- Department of Physiology & Membrane Biology and Institute for Pediatric Regenerative Medicine, Shriners Hospital for Children, University of California Davis School of Medicine, Sacramento, CA, United States
Ion channels are expressed throughout nervous system development. The type and diversity of conductances and gating mechanisms vary at different developmental stages and with the progressive maturational status of neural cells. The variety of ion channels allows for distinct signaling mechanisms in developing neural cells that in turn regulate the needed cellular processes taking place during each developmental period. These include neural cell proliferation and neuronal differentiation, which are crucial for developmental events ranging from the earliest steps of morphogenesis of the neural tube through the establishment of neuronal circuits. Here, we compile studies assessing the ontogeny of ionic currents in the developing nervous system. We then review work demonstrating a role for ion channels in neural tube formation, to underscore the necessity of the signaling downstream ion channels even at the earliest stages of neural development. We discuss the function of ion channels in neural cell proliferation and neuronal differentiation and conclude with how the regulation of all these morphogenetic and cellular processes by electrical activity enables the appropriate development of the nervous system and the establishment of functional circuits adapted to respond to a changing environment.
Introduction
Nervous system development is a complex process in which neural cells undergo a transformation from neural stem cells to highly specialized neurons and glia to form different brain structures and spinal cord and establish circuitry that facilitates simple to advanced neural functions.
Many cues have been recognized as drivers of the first steps in nervous system development. Morphogenetic proteins and growth factors regulate the number and type of neural cells as well as the morphogenesis of the neural tube. These include Sonic hedgehog (Shh), Bone Morphogenetic Proteins (BMPs), Wnts and trophic factors such as EGF, IGF, NGF, BDNF to mention few. Most of these factors are not exclusive to the organogenesis of the brain and spinal cord but instead support growth and act as morphogens of every tissue and organ in the developing embryo. Mechanistically, these developmental cues trigger a transcriptional combinatorial code that accompanies cells through their cell cycle progression and exit, differentiation and maturation (Cohen et al., 2013; Bier and De Robertis, 2015).
Also, some of the pathways triggered by these proteins intertwine with second messenger signaling, like those driven by spatiotemporal changes in cAMP, inositol triphosphate (IP3) and Ca2+ concentrations, through the recruitment of specialized enzymes that imprint posttranslational modifications in effector proteins (Borodinsky et al., 2015). For instance, in embryonic Xenopus spinal cord opposing gradients of BMPs and Shh regulate neuronal differentiation across the dorsoventral axis by modulating the frequency of Ca2+ transients in developing neurons (Belgacem and Borodinsky, 2011; Swapna and Borodinsky, 2012). While Shh increases Ca2+ spike activity through recruiting transient receptor potential channels (TRPC) and IP3 receptor-operated Ca2+ release from stores in ventral domains of the spinal cord (Belgacem and Borodinsky, 2011, 2015), BMPs decrease Ca2+ spike activity of dorsal neurons through the activation of p38 MAP kinase and inhibition of Na+ conductance necessary for activating voltage-gated Ca2+ channels (Swapna and Borodinsky, 2012). Similarly, morphogenetic proteins of the Wnt family acting through non-canonical pathways (Slusarski et al., 1997; Sheldahl et al., 1999) regulate neuromorphogenesis. Specifically, Wnt5a recruits the receptors Frizzled and Ryk that trigger Ca2+ transients mediated by TRPC and IP3 receptors to regulate axon growth and guidance of rodent corticospinal neurons grown in vitro (Li et al., 2009). All these studies share a common effector that is Ca2+ dynamics. This indicates that neural activity, a modifier of [Ca2+]i, might also be a driving force for neural development either in concert or independently of morphogenetic protein actions.
Neural activity is a feature of the maturing and mature nervous system, which during development facilitates the refinement of neural connections. The expression of ion channels in mature neurons is intrinsic to neuronal function. Diverse ion conductances are indispensable for neurotransmission, thus, the roles of different ion channels in synaptic function and neuronal excitability have been extensively studied. In contrast, the neurophysiological features of neural cells before synapse formation and before neuronal differentiation has not been as strong a focus of attention as those of mature neurons. Nevertheless, studies have argued that other forms of neural activity are present in neural cells throughout nervous system development (Spitzer, 2006; Smith and Walsh, 2020).
This activity may not be structured under a classical chemical synapse, but it is certainly dependent on ion channels gated by diverse mechanisms. Expression of voltage- and neurotransmitter-gated ion channels as well as transient receptor potential (TRP) channels, among others, is apparent in neural stem cells as early as neural plate stages (Abdul-Wajid et al., 2015; Sequerra et al., 2018; Spencer et al., 2019). Moreover, ion channels have been shown to participate in the formation of the brain and spinal cord during one of the first developmental steps known as neural tube formation (Abdul-Wajid et al., 2015; Sequerra et al., 2018).
Here, we review studies addressing the pattern of expression of ion channels during development in neural cells before and during synapse formation. We compile investigations demonstrating a role for ion channels in neural cell proliferation, neural tube formation, and neuronal differentiation and discuss the consequences of having neural activity functioning in the early stages of nervous system development.
Ontogeny of Ion Channel Expression in Excitable Tissues
The excitable nature of neurons and muscle cells is dependent on the specific expression of ion channels and their subcellular localization in these cells. Seminal studies have investigated the developmental appearance of excitability in neurons and muscle cells through the progressive and differential expression of ion channels. Embryonic spinal cord neurons have served as a powerful model for the study of the ontogeny of excitability during development. Action potentials in Xenopus laevis spinal cord neurons are first recorded 8 h after exiting the cell cycle, when, these events manifest spontaneously, are Ca2+-dependent and long in duration (Spitzer and Lamborghini, 1976; Holliday and Spitzer, 1990; Gu et al., 1994; Gu and Spitzer, 1995). Developmental upregulation in the expression of an inward rectifier voltage-gated K+ channel contributes to shorten the action potential duration and shifts it from Ca2+- to Na+-mediated (Barish, 1986; O’Dowd et al., 1988). The identity of specific Ca2+, Na+ and K+ voltage-gated channel subunits for which their expression is developmentally regulated have been investigated (Harris, 1988; Ribera and Spitzer, 1992; Spitzer and Ribera, 1998). In particular, Kv1.1 and Kv2.2 appear progressively and respectively in immature and mature spinal cord neurons to contribute to the increased K+ current as development advances (Gurantz et al., 1996). Similarly, studies in other species have shown developmentally-regulated expression of ion channels during spinal cord neuron differentiation that results in the progressive appearance of ionic currents in these neurons. For instance, T-type Ca2+ currents are dominant at the earliest embryonic stage of chick limb motor neuron development, while later T currents decrease and N and L Ca2+ currents increase (McCobb et al., 1989). Moreover, changes in Na+ and K+ currents in these motor neurons during embryonic development result in changes in action potential amplitude and duration, respectively, which in turn, modify the instructions of motor neurons to the muscle (McCobb et al., 1990).
In addition to voltage-gated ion channels and their fundamental role in contributing to the excitability of developing neurons and muscle cells, other types of ion channels are also present at the early stages of embryonic development. These channels are gated by diverse mechanisms, including notably, neurotransmitter-operated channels. GABA and glutamate receptors are expressed in immature Xenopus spinal cord neurons and their activation contributes to the spontaneous Ca2+ spike activity in these cells before and during synapse formation (Root et al., 2008). Glutamate-operated channels are involved in the electrical coupling of developing mouse motor neurons (Personius et al., 2008). Expression of subunits of acetylcholine-gated channels, is developmentally regulated in chicken motor neurons and skeletal muscle (Keiger et al., 2003). Several subunits are expressed in motor neurons and muscle before muscle innervation and others are downregulated after completion of apoptosis of developing motor neurons (Keiger et al., 2003). Similarly, NMDA receptors are present and active at the neuromuscular junction during motor neuron axon pruning in early postnatal mouse development (Personius et al., 2016).
Our recently published study shows that the cold-sensitive channel TRPM8 is expressed in the developing Xenopus embryo (Spencer et al., 2019). Both mRNA and protein are detected since the early stages of neural tube formation (Spencer et al., 2019) and transcripts are enriched in neural tissue (Session et al., 2016). During spinal neuron differentiation, TRPM8 protein appears enriched in the ventral domain of the embryonic spinal cord and makes a major contribution to the calcium spike activity of ventral spinal cord neurons at cold temperatures (Spencer et al., 2019). Similarly, in other species developing spinal cord neurons also express temperature-sensitive ion channels including motor neurons, which express TRPV2 that regulates axon outgrowth (Shibasaki et al., 2010), and in early postnatal mouse motor neurons contributes to their electrical properties (Bouhadfane et al., 2013).
