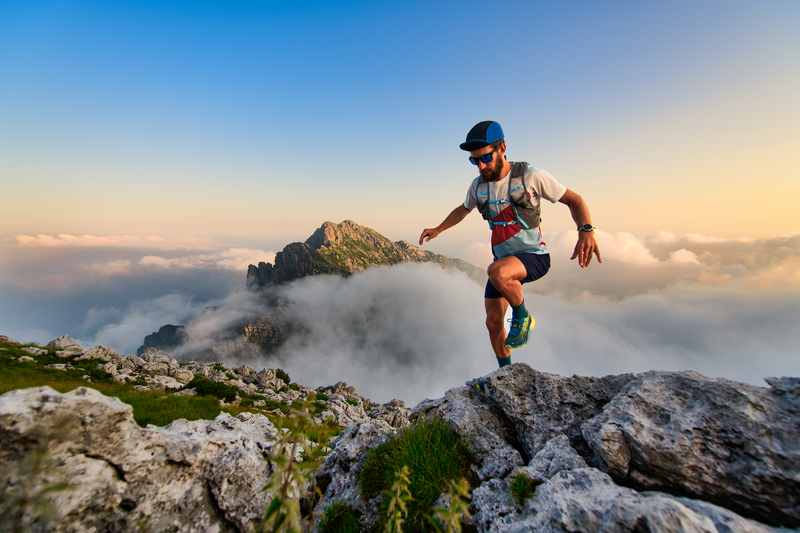
94% of researchers rate our articles as excellent or good
Learn more about the work of our research integrity team to safeguard the quality of each article we publish.
Find out more
REVIEW article
Front. Mol. Neurosci. , 19 December 2019
Sec. Molecular Signalling and Pathways
Volume 12 - 2019 | https://doi.org/10.3389/fnmol.2019.00313
This article is part of the Research Topic Emerging Mechanisms in Dynamic GABAergic Inhibition View all 16 articles
The dynamic modulation of receptor diffusion-trapping at inhibitory synapses is crucial to synaptic transmission, stability, and plasticity. In this review article, we will outline the progression of understanding of receptor diffusion dynamics at the plasma membrane. We will discuss how regulation of reversible trapping of receptor-scaffold interactions in combination with theoretical modeling approaches can be used to quantify these chemical interactions at the postsynapse of living cells.
Synaptic organization is a dynamic multiscale process in neuronal cell networks. The role of receptor diffusion-trapping in the plasma membrane is now understood to be a molecular mechanism resulting from chemical interactions and is crucial for synapse formation, stability, and plasticity in neurons.
The fluid mosaic model postulated by Singer and Nicolson (1972) reflects the thermodynamics and interactions of the individual components which have a critical role in the composition and organization of biological membranes. This idea was further enforced when, in 1974, the receptor protein rhodopsin was shown to be in constant motion in the lipid bilayer (Poo and Cone, 1974). Beside molecular turnover, it became obvious that synaptic molecular components were dynamic, enabling activity-dependent regulation of synaptic functions. The importance of molecular turnover at the synapse, as a regulator of synaptic strength and memory, was suggested by Crick (1984). Crick noted that the individual molecular components of the synapse were subject to characteristic times of turnover shorter than that of memory. Crick postulated that post-translational modifications of the molecules at the synapse could explain a longer-term persistent state of synaptic strength contributing to memory, despite a molecular turnover within days. Since then, there has been a concentrated effort into uncoupling membrane composition, diffusion dynamics and activity-dependent synaptic regulation with long-term structural stability.
This review will focus on the recent advances in our understanding of molecule dynamics in inhibitory synapses, covering technological advancements that have enabled probing of receptor and scaffold protein dynamics, organization and regulation.
Underlying fundamental processes controlling synaptic receptor delivery and removal, and the implications of these in synaptic strength have been of intense interest over the last 20 years. It was previously known that regulation of receptor number at the post synapse influenced plasticity at both excitatory and inhibitory synapses (e.g., Nusser et al., 1998; Hayashi et al., 2000; reviewed in Turrigiano, 2000). It was originally postulated that the dynamic turnover was driven exclusively by endocytosis and exocytosis of receptors and scaffold molecules to the membrane following de novo receptor synthesis or recycling. GABAA receptor (GABAAR) exocytosis and endocytosis via a clathrin-mediated pathway demonstrated exchange between the surface and intracellular compartments of the synapse (Kittler et al., 2000). Further, it was shown glycine receptor (GlyR) exocytosis occurred predominantly at extrasynaptic sites in the cell body and initial portion of dendrites in spinal cord neurons, and that this exocytosis was not directed or synapse-specific (Rosenberg et al., 2001). GABAAR exocytosis was also shown to be extrasynaptic followed by recruitment to synapses via lateral diffusion in the membrane in hippocampal neurons (Thomas et al., 2005; Bogdanov et al., 2006). Studies of excitatory synapses have showed AMPAR GluR1 subunits are initially inserted at extrasynaptic sites, whereas the GluR2 subunit is inserted in spines closer to synapses (Passafaro et al., 2001) and thus subunit specificity may further regulate receptor delivery. Further, in hippocampal pyramidal neurons, AMPARs were shown to enter spines preferentially following membrane insertion in the adjoining dendritic shaft (Yudowski et al., 2007). The balance of exocytosis and endocytosis regulates the number of postsynaptic receptors and has long been regarded as the main cellular mechanism underlying long-term potentiation (LTP) and long-term depression (LTD) (Mammen et al., 1997; Nishimune et al., 1998; Lüthi et al., 1999; Song and Huganir, 2002; Park et al., 2004; Tanaka and Hirano, 2012; Fujii et al., 2018).
However, in addition to receptor exocytosis and endocytosis, lateral receptor diffusion and trapping within the postsynaptic membrane has since been established as a key mediator of synaptic strength and plasticity. In 2001, Meier et al. (2001) demonstrated the lateral diffusion of the GlyR at the cell surface via the use of 500 nm latex beads. Additionally, they confirmed GlyR diffusion alternated between diffusive and confined states, with confinement spatially associated with the scaffold protein gephyrin. This led them to propose a dynamic equilibrium between pools of stabilized and freely mobile receptors (Figure 1). This lateral diffusion was then directly demonstrated via the tracking of quantum dots (QDs) bound to surface GlyRs (Dahan et al., 2003). This lateral movement from extrasynaptic pools and switching from free to confined Brownian motion has since been generalized for most neurotransmitter receptors (Thomas et al., 2005; Bogdanov et al., 2006; Pooler and McIlhinney, 2007; Lévi et al., 2008; Bannai et al., 2009; Choquet, 2010; Renner et al., 2017). Differences in diffusion of receptors at extrasynaptic and synaptic sites vary up to 10-fold, as shown for the GABAAR (Bannai et al., 2009; de Luca et al., 2017; Hannan et al., 2019) and the GlyR (Dahan et al., 2003; Lévi et al., 2008; Calamai et al., 2009). The characteristic time for receptor exchange by lateral receptor movement is much faster than that related to receptor recycling from internal stores or de novo receptor synthesis (Renner et al., 2008).
