neuron 29, 717–727. doi: 10.1016/s0896-6273(01)00246-x
Kuramasu, A., Sukegawa, J., Sato, T., Sakurai, E., Watanabe, T., Yanagisawa, T., et al. (2011). The hydrophobic amino acids in putative helix 8 in carboxy-terminus of histamine H(3) receptor are involved in receptor-G-protein coupling. Cell. Signal. 23, 1843–1849. doi: 10.1016/j.cellsig.2011.06.021
Ladarre, D., Roland, A. B., Biedzinski, S., Ricobaraza, A., and Lenkei, Z. (2014). Polarized cellular patterns of endocannabinoid production and detection shape cannabinoid signaling in neurons. Front. Cell Neurosci. 8:426. doi: 10.3389/fncel.2014.00426
Laprairie, R. B., Bagher, A. M., Kelly, M. E., and Denovan-Wright, E. M. (2016). Biased type 1 cannabinoid receptor signaling influences neuronal viability in a cell culture model of huntington disease. Mol. Pharmacol. 89, 364–375. doi: 10.1124/mol.115.101980
Laprairie, R. B., Bagher, A. M., Kelly, M. E., Dupre, D. J., and Denovan-Wright, E. M. (2014). Type 1 cannabinoid receptor ligands display functional selectivity in a cell culture model of striatal medium spiny projection neurons. J. Biol. Chem. 289, 24845–24862. doi: 10.1074/jbc.m114.557025
Lasiecka, Z. M., and Winckler, B. (2011). Mechanisms of polarized membrane trafficking in neurons – focusing in on endosomes. Mol. Cell. Neurosci. 48, 278–287. doi: 10.1016/j.mcn.2011.06.013
Lee, S. E., Jeong, S., Lee, U., and Chang, S. (2019). SGIP1alpha functions as a selective endocytic adaptor for the internalization of synaptotagmin 1 at synapses. Mol. Brain 12:41.
Leterrier, C., Bonnard, D., Carrel, D., Rossier, J., and Lenkei, Z. (2004). Constitutive endocytic cycle of the CB1 cannabinoid receptor. J. Biol. Chem. 279, 36013–36021. doi: 10.1074/jbc.m403990200
Leterrier, C., Laine, J., Darmon, M., Boudin, H., Rossier, J., and Lenkei, Z. (2006). Constitutive activation drives compartment-selective endocytosis and axonal targeting of type 1 cannabinoid receptors. J. Neurosci. 26, 3141–3153. doi: 10.1523/jneurosci.5437-05.2006
Li, P., Merrill, S. A., Jorgensen, E. M., and Shen, K. (2016). Two Clathrin adaptor protein complexes instruct axon-dendrite polarity. Neuron 90, 564–580. doi: 10.1016/j.neuron.2016.04.020
Lu, H. C., and Mackie, K. (2016). An introduction to the endogenous cannabinoid system. Biol. Psychiatry 79, 516–525. doi: 10.1016/j.biopsych.2015.07.028
Luarte, A., Cornejo, V. H., Bertin, F., Gallardo, J., and Couve, A. (2018). The axonal endoplasmic reticulum: one organelle-many functions in development, maintenance, and plasticity. Dev. Neurobiol. 78, 181–208. doi: 10.1002/dneu.22560
Luchicchi, A., and Pistis, M. (2012). Anandamide and 2-arachidonoylglycerol: pharmacological properties, functional features, and emerging specificities of the two major endocannabinoids. Mol. Neurobiol. 46, 374–392. doi: 10.1007/s12035-012-8299-0
Mackie, K., and Hille, B. (1992). Cannabinoids inhibit N-type calcium channels in neuroblastoma-glioma cells. Proc. Natl. Acad. Sci. U.S.A. 89, 3825–3829. doi: 10.1073/pnas.89.9.3825
Marinelli, S., Pacioni, S., Cannich, A., Marsicano, G., and Bacci, A. (2009). Self-modulation of neocortical pyramidal neurons by endocannabinoids. Nat. Neurosci. 12, 1488–1490. doi: 10.1038/nn.2430
Markx, D., Schuhholz, J., Abadier, M., Beier, S., Lang, M., and Moepps, B. (2019). Arginine 313 of the putative 8th helix mediates Galphaq/14 coupling of human CC chemokine receptors CCR2a and CCR2b. Cell. Signal. 53, 170–183. doi: 10.1016/j.cellsig.2018.10.007
Marsicano, G., Wotjak, C. T., Azad, S. C., Bisogno, T., Rammes, G., Cascio, M. G., et al. (2002). The endogenous cannabinoid system controls extinction of aversive memories. Nature 418, 530–534. doi: 10.1038/nature00839