Expression of these diverse types of channels appears to start at even earlier stages of neural development, before the neural tube is formed. At neural plate stages, Xenopus neuroectodermal cells exhibit Ca2+ transients (Abdul-Wajid et al., 2015; Christodoulou and Skourides, 2015; Sequerra et al., 2018) that are mediated partially by T-type Ca2+ channels (Abdul-Wajid et al., 2015) and by NMDA receptors, as demonstrated by our recently published study (Sequerra et al., 2018).
Transcripts and proteins for glutamate (Root et al., 2008; Session et al., 2016; Sequerra et al., 2018) and GABA (Barker et al., 1998; Root et al., 2008; Session et al., 2016) release and reception, among many other neurotransmitters (Choi et al., 1998; Messenger et al., 1999), are detected during neural plate stages. Accordingly, the role of neurotransmitter signaling and ion channels in neural tube formation demands further investigation.
Neural Tube Formation and Ion Channels
The process of neural tube formation consists of transforming a flat layer of cells known as the neural plate into a tubular structure from which the brain and spinal cord originate. The cellular events encompassing neural tube morphogenesis, all of which are tightly regulated, include neural plate cell proliferation, apicobasal polarization, apical constriction, elongation, cell intercalation, migration and differentiation (Wallingford et al., 2013; Nikolopoulou et al., 2017). Intriguingly, the use of antiepileptic drugs (AEDs) during pregnancy increases the incidence of neural tube defects (NTDs) by unclear mechanisms (Robert and Guibaud, 1982; Lindhout and Schmidt, 1986; Rosa, 1991).
Our recently published study (Sequerra et al., 2018) shows that glutamate signaling is present during neural plate stages in Xenopus laevis embryos. We demonstrated that during neural tube formation neural plate cells exhibit Ca2+ transients partly mediated by NMDA receptors. Inhibiting glutamate signaling, through pharmacological inhibition of NMDA receptors or downregulation of the GluN1 subunit, induces NTDs (Figure 1). Valproic acid, an AED known to increase the incidence of NTDs in humans and animal models (Rosa, 1991; Lindhout et al., 1992; Padmanabhan and Ahmed, 1996), also inhibits Ca2+ dynamics in the neural plate to a similar extent as inhibition of NMDA receptors. Moreover, preincubating embryos with NMDA partially rescues both the number of Ca2+ transients in the folding neural plate and the valproic acid-induced NTD phenotype (Sequerra et al., 2018). Additionally, both valproic acid- and deficient NMDA receptor signaling-induced NTDs are completely rescued by enhancing ERK1/2 activation (Sequerra et al., 2018). These findings demonstrate that neurotransmitter signaling is present during the earliest stages of nervous system development and is fundamental for the morphogenesis of the neural tube (Figure 1). These discoveries suggest that primary targets of AEDs are already present and functional in neural plate stages. Thus, exposure of the fetus to these drugs during the critical period of neural tube formation may interfere with necessary neural activity and signaling leading to NTDs.
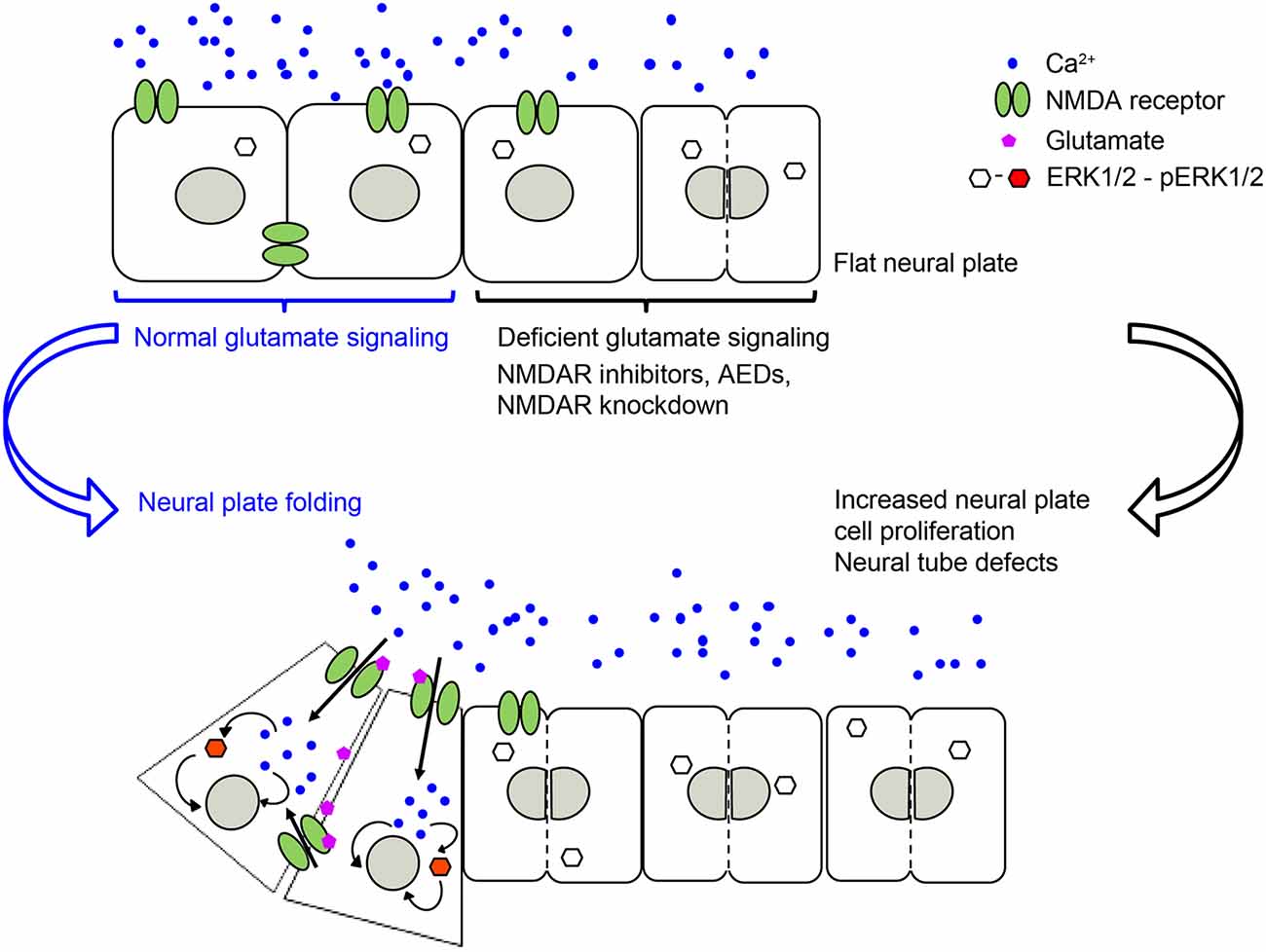
Figure 1. NMDA receptor-mediated signaling is necessary for regulating neural plate cell proliferation in neurulating Xenopus laevis embryos, which in turn is required for appropriate morphogenesis of the neural tube. Genetic or pharmacological inhibition of NMDA receptor activity, including antiepileptic drugs (AEDs) leads to an increase in proliferating neural plate cells, which in turn leads to neural tube defects (NTDs). Based on Sequerra et al. (2018).
Many other neurotransmitter signaling systems have been identified as participants in the process of neural tube formation. Inhibiting serotonin receptors 5HT2B interferes with mouse neural tube closure and morphogenesis (Choi et al., 1998). The expression of these receptors during neural tube formation appears enriched in neural crest cells, which also explains morphological defects of the developing heart (Choi et al., 1998).
Administering GABAA and GABAB receptor ligands to pregnant rats alters embryos’ neural tube formation leading to NTDs (Briner, 2001). The fact that both agonists and antagonists of GABA receptors elicit these defects suggest that balanced signaling is required for the appropriate morphogenesis of the neural tube. Additionally, incubating elevated-neural-fold stage rat embryos with diazepam for 24 h prevents neural tube closure (Smedley and Stanisstreet, 1986), which may result from targeting the benzodiazepine domain of GABAA receptors. Another chloride conductance-mediated neurotransmitter system that affects neural tube formation is glycine. Blocking glycinergic signaling by administering strychnine to pregnant rats results in embryos with anencephaly, anterior NTD (García-Alcocer et al., 2005).
Similarly, enhancing or inhibiting NO levels by enhancing BMP signaling or inhibiting NO synthase in chicken embryos induces NTDs (Traister et al., 2004). Low NO levels appear to facilitate neural plate cell proliferation and to decrease apoptosis, and vice-versa when NO levels are high. Hence, NO signaling dynamically regulates the number of neural plate cells that in turn is important for neural tube morphogenesis (Traister et al., 2004).
Noradrenaline promotes neuronal differentiation by upregulating expression of N-tubulin in noggin-expressing neural plate cells, which is prevented by inhibiting α-adrenergic receptors (Messenger et al., 1999). Noradrenergic signaling in the neuroectoderm promotes further neuronal differentiation by enabling the expression of dedicated neurofilament-associated protein related to the acquisition of specialized neuronal morphology and function (Messenger et al., 1999).