Figure 1. Inhibitory receptor diffusion-trapping. (1) Overview schematic of pre- and postsynaptic inhibitory neuronal membranes, exocytosis, and lateral diffusion. (2) The dynamic equilibrium between stabilized and freely mobile receptors, at the synapse and extrasynaptically, respectively. (3) Receptor diffusion-trapping depends not only on chemical interactions with synaptic components but also on non-specific obstacles, such as lipid rafts, leading to molecular crowding. (4) Activity regulation of receptor mobility can affect post-translational modifications of receptors and scaffold proteins and subsequently their immobilization at synapses. Exchange of receptors between synapses can fine-tune network activity.
At the postsynaptic membrane, there are multiple aspects that may influence receptor lateral diffusion. The transient trapping at synapses of laterally diffusing molecules can result from interactions of receptors with other proteins at the membrane such as scaffold molecules, acting as diffusion traps, or from non-specific obstacles, such as molecular crowding, lipid composition and the sub-membrane cytoskeleton (Figure 1).
Interaction of receptors with scaffold molecules represents one of the primary effectors of synaptic diffusion. At the inhibitory synapse, gephyrin interactions have been analyzed for their influence on GABAAR (e.g., Jacob et al., 2005; Petrini et al., 2014) and GlyR (e.g., Meier et al., 2001; Meier and Grantyn, 2004) mobility. GlyRs and GABAARs diffuse far more freely at extrasynaptic sites than when confined in inhibitory synapses at gephyrin clusters. Specifically, gephyrin interaction with receptors at synapses causes transient receptor retention (Meier et al., 2001; Dahan et al., 2003; Calamai et al., 2009; Specht et al., 2011). Furthermore, the binding of the GABAAR to gephyrin and subsequent increased dwell time of GABAAR at gephyrin-positive synaptic sites affected the synaptic strength of inhibition (Mukherjee et al., 2011). A comparable decrease in diffusion of metabotropic- and AMPA-type glutamate receptors upon binding to their respective scaffold molecules has also been observed (Borgdorff and Choquet, 2002; Sergé et al., 2002).
Competition between receptors, including their subunit composition, may further regulate lateral movement and accumulation into synapses. Lateral diffusion of GABAARs containing α5 or α2 subunits were reported to be modulated by GABABRs for binding to scaffold proteins (Gerrow and Triller, 2014). It was recently shown that GABAARs comprised of different subunit combinations have variable diffusion and synaptic retention rates (Hannan et al., 2019). Additional regulation of receptor diffusion may hence be inferred through subunit-specific regulations, leading to coordinated molecular and functional specificity. Likewise, different diffusion properties arise from contrasting affinities of GABAAR and GlyR subunits for gephyrin (Tretter et al., 2008; Maric et al., 2011; Kowalczyk et al., 2013). Finally, the multivalency of the gephyrin scaffold network is also likely to further regulate the molecular organization and diffusion of receptors at the membrane (Specht et al., 2013).
Physical barriers such as cholesterol, phospholipids, other receptors and the cytoskeleton can also regulate the diffusion-trap mechanism. The physical properties of the plasma membrane, including surface geometry, curvature and viscosity determine the flux of receptors (for review, see Marguet et al., 2006). Lipid raft domains can reduce lateral mobility of receptors (Allen et al., 2007), while cholesterol depletion affects apparent membrane viscosity and subsequently receptor diffusion properties (Renner et al., 2009). Thus controlling membrane lipid composition, including cholesterol, can have consequences on molecular flow in and out of the postsynapse. Furthermore, phospholipids within the membrane themselves can act as local messengers in neurotransmission (García-Morales et al., 2015). The tuning of GlyR lateral diffusion has been shown at synaptic and extrasynaptic sites upon F-actin and microtubule disruption respectively (Charrier et al., 2006). Increased lateral diffusion upon dissociation of GABAARs from their actin anchor, radixin, lead to increased synaptic expression (Loebrich et al., 2006; Hausrat et al., 2015). Additionally, gephyrin interacts with actin filaments via several proteins including profilin, Mena/Vasp (Mammoto et al., 1998; Giesemann et al., 2003). Thus the regulation of scaffold trafficking by the cytoskeleton can also affect receptor lateral diffusion and synapse accumulation. Furthermore, activity-dependent extracellular matrix (ECM) modifications may also have structural and functional consequences on receptor lateral mobility (Dityatev et al., 2010). In fact, the secreted ECM molecule Reelin has been shown to regulate the surface distribution and diffusion of NMDA receptors in hippocampal neurons (Groc et al., 2007). The ECM protein thrombospondin-1 increased the lateral diffusion and endocytosis of AMPARs and increased synaptic accumulation of GlyRs in rat spinal cord neurons (Hennekinne et al., 2013). These effects on GlyRs are also dependent on increased excitation as well as the presence of β-1 integrins. Gephyrin clustering itself has been shown to be tuned by integrin-mediated interactions leading to GlyR trapping at the synapse (Charrier et al., 2010). Consequently, the presence of other trans-membrane proteins, in particular those involved in ECM binding such as integrins, can affect neurotransmitter diffusion and synaptic trapping.
Hence surface availability is governed by a combination of processes, such as receptor exocytosis, lateral mobility, diffusion-trapping, dynamic interactions with membrane components, molecular crowding.
Several approaches have been utilized with the aim of quantifying molecular-scale dynamics in cells. Single-molecule fluorescence imaging via low-density antibody labeling of GluR2-containing AMPARs enabled visualization of receptor entry and exit at synapses (Tardin et al., 2003). Advances in super-resolution imaging techniques, such as single-particle tracking (SPT) using QDs or coupled with photoactivated localization microscopy (PALM), or universal point accumulation for imaging in nanoscale topography (uPAINT) have enabled further analysis within synapses. uPAINT relies on the binding of fluorescently-labeled ligands or dye-coupled antibodies to the molecule of interest (Sharonov and Hochstrasser, 2006; Giannone et al., 2010). SPT using QDs relies on QD-conjugated antibodies, whereas sptPALM relies on endogenous expression of fluorescent proteins tagged to the target molecule. SPT and uPAINT techniques produce thousands of trajectories generating dense diffusion information with high spatiotemporal resolution. Although it depends on the length of the trajectories, this enables differentiation of active, confined or random movements. These movements can be followed on the cell surface and the landscape of the diffusion dynamics mapped. The most common parameters calculated from these techniques include the diffusion coefficient (D) reflecting the area explored and the mean squared displacement (MSD), the function (f(t)) of which, describes the diffusion behavior over time i.e., Brownian, confined or directed (e.g., Kusumi et al., 1993; Saxton and Jacobson, 1997). QDs bound to receptors and their diffusion provided the first direct demonstration that receptors enter and exit the postsynapse via lateral diffusion (Dahan et al., 2003). Although they blink, QD fluorescence is more stable than that of conventional fluorophores with an average size of ~10–15 nm (or bigger if one includes the binding components). Multiple exchanges of GlyRs between extrasynaptic and synaptic domains were observed, with free and confined states respectively. GlyRs were also tracked from one synaptic site to another 4–5 μm away demonstrating synaptic exchange by lateral diffusion of receptors. The D was ~0.1 μm2/s outside of the synapses, matching that expected for free Brownian diffusion in a lipid bilayer. The D then decreased to ~0.02 μm2/s (or lower) as it entered the synapse, corresponding to confined movement. QD tracking allows for relatively long acquisition times, yielding long trajectories where changes in diffusion properties can be mapped, however labeling density is low. In comparison, sptPALM, due to the fast bleaching of the fluorophores used, produces much shorter trajectories, but in far larger numbers. Thus, multiple-target tracing (MTT) has been employed to reconnect the single-molecule trajectories and extract their molecular dynamics (Sergé et al., 2008).