Martini, L., Thompson, D., Kharazia, V., and Whistler, J. L. (2010). Differential regulation of behavioral tolerance to WIN55,212-2 by GASP1. Neuropsychopharmacology 35, 1363–1373. doi: 10.1038/npp.2010.6
Martini, L., Waldhoer, M., Pusch, M., Kharazia, V., Fong, J., Lee, J. H., et al. (2007). Ligand-induced down-regulation of the cannabinoid 1 receptor is mediated by the G-protein-coupled receptor-associated sorting protein GASP1. FASEB J. 21, 802–811. doi: 10.1096/fj.06-7132com
Mascia, F., Klotz, L., Lerch, J., Ahmed, M. H., Zhang, Y., and Enz, R. (2017). CRIP1a inhibits endocytosis of G-protein coupled receptors activated by endocannabinoids and glutamate by a common molecular mechanism. J. Neurochem. 141, 577–591. doi: 10.1111/jnc.14021
Mato, S., Robbe, D., Puente, N., Grandes, P., and Manzoni, O. J. (2005). Presynaptic homeostatic plasticity rescues long-term depression after chronic Delta 9-tetrahydrocannabinol exposure. J. Neurosci. 25, 11619–11627. doi: 10.1523/jneurosci.2294-05.2005
Mazier, W., Saucisse, N., Gatta-Cherifi, B., and Cota, D. (2015). The endocannabinoid system: pivotal orchestrator of obesity and metabolic disease. Trends Endocrino.l Metab. 26, 524–537. doi: 10.1016/j.tem.2015.07.007
McDonald, N. A., Henstridge, C. M., Connolly, C. N., and Irving, A. J. (2007a). An essential role for constitutive endocytosis, but not activity, in the axonal targeting of the CB1 cannabinoid receptor. Mol. Pharmacol. 71, 976–984. doi: 10.1124/mol.106.029348
McDonald, N. A., Henstridge, C. M., Connolly, C. N., and Irving, A. J. (2007b). Generation and functional characterization of fluorescent, N-terminally tagged CB1 receptor chimeras for live-cell imaging. Mol. Cell. Neurosci. 35, 237–248. doi: 10.1016/j.mcn.2007.02.016
McIntosh, H. H., Song, C., and Howlett, A. C. (1998). CB1 cannabinoid receptor: cellular regulation and distribution in N18TG2 neuroblastoma cells. Brain Res. Mol. Brain Res. 53, 163–173. doi: 10.1016/s0169-328x(97)00294-5
Miller, S. G., Carnell, L., and Moore, H. H. (1992). Post-Golgi membrane traffic: brefeldin A inhibits export from distal Golgi compartments to the cell surface but not recycling. J. Cell Biol. 118, 267–283. doi: 10.1083/jcb.118.2.267
Moore, C. A., Milano, S. K., and Benovic, J. L. (2007). Regulation of receptor trafficking by GRKs and arrestins. Annu. Rev. Physiol. 69, 451–482. doi: 10.1146/annurev.physiol.69.022405.154712
Morgan, D. J., Davis, B. J., Kearn, C. S., Marcus, D., Cook, A. J., Wager-Miller, J., et al. (2014). Mutation of putative GRK phosphorylation sites in the cannabinoid receptor 1 (CB1R) confers resistance to cannabinoid tolerance and hypersensitivity to cannabinoids in mice. J. Neurosci. 34, 5152–5163. doi: 10.1523/jneurosci.3445-12.2014
Moser, E., Kargl, J., Whistler, J. L., Waldhoer, M., and Tschische, P. (2010). G protein-coupled receptor-associated sorting protein 1 regulates the postendocytic sorting of seven-transmembrane-spanning G protein-coupled receptors. Pharmacology 86, 22–29. doi: 10.1159/000314161
Murakami, M., and Kouyama, T. (2008). Crystal structure of squid rhodopsin. Nature 453, 363–367. doi: 10.1038/nature06925
Nakatsu, F., Okada, M., Mori, F., Kumazawa, N., Iwasa, H., Zhu, G., et al. (2004). Defective function of GABA-containing synaptic vesicles in mice lacking the AP-3B clathrin adaptor. J. Cell Biol. 167, 293–302. doi: 10.1083/jcb.200405032
Navarrete, M., and Araque, A. (2008). Endocannabinoids mediate neuron-astrocyte communication. Neuron 57, 883–893. doi: 10.1016/j.neuron.2008.01.029
