- 1Department of Neurosurgery, Tangdu Hospital, Air Force Military Medical University, Xi’an, China
- 2Beijing Institute of Biotechnology, Beijing, China
- 3Department of Neurosurgery, Xi’an International Medical Center, Xi’an, China
- 4The School of Medicine, Medical Sciences and Nutrition, Institute of Medical Sciences, University of Aberdeen, Aberdeen, United Kingdom
- 5Department of Psychiatry, The Second Xiangya Hospital, Central South University, Changsha, China
The small GTPases from the Ras superfamily play crucial roles in basic cellular processes during practically the entire process of neurodevelopment, including neurogenesis, differentiation, gene expression, membrane and protein traffic, vesicular trafficking, and synaptic plasticity. Small GTPases are key signal transducing enzymes that link extracellular cues to the neuronal responses required for the construction of neuronal networks, as well as for synaptic function and plasticity. Different subfamilies of small GTPases have been linked to a number of non-neoplastic cerebral diseases such as Alzheimer’s disease (AD), Parkinson’s disease (PD), intellectual disability, epilepsy, drug addiction, Huntington’s disease (HD), amyotrophic lateral sclerosis (ALS) and a large number of idiopathic cerebral diseases. Here, we attempted to make a clearer illustration of the relationship between Ras superfamily GTPases and non-neoplastic cerebral diseases, as well as their roles in the neural system. In future studies, potential treatments for non-neoplastic cerebral diseases which are based on small GTPase related signaling pathways should be explored further. In this paper, we review all the available literature in support of this possibility.
Introduction
Small GTPases are defined by their basic biochemical activity of binding GTP and hydrolyzing it to GDP, which is called the guanosine triphosphate (GTP)/guanosine diphosphate (GDP) cycle (Bourne et al., 1991). Although similar to the heterotrimeric G protein α subunits in biochemistry and function, small GTPases function as monomeric G proteins (Claing, 2013). In addition to their high affinity and hydrolysis activity for GTP, small GTPases have two states, a GTP-bound state and a GDP-bound state. The activated small G-proteins are mainly regulated by three crucial factors: Guanosine nucleotide dissociation inhibitors (GDIs), guanine nucleotide exchange factors (GEFs) and GTPase activator proteins (GAPs) (Buday and Downward, 2008; Vigil et al., 2010; Liu et al., 2017). Figure 1 shows a simple schematic diagram of their function. In this cycle, GAPs promote GTP hydrolysis, and GEFs stimulate the exchange of GDP for GTP (McCormick, 1998). GDIs can be defined as a class of proteins interacted with small GTPases, which not only prevent exchange (maintaining the small GTPases in an off-state), but also prevent the small GTPase from localizing at the membrane (Cherfils and Zeghouf, 2013). Importantly, these molecular switches affect almost all cellular processes such as gene expression, microtubule organization, cytoskeleton reorganization, and vesicular and nuclear transport (Johnson and Chen, 2012). Small GTPases can also be influenced by post-translational modifications such as phosphorylation or ubiquitination, which can regulate protein stability and subcellular localization (Ahearn et al., 2011).