Ca2+ signaling is a plausible common denominator for the action of diverse neurotransmitter systems on neural tube formation. Sources of Ca2+ can be intracellular from stores or extracellular through Ca2+ influx. Early studies in cultured rat embryos during cephalic neural fold elevation and neural tube closure assessed the role of Ca2+ influx and found that reducing it causes opening of the elevated neural folds (Smedley and Stanisstreet, 1986). We found that inhibiting the NMDA receptor function decreases the number of Ca2+ transients in the neural plate, suggesting that additional mechanisms other than these ionotropic glutamate receptors contribute to neural plate cell Ca2+ dynamics during folding (Sequerra et al., 2018). An interesting pattern of ionic current is present in the folding neural plate in Xenopus laevis embryos consisting of a Na+-dependent inward current which is stronger in the mid-lateral neural plate and decreases near the midline of the neural groove (Robinson and Stump, 1984). Similarly, a comparable mediolateral pattern of resting membrane potential is observed during neural plate folding in this species (Pai et al., 2015). Disruption of this membrane potential pattern, by overexpression of the potassium channel Kv1.5 or GlyR and incubation with chloride channel agonist, leads to defects in brain morphogenesis (Pai et al., 2015). The level of membrane polarization, the makeup of ion channels expressed in the neural plate and the exposure to mediators or modulators of neurotransmitter signaling will differentially recruit Ca2+ currents with characteristic kinetic parameters to transduce the signaling required for neural tube morphogenesis. Indeed, T-type Ca2+ channels are involved in neural tube formation and loss of them impairs neural fold closure in Xenopus laevis and Ciona embryos. Ca2+ influx through these channels is necessary for the regulation of cell adhesion during neural tube formation by ephrin signaling (Abdul-Wajid et al., 2015). Furthermore, these Ca2+ transients seem to regulate apical actin dynamics in superficial neural plate cells of Xenopus laevis embryos, which, in turn, regulates neural plate cell apical constriction necessary for neural tube formation (Christodoulou and Skourides, 2015).
Further investigation is needed to identify the molecular mechanisms eliciting Ca2+ signaling and downstream effectors recruited for neural plate folding and neural tube formation. The elucidation of these mechanisms will contribute to the delineation of safe therapies for the treatment of epilepsy during pregnancy.
Neural Cell Proliferation and Ion Channels
The generation of the appropriate number of neurons and glial cells is essential not only during nervous system development but also in the adult brain where neurogenesis occurs in the hippocampus and olfactory bulb, and the peripheral nervous system during regeneration and remodeling. Thus, this is a highly regulated process because the dysregulated proliferation of neural stem cells can lead from tumors to neurodevelopmental disorders and birth defects like NTDs.
The expression of ion channels during the early stages of development supports a role for them in the relevant cellular processes pertinent to these stages including neural cell proliferation. Different types of ion channels including voltage-gated, neurotransmitter-gated, TRPC and store-operated Ca2+ channels have all been implicated in regulating neural plate cell proliferation.
The action of glutamate-mediated regulation of neural plate cell proliferation is apparent as early as neural plate stages. We found that blocking NMDA receptor signaling increases neural plate cell proliferation in Xenopus embryos, and, likely as a consequence, impairs lateromedial migration leading to NTDs. An increase in neural plate cell proliferation is also apparent by incubating embryos with the AED valproic acid (Sequerra et al., 2018), in contrast to the off-target inhibitory effect of valproic acid on cell proliferation as an inhibitor of histone deacetylase (Lane and Chabner, 2009). Altogether, these studies suggest that it is the AED action on its primary targets and its interference with neural activity that is responsible for inducing NTDs due to enhancing neural plate cell proliferation.
Regulation of neural plate cell proliferation by glutamate-gated ion channels is present at later developmental stages during corticogenesis in the rodent brain. The effects of glutamate signaling on neural progenitor proliferation vary depending on the nervous system structure, the developmental stage and the type of model system and manipulation used in specific studies. Glutamate decreases the number of proliferating embryonic rat cortical cells through an AMPA/Kainate receptor-dependent mechanism that leads to depolarization of neural progenitors in the ventricular zone and activation of voltage-gated Ca2+ channels (LoTurco et al., 1995). In contrast, during embryonic rat striatal neurogenesis, it has been shown that glutamate signaling increases neural progenitor proliferation by an NMDA receptor-dependent mechanism in the ventral telencephalon (Luk et al., 2003). In vitro studies on embryonic rat hippocampal neural progenitors reveal that stimulating glutamate signaling enhances neural cell proliferation or neuronal differentiation depending on the temporal pattern of NMDA receptor activation (Joo et al., 2007), suggesting that the apparent discrepancies between studies focused on different brain regions, developmental stages and experimental preparations might be rooted in different spatiotemporal profiles of downstream glutamate signaling.
Another important neurotransmitter-gated ion channel that participates in neural progenitor cell proliferation is the GABAA receptor. In vivo studies in the neonatal mouse subventricular zone (Young et al., 2012) and in vitro studies in cerebellar granule cells (Fiszman et al., 1999) show that GABAA receptor-induced depolarization enhances neural progenitor cell proliferation. In contrast, activation of this receptor in the ventricular zone of the rat embryonic neocortex (LoTurco et al., 1995) and isolated neural precursor cells of the early postnatal rat striatum (Nguyen et al., 2003) show that GABA signaling recruits voltage-gated Ca2+ channels and inhibits cell cycle progression. Even in the adult mouse brain, the GABAA receptor seems crucial in regulating neurogenesis by controlling hippocampal neural progenitors. GABAA receptor γ2 subunit-mediated signaling is responsible for controlling the experience-dependent transition between quiescence vs. proliferative states of the mouse hippocampal neural stem cell niche (Song et al., 2012). In particular, γ2-GABAA receptor favors the quiescence of adult neural stem cells (Song et al., 2012). Moreover, a diazepam binding inhibitor is expressed in neural stem cells of the postnatal hippocampal subgranular zone and enhances proliferation by dampening GABAA receptor signaling (Dumitru et al., 2017). All of these studies clearly demonstrate a role for glutamate- and GABA-gated ion channels in regulating neural progenitor proliferation.
Other ion channels directly involved in regulating neural cell proliferation include the voltage-gated ion channels. Moreover, some actions of the neurotransmitter receptor-gated ion channels seem to converge into the recruitment of voltage-gated ion channels via membrane depolarization. In particular, voltage-gated Ca2+ channels are pivotal for the regulation of neural progenitor proliferation and mouse embryonic cortical layer formation (Malmersjö et al., 2013). Voltage-gated Ca2+ channels enable Ca2+ transients that propagate through a network of neural progenitors connected by gap junctions. Inhibiting this electrotonic transmission decreases neural progenitor proliferation suggesting that correlated Ca2+ transients are necessary for the regulation of neural progenitor proliferation (Malmersjö et al., 2013). In contrast, in frog embryos membrane hyperpolarization that presumably impedes activation of voltage-gated Ca2+ channels in embryonic neural cells increases neural cell proliferation that has detrimental consequences to brain morphogenesis (Pai et al., 2015). These studies suggest that as the differential impact glutamate signaling has on neural proliferation and differentiation, the impact voltage-gated Ca2+ channel-mediated signaling has, depends on developmental timing and location.
In addition to Ca2+ channels, voltage-gated K+ and Na+ channels are involved in regulating neural cell proliferation. The voltage-gated Na+ channel β1 subunit is necessary for inhibiting granule cell precursor proliferation during the first week of mouse postnatal dentate gyrus development (Brackenbury et al., 2013). Another instance of the role of voltage-gated Na+ channels was discovered recently in Drosophila larvae, where the single pore-forming voltage-gated Na+ channel α subunit, paralytic, regulates neural progenitor proliferation and survival (Piggott et al., 2019). In the adult nervous system the epithelial Na+ channel regulates neural stem cell proliferation in the subependymal zone and consequently neurogenesis in the mouse olfactory bulb (Petrik et al., 2018). In zebrafish, the homolog of voltage-gated K+ channel α-subunit Kv6.4 regulates the proliferation of cells lining the embryonic brain ventricles (Shen et al., 2016). A gain-of-function mutation of this subunit decreases cell proliferation, while the loss of Kv6.4 increases it. Moreover, the Kv6.4 action appears antagonized by the expression of a homolog to the delayed rectifier K+ channel subunit Kv2.1, for which gain and loss of function manipulations cause the opposite effects on neural progenitor proliferation and ventricular brain development (Shen et al., 2016).