Nealon, C. M., Henderson-Redmond, A. N., Hale, D. E., and Morgan, D. J. (2019). Tolerance to WIN55,212-2 is delayed in desensitization-resistant S426A/S430A mice. Neuropharmacology 148, 151–159. doi: 10.1016/j.neuropharm.2018.12.026
Nguyen, P. T., Schmid, C. L., Raehal, K. M., Selley, D. E., Bohn, L. M., and Sim-Selley, L. J. (2012). beta-arrestin2 regulates cannabinoid CB1 receptor signaling and adaptation in a central nervous system region-dependent manner. Biol. Psychiatry 71, 714–724. doi: 10.1016/j.biopsych.2011.11.027
Nie, J., and Lewis, D. L. (2001a). Structural domains of the CB1 cannabinoid receptor that contribute to constitutive activity and G-protein sequestration. J. Neurosci. 21, 8758–8764. doi: 10.1523/jneurosci.21-22-08758.2001
Nie, J., and Lewis, D. L. (2001b). The proximal and distal C-terminal tail domains of the CB1 cannabinoid receptor mediate G protein coupling. Neuroscience 107, 161–167. doi: 10.1016/s0306-4522(01)00335-9
Niehaus, J. L., Liu, Y., Wallis, K. T., Egertova, M., Bhartur, S. G., Mukhopadhyay, S., et al. (2007). CB1 cannabinoid receptor activity is modulated by the cannabinoid receptor interacting protein CRIP 1a. Mol. Pharmacol. 72, 1557–1566. doi: 10.1124/mol.107.039263
Nordstrom, R., and Andersson, H. (2006). Amino-terminal processing of the human cannabinoid receptor 1. J. Recept. Signal Transduct. Res. 26, 259–267. doi: 10.1080/10799890600758252
Nyiri, G., Cserep, C., Szabadits, E., Mackie, K., and Freund, T. F. (2005). CB1 cannabinoid receptors are enriched in the perisynaptic annulus and on preterminal segments of hippocampal GABAergic axons. Neuroscience 136, 811–822. doi: 10.1016/j.neuroscience.2005.01.026
Oddi, S., Dainese, E., Sandiford, S., Fezza, F., Lanuti, M., Chiurchiu, V., et al. (2012). Effects of palmitoylation of Cys(415) in helix 8 of the CB(1) cannabinoid receptor on membrane localization and signalling. Br. J. Pharmacol. 165, 2635–2651. doi: 10.1111/j.1476-5381.2011.01658.x
Oddi, S., Stepniewski, T. M., Totaro, A., Selent, J., Scipioni, L., Dufrusine, B., et al. (2017). Palmitoylation of cysteine 415 of CB1 receptor affects ligand-stimulated internalization and selective interaction with membrane cholesterol and caveolin 1. Biochim. Biophys. Acta Mol. Cell Biol. Lipids 1862, 523–532. doi: 10.1016/j.bbalip.2017.02.004
Oddi, S., Totaro, A., Scipioni, L., Dufrusine, B., Stepniewski, T. M., Selent, J., et al. (2018). Role of palmitoylation of cysteine 415 in functional coupling CB1 receptor to Galphai2 protein. Biotechnol. Appl. Biochem. 65, 16–20. doi: 10.1002/bab.1575
Odorizzi, G., Cowles, C. R., and Emr, S. D. (1998). The AP-3 complex: a coat of many colours. Trends Cell Biol. 8, 282–288. doi: 10.1016/s0962-8924(98)01295-1
Ohno-Shosaku, T., Hashimotodani, Y., Ano, M., Takeda, S., Tsubokawa, H., and Kano, M. (2007). Endocannabinoid signalling triggered by NMDA receptor-mediated calcium entry into rat hippocampal neurons. J. Physiol. 584, 407–418. doi: 10.1113/jphysiol.2007.137505
Ohno-Shosaku, T., Maejima, T., and Kano, M. (2001). Endogenous cannabinoids mediate retrograde signals from depolarized postsynaptic neurons to presynaptic terminals. Neuron 29, 729–738. doi: 10.1016/s0896-6273(01)00247-1
Ohno-Shosaku, T., Shosaku, J., Tsubokawa, H., and Kano, M. (2002). Cooperative endocannabinoid production by neuronal depolarization and group I metabotropic glutamate receptor activation. Eur. J. Neurosci. 15, 953–961. doi: 10.1046/j.1460-9568.2002.01929.x
Pan, X., Ikeda, S. R., and Lewis, D. L. (1996). Rat brain cannabinoid receptor modulates N-type Ca2+ channels in a neuronal expression system. Mol. Pharmacol. 49, 707–714.