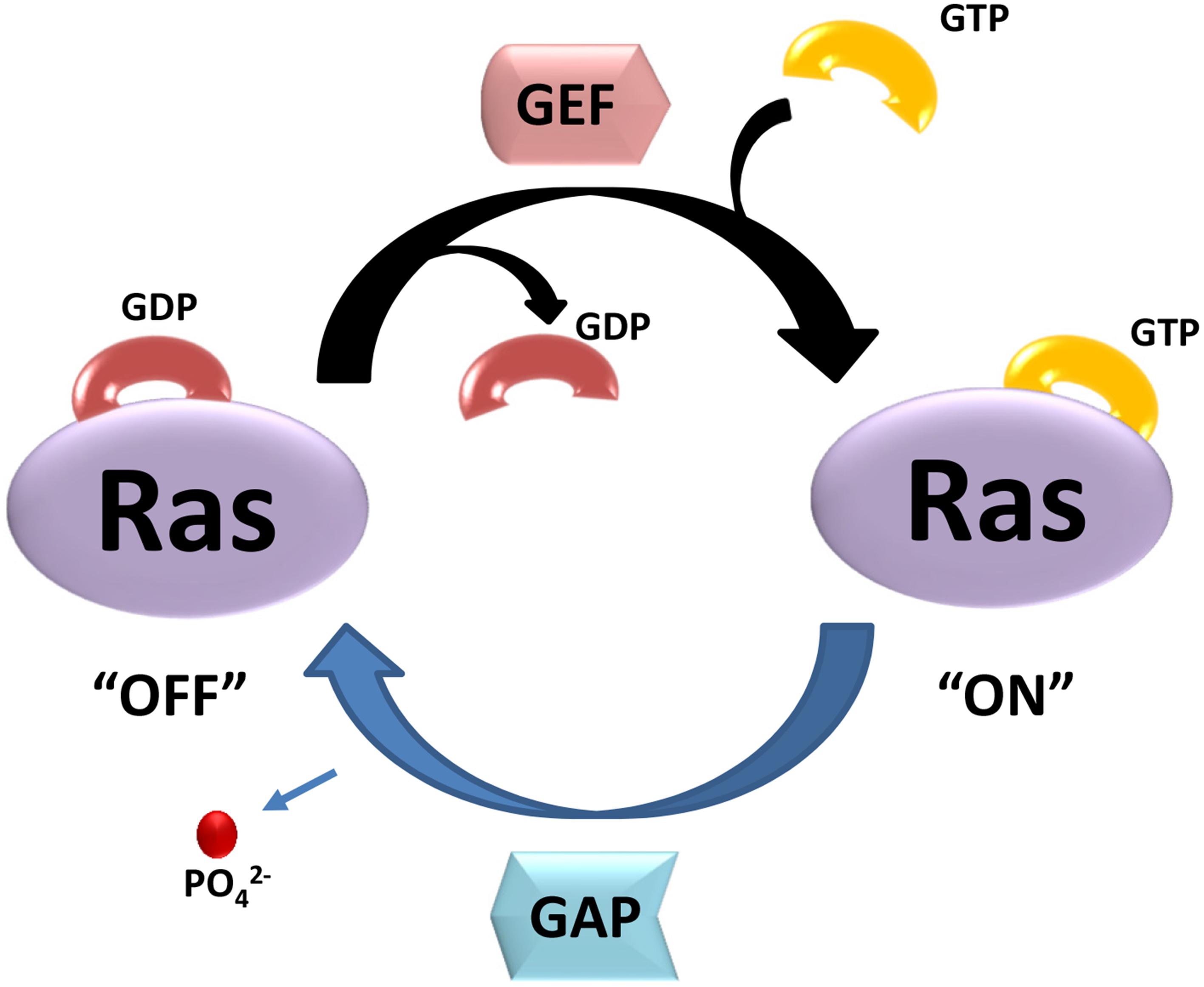
Figure 1. The two state of small GTPases. GTP- and GDP-bound state are regulated by GEFs and GAPs. GEFs stimulate the exchange of GDP for GTP, resulting activation of Ras (“ON”). GAPs promote GTP hydrolysis, and return Ras to GDP-bound state (“OFF”).