Recently, methods to analyze the movement of single molecule trajectories have been advanced with the aim to describe more accurately kinetics of individual interactions in native cell environments. Measuring the D of a whole trajectory does not take into account transient stabilizations via interactions with other molecules at given locations. Instead, the localized effective binding energy is more ideally suited to analyzing such biochemical interactions (Masson et al., 2014). Therefore, Masson et al. (2014) suggested an approach using Bayesian inference and overdamped Langevin equations to analyze the molecular motion. This generates an energy landscape which takes into account the heterogeneous diffusivity in the cell membrane. The depth of the energy trap is modulated by biochemical interactions between the receptor and scaffold proteins (Masson et al., 2014). This spatial cartography demonstrated that the presence of gephyrin clusters coincided with energy minima and hence was consistent with transient stabilization of receptors at synapses (El Beheiry et al., 2015). Consequently, the neuronal membrane has to be considered as a statistical field with constantly moving and transiently trapped molecules rather than formed by the juxtaposition of domains with fixed compositions. Using computer simulations and mathematical modeling, insight into molecular dynamics and the relationship between transient receptor trapping and local chemical reactions has been estimated. Moreover, using a Markovian approach, synaptic weight could be expressed as fluctuations in the number of bound receptors in the postsynapse (Holcman and Triller, 2006). Langevin equation models have enabled an analysis of molecular interactions of AMPARs (Hoze et al., 2012) and GlyRs (Masson et al., 2014; El Beheiry et al., 2015) in the postsynaptic membrane. Additionally, the geometrical effect of membrane curvature on the 2D projected stochastic trajectory of a molecule affects diffusion properties (Domanov et al., 2011; Renner et al., 2011). The introduction of another parameter different from MTT based tracking and reconnection, the packing coefficient (Pc), allows characterization of the movement of a given molecule along its trajectory, thus as a function of time, independently of its overall diffusivity (Renner et al., 2017). It can also be utilized to derivate the effective Kon and Koff of a receptor to its scaffold. A cooperative mesoscopic model of the reciprocal stabilization of synaptic receptors and scaffolding proteins allowed accounting for the synapse “stability” as a quasi-equilibrium (Sekimoto and Triller, 2009). Then, using an out-of-equilibrium model, it has been proposed that the size of the scaffold clusters can be explained by the aggregation of gephyrin proteins diffusing in the sub-membrane space whilst bound to the GlyR, balanced against membrane turnover “aggregation-removal model” (Ranft et al., 2017).
The combination of theoretical modeling with single-molecule experimental data can now provide a quantification of synapse receptor dynamics in relation to the chemical modulation of these dynamics in the live cell environment, thus the concept of “chemistry in-cellulo” (Salvatico et al., 2015). This mixed experimental and theoretical approach will enable comprehension of how the dynamic movement of receptors and their interactions with other proteins can lead to longer-term stabilizations as well as the chemical determinants of receptor number and synapse function.
Many studies in recent years have concentrated on molecular mechanisms of inhibitory neurotransmission and synaptic scaffold protein modification that influence the local interactions and diffusion events underlying synaptic plasticity. It is now established that receptor diffusion-trapping at synapses can be affected by neuronal activity (Figure 1). Increased mobility of QD-labeled GlyRs and GABAARs has been seen upon increased excitatory neuronal activity (Lévi et al., 2008; Bannai et al., 2009; Muir et al., 2010). Application of TTX to spinal cord neurons demonstrated reduced lateral diffusion of GlyR with an increase in receptor cluster number, but not for GABAARs (Lévi et al., 2008). However the same effect was not seen for GABAARs in hippocampal cells, instead, TTX application reduced GABAAR lateral diffusion by means of an NMDA-calcineurin-dependent mechanism (Bannai et al., 2009), suggesting cell type and receptor type plays an additional regulatory role. In fact the involvement of NMDARs in the exocytosis of GABAARs is CaMKII-dependent, and consequently potentiates inhibitory transmission (iLTP) (Marsden et al., 2007). It should be noted NMDAR-induced iLTP leads to a moderate intracellular calcium recruitment and activation of CaMKII (Lucchesi et al., 2011; Petrini et al., 2014). Conversely, iLTD via NMDARs and voltage-gated calcium channels leads to a massive increase in intracellular calcium and subsequent recruitment of calcineurin to inhibitory synapses (Bannai et al., 2009; Muir et al., 2010). These converging pathways constitute a fine-tuning of activity-dependent GABAAR diffusion dynamics and thus inhibition. More precisely, calcineurin-induced phosphorylation of GABAARs following NMDA activation confirmed the GABAAR dispersal with important implications for activity-dependent control of synaptic inhibition (Muir et al., 2010). Conversely, GABAAR cluster promotion at the postsynapse and enhanced GABAergic signaling via a metabotropic glutamate receptor-induced IP3 and PKC signaling pathway show spatiotemporal signaling patterns of calcium can fine-tune GABAAR availability (Bannai et al., 2015). As shown in spinal cord neurons, PKC also phosphorylates the GlyR β-subunit at residue S403 (Specht et al., 2011). Consequently cross talk and competition between GABAARs and GlyRs, at mixed GABA-Gly synapses in the spinal cord, adds an additional layer of complexity to the regulation of synaptic inhibition. Combining experimental work and theory it has been hypothesized that the long-term stability of synaptic cluster size obeys a dynamic equilibrium between the attraction of scaffold molecules to each other and the repulsion of receptor-receptor interactions (Haselwandter et al., 2011). Other synaptic components also impact these interactions. Upon chemical iLTP, GABAARs are immobilized at synapses following active gephyrin recruitment in hippocampal neurons, the mechanism of which requires phosphorylation of GABAAR-β3 by CaMKIIα (Petrini et al., 2014). Whilst gephyrin plays a critical role in GABAAR membrane clustering, gephyrin-independent mechanisms of GABAAR stabilization also exist. Following sustained excitatory activity GABAAR mobility and clustering was shown to be independent of gephyrin clustering in hippocampal neurons (Niwa et al., 2012). More recently, QD-SPT combined with optogenetics to control calcium flow with high temporal precision showed inter-synaptic lateral diffusion of GABAARs in a desensitized state in hippocampal neurons (de Luca et al., 2017). Synapses were typically 2–4 μm apart, with intersynaptic diffusion occurring in ~15% trajectories at a D of 0.07 μm2S−1. Further, they showed that glutamatergic activity limits this inter-synaptic diffusion via trapping GABAARs at excitatory synapses. They suggested this might present a mechanism by which a memory of recent activation is transmitted to neighboring synapses. In addition to regulation of inhibitory synaptic receptors via direct neuronal activity, microglia have also been implicated in receptor dynamics. Prostaglandin E2 from microglia was recently shown to regulate GlyR diffusion dynamics and synaptic trapping but not GABAergic synapses (Cantaut-Belarif et al., 2017). Importantly, this demonstrated that microglia could regulate the plasticity of glycinergic synapses by tuning GlyR diffusion-trapping. Hence diffusion-trapping is not a cell-autonomous event. Additional fine-tuning of receptor diffusion dynamics may further occur in certain inflammatory states.