Pan, X., Ikeda, S. R., and Lewis, D. L. (1998). SR 141716A acts as an inverse agonist to increase neuronal voltage-dependent Ca2+ currents by reversal of tonic CB1 cannabinoid receptor activity. Mol. Pharmacol. 54, 1064–1072. doi: 10.1124/mol.54.6.1064
Pandey, A., Leblanc, D. M., Parmar, H. B., Pham, T. T. T., Sarker, M., Xu, L., et al. (2019). Structure, amphipathy, and topology of the membrane-proximal helix 8 influence apelin receptor plasma membrane localization. Biochim. Biophys. Acta Biomembr. 1861:183036. doi: 10.1016/j.bbamem.2019.183036
Parmar, V. K., Grinde, E., Mazurkiewicz, J. E., and Herrick-Davis, K. (2017). Beta2-adrenergic receptor homodimers: role of transmembrane domain 1 and helix 8 in dimerization and cell surface expression. Biochim. Biophys. Acta Biomembr. 1859, 1445–1455. doi: 10.1016/j.bbamem.2016.12.007
Pertwee, R. G. (2005). Pharmacological actions of cannabinoids. Handb. Exp. Pharmacol. 2005, 1–51. doi: 10.1007/3-540-26573-2_1
Piserchio, A., Zelesky, V., Yu, J., Taylor, L., Polgar, P., and Mierke, D. F. (2005). Bradykinin B2 receptor signaling: structural and functional characterization of the C-terminus. Biopolymers 80, 367–373. doi: 10.1002/bip.20220
Puighermanal, E., Marsicano, G., Busquets-Garcia, A., Lutz, B., Maldonado, R., and Ozaita, A. (2009). Cannabinoid modulation of hippocampal long-term memory is mediated by mTOR signaling. Nat. Neurosci. 12, 1152–1158. doi: 10.1038/nn.2369
Razani, B., Woodman, S. E., and Lisanti, M. P. (2002). Caveolae: from cell biology to animal physiology. Pharmacol. Rev. 54, 431–467. doi: 10.1124/pr.54.3.431
Robbe, D., Kopf, M., Remaury, A., Bockaert, J., and Manzoni, O. J. (2002). Endogenous cannabinoids mediate long-term synaptic depression in the nucleus accumbens. Proc. Natl. Acad. Sci. U.S.A. 99, 8384–8388. doi: 10.1073/pnas.122149199
Roche, J. P., Bounds, S., Brown, S., and Mackie, K. (1999). A mutation in the second transmembrane region of the CB1 receptor selectively disrupts G protein signaling and prevents receptor internalization. Mol. Pharmacol. 56, 611–618. doi: 10.1124/mol.56.3.611
Rozenfeld, R., and Devi, L. A. (2008). Regulation of CB1 cannabinoid receptor trafficking by the adaptor protein AP-3. FASEB J. 22, 2311–2322. doi: 10.1096/fj.07-102731
Rutz, C., Klein, W., and Schulein, R. (2015). N-terminal signal peptides of g protein-coupled receptors: significance for receptor biosynthesis. Trafficking, and signal transduction. Prog. Mol. Biol. Transl. Sci. 132, 267–287.