Non-voltage-gated ion channels have also been implicated in regulating neural cell proliferation. For instance, TRPC1 participates in bFGF/FGFR1-mediated proliferation of embryonic rat neural stem cells through a Ca2+-dependent mechanism (Fiorio Pla et al., 2005). Similarly, TRPC1 mediates the proliferative effect of PDGF-BB on rat hippocampal neuronal progenitors, which rescues the detrimental effect on neurogenesis inflicted by HIV infection and cocaine (Yao et al., 2012). Embryonic and adult mouse neural stem cells express Ca2+ release-activated Ca2+ channels that enable store-operated Ca2+ entry in these cells mediated by Orai1 and STIM1. Downregulating the expression of these molecules decreases in vitro and in vivo proliferation of neural stem cells (Somasundaram et al., 2014).
The specific effect that is triggered downstream of all these ion channels on cell proliferation varies among these studies. This is likely due to differences in downstream signaling, recruitment of molecular partners, environmental milieu and maturational status of the cells subjected to these ion channel-triggered signaling mechanisms. There are many downstream signaling effectors reported to mediate ion channel-dependent regulation of neural cell proliferation. For instance, downstream of voltage-gated K+ channels and membrane depolarization, oligodendrocyte progenitor proliferation is regulated by controlling the progression of the cell cycle at the G1 phase, likely through a cAMP and cyclin-dependent kinase inhibitors p27Kip1 and p21CIP1 mechanism (Ghiani et al., 1999). In contrast, downstream of GABAA receptor-triggered hyperpolarization in embryonic and neural crest stem cells, S-phase checkpoint kinases of the phosphatidylinositol-3-OH kinase-related kinase family and, subsequently the histone variant H2AX, regulate proliferation (Andäng et al., 2008). Recruitment of the ERK pathway is a shared effector of several ion channel-regulated neural cell proliferation pathways. TRPC1 activation is necessary for the recruitment of PDGF-BB-induced ERK/CREB and mTOR/eukaryotic translation initiation factor 4E-binding protein-p70S6K and nuclear factor-κB in rat hippocampal neural progenitors (Yao et al., 2012). Similarly, activation of the epithelial Na+ channel by fluid flow elicits Ca2+ dynamics through Ca2+ release-activated channels that activate ERK, regulating neural cell proliferation in the mouse subependymal zone in the olfactory bulb (Petrik et al., 2018). Additionally, stimulation of the NMDA receptor in vivo activates ERK in neural plate cells during Xenopus neural tube formation. In turn, constitutive activation of ERK during neural plate folding completely rescues the NTD phenotype induced by dysregulated neural plate cell proliferation due to blocking NMDA receptors or incubation with the AED valproic acid (Sequerra et al., 2018; Figure 1).
Neuronal Differentiation and Ion Channels
It has been long recognized that expression of ion channels and prominently voltage-gated ion channels, their density, clustering, and subcellular localization are at the core of what distinguishes a neuron from other cell types. This fundamental question was addressed by pioneering studies from the Mandel lab when they cloned the transcription factor REST and identified it as a silencer element active in nonneuronal cells. In contrast, the absence of REST in neurons allows for the expression of the type II Na+ channel, which in turn assigns a functional neuronal identity to developing nervous system cells (Maue et al., 1990; Kraner et al., 1992; Chong et al., 1995). In turn, the specific makeup of ion channels expressed by developing neurons is essential for the different aspects of neuronal differentiation, including the acquisition of appropriate morphology, specification of neurotransmitter phenotype, axonal and dendritic growth and pathfinding, synaptogenesis and establishment of connections with corresponding partners, and physiological features that contribute to the precise characteristics of the developing circuit in which each differentiating neuron is meant to participate. Work from the Spitzer lab identified that Ca2+ spikes generated by voltage-gated Ca2+ and Na+ channels in embryonic neurons (Gu et al., 1994) regulate the specification of neurotransmitter phenotype that spinal cord mature neurons exhibit at larval stages of Xenopus laevis development (Gu and Spitzer, 1995; Borodinsky et al., 2004). These studies demonstrated that the frequency of Ca2+ spikes that embryonic neurons exhibit is important for the type of neurotransmitter spinal cord neurons express, following a homeostatic rule by which higher spike frequency leads to expression of inhibitory neurotransmitters while suppression of these spikes results in expression of excitatory neurotransmitters (Borodinsky et al., 2004). They also found that Ca2+ waves, dependent on both extracellular Ca2+ and Ca2+ release from ion channels present in intracellular Ca2+ stores such as ryanodine receptors, are apparent in growth cones of developing neurons (Gu et al., 1994) and are important for axon extension and guidance (Gu et al., 1994; Gu and Spitzer, 1995; Gomez and Spitzer, 1999).
K+ channels have been at the center of the process of neuronal differentiation in a variety of central nervous system structures and species. Weaver mice carry a mutation in a G-protein coupled inward rectifier K+ channel, GIRK2, that affects the pore-forming domain of the protein and impairs cerebellar granule neuron differentiation immediately after cell cycle exit (Patil et al., 1995). Expression of K+ channels, in turn, is a key determinant of the spontaneous Ca2+ activity that developing neurons exhibit. For instance, K+ channels like the small conductance Ca2+-activated K+ channel SK2 are expressed in immature Purkinje cells in the postnatal rat cerebellum and establish a feedback loop with Ca2+ influx and activation of Ca2+ channels to regulate the spatiotemporal features of Ca2+ transients in developing neurons (Cingolani et al., 2002). In particular, the developmentally regulated expression of SK2 elicits an after hyperpolarizing current in immature Purkinje neurons regulating their firing pattern during neuronal differentiation (Patil et al., 1995). Similarly, the acquisition of a mature neuronal action potential during spinal cord neuron differentiation is dependent on the expression of a delayed rectifier K+ channel during a critical period of development (Ribera and Spitzer, 1989, 1992).
While all these studies are focused on specific ion channels and their role in different aspects of neuronal differentiation, they converge on shaping the spontaneous Ca2+ activity that differentiating neurons exhibit during development either directly or indirectly. Another instance of direct regulation of Ca2+ activity in developing neurons that connects the process of differentiation with the environment in which embryos develop is represented by the role of TRPM8, cold-sensitive channel, in spinal cord neuron differentiation (Spencer et al., 2019; Figure 2). Activation of TRPM8 at cold temperature increases Ca2+ spike frequency in ventral spinal cord neurons of Xenopus laevis embryos. In turn, Ca2+ spike activity regulates the expression of Hb9 (Spencer et al., 2019), a transcription factor known to be necessary for motor neuron phenotype specification and maintenance (Arber et al., 1999; Thaler et al., 1999). As a result, embryos grown at cold temperatures develop into larvae that possess a higher number of differentiated motor neurons innervating the axial musculature (Spencer et al., 2019; Figure 2). Similarly, mushroom body Kenyon neurons from Drosophila flies raised in high temperatures exhibit an increase in high-order axonal branching that is dependent on temperature-dependent excitability of these developing neurons mediated by Na+, Ca2+, and K+ channels and the downstream interaction between Ca2+ and cAMP dynamics (Peng et al., 2007).