To date, 167 small GTPases have been identified in humans (Supplementary Table S1) (Rojas et al., 2012; Liu et al., 2017). We compared the G-domin region of those small GTPases, and found that there exist 5 repeated sequences. After removing 5 repeated amino acid sequences that are identical, the resulting 162 amino acid sequences were analyzed and a sequence-homology based phylogenetic tree (Figure 2) was constructed using the Neighbor-Joining method (Saitou and Nei, 1987). According to sequence and functional similarity, small GTPases can be divided into five main families whose eponymous members are Ras, Rho, Rab, ADP-ribosylation factor (Arf) and Ran (Wennerberg et al., 2005). Ras family members, the first members of the superfamily to be discovered, are signal nodes mediating the responses to various extracellular stimuli and can regulate cell proliferation, differentiation, morphology, and apoptosis by binding to a variety of effector molecules with different catalytic activities to regulate the cytoplasmic signaling network (Karnoub and Weinberg, 2008). The Rho family includes more than 20 proteins. This family is known for its role in actin cytoskeleton remodeling and cell polarity (Fransson et al., 2003; Heo and Meyer, 2003). The three best known members are Rho (A, B, C), Rac and Cdc42 (Takai et al., 2001; Shenoy and Lefkowitz, 2011; Reiter et al., 2012; Claing, 2013). Among them, RhoA, Rac1, and Cdc42 have been studied the most (Schwartz and Shattil, 2000; Rolfe et al., 2005; Grosshans et al., 2006). The Rab family is by far the largest family of the Ras superfamily in humans (Rojas et al., 2012). Proteins of this family participate in vesicle formation, movement and fusion, and vesicular cargo trafficking (Pereira-Leal and Seabra, 2001; Segev, 2001). The Arf family includes 30 proteins and is also involved in vesicle trafficking. Ran is the only member found in the Ran family, which is present in all eukaryotic lineages and involved in nuclear transport (Weis, 2003).
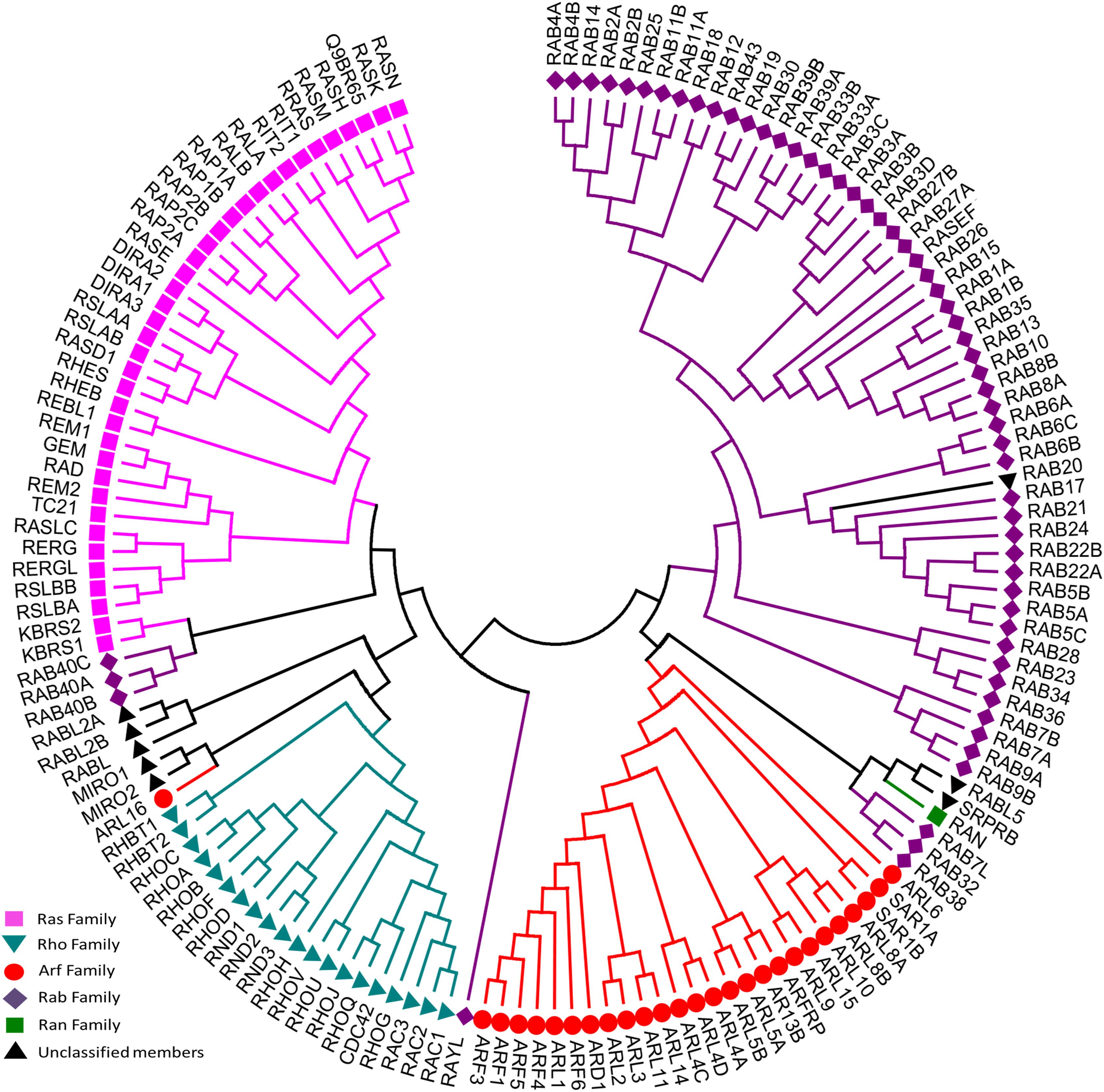
Figure 2. Evolutionary relationships of human Ras superfamily. The evolutionary history was inferred using the Neighbor-Joining method. After removing identical sequences, 162 amino acid sequences (Supplementary Table S1) are used for the analysis. All ambiguous positions were removed for each sequence pair. The original classification was indicated by different colors: Ras (lilac), Rho (cyan), Rab (purple), Arf (red), Ran (green), and Unclassified members (blank). Evolutionary analyses were conducted in MEGA7.