Salahpour, A., Angers, S., Mercier, J. F., Lagace, M., Marullo, S., and Bouvier, M. (2004). Homodimerization of the beta2-adrenergic receptor as a prerequisite for cell surface targeting. J. Biol. Chem. 279, 33390–33397. doi: 10.1074/jbc.m403363200
Salazar, G., Love, R., Styers, M. L., Werner, E., Peden, A., Rodriguez, S., et al. (2004). AP-3-dependent mechanisms control the targeting of a chloride channel (ClC-3) in neuronal and non-neuronal cells. J. Biol. Chem. 279, 25430–25439. doi: 10.1074/jbc.m402331200
Sarnataro, D., Grimaldi, C., Pisanti, S., Gazzerro, P., Laezza, C., Zurzolo, C., et al. (2005). Plasma membrane and lysosomal localization of CB1 cannabinoid receptor are dependent on lipid rafts and regulated by anandamide in human breast cancer cells. FEBS Lett. 579, 6343–6349. doi: 10.1016/j.febslet.2005.10.016
Scales, S. J., and Scheller, R. H. (1999). Lipid membranes shape up. Nature 401, 123–124. doi: 10.1038/43582
Sensoy, O., and Weinstein, H. (2015). A mechanistic role of Helix 8 in GPCRs: computational modeling of the dopamine D2 receptor interaction with the GIPC1-PDZ-domain. Biochim. Biophys. Acta 1848, 976–983. doi: 10.1016/j.bbamem.2014.12.002
Shimamura, T., Hiraki, K., Takahashi, N., Hori, T., Ago, H., Masuda, K., et al. (2008). Crystal structure of squid rhodopsin with intracellularly extended cytoplasmic region. J. Biol. Chem. 283, 17753–17756. doi: 10.1074/jbc.c800040200
Simon, A. C., Loverdo, C., Gaffuri, A. L., Urbanski, M., Ladarre, D., Carrel, D., et al. (2013). Activation-dependent plasticity of polarized GPCR distribution on the neuronal surface. J. Mol. Cell Biol. 5, 250–265. doi: 10.1093/jmcb/mjt014
Simonin, F., Karcher, P., Boeuf, J. J., Matifas, A., and Kieffer, B. L. (2004). Identification of a novel family of G protein-coupled receptor associated sorting proteins. J. Neurochem. 89, 766–775. doi: 10.1111/j.1471-4159.2004.02411.x
Sim-Selley, L. J., Schechter, N. S., Rorrer, W. K., Dalton, G. D., Hernandez, J., Martin, B. R., et al. (2006). Prolonged recovery rate of CB1 receptor adaptation after cessation of long-term cannabinoid administration. Mol. Pharmacol. 70, 986–996. doi: 10.1124/mol.105.019612
Singh, S. N., Bakshi, K., Mercier, R. W., Makriyannis, A., and Pavlopoulos, S. (2011). Binding between a distal C-terminus fragment of cannabinoid receptor 1 and arrestin-2. Biochemistry 50, 2223–2234. doi: 10.1021/bi1018144
Smith, T. H., Blume, L. C., Straiker, A., Cox, J. O., David, B. G., Mcvoy, J. R., et al. (2015). Cannabinoid receptor-interacting protein 1a modulates CB1 receptor signaling and regulation. Mol. Pharmacol. 87, 747–765. doi: 10.1124/mol.114.096495
Soltesz, I., Alger, B. E., Kano, M., Lee, S. H., Lovinger, D. M., Ohno-Shosaku, T., et al. (2015). Weeding out bad waves: towards selective cannabinoid circuit control in epilepsy. Nat. Rev. Neurosci. 16, 264–277. doi: 10.1038/nrn3937
Song, C., and Howlett, A. C. (1995). Rat brain cannabinoid receptors are N-linked glycosylated proteins. Life Sci. 56, 1983–1989. doi: 10.1016/0024-3205(95)00179-a
Soria-Gomez, E., Massa, F., Bellocchio, L., Rueda-Orozco, P. E., Ciofi, P., Cota, D., et al. (2014). Cannabinoid type-1 receptors in the paraventricular nucleus of the hypothalamus inhibit stimulated food intake. Neuroscience 263, 46–53. doi: 10.1016/j.neuroscience.2014.01.005