Recent work into the organization within synaptic clusters of receptor proteins and scaffold molecules have revealed the existence of subsynaptic domains in both excitatory (e.g., MacGillavry et al., 2013; Nair et al., 2013) and inhibitory (e.g., Specht et al., 2013; Crosby et al., 2019) synapses. In spinal cord neurons the stoichiometry of gephyrin to GlyR binding sites was estimated to be approximately 1:1 (Specht et al., 2013). Incorporating super-resolution microscopy and model simulations, gephyrin stabilization in nano-domains was visualized upon iLTP which in turn stabilized the number of GABAARs in mouse hippocampal neurons (Pennacchietti et al., 2017). In a separate study, QD-SPT of GABAAR diffusion in rat hippocampal neurons showed GSK-3β and ERK1/2 differentially altered the gephyrin scaffold mesh, which as a result affected GABAAR surface dynamics (Battaglia et al., 2018). They found that gephyrin microdomain compaction was regulated by phosphorylation in an activity-dependent way. Future work into this nano-organization and its control on intrasynaptic diffusion will allow understanding of long-term synaptic stability and GABAAR/GlyR competition at inhibitory synapses.
Diffusion trapping of receptors at synapses tunes receptor number, hence regulating neuronal activity with functional consequences on synaptic plasticity (Choquet and Triller, 2013; Petrini and Barberis, 2014). Plasticity associated changes in lateral mobility have been shown in inhibitory (e.g., Bannai et al., 2009; Petrini et al., 2014) and excitatory synapses (e.g., Ehlers et al., 2007; Makino and Malinow, 2009). In one such example, tracking surface GABAARs on cultured hippocampal neurons during chemical iLTP showed synaptic recruitment of gephyrin from extrasynaptic regions was promoted by CamKII-dependent phosphorylation of GABAAR-β3 at Ser838 (Petrini et al., 2014). Further, they showed that impairment of gephyrin assembly prevented chemical iLTP with an associated decrease in GABAAR immobilization at synapses. Concurrently, changes in the exocytosis of inhibitory receptors can also occur upon neuronal activation, but over slower time courses (Marsden et al., 2007). This activity-dependent plasticity is hence determined by diffusion of the molecular synaptic components and the underlying mechanisms that regulate receptor availability across multi-time scales.
The link between lateral diffusion of receptors and their confinement at synapses with behavior is not yet understood. However, one mechanism has recently been described, linking RhoA/ROCK activity-dependent phosphorylation of radixin which in turn uncouples GABAAR-α5 from extrasynaptic sites enabling their enrichment at synapses (Hausrat et al., 2015). This radixin phosphorylation was shown to occur in wild-type mice during short-term memory and reversal learning. In excitatory synapses, interfering with AMPAR surface diffusion impaired synaptic potentiation of Schaffer collaterals and commissural inputs to the CA1 of the mouse hippocampus in cultured slices and in vivo (Penn et al., 2017). Moreover, they showed AMPAR immobilization in the hippocampus in vivo inhibited fear conditioning. Thus, lateral diffusion of receptors and their temporal confinement at both excitatory and inhibitory synapses is likely to be a fundamental mechanism involved in learning and memory.
Affecting local and network-wide activity, diffusion dynamics may be implicated in certain neuropathologies. Benzodiazepines (BZDs) are widely used to treat many neurological and psychiatric diseases. It is now thought that in addition to their effects on receptor gating, membrane dynamics are also affected. SPT experiments of GABAARs in mouse hippocampal neurons upon addition of the GABAAR agonist muscimol showed accelerated GABAAR diffusion, which was subsequently abolished upon addition of the BZD agonist diazepam (Gouzer et al., 2014). Using SPT in hippocampal neurons, diazepam was shown to increase synaptic stabilization and clustering of GABAARs and decreased their lateral diffusion upon sustained neuronal activity but not at rest (Gouzer et al., 2014; Lévi et al., 2015). Acute estradiol treatment has also been demonstrated to decrease the confinement of GABAARs, reducing their dwell time in synaptic compartments and increasing the D at extrasynaptic sites (Mukherjee et al., 2017). These results have a direct impact on the design of therapeutic compounds for diseases arising from dysregulation of inhibition.
The plasma membrane is dynamic and trans-membrane molecules such as receptors diffuse laterally. These processes provide mechanisms for regulation of receptor number at synapses and thus function and plasticity. Recent results have isolated various pathways involved in receptor diffusion control, however, there are many important questions still to be answered. The contribution of receptor dynamics in synapse development, maturation, and refinement in both health and disease is yet to be fully explored. The interplay of GABAAR and GlyR competition within inhibitory synapses in different brain regions, alongside distance and distribution of inhibitory and excitatory receptors is likely to underpin activity-dependent modification of synapse strength.
Whilst there have been huge technological advancements over a relatively short period of time, there remains inherent limitations in the currently used techniques for analyzing lateral membrane diffusion. QDs are a popular choice due to their photostability, bright fluorescence, long trajectories and ability to multiplex (Cutler et al., 2013; Kakizuka et al., 2016; Renner et al., 2017). However, their large size complexed with antibodies can sterically hinder lateral mobility (Abraham et al., 2017) and low-density labeling strategies mean only a fraction of the molecules are probed. The use of sptPALM enables direct genetic tagging of target molecules with a fluorescent protein, either by lentiviral expression or knock-in animal models, allowing tracking of all target molecules and analysis of endogenous molecule copy number (Lee et al., 2012; Specht et al., 2013). However incorrect protein folding and targeted degradation of the fluorescent protein-target complex can occur (Tanudji et al., 2002; Stepanenko et al., 2013; Guo et al., 2014) and trajectories are shorter than those of QDs. uPAINT relies on binding of high affinity fluorescently tagged antibodies or ligands to the target (Giannone et al., 2010). The main drawback of this technique is the saturation of the target with bleached ligands. Future technical developments will include the manipulation of fluorescent proteins and organic probes to be smaller and brighter, improvements in microscope set-ups to track multiple proteins simultaneously, improved resolution in 3D imaging and tracking, and use of brain slices and in vivo set-ups will provide additional comprehension of diffusion dynamics within a biologically relevant microenvironment.
All authors listed have made a substantial, direct and intellectual contribution to the work, and approved it for publication.
SM is supported by a Fondation pour la Recherche Médicale (FRM) postdoctoral fellowship (SPF201809007132).
The authors declare that the research was conducted in the absence of any commercial or financial relationships that could be construed as a potential conflict of interest.
The handling Editor declared a past co-authorship with one of the authors AT.