All the proteins have a GTP-binding domain of about 20 kDa, also known as the G-domain (Bourne et al., 1991; Takai et al., 2001). As shown in Figure 3, this domain consists of five α helices (A1–A5), six β-strands (B1–B6) and five polypeptide loops (G1–G5). This domain is highly conserved overall, and especially the loops (Paduch et al., 2001). When bound to GDP, small GTPases are inactive. The two states have similar conformations and can be distinguished by two functional loop regions: switch I (corresponds to the G2 loop) and switch II (corresponds to the G3 loop and part of the A2 helix) (Figure 3) (Jurnak, 1985; Paduch et al., 2001). The G1 loop is located between the B1 strands and A1 helix with the motif X1X1X1GXXXXGK (S/T), where X1 is leucine (L), valine (V) or isoleucine (I), and X is any amino acid. The α- and β-phosphate groups can be bound to this motif (Knihtila et al., 2015). The G2 loop, with only one conserved threonine (T), that connects the A1 helix and the B2 strand is responsible for the binding of Mg2+ via conserved amino acid residues. The G3 loop is at the N-terminus of the A2 helix with the motif XXXXDXXGX. Its main function is to bind Mg2+ and the γ-phosphoric acid group of GTP or GDP. The G4 loop containing the motif XXX (G/A) (T/N) KXD and the G5 loop are mainly responsible for the recognition of the guanine base (Figure 4). Although the G-domain is conserved in the superfamily, there are also individual difference among the different small GTPase families. For example, in G1 loop, the sixth position G is only conserved in the Rho, Rab, and Arf families. On the other hand, covalent post-translational modification by lipids is another feature of small GTPases. These modifications are essential for facilitating membrane association and subcellular localization, which is critical for their respective biological activities (Hancock, 2003; Prior and Hancock, 2012). The C-terminal cysteine residue of the motif can be prenylated by farnesyltransferase or geranylgeranyltransferase. This modification was observed for most Ras, Rho, and Rab family members despite the different motifs, which is composed of CAAX (C is Cys, A is aliphatic and X is any amino acid) for Ras and Rho family proteins, and CC, CXC, CCX, CCXX, or CCXXX for the Rab family (Cox and Der, 2002; Wennerberg et al., 2005). Some Ras superfamily members without lipid modification, such as Rit, RhoBTB, Miro, and Sar1, can also associate with membranes (Wennerberg et al., 2005). Arf family proteins lack C-terminal lipid modification signals and some members are modified at their N-termini by a myristoylation (Colicelli, 2004). By contrast, Ran does not exhibit any detectable modification by lipids and binding to membranes (Wennerberg et al., 2005).