Spomer, L., Gertzen, C. G., Schmitz, B., Haussinger, D., Gohlke, H., and Keitel, V. (2014). A membrane-proximal, C-terminal alpha-helix is required for plasma membrane localization and function of the G Protein-coupled receptor (GPCR) TGR5. J. Biol. Chem. 289, 3689–3702. doi: 10.1074/jbc.m113.502344
Stadel, R., Ahn, K. H., and Kendall, D. A. (2011). The cannabinoid type-1 receptor carboxyl-terminus, more than just a tail. J. Neurochem. 117, 1–18. doi: 10.1111/j.1471-4159.2011.07186.x
Straiker, A., Wager-Miller, J., Hutchens, J., and Mackie, K. (2012a). Differential signalling in human cannabinoid CB1 receptors and their splice variants in autaptic hippocampal neurones. Br. J. Pharmacol. 165, 2660–2671. doi: 10.1111/j.1476-5381.2011.01744.x
Straiker, A., Wager-Miller, J., and Mackie, K. (2012b). The CB1 cannabinoid receptor C-terminus regulates receptor desensitization in autaptic hippocampal neurones. Br. J. Pharmacol. 165, 2652–2659. doi: 10.1111/j.1476-5381.2011.01743.x
Sugiura, H., Iwata, K., Matsuoka, M., Hayashi, H., Takemiya, T., Yasuda, S., et al. (2004). Inhibitory role of endophilin 3 in receptor-mediated endocytosis. J. Biol. Chem. 279, 23343–23348. doi: 10.1074/jbc.m312607200
Tappe-Theodor, A., Agarwal, N., Katona, I., Rubino, T., Martini, L., Swiercz, J., et al. (2007). A molecular basis of analgesic tolerance to cannabinoids. J. Neurosci. 27, 4165–4177. doi: 10.1523/jneurosci.5648-06.2007
Trevaskis, J., Walder, K., Foletta, V., Kerr-Bayles, L., Mcmillan, J., Cooper, A., et al. (2005). Src homology 3-domain growth factor receptor-bound 2-like (endophilin) interacting protein 1, a novel neuronal protein that regulates energy balance. Endocrinology 146, 3757–3764. doi: 10.1210/en.2005-0282
Turu, G., and Hunyady, L. (2010). Signal transduction of the CB1 cannabinoid receptor. J. Mol. Endocrinol. 44, 75–85. doi: 10.1677/jme-08-0190
Turu, G., Simon, A., Gyombolai, P., Szidonya, L., Bagdy, G., Lenkei, Z., et al. (2007). The role of diacylglycerol lipase in constitutive and angiotensin AT1 receptor-stimulated cannabinoid CB1 receptor activity. J. Biol. Chem. 282, 7753–7757. doi: 10.1074/jbc.c600318200
Twitchell, W., Brown, S., and Mackie, K. (1997). Cannabinoids inhibit N- and P/Q-type calcium channels in cultured rat hippocampal neurons. J. Neurophysiol. 78, 43–50. doi: 10.1152/jn.1997.78.1.43
Uchigashima, M., Narushima, M., Fukaya, M., Katona, I., Kano, M., and Watanabe, M. (2007). Subcellular arrangement of molecules for 2-arachidonoyl-glycerol-mediated retrograde signaling and its physiological contribution to synaptic modulation in the striatum. J. Neurosci. 27, 3663–3676. doi: 10.1523/jneurosci.0448-07.2007
Uezu, A., Horiuchi, A., Kanda, K., Kikuchi, N., Umeda, K., Tsujita, K., et al. (2007). SGIP1alpha is an endocytic protein that directly interacts with phospholipids and Eps15. J. Biol. Chem. 282, 26481–26489. doi: 10.1074/jbc.m703815200
Whistler, J. L., Enquist, J., Marley, A., Fong, J., Gladher, F., Tsuruda, P., et al. (2002). Modulation of postendocytic sorting of G protein-coupled receptors. Science 297, 615–620. doi: 10.1126/science.1073308