Abraham, L., Lu, H. Y., Falcão, R. C., Scurll, J., Jou, T., Irwin, B., et al. (2017). Limitations of Qdot labelling compared to directly-conjugated probes for single particle tracking of B cell receptor mobility. Sci. Rep. 7:11379. doi: 10.1038/s41598-017-11563-9
Allen, J. A., Halverson-Tamboli, R. A., and Rasenick, M. M. (2007). Lipid raft microdomains and neurotransmitter signalling. Nat. Rev. Neurosci. 8, 128–140. doi: 10.1038/nrn2059
Bannai, H., Lévi, S., Schweizer, C., Inoue, T., Launey, T., Racine, V., et al. (2009). Activity-dependent tuning of inhibitory neurotransmission based on GABAAR diffusion dynamics. Neuron 62, 670–682. doi: 10.1016/j.neuron.2009.04.023
Bannai, H., Niwa, F., Sherwood, M. W., Shrivastava, A. N., Arizono, M., Miyamoto, A., et al. (2015). Bidirectional control of synaptic GABAAR clustering by glutamate and calcium. Cell Rep. 13, 2768–2780. doi: 10.1016/j.celrep.2015.12.002
Battaglia, S., Renner, M., Russeau, M., Côme, E., Tyagarajan, S. K., and Lévi, S. (2018). Activity-dependent inhibitory synapse scaling is determined by gephyrin phosphorylation and subsequent regulation of GABAA receptor diffusion. eNeuro 5:ENEURO.0203-17.2017. doi: 10.1523/eneuro.0203-17.2017
Bogdanov, Y., Michels, G., Armstrong-Gold, C., Haydon, P. G., Lindstrom, J., Pangalos, M., et al. (2006). Synaptic GABAA receptors are directly recruited from their extrasynaptic counterparts. EMBO J. 25, 4381–4389. doi: 10.1038/sj.emboj.7601309
Borgdorff, A. J., and Choquet, D. (2002). Regulation of AMPA receptor lateral movements. Nature 417, 649–653. doi: 10.1038/nature00780
Calamai, M., Specht, C. G., Heller, J., Alcor, D., Machado, P., Vannier, C., et al. (2009). Gephyrin oligomerization controls GlyR mobility and synaptic clustering. J. Neurosci. 29, 7639–7648. doi: 10.1523/JNEUROSCI.5711-08.2009
Cantaut-Belarif, Y., Antri, M., Pizzarelli, R., Colasse, S., Vaccari, I., Soares, S., et al. (2017). Microglia control the glycinergic but not the GABAergic synapses via prostaglandin E2 in the spinal cord. J. Cell Biol. 216, 2979–2989. doi: 10.1083/jcb.201607048
Charrier, C., Ehrensperger, M.-V., Dahan, M., Lévi, S., and Triller, A. (2006). Cytoskeleton regulation of glycine receptor number at synapses and diffusion in the plasma membrane. J. Neurosci. 26, 8502–8511. doi: 10.1523/JNEUROSCI.1758-06.2006
Charrier, C., Machado, P., Tweedie-Cullen, R. Y., Rutishauser, D., Mansuy, I. M., and Triller, A. (2010). A crosstalk between β1 and β3 integrins controls glycine receptor and gephyrin trafficking at synapses. Nat. Neurosci. 13, 1388–1395. doi: 10.1038/nn.2645
Choquet, D. (2010). Fast AMPAR trafficking for a high-frequency synaptic transmission. Eur. J. Neurosci. 32, 250–260. doi: 10.1111/j.1460-9568.2010.07350.x
Choquet, D., and Triller, A. (2013). The dynamic synapse. Neuron 80, 691–703. doi: 10.1016/j.neuron.2013.10.013
Crick, F. (1984). Neurobiology: memory and molecular turnover. Nature 312:101. doi: 10.1038/312101a0
Crosby, K. C., Gookin, S. E., Garcia, J. D., Hahm, K. M., Dell’Acqua, M. L., and Smith, K. R. (2019). Nanoscale subsynaptic domains underlie the organization of the inhibitory synapse. Cell Rep. 26, 3284.e3–3297.e3. doi: 10.1016/j.celrep.2019.02.070
Cutler, P. J., Malik, M. D., Liu, S., Byars, J. M., Lidke, D. S., and Lidke, K. A. (2013). Multi-color quantum dot tracking using a high-speed hyperspectral line-scanning microscope. PLoS One 8:e64320. doi: 10.1371/journal.pone.0064320
Dahan, M., Lévi, S., Luccardini, C., Rostaing, P., Riveau, B., and Triller, A. (2003). Diffusion dynamics of glycine receptors revealed by single-quantum dot tracking. Science 302, 442–445. doi: 10.1126/science.1088525
de Luca, E., Ravasenga, T., Petrini, E. M., Polenghi, A., Nieus, T., Guazzi, S., et al. (2017). Inter-synaptic lateral diffusion of GABAA receptors shapes inhibitory synaptic currents. Neuron 95, 63.e5–69.e5. doi: 10.1016/j.neuron.2017.06.022
Dityatev, A., Schachner, M., and Sonderegger, P. (2010). The dual role of the extracellular matrix in synaptic plasticity and homeostasis. Nat. Rev. Neurosci. 11, 735–746. doi: 10.1038/nrn2898
Domanov, Y. A., Aimon, S., Toombes, G. E. S., Renner, M., Quemeneur, F., Triller, A., et al. (2011). Mobility in geometrically confined membranes. Proc. Natl. Acad. Sci. U S A 108, 12605–12610. doi: 10.1073/pnas.1102646108
Ehlers, M. D., Heine, M., Groc, L., Lee, M.-C., and Choquet, D. (2007). Diffusional trapping of GluR1 AMPA receptors by input-specific synaptic activity. Neuron 54, 447–460. doi: 10.1016/j.neuron.2007.04.010
El Beheiry, M., Dahan, M., and Masson, J.-B. (2015). InferenceMAP: mapping of single-molecule dynamics with Bayesian inference. Nat. Methods 12, 594–595. doi: 10.1038/nmeth.3441
Fujii, S., Tanaka, H., and Hirano, T. (2018). Suppression of AMPA receptor exocytosis contributes to hippocampal LTD. J. Neurosci. 38, 5523–5537. doi: 10.1523/JNEUROSCI.3210-17.2018
García-Morales, V., Montero, F., González-Forero, D., Rodríguez-Bey, G., Gómez-Pérez, L., Medialdea-Wandossell, M. J., et al. (2015). Membrane-derived phospholipids control synaptic neurotransmission and plasticity. PLoS Biol. 13:e1002153. doi: 10.1371/journal.pbio.1002153
Gerrow, K., and Triller, A. (2014). GABAA receptor subunit composition and competition at synapses are tuned by GABAB receptor activity. Mol. Cell. Neurosci. 60, 97–107. doi: 10.1016/j.mcn.2014.04.001
Giannone, G., Hosy, E., Levet, F., Constals, A., Schulze, K., Sobolevsky, A. I., et al. (2010). Dynamic superresolution imaging of endogenous proteins on living cells at ultra-high density. Biophys. J. 99, 1303–1310. doi: 10.1016/j.bpj.2010.06.005
Giesemann, T., Schwarz, G., Nawrotzki, R., Berhörster, K., Rothkegel, M., Schlüter, K., et al. (2003). Complex formation between the postsynaptic scaffolding protein gephyrin, profilin, and Mena: a possible link to the microfilament system. J. Neurosci. 23, 8330–8339. doi: 10.1523/JNEUROSCI.23-23-08330.2003
Gouzer, G., Specht, C. G., Allain, L., Shinoe, T., and Triller, A. (2014). Benzodiazepine-dependent stabilization of GABAA receptors at synapses. Mol. Cell. Neurosci. 63, 101–113. doi: 10.1016/j.mcn.2014.10.004
Groc, L., Choquet, D., Stephenson, F. A., Verrier, D., Manzoni, O. J., and Chavis, P. (2007). NMDA receptor surface trafficking and synaptic subunit composition are developmentally regulated by the extracellular matrix protein reelin. J. Neurosci. 27, 10165–10175. doi: 10.1523/JNEUROSCI.1772-07.2007
Guo, M., Gelman, H., and Gruebele, M. (2014). Coupled protein diffusion and folding in the cell. PLoS One 9:e113040. doi: 10.1371/journal.pone.0113040
Hannan, S., Minere, M., Harris, J., Izquierdo, P., Thomas, P., Tench, B., et al. (2019). GABAAR isoform and subunit structural motifs determine synaptic and extrasynaptic receptor localisation. Neuropharmacology doi: 10.1016/j.neuropharm.2019.02.022 [Epub ahead of print].