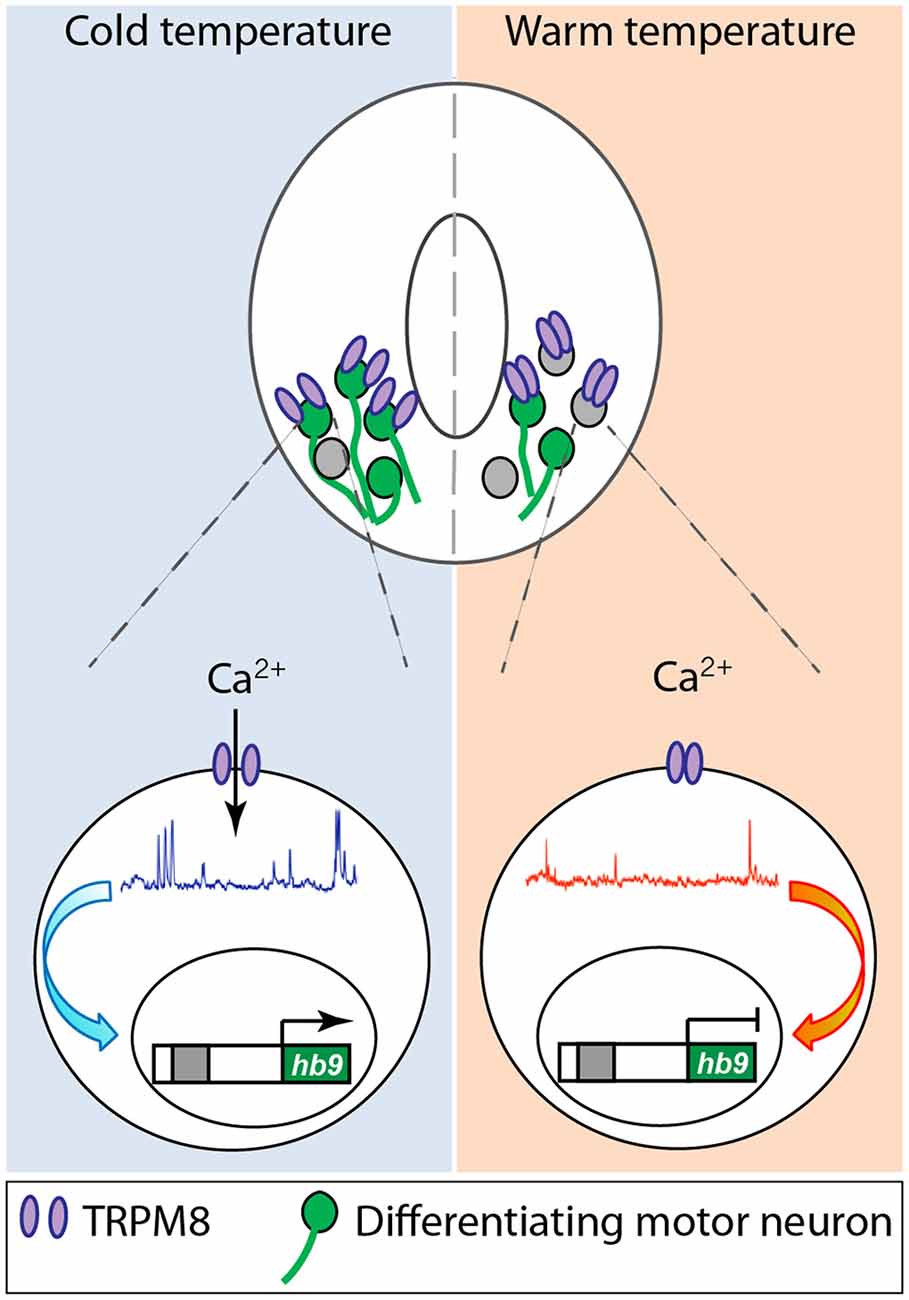
Figure 2. Cold temperature activates TRPM8 expressed in embryonic Xenopus laevis ventral spinal cord neurons, increasing Ca2+ spike frequency that upregulates expression of the motor neuron phenotype master transcription factor hb9, resulting in an increase in number of motor neurons in animals grown at cold temperature. Based on Spencer et al. (2019).
Neurotransmitter-gated channels that regulate neuronal differentiation also involve Ca2+ dynamics downstream of neurotransmitter receptor activation. NMDA receptor function is necessary for the development of dendritic arbors in differentiating retinotectal neurons and to establish appropriate retinotectal topographic maps (Cline and Constantine-Paton, 1989; Rajan and Cline, 1998). The role of NMDA receptors appears to be specific to this glutamate-gated channel and not to its participation in overall neural activity since enhancing NMDA receptor function by increasing the levels of co-agonist D-serine without affecting glutamatergic neurotransmission alters synaptic maturation through hyperstabilization of axon branches in the developing frog visual system (Van Horn et al., 2017). Similarly, GABAergic depolarizing signals during rodent embryonic cortical neuron development recruit L-type Ca2+ channels to regulate neuritogenesis (Maric et al., 2001). Moreover, eliminating this depolarizing action of GABA in a subset of rat ventricular progenitors and the cortical neurons derived from them impairs their morphological maturation (Cancedda et al., 2007).
Neurotransmitter modulation of neuronal differentiation through direct or indirect regulation of ion channel activity is a function shared by neurotrophic factors. Neurotrophin 3 signaling regulates the specification of neuronal phenotype through a voltage-gated Ca2+ channel-dependent mechanism that results in a higher number of calbindin-expressing mouse hippocampal pyramidal neurons when Neurotrophin-3 signaling is enhanced and lower when it is decreased (Boukhaddaoui et al., 2001). Brain-derived neurotrophic factor regulates axonal pathfinding during neuronal differentiation by eliciting transient increases in [Ca2+]i in growth cones of rat cerebellar granule cells and Xenopus spinal cord neurons through the recruitment of TRPC channel activity (Li et al., 2005; Shim et al., 2005; Wang and Poo, 2005).
Ion channels can be mechanically gated and participate in the differentiation of developing neurons. Piezo 1, a mechanosensitive channel, mediates axonal growth and pathfinding of Xenopus retinal ganglion cells that direct their growth towards softer tissue (Koser et al., 2016).
Voltage-gated Na+ channels are involved in regulating axonal outgrowth and morphology. In zebrafish, knockdown of the Nav1.6a alters axonal outgrowth and morphology of dorsally and ventrally projecting secondary motor neurons (Pineda and Ribera, 2008).
Downstream of channel activity local and whole-cell effectors are recruited to change, for example, directionality and growth rate of neurites and neurotransmitter specification, respectively. Ca2+ transients recruit activity-dependent transcription factors like CREB (Belgacem and Borodinsky, 2015) or cJUN (Marek et al., 2010) to regulate neurotransmitter phenotype expression in spinal cord neurons. L-type Ca2+ channels are recruited downstream of the clustering of the neural cell adhesion molecule 2, inducing submembrane Ca2+ spikes that in turn activate the protein tyrosine kinase c-Src, which results in CaMKII activation and increases in filopodia density, neurite outgrowth and branching (Sheng et al., 2015).
Alternatively, ion channels may trigger downstream signaling relevant for neuronal differentiation independently of ion permeation. For example, in some instances, the channel itself regulates transcription, as shown for the C-terminal fragment of Cav1.2 that translocates to the nucleus and acts as a transcription factor controlling rat neuronal differentiation (Gomez-Ospina et al., 2006). Remarkably, a point mutation in this channel that causes Timothy Syndrome, a neurodevelopmental disorder within the autism spectrum disorders, alters dendrite dynamics independent of Ca2+ permeation and is dependent on localized RhoA activation (Krey et al., 2013). This mutation appears to interfere with a developmental switch in alternative splicing of this channel, leading to persistent expression of gain of function mutant channels that result in a Ca2+-dependent imbalance in the numbers of subtypes of differentiated cortical neurons (Panagiotakos et al., 2019).
Conclusions
The studies presented demonstrate that ion channels are expressed from the very first stages of neural development. Furthermore, the signaling mechanisms triggered by these ion channels participate in all the relevant cellular processes of early development including neural cell proliferation and neuronal differentiation, mostly through imprinting specific spatiotemporal Ca2+ dynamics in developing neural cells. The participation of neural activity via ion channel expression throughout neural development poses the question of whether this makes the developing nervous system more vulnerable to “hijacking” of the necessary signaling mechanisms by exogenous unwanted factors. For instance, we have shown that incubating embryos with the AED valproic acid interferes with Ca2+ dynamics in neural plate cells and results in NTDs (Sequerra et al., 2018). The counterpart to this apparent vulnerability resides in the plasticity that having neural activity intertwined as a driving developmental mechanism confers upon the nervous system, allowing it to adapt to the changing environment. Indeed, we discovered that animals grown at cold temperatures which exhibit a higher number of motor neurons through a cold temperature-mediated, Ca2+ activity-dependent mechanism operating in embryonic spinal cord neurons, exhibit an enhanced escape locomotor performance compared to siblings grown at warm temperatures when tested at cold temperatures (Spencer et al., 2019). Expression of ion channels amplifies the robustness of the developing nervous system by enabling a functional proofreading of the system resulting from the instruction of other developmental cues, according to the surrounding environment.
Author Contributions
RG, KS and LB wrote the manuscript.
Funding
Research in the Borodinsky lab is supported by National Science Foundation (NSF) 1754340, National Institutes of Health (NIH)-NINDS R01NS105886 and R01NS113859 and Shriners Hospital for Children 86700-NCA, 85220-NCA and 85300-NCA grants to LB.
Conflict of Interest
The authors declare that the research was conducted in the absence of any commercial or financial relationships that could be construed as a potential conflict of interest.
References
Abdul-Wajid, S., Morales-Diaz, H., Khairallah, S. M., and Smith, W. C. (2015). T-type calcium channel regulation of neural tube closure and EphrinA/EPHA expression. Cell Rep. 13, 829–839. doi: 10.1016/j.celrep.2015.09.035
Andäng, M., Hjerling-Leffler, J., Moliner, A., Lundgren, T. K., Castelo-Branco, G., Nanou, E., et al. (2008). Histone H2AX-dependent GABAA receptor regulation of stem cell proliferation. Nature 451, 460–464. doi: 10.1038/nature06488
Arber, S., Han, B., Mendelsohn, M., Smith, M., Jessell, T. M., and Sockanathan, S. (1999). Requirement for the homeobox gene Hb9 in the consolidation of motor neuron identity. Neuron 23, 659–674. doi: 10.1016/s0896-6273(01)80026-x
Barish, M. E. (1986). Differentiation of voltage-gated potassium current and modulation of excitability in cultured amphibian spinal neurones. J. Physiol. 375, 229–250. doi: 10.1113/jphysiol.1986.sp016114
Barker, J. L., Behar, T., Li, Y. X., Liu, Q. Y., Ma, W., Maric, D., et al. (1998). GABAergic cells and signals in CNS development. Perspect. Dev. Neurobiol. 5, 305–322.