Wickert, M., Hildick, K. L., Baillie, G. L., Jelinek, R., Aparisi Rey, A., Monory, K., et al. (2018). The F238L point mutation in the cannabinoid type 1 receptor enhances basal endocytosis via lipid rafts. Front. Mol. Neurosci. 11:230. doi: 10.3389/fnmol.2018.00230
Wilhelm, B. G., Mandad, S., Truckenbrodt, S., Krohnert, K., Schafer, C., Rammner, B., et al. (2014). Composition of isolated synaptic boutons reveals the amounts of vesicle trafficking proteins. Science 344, 1023–1028. doi: 10.1126/science.1252884
Wilson, R. I., and Nicoll, R. A. (2001). Endogenous cannabinoids mediate retrograde signalling at hippocampal synapses. Nature 410, 588–592. doi: 10.1038/35069076
Wilson, R. I., and Nicoll, R. A. (2002). Endocannabinoid signaling in the brain. Science 296, 678–682. doi: 10.1126/science.1063545
Wood, S. A., and Brown, W. J. (1992). The morphology but not the function of endosomes and lysosomes is altered by brefeldin A. J. Cell Biol. 119, 273–285. doi: 10.1083/jcb.119.2.273
Wu, D. F., Yang, L. Q., Goschke, A., Stumm, R., Brandenburg, L. O., Liang, Y. J., et al. (2008). Role of receptor internalization in the agonist-induced desensitization of cannabinoid type 1 receptors. J. Neurochem. 104, 1132–1143. doi: 10.1111/j.1471-4159.2007.05063.x
Yang, H. S., Sun, N., Zhao, X., Kim, H. R., Park, H. J., Kim, K. M., et al. (2019). Role of helix 8 in dopamine receptor signaling. Biomol. Ther. 27, 514–521.
Yoshida, T., Fukaya, M., Uchigashima, M., Miura, E., Kamiya, H., Kano, M., et al. (2006). Localization of diacylglycerol lipase-alpha around postsynaptic spine suggests close proximity between production site of an endocannabinoid, 2-arachidonoyl-glycerol, and presynaptic cannabinoid CB1 receptor. J. Neurosci. 26, 4740–4751. doi: 10.1523/jneurosci.0054-06.2006
Keywords: endocannabinoid system, cannabinoid type 1 receptor, trafficking, protein-protein interactions, synaptic regulation, retrograde synaptic signaling
Citation: Fletcher-Jones A, Hildick KL, Evans AJ, Nakamura Y, Henley JM and Wilkinson KA (2020) Protein Interactors and Trafficking Pathways That Regulate the Cannabinoid Type 1 Receptor (CB1R). Front. Mol. Neurosci. 13:108. doi: 10.3389/fnmol.2020.00108
Received: 06 April 2020; Accepted: 20 May 2020;
Published: 12 June 2020.
Edited by:
István Katona, Institute of Experimental Medicine (MTA), HungaryReviewed by:
Robert B. Laprairie, University of Saskatchewan, CanadaKenneth Mackie, Indiana University Bloomington, United States
Copyright © 2020 Fletcher-Jones, Hildick, Evans, Nakamura, Henley and Wilkinson. This is an open-access article distributed under the terms of the Creative Commons Attribution License (CC BY). The use, distribution or reproduction in other forums is permitted, provided the original author(s) and the copyright owner(s) are credited and that the original publication in this journal is cited, in accordance with accepted academic practice. No use, distribution or reproduction is permitted which does not comply with these terms.
*Correspondence: Alexandra Fletcher-Jones, alex.fletcher-jones@inserm.fr; Jeremy M. Henley, j.m.henley@bristol.ac.uk; Kevin A. Wilkinson, kevin.wilkinson@bristol.ac.uk
†Present address: Alexandra Fletcher-Jones, Neurocentre Magendie, INSERM 1215, Bordeaux, France; Ashley J. Evans, Drug Discovery Unit, Cancer Research UK Manchester Institute, The University of Manchester, Macclesfield, United Kingdom