Haselwandter, C. A., Calamai, M., Kardar, M., Triller, A., and da Silveira, R. A. (2011). Formation and stability of synaptic receptor domains. Phys. Rev. Lett. 106:238104. doi: 10.1103/physrevlett.106.238104
Hausrat, T. J., Muhia, M., Gerrow, K., Thomas, P., Hirdes, W., Tsukita, S., et al. (2015). Radixin regulates synaptic GABAA receptor density and is essential for reversal learning and short-term memory. Nat. Commun. 6:6872. doi: 10.1038/ncomms7872
Hayashi, Y., Shi, S. H., Esteban, J. A., Piccini, A., Poncer, J. C., and Malinow, R. (2000). Driving AMPA receptors into synapses by LTP and CaMKII: requirement for GluR1 and PDZ domain interaction. Science 287, 2262–2267. doi: 10.1126/science.287.5461.2262
Hennekinne, L., Colasse, S., Triller, A., and Renner, M. (2013). Differential control of thrombospondin over synaptic glycine and AMPA receptors in spinal cord neurons. J. Neurosci. 33, 11432–11439. doi: 10.1523/JNEUROSCI.5247-12.2013
Holcman, D., and Triller, A. (2006). Modeling synaptic dynamics driven by receptor lateral diffusion. Biophys. J. 91, 2405–2415. doi: 10.1529/biophysj.106.081935
Hoze, N., Nair, D., Hosy, E., Sieben, C., Manley, S., Herrmann, A., et al. (2012). Heterogeneity of AMPA receptor trafficking and molecular interactions revealed by superresolution analysis of live cell imaging. Proc. Natl. Acad. Sci. U S A 109, 17052–17057. doi: 10.1073/pnas.1204589109
Jacob, T. C., Bogdanov, Y. D., Magnus, C., Saliba, R. S., Kittler, J. T., Haydon, P. G., et al. (2005). Gephyrin regulates the cell surface dynamics of synaptic GABAA receptors. J. Neurosci. 25, 10469–10478. doi: 10.1523/JNEUROSCI.2267-05.2005
Kakizuka, T., Ikezaki, K., Kaneshiro, J., Fujita, H., Watanabe, T. M., and Ichimura, T. (2016). Simultaneous nano-tracking of multiple motor proteins via spectral discrimination of quantum dots. Biomed. Opt. Express 7, 2475–2493. doi: 10.1364/boe.7.002475
Kittler, J. T., Delmas, P., Jovanovic, J. N., Brown, D. A., Smart, T. G., and Moss, S. J. (2000). Constitutive endocytosis of GABAA receptors by an association with the adaptin AP2 complex modulates inhibitory synaptic currents in hippocampal neurons. J. Neurosci. 20, 7972–7977. doi: 10.1523/JNEUROSCI.20-21-07972.2000
Kowalczyk, S., Winkelmann, A., Smolinsky, B., Förstera, B., Neundorf, I., Schwarz, G., et al. (2013). Direct binding of GABAA receptor β2 and β3 subunits to gephyrin. Eur. J. Neurosci. 37, 544–554. doi: 10.1111/ejn.12078
Kusumi, A., Sako, Y., and Yamamoto, M. (1993). Confined lateral diffusion of membrane receptors as studied by single particle tracking (nanovid microscopy). Effects of calcium-induced differentiation in cultured epithelial cells. Biophys. J. 65, 2021–2040. doi: 10.1016/s0006-3495(93)81253-0
Lee, S.-H., Shin, J. Y., Lee, A., and Bustamante, C. (2012). Counting single photoactivatable fluorescent molecules by photoactivated localization microscopy (PALM). Proc. Natl. Acad. Sci. U S A 109, 17436–17441. doi: 10.1073/pnas.1215175109
Lévi, S., Le Roux, N., Eugène, E., and Poncer, J. C. (2015). Benzodiazepine ligands rapidly influence GABAA receptor diffusion and clustering at hippocampal inhibitory synapses. Neuropharmacology 88, 199–208. doi: 10.1016/j.neuropharm.2014.06.002
Lévi, S., Schweizer, C., Bannai, H., Pascual, O., Charrier, C., and Triller, A. (2008). Homeostatic regulation of synaptic GlyR numbers driven by lateral diffusion. Neuron 59, 261–273. doi: 10.1016/j.neuron.2008.05.030
Loebrich, S., Bähring, R., Katsuno, T., Tsukita, S., and Kneussel, M. (2006). Activated radixin is essential for GABAA receptor α5 subunit anchoring at the actin cytoskeleton. EMBO J. 25, 987–999. doi: 10.1038/sj.emboj.7600995
Lucchesi, W., Mizuno, K., and Giese, K. P. (2011). Novel insights into CaMKII function and regulation during memory formation. Brain Res. Bull. 85, 2–8. doi: 10.1016/j.brainresbull.2010.10.009
Lüthi, A., Chittajallu, R., Duprat, F., Palmer, M. J., Benke, T. A., Kidd, F. L., et al. (1999). Hippocampal LTD expression involves a pool of AMPARs regulated by the NSF-GluR2 interaction. Neuron 24, 389–399. doi: 10.1016/s0896-6273(00)80852-1
MacGillavry, H. D., Song, Y., Raghavachari, S., and Blanpied, T. A. (2013). Nanoscale scaffolding domains within the postsynaptic density concentrate synaptic AMPA receptors. Neuron 78, 615–622. doi: 10.1016/j.neuron.2013.03.009
Makino, H., and Malinow, R. (2009). AMPA receptor incorporation into synapses during LTP: the role of lateral movement and exocytosis. Neuron 64, 381–390. doi: 10.1016/j.neuron.2009.08.035
Mammen, A., Huganir, R., and O’Brien, R.-J. (1997). Redistribution and stabilization of cell surface glutamate receptors during synapse formation. J. Neurosci. 17, 7351–7358. doi: 10.1523/JNEUROSCI.17-19-07351.1997
Mammoto, A., Sasaki, T., Asakura, T., Hotta, I., Imamura, H., Takahashi, K., et al. (1998). Interactions of drebrin and gephyrin with profilin. Biochem. Biophys. Res. Commun. 243, 86–89. doi: 10.1006/bbrc.1997.8068
Marguet, D., Lenne, P.-F., Rigneault, H., and He, H.-T. (2006). Dynamics in the plasma membrane: how to combine fluidity and order. EMBO J. 25, 3446–3457. doi: 10.1038/sj.emboj.7601204
Maric, H.-M., Mukherjee, J., Tretter, V., Moss, S. J., and Schindelin, H. (2011). Gephyrin-mediated γ-aminobutyric acid type A and glycine receptor clustering relies on a common binding site. J. Biol. Chem. 286, 42105–42114. doi: 10.1074/jbc.M111.303412