Belgacem, Y. H., and Borodinsky, L. N. (2011). Sonic hedgehog signaling is decoded by calcium spike activity in the developing spinal cord. Proc. Natl. Acad. Sci. U S A 108, 4482–4487. doi: 10.1073/pnas.1018217108
Belgacem, Y. H., and Borodinsky, L. N. (2015). Inversion of Sonic hedgehog action on its canonical pathway by electrical activity. Proc. Natl. Acad. Sci. U S A 112, 4140–4145. doi: 10.1073/pnas.1419690112
Bier, E., and De Robertis, E. M. (2015). EMBRYO DEVELOPMENT. BMP gradients: a paradigm for morphogen-mediated developmental patterning. Science 348:aaa5838. doi: 10.1126/science.aaa5838
Borodinsky, L. N., Belgacem, Y. H., Swapna, I., Visina, O., Balashova, O. A., Sequerra, E. B., et al. (2015). Spatiotemporal integration of developmental cues in neural development. Dev. Neurobiol. 75, 349–359. doi: 10.1002/dneu.22254
Borodinsky, L. N., Root, C. M., Cronin, J. A., Sann, S. B., Gu, X., and Spitzer, N. C. (2004). Activity-dependent homeostatic specification of transmitter expression in embryonic neurons. Nature 429, 523–530. doi: 10.1038/nature02518
Bouhadfane, M., Tazerart, S., Moqrich, A., Vinay, L., and Brocard, F. (2013). Sodium-mediated plateau potentials in lumbar motoneurons of neonatal rats. J. Neurosci. 33, 15626–15641. doi: 10.1523/JNEUROSCI.1483-13.2013
Boukhaddaoui, H., Sieso, V., Scamps, F., and Valmier, J. (2001). An activity-dependent neurotrophin-3 autocrine loop regulates the phenotype of developing hippocampal pyramidal neurons before target contact. J. Neurosci. 21, 8789–8797. doi: 10.1523/JNEUROSCI.21-22-08789.2001
Brackenbury, W. J., Yuan, Y., O’Malley, H. A., Parent, J. M., and Isom, L. L. (2013). Abnormal neuronal patterning occurs during early postnatal brain development of Scn1b-null mice and precedes hyperexcitability. Proc. Natl. Acad. Sci. U S A 110, 1089–1094. doi: 10.1073/pnas.1208767110
Briner, W. (2001). The effect of GABA receptor ligands in experimental spina bifida occulta. BMC Pharmacol. 1:2. doi: 10.1186/1471-2210-1-2
Cancedda, L., Fiumelli, H., Chen, K., and Poo, M.-M. (2007). Excitatory GABA action is essential for morphological maturation of cortical neurons in vivo. J. Neurosci. 27, 5224–5235. doi: 10.1523/JNEUROSCI.5169-06.2007
Choi, D.-S., Kellermann, O., Richard, S., Colas, J.-F., Bolanos-Jimenez, F., Tournois, C., et al. (1998). Mouse 5-HT2B receptor-mediated serotonin trophic functionsa. Ann. N Y Acad. Sci. 861, 67–73. doi: 10.1111/j.1749-6632.1998.tb10174.x
Chong, J. A., Tapia-Ramírez, J., Kim, S., Toledo-Aral, J. J., Zheng, Y., Boutros, M. C., et al. (1995). REST: a mammalian silencer protein that restricts sodium channel gene expression to neurons. Cell 80, 949–957. doi: 10.1016/0092-8674(95)90298-8
Christodoulou, N., and Skourides, P. A. (2015). Cell-autonomous Ca2+ flashes elicit pulsed contractions of an apical actin network to drive apical constriction during neural tube closure. Cell Rep. 13, 2189–2202. doi: 10.1016/j.celrep.2015.11.017
Cingolani, L. A., Gymnopoulos, M., Boccaccio, A., Stocker, M., and Pedarzani, P. (2002). Developmental regulation of small-conductance Ca2+-activated K+ channel expression and function in rat Purkinje neurons. J. Neurosci. 22, 4456–4467. doi: 10.1523/JNEUROSCI.22-11-04456.2002
Cline, H. T., and Constantine-Paton, M. (1989). NMDA receptor antagonists disrupt the retinotectal topographic map. Neuron 3, 413–426. doi: 10.1016/0896-6273(89)90201-8
Cohen, M., Briscoe, J., and Blassberg, R. (2013). Morphogen interpretation: the transcriptional logic of neural tube patterning. Curr. Opin. Genet. Dev. 23, 423–428. doi: 10.1016/j.gde.2013.04.003
Dumitru, I., Neitz, A., Alfonso, J., and Monyer, H. (2017). Diazepam binding inhibitor promotes stem cell expansion controlling environment-dependent neurogenesis. Neuron 94, 125.e5–137.e5. doi: 10.1016/j.neuron.2017.03.003
Fiorio Pla, A., Maric, D., Brazer, S.-C., Giacobini, P., Liu, X., Chang, Y. H., et al. (2005). Canonical transient receptor potential 1 plays a role in basic fibroblast growth factor (bFGF)/FGF receptor-1-induced Ca2+ entry and embryonic rat neural stem cell proliferation. J. Neurosci. 25, 2687–2701. doi: 10.1523/JNEUROSCI.0951-04.2005
Fiszman, M. L., Borodinsky, L. N., and Neale, J. H. (1999). GABA induces proliferation of immature cerebellar granule cells grown in vitro. Dev. Brain Res. 115, 1–8. doi: 10.1016/s0165-3806(99)00035-8
García-Alcocer, G., Martínez-Torres, A., and Miledi, R. (2005). Strychnine induces embryotoxicity in rat neurulation. Neurotoxicol. Teratol. 27, 855–859. doi: 10.1016/j.ntt.2005.06.020
Ghiani, C. A., Yuan, X., Eisen, A. M., Knutson, P. L., DePinho, R. A., McBain, C. J., et al. (1999). Voltage-activated K+ channels and membrane depolarization regulate accumulation of the cyclin-dependent kinase inhibitors p27Kip1 and p21CIP1 in glial progenitor cells. J. Neurosci. 19, 5380–5392. doi: 10.1523/JNEUROSCI.19-13-05380.1999
Gomez, T. M., and Spitzer, N. C. (1999). In vivo regulation of axon extension and pathfinding by growth-cone calcium transients. Nature 397, 350–355. doi: 10.1038/16927
Gomez-Ospina, N., Tsuruta, F., Barreto-Chang, O., Hu, L., and Dolmetsch, R. (2006). The C terminus of the L-type voltage-gated calcium channel CaV1.2 encodes a transcription factor. Cell 127, 591–606. doi: 10.1016/j.cell.2006.10.017
Gu, X., Olson, E. C., and Spitzer, N. C. (1994). Spontaneous neuronal calcium spikes and waves during early differentiation. J. Neurosci. 14, 6325–6335. doi: 10.1523/JNEUROSCI.14-11-06325.1994
Gu, X., and Spitzer, N. C. (1995). Distinct aspects of neuronal differentiation encoded by frequency of spontaneous Ca2+ transients. Nature 375, 784–787. doi: 10.1038/375784a0
Gurantz, D., Ribera, A. B., and Spitzer, N. C. (1996). Temporal regulation of Shaker- and Shab-like potassium channel gene expression in single embryonic spinal neurons during K+ current development. J. Neurosci. 16, 3287–3295. doi: 10.1523/JNEUROSCI.16-10-03287.1996
Harris, G. (1988). Changes in densities and kinetics of delayed rectifier potassium channels during neuronal differentiation. Neuron 1, 739–750. doi: 10.1016/0896-6273(88)90172-9
Holliday, J., and Spitzer, N. C. (1990). Spontaneous calcium influx and its roles in differentiation of spinal neurons in culture. Dev. Biol. 141, 13–23. doi: 10.1016/0012-1606(90)90098-4
Joo, J.-Y., Kim, B.-W., Lee, J.-S., Park, J.-Y., Kim, S., Yun, Y.-J., et al. (2007). Activation of NMDA receptors increases proliferation and differentiation of hippocampal neural progenitor cells. J. Cell Sci. 120, 1358–1370. doi: 10.1242/jcs.002154
Keiger, C. J., Prevette, D., Conroy, W. G., and Oppenheim, R. W. (2003). Developmental expression of nicotinic receptors in the chick and human spinal cord. J. Comp. Neurol. 455, 86–99. doi: 10.1002/cne.10468
Koser, D. E., Thompson, A. J., Foster, S. K., Dwivedy, A., Pillai, E. K., Sheridan, G. K., et al. (2016). Mechanosensing is critical for axon growth in the developing brain. Nat. Neurosci. 19, 1592–1598. doi: 10.1038/nn.4394
Kraner, S. D., Chong, J. A., Tsay, H. J., and Mandel, G. (1992). Silencing the type II sodium channel gene: a model for neural-specific gene regulation. Neuron 9, 37–44. doi: 10.1016/0896-6273(92)90218-3
Krey, J. F., Paşca, S. P., Shcheglovitov, A., Yazawa, M., Schwemberger, R., Rasmusson, R., et al. (2013). Timothy syndrome is associated with activity-dependent dendritic retraction in rodent and human neurons. Nat. Neurosci. 16, 201–209. doi: 10.1038/nn.3307
Lane, A. A., and Chabner, B. A. (2009). Histone deacetylase inhibitors in cancer therapy. J. Clin. Oncol. 27, 5459–5468. doi: 10.1200/JCO.2009.22.1291
Li, L., Hutchins, B. I., and Kalil, K. (2009). Wnt5a induces simultaneous cortical axon outgrowth and repulsive axon guidance through distinct signaling mechanisms. J. Neurosci. 29, 5873–5883. doi: 10.1523/JNEUROSCI.0183-09.2009
Li, Y., Jia, Y.-C., Cui, K., Li, N., Zheng, Z.-Y., Wang, Y., et al. (2005). Essential role of TRPC channels in the guidance of nerve growth cones by brain-derived neurotrophic factor. Nature 434, 894–898. doi: 10.1038/nature03477
Lindhout, D., Omtzigt, J. G., and Cornel, M. C. (1992). Spectrum of neural-tube defects in 34 infants prenatally exposed to antiepileptic drugs. Neurology 42, 111–118.