Marsden, K. C., Beattie, J. B., Friedenthal, J., and Carroll, R. C. (2007). NMDA receptor activation potentiates inhibitory transmission through GABA receptor-associated protein-dependent exocytosis of GABAA receptors. J. Neurosci. 27, 14326–14337. doi: 10.1523/JNEUROSCI.4433-07.2007
Masson, J.-B., Dionne, P., Salvatico, C., Renner, M., Specht, C. G., Triller, A., et al. (2014). Mapping the energy and diffusion landscapes of membrane proteins at the cell surface using high-density single-molecule imaging and bayesian inference: application to the multiscale dynamics of glycine receptors in the neuronal membrane. Biophys. J. 106, 74–83. doi: 10.1016/j.bpj.2013.10.027
Meier, J., and Grantyn, R. (2004). A gephyrin-related mechanism restraining glycine receptor anchoring at GABAergic synapses. J. Neurosci. 24, 1398–1405. doi: 10.1523/JNEUROSCI.4260-03.2004
Meier, J., Vannier, C., Sergé, A., Triller, A., and Choquet, D. (2001). Fast and reversible trapping of surface glycine receptors by gephyrin. Nat. Neurosci. 4, 253–260. doi: 10.1038/85099
Muir, J., Arancibia-Carcamo, I. L., MacAskill, A. F., Smith, K. R., Griffin, L. D., and Kittler, J. T. (2010). NMDA receptors regulate GABAA receptor lateral mobility and clustering at inhibitory synapses through serine 327 on the 2 subunit. Proc. Natl. Acad. Sci. U S A 107, 16679–16684. doi: 10.1073/pnas.1000589107
Mukherjee, J., Cardarelli, R. A., Cantaut-Belarif, Y., Deeb, T. Z., Srivastava, D. P., Tyagarajan, S. K., et al. (2017). Estradiol modulates the efficacy of synaptic inhibition by decreasing the dwell time of GABAA receptors at inhibitory synapses. Proc. Natl. Acad. Sci. U S A 114, 11763–11768. doi: 10.1073/pnas.1705075114
Mukherjee, J., Kretschmannova, K., Gouzer, G., Maric, H.-M., Ramsden, S., Tretter, V., et al. (2011). The residence time of GABAARs at inhibitory synapses is determined by direct binding of the receptor 1 subunit to gephyrin. J. Neurosci. 31, 14677–14687. doi: 10.1523/JNEUROSCI.2001-11.2011
Nair, D., Hosy, E., Petersen, J. D., Constals, A., Giannone, G., Choquet, D., et al. (2013). Super-resolution imaging reveals that AMPA receptors inside synapses are dynamically organized in nanodomains regulated by PSD95. J. Neurosci. 33, 13204–13224. doi: 10.1523/JNEUROSCI.2381-12.2013
Nishimune, A., Isaac, J. T., Molnar, E., Noel, J., Nash, S. R., Tagaya, M., et al. (1998). NSF binding to GluR2 regulates synaptic transmission. Neuron 21, 87–97. doi: 10.1016/s0896-6273(00)80517-6
Niwa, F., Bannai, H., Arizono, M., Fukatsu, K., Triller, A., and Mikoshiba, K. (2012). Gephyrin-independent GABAAR mobility and clustering during plasticity. PLoS One 7:e36148. doi: 10.1371/journal.pone.0036148
Nusser, Z., Hájos, N., Somogyi, P., and Mody, I. (1998). Increased number of synaptic GABAA receptors underlies potentiation at hippocampal inhibitory synapses. Nature 395, 172–177. doi: 10.1038/25999
Park, M., Penick, E. C., Edwards, J. G., Kauer, J. A., and Ehlers, M. D. (2004). Recycling endosomes supply AMPA receptors for LTP. Science 305, 1972–1975. doi: 10.1126/science.1102026
Passafaro, M., Piëch, V., and Sheng, M. (2001). Subunit-specific temporal and spatial patterns of AMPA receptor exocytosis in hippocampal neurons. Nat. Neurosci. 4, 917–926. doi: 10.1038/nn0901-917
Penn, A. C., Zhang, C. L., Georges, F., Royer, L., Breillat, C., Hosy, E., et al. (2017). Hippocampal LTP and contextual learning require surface diffusion of AMPA receptors. Nature 549, 384–388. doi: 10.1038/nature23658
Pennacchietti, F., Vascon, S., Nieus, T., Rosillo, C., Das, S., Tyagarajan, S. K., et al. (2017). Nanoscale molecular reorganization of the inhibitory postsynaptic density is a determinant of GABAergic synaptic potentiation. J. Neurosci. 37, 1747–1756. doi: 10.1523/JNEUROSCI.0514-16.2016
Petrini, E. M., and Barberis, A. (2014). Diffusion dynamics of synaptic molecules during inhibitory postsynaptic plasticity. Front. Cell. Neurosci. 8:300. doi: 10.3389/fncel.2014.00300
Petrini, E. M., Ravasenga, T., Hausrat, T. J., Iurilli, G., Olcese, U., Racine, V., et al. (2014). Synaptic recruitment of gephyrin regulates surface GABAA receptor dynamics for the expression of inhibitory LTP. Nat. Commun. 5:3921. doi: 10.1038/ncomms4921
Poo, M., and Cone, R. A. (1974). Lateral diffusion of rhodopsin in the photoreceptor membrane. Nature 247, 438–441. doi: 10.1038/247438a0
Pooler, A. M., and McIlhinney, R. A. J. (2007). Lateral diffusion of the GABAB receptor is regulated by the GABAB2 C terminus. J. Biol. Chem. 282, 25349–25356. doi: 10.1074/jbc.M702358200
Ranft, J., Almeida, L. G., Rodriguez, P. C., Triller, A., and Hakim, V. (2017). An aggregation-removal model for the formation and size determination of post-synaptic scaffold domains. PLoS Comput. Biol. 13:e1005516. doi: 10.1371/journal.pcbi.1005516
Renner, M., Choquet, D., and Triller, A. (2009). Control of the postsynaptic membrane viscosity. J. Neurosci. 29, 2926–2937. doi: 10.1523/JNEUROSCI.4445-08.2009
Renner, M., Specht, C. G., and Triller, A. (2008). Molecular dynamics of postsynaptic receptors and scaffold proteins. Curr. Opin. Neurobiol. 18, 532–540. doi: 10.1016/j.conb.2008.09.009
Renner, M., Domanov, Y., Sandrin, F., Izeddin, I., Bassereau, P., and Triller, A. (2011). Lateral diffusion on tubular membranes: quantification of measurements bias. PLoS One 6:e25731. doi: 10.1371/journal.pone.0025731
Renner, M., Wang, L., Levi, S., Hennekinne, L., and Triller, A. (2017). A simple and powerful analysis of lateral subdiffusion using single particle tracking. Biophys. J. 113, 2452–2463. doi: 10.1016/j.bpj.2017.09.017