Lindhout, D., and Schmidt, D. (1986). In utero exposure to valproate and neural tube defects. Lancet 327, 1392–1393. doi: 10.1016/s0140-6736(86)91711-3
LoTurco, J. J., Owens, D. F., Heath, M. J., Davis, M. B., and Kriegstein, A. R. (1995). GABA and glutamate depolarize cortical progenitor cells and inhibit DNA synthesis. Neuron 15, 1287–1298. doi: 10.1016/0896-6273(95)90008-x
Luk, K. C., Kennedy, T. E., and Sadikot, A. F. (2003). Glutamate promotes proliferation of striatal neuronal progenitors by an NMDA receptor-mediated mechanism. J. Neurosci. 23, 2239–2250. doi: 10.1523/JNEUROSCI.23-06-02239.2003
Malmersjö, S., Rebellato, P., Smedler, E., Planert, H., Kanatani, S., Liste, I., et al. (2013). Neural progenitors organize in small-world networks to promote cell proliferation. Proc. Natl. Acad. Sci. U S A 110, E1524–E1532. doi: 10.1073/pnas.1220179110
Marek, K. W., Kurtz, L. M., and Spitzer, N. C. (2010). cJun integrates calcium activity and tlx3 expression to regulate neurotransmitter specification. Nat. Neurosci. 13, 944–950. doi: 10.1038/nn.2582
Maric, D., Liu, Q. Y., Maric, I., Chaudry, S., Chang, Y. H., Smith, S. V., et al. (2001). GABA expression dominates neuronal lineage progression in the embryonic rat neocortex and facilitates neurite outgrowth via GABAA autoreceptor/Cl− channels. J. Neurosci. 21, 2343–2360. doi: 10.1523/JNEUROSCI.21-07-02343.2001
Maue, R. A., Kraner, S. D., Goodman, R. H., and Mandel, G. (1990). Neuron-specific expression of the rat brain type II sodium channel gene is directed by upstream regulatory elements. Neuron 4, 223–231. doi: 10.1016/0896-6273(90)90097-y
McCobb, D. P., Best, P. M., and Beam, K. G. (1989). Development alters the expression of calcium currents in chick limb motoneurons. Neuron 2, 1633–1643. doi: 10.1016/0896-6273(89)90052-4
McCobb, D. P., Best, P. M., and Beam, K. G. (1990). The differentiation of excitability in embryonic chick limb motoneurons. J. Neurosci. 10, 2974–2984. doi: 10.1523/JNEUROSCI.10-09-02974.1990
Messenger, N. J., Rowe, S. J., and Warner, A. E. (1999). The neurotransmitter noradrenaline drivesnoggin-expressing ectoderm cells to activaten-tubulinand become neurons. Dev. Biol. 205, 224–232. doi: 10.1006/dbio.1998.9125
Nguyen, L., Malgrange, B., Breuskin, I., Bettendorff, L., Moonen, G., Belachew, S., et al. (2003). Autocrine/paracrine activation of the GABAA receptor inhibits the proliferation of neurogenic polysialylated neural cell adhesion molecule-positive (PSA-NCAM+) precursor cells from postnatal striatum. J. Neurosci. 23, 3278–3294. doi: 10.1523/JNEUROSCI.23-08-03278.2003
Nikolopoulou, E., Galea, G. L., Rolo, A., Greene, N. D. E., and Copp, A. J. (2017). Neural tube closure: cellular, molecular and biomechanical mechanisms. Development 144, 552–566. doi: 10.1242/dev.145904
O’Dowd, D. K., Ribera, A. B., and Spitzer, N. C. (1988). Development of voltage-dependent calcium, sodium, and potassium currents in Xenopus spinal neurons. J. Neurosci. 8, 792–805. doi: 10.1523/JNEUROSCI.08-03-00792.1988
Padmanabhan, R., and Ahmed, I. (1996). Sodium valproate augments spontaneous neural tube defects and axial skeletal malformations into mouse fetuses. Reprod. Toxicol. 10, 345–363. doi: 10.1016/0890-6238(96)00081-0
Pai, V. P., Lemire, J. M., Paré, J. F., Lin, G., Chen, Y., and Levin, M. (2015). Endogenous gradients of resting potential instructively pattern embryonic neural tissue via Notch signaling and regulation of proliferation. J. Neurosci. 35, 4366–4385. doi: 10.1523/JNEUROSCI.1877-14.2015
Panagiotakos, G., Haveles, C., Arjun, A., Petrova, R., Rana, A., Portmann, T., et al. (2019). Aberrant calcium channel splicing drives defects in cortical differentiation in Timothy syndrome. Elife 8:e51037. doi: 10.7554/eLife.51037
Patil, N., Cox, D. R., Bhat, D., Faham, M., Myers, R. M., and Peterson, A. S. (1995). A potassium channel mutation in weaver mice implicates membrane excitability in granule cell differentiation. Nat. Genet. 11, 126–129. doi: 10.1038/ng1095-126
Peng, I. F., Berke, B. A., Zhu, Y., Lee, W. H., Chen, W., and Wu, C. F. (2007). Temperature-dependent developmental plasticity of Drosophila neurons: cell-autonomous roles of membrane excitability, Ca2+ influx, and cAMP signaling. J. Neurosci. 27, 12611–12622. doi: 10.1523/JNEUROSCI.2179-07.2007
Personius, K. E., Karnes, J. L., and Parker, S. D. (2008). NMDA receptor blockade maintains correlated motor neuron firing and delays synapse competition at developing neuromuscular junctions. J. Neurosci. 28, 8983–8992. doi: 10.1523/JNEUROSCI.5226-07.2008
Personius, K. E., Slusher, B. S., and Udin, S. B. (2016). Neuromuscular NMDA receptors modulate developmental synapse elimination. J. Neurosci. 36, 8783–8789. doi: 10.1523/JNEUROSCI.1181-16.2016
Petrik, D., Myoga, M. H., Grade, S., Gerkau, N. J., Pusch, M., Rose, C. R., et al. (2018). Epithelial sodium channel regulates adult neural stem cell proliferation in a flow-dependent manner. Cell Stem Cell 22, 865.e8–878.e8. doi: 10.1016/j.stem.2018.04.016
Piggott, B. J., Peters, C. J., He, Y., Huang, X., Younger, S., Jan, L. Y., et al. (2019). Paralytic, the Drosophila voltage-gated sodium channel, regulates proliferation of neural progenitors. Genes Dev. 33, 1739–1750. doi: 10.1101/gad.330597.119
Pineda, R. H., and Ribera, A. B. (2008). Dorsal-ventral gradient for neuronal plasticity in the embryonic spinal cord. J. Neurosci. 28, 3824–3834. doi: 10.1523/JNEUROSCI.0242-08.2008
Rajan, I., and Cline, H. T. (1998). Glutamate receptor activity is required for normal development of tectal cell dendrites in vivo. J. Neurosci. 18, 7836–7846. doi: 10.1523/JNEUROSCI.18-19-07836.1998
Ribera, A. B., and Spitzer, N. C. (1989). A critical period of transcription required for differentiation of the action potential of spinal neurons. Neuron 2, 1055–1062. doi: 10.1016/0896-6273(89)90229-8
Ribera, A. B., and Spitzer, N. C. (1992). Developmental regulation of potassium channels and the impact on neuronal differentiation. Ion Channels 3, 1–38. doi: 10.1007/978-1-4615-3328-3_1
Robert, E., and Guibaud, P. (1982). Maternal valproic acid and congenital neural tube defects. Lancet 320:937. doi: 10.1016/s0140-6736(82)90908-4
Robinson, K. R., and Stump, R. F. (1984). Self-generated electrical currents through Xenopus neurulae. J. Physiol. 352, 339–352. doi: 10.1113/jphysiol.1984.sp015295
Root, C. M., Velázquez-Ulloa, N. A., Monsalve, G. C., Minakova, E., and Spitzer, N. C. (2008). Embryonically expressed GABA and glutamate drive electrical activity regulating neurotransmitter specification. J. Neurosci. 28, 4777–4784. doi: 10.1523/JNEUROSCI.4873-07.2008
Rosa, F. W. (1991). Spina bifida in infants of women treated with carbamazepine during pregnancy. N. Engl. J. Med. 324, 674–677. doi: 10.1056/nejm199103073241006
Sequerra, E. B., Goyal, R., Castro, P. A., Levin, J. B., and Borodinsky, L. N. (2018). NMDA receptor signaling is important for neural tube formation and for preventing antiepileptic drug-induced neural tube defects. J. Neurosci. 38, 4762–4773. doi: 10.1523/JNEUROSCI.2634-17.2018
Session, A. M., Uno, Y., Kwon, T., Chapman, J. A., Toyoda, A., Takahashi, S., et al. (2016). Genome evolution in the allotetraploid frog Xenopus laevis. Nature 538, 336–343. doi: 10.1038/nature19840
Sheldahl, L. C., Park, M., Malbon, C. C., and Moon, R. T. (1999). Protein kinase C is differentially stimulated by Wnt and Frizzled homologs in aG-protein-dependent manner. Curr. Biol. 9, 695–698. doi: 10.1016/s0960-9822(99)80310-8
Shen, H., Bocksteins, E., Kondrychyn, I., Snyders, D., and Korzh, V. (2016). Functional antagonism of voltage-gated K+ channel α-subunits in the developing brain ventricular system. Development 143, 4249–4260. doi: 10.1242/dev.140467
Sheng, L., Leshchyns’ka, I., and Sytnyk, V. (2015). Neural cell adhesion molecule 2 promotes the formation of filopodia and neurite branching by inducing submembrane increases in Ca2+ levels. J. Neurosci. 35, 1739–1752. doi: 10.1523/JNEUROSCI.1714-14.2015
Shibasaki, K., Murayama, N., Ono, K., Ishizaki, Y., and Tominaga, M. (2010). TRPV2 enhances axon outgrowth through its activation by membrane stretch in developing sensory and motor neurons. J. Neurosci. 30, 4601–4612. doi: 10.1523/JNEUROSCI.5830-09.2010
Shim, S., Goh, E. L., Ge, S., Sailor, K., Yuan, J. P., Roderick, H. L., et al. (2005). XTRPC1-dependent chemotropic guidance of neuronal growth cones. Nat. Neurosci. 8, 730–735. doi: 10.1038/nn1459
Slusarski, D. C., Corces, V. G., and Moon, R. T. (1997). Interaction of Wnt and a Frizzled homologue triggers G-protein-linked phosphatidylinositol signalling. Nature 390, 410–413. doi: 10.1038/37138
Smedley, M. J., and Stanisstreet, M. (1986). Calcium and neurulation in mammalian embryos. J. Embryol. Exp. Morphol. 93, 167–178.
Smith, R. S., and Walsh, C. A. (2020). Ion channel functions in early brain development. Trends Neurosci. 43, 103–114. doi: 10.1016/j.tins.2019.12.004
Somasundaram, A., Shum, A. K., McBride, H. J., Kessler, J. A., Feske, S., Miller, R. J., et al. (2014). Store-operated CRAC channels regulate gene expression and proliferation in neural progenitor cells. J. Neurosci. 34, 9107–9123. doi: 10.1523/JNEUROSCI.0263-14.2014
Song, J., Zhong, C., Bonaguidi, M. A., Sun, G. J., Hsu, D., Gu, Y., et al. (2012). Neuronal circuitry mechanism regulating adult quiescent neural stem-cell fate decision. Nature 489, 150–154. doi: 10.1038/nature11306
Spencer, K. A., Belgacem, Y. H., Visina, O., Shim, S., Genus, H., and Borodinsky, L. N. (2019). Growth at cold temperature increases the number of motor neurons to optimize locomotor function. Curr. Biol. 29, 1787.e5–1799.e5. doi: 10.1016/j.cub.2019.04.072
Spitzer, N. C. (2006). Electrical activity in early neuronal development. Nature 444, 707–712. doi: 10.1038/nature05300
Spitzer, N. C., and Lamborghini, J. E. (1976). The development of the action potential mechanism of amphibian neurons isolated in culture. Proc. Natl. Acad. Sci. U S A 73, 1641–1645. doi: 10.1073/pnas.73.5.1641
Spitzer, N. C., and Ribera, A. B. (1998). Development of electrical excitability in embryonic neurons: mechanisms and roles. J. Neurobiol. 37, 190–197. doi: 10.1002/(sici)1097-4695(199810)37:1<190::aid-neu14>3.0.co;2-i
Swapna, I., and Borodinsky, L. N. (2012). Interplay between electrical activity and bone morphogenetic protein signaling regulates spinal neuron differentiation. Proc. Natl. Acad. Sci. U S A 109, 16336–16341. doi: 10.1073/pnas.1202818109
Thaler, J., Harrison, K., Sharma, K., Lettieri, K., Kehrl, J., and Pfaff, S. L. (1999). Active suppression of interneuron programs within developing motor neurons revealed by analysis of homeodomain factor HB9. Neuron 23, 675–687. doi: 10.1016/s0896-6273(01)80027-1
Traister, A., Abashidze, S., Gold, V., Yairi, R., Michael, E., Plachta, N., et al. (2004). BMP controls nitric oxide-mediated regulation of cell numbers in the developing neural tube. Cell Death Differ. 11, 832–841. doi: 10.1038/sj.cdd.4401404
Van Horn, M. R., Strasser, A., Miraucourt, L. S., Pollegioni, L., and Ruthazer, E. S. (2017). The gliotransmitter d-serine promotes synapse maturation and axonal stabilization in vivo. J. Neurosci. 37, 6277–6288. doi: 10.1523/JNEUROSCI.3158-16.2017
Wallingford, J. B., Niswander, L. A., Shaw, G. M., and Finnell, R. H. (2013). The continuing challenge of understanding, preventing and treating neural tube defects. Science 339:1222002. doi: 10.1126/science.1222002
Wang, G. X., and Poo, M. M. (2005). Requirement of TRPC channels in netrin-1-induced chemotropic turning of nerve growth cones. Nature 434, 898–904. doi: 10.1038/nature03478
Yao, H., Duan, M., Yang, L., and Buch, S. (2012). Platelet-derived growth factor-BB restores human immunodeficiency virus tat-cocaine-mediated impairment of neurogenesis: role of TRPC1 channels. J. Neurosci. 32, 9835–9847. doi: 10.1523/JNEUROSCI.0638-12.2012
Keywords: neural tube formation, neural cell proliferation, neuronal differentiation, glutamate signaling, NMDA receptor, TRPM8, motor neuron differentiation, spinal cord development
Citation: Goyal R, Spencer KA and Borodinsky LN (2020) From Neural Tube Formation Through the Differentiation of Spinal Cord Neurons: Ion Channels in Action During Neural Development. Front. Mol. Neurosci. 13:62. doi: 10.3389/fnmol.2020.00062
Received: 17 February 2020; Accepted: 01 April 2020;
Published: 24 April 2020.
Edited by:
Michael Levin, Tufts University, United StatesReviewed by:
Nicholas C. Spitzer, University of California, San Diego, United StatesLoren Runnels, Rutgers Biomedical and Health Sciences, United States
Copyright © 2020 Goyal, Spencer and Borodinsky. This is an open-access article distributed under the terms of the Creative Commons Attribution License (CC BY). The use, distribution or reproduction in other forums is permitted, provided the original author(s) and the copyright owner(s) are credited and that the original publication in this journal is cited, in accordance with accepted academic practice. No use, distribution or reproduction is permitted which does not comply with these terms.
*Correspondence: Laura N. Borodinsky, bG5ib3JvZGluc2t5QHVjZGF2aXMuZWR1