Rosenberg, M., Meier, J., Triller, A., and Vannier, C. (2001). Dynamics of glycine receptor insertion in the neuronal plasma membrane. J. Neurosci. 21, 5036–5044. doi: 10.1523/jneurosci.21-14-05036.2001
Salvatico, C., Specht, C. G., and Triller, A. (2015). Synaptic receptor dynamics: from theoretical concepts to deep quantification and chemistry in cellulo. Neuropharmacology 88, 2–9. doi: 10.1016/j.neuropharm.2014.09.020
Saxton, M. J., and Jacobson, K. (1997). Single-particle tracking: applications to membrane dynamics. Annu. Rev. Biophys. Biomol. Struct. 26, 373–399. doi: 10.1146/annurev.biophys.26.1.373
Sekimoto, K., and Triller, A. (2009). Compatibility between itinerant synaptic receptors and stable postsynaptic structure. Phys. Rev. E Stat. Nonlin. Soft Matter Phys. 79:031905. doi: 10.1103/physreve.79.031905
Sergé, A., Bertaux, N., Rigneault, H., and Marguet, D. (2008). Dynamic multiple-target tracing to probe spatiotemporal cartography of cell membranes. Nat. Methods 5, 687–694. doi: 10.1038/nmeth.1233
Sergé, A., Fourgeaud, L., Hémar, A., and Choquet, D. (2002). Receptor activation and homer differentially control the lateral mobility of metabotropic glutamate receptor 5 in the neuronal membrane. J. Neurosci. 22, 3910–3920. doi: 10.1523/jneurosci.22-10-03910.2002
Sharonov, A., and Hochstrasser, R. M. (2006). Wide-field subdiffraction imaging by accumulated binding of diffusing probes. Proc. Natl. Acad. Sci. U S A 103, 18911–18916. doi: 10.1073/pnas.0609643104
Singer, S. J., and Nicolson, G. L. (1972). The fluid mosaic model of the structure of cell membranes. Science 175, 720–731. doi: 10.1126/science.175.4023.720
Song, I., and Huganir, R. L. (2002). Regulation of AMPA receptors during synaptic plasticity. Trends Neurosci. 25, 578–588. doi: 10.1016/s0166-2236(02)02270-1
Specht, C. G., Grünewald, N., Pascual, O., Rostgaard, N., Schwarz, G., and Triller, A. (2011). Regulation of glycine receptor diffusion properties and gephyrin interactions by protein kinase C. EMBO J. 30, 3842–3853. doi: 10.1038/emboj.2011.276
Specht, C. G., Izeddin, I., Rodriguez, P. C., El Beheiry, M., Rostaing, P., Darzacq, X., et al. (2013). Quantitative nanoscopy of inhibitory synapses: counting gephyrin molecules and receptor binding sites. Neuron 79, 308–321. doi: 10.1016/j.neuron.2013.05.013
Stepanenko, O. V., Stepanenko, O. V., Kuznetsova, I. M., Verkhusha, V. V., and Turoverov, K. K. (2013). Beta-barrel scaffold of fluorescent proteins: folding, stability and role in chromophore formation. Int. Rev. Cell Mol. Biol. 302, 221–278. doi: 10.1016/B978-0-12-407699-0.00004-2
Tanaka, H., and Hirano, T. (2012). Visualization of subunit-specific delivery of glutamate receptors to postsynaptic membrane during hippocampal long-term potentiation. Cell Rep. 1, 291–298. doi: 10.1016/j.celrep.2012.02.004
Tanudji, M., Hevi, S., and Chuck, S. L. (2002). Improperly folded green fluorescent protein is secreted via a non-classical pathway. J. Cell Sci. 115, 3849–3857. doi: 10.1242/jcs.00047
Tardin, C., Cognet, L., Bats, C., Lounis, B., and Choquet, D. (2003). Direct imaging of lateral movements of AMPA receptors inside synapses. EMBO J. 22, 4656–4665. doi: 10.1093/emboj/cdg463
Thomas, P., Mortensen, M., Hosie, A. M., and Smart, T. G. (2005). Dynamic mobility of functional GABAA receptors at inhibitory synapses. Nat. Neurosci. 8, 889–897. doi: 10.1038/nn1483
Tretter, V., Jacob, T. C., Mukherjee, J., Fritschy, J.-M., Pangalos, M. N., and Moss, S. J. (2008). The clustering of GABAA receptor subtypes at inhibitory synapses is facilitated via the direct binding of receptor 2 subunits to gephyrin. J. Neurosci. 28, 1356–1365. doi: 10.1523/JNEUROSCI.5050-07.2008
Turrigiano, G. G. (2000). AMPA receptors unbound: membrane cycling and synaptic plasticity. Neuron 26, 5–8. doi: 10.1016/s0896-6273(00)81131-9
Keywords: diffusion-trapping, inhibitory synapse, GABAA receptor, glycine receptor, gephyrin, single-particle tracking
Citation: Maynard SA and Triller A (2019) Inhibitory Receptor Diffusion Dynamics. Front. Mol. Neurosci. 12:313. doi: 10.3389/fnmol.2019.00313
Received: 06 August 2019; Accepted: 04 December 2019;
Published: 19 December 2019.
Edited by:
Shiva K. Tyagarajan, University of Zurich, SwitzerlandReviewed by:
Enrica Maria Petrini, Italian Institute of Technology (IIT), ItalyCopyright © 2019 Maynard and Triller. This is an open-access article distributed under the terms of the Creative Commons Attribution License (CC BY). The use, distribution or reproduction in other forums is permitted, provided the original author(s) and the copyright owner(s) are credited and that the original publication in this journal is cited, in accordance with accepted academic practice. No use, distribution or reproduction is permitted which does not comply with these terms.
*Correspondence: Antoine Triller, dHJpbGxlckBiaW9sb2dpZS5lbnMuZnI=
Disclaimer: All claims expressed in this article are solely those of the authors and do not necessarily represent those of their affiliated organizations, or those of the publisher, the editors and the reviewers. Any product that may be evaluated in this article or claim that may be made by its manufacturer is not guaranteed or endorsed by the publisher.
Research integrity at Frontiers
Learn more about the work of our research integrity team to safeguard the quality of each article we publish.