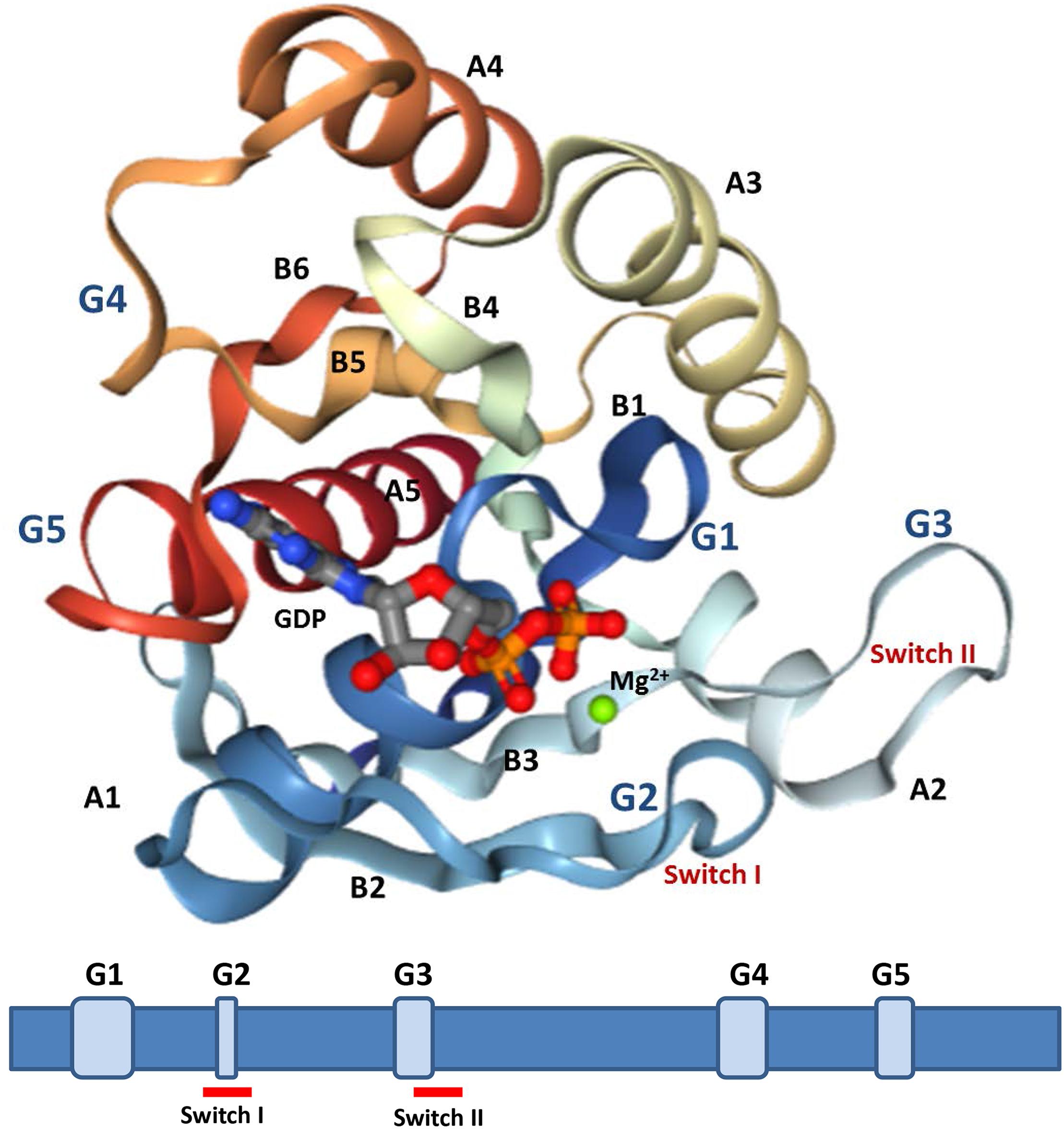
Figure 3. Structures analysis of Ras. The crystal structures of Ras GDP Mg2+ complex (PDB 4q21) is showed (upper). This structure contains five α-helices (A1–A5), six β-strands (B1–B6), and five polypeptide loops (G1–G5) and the position relationship among various parts is displayed (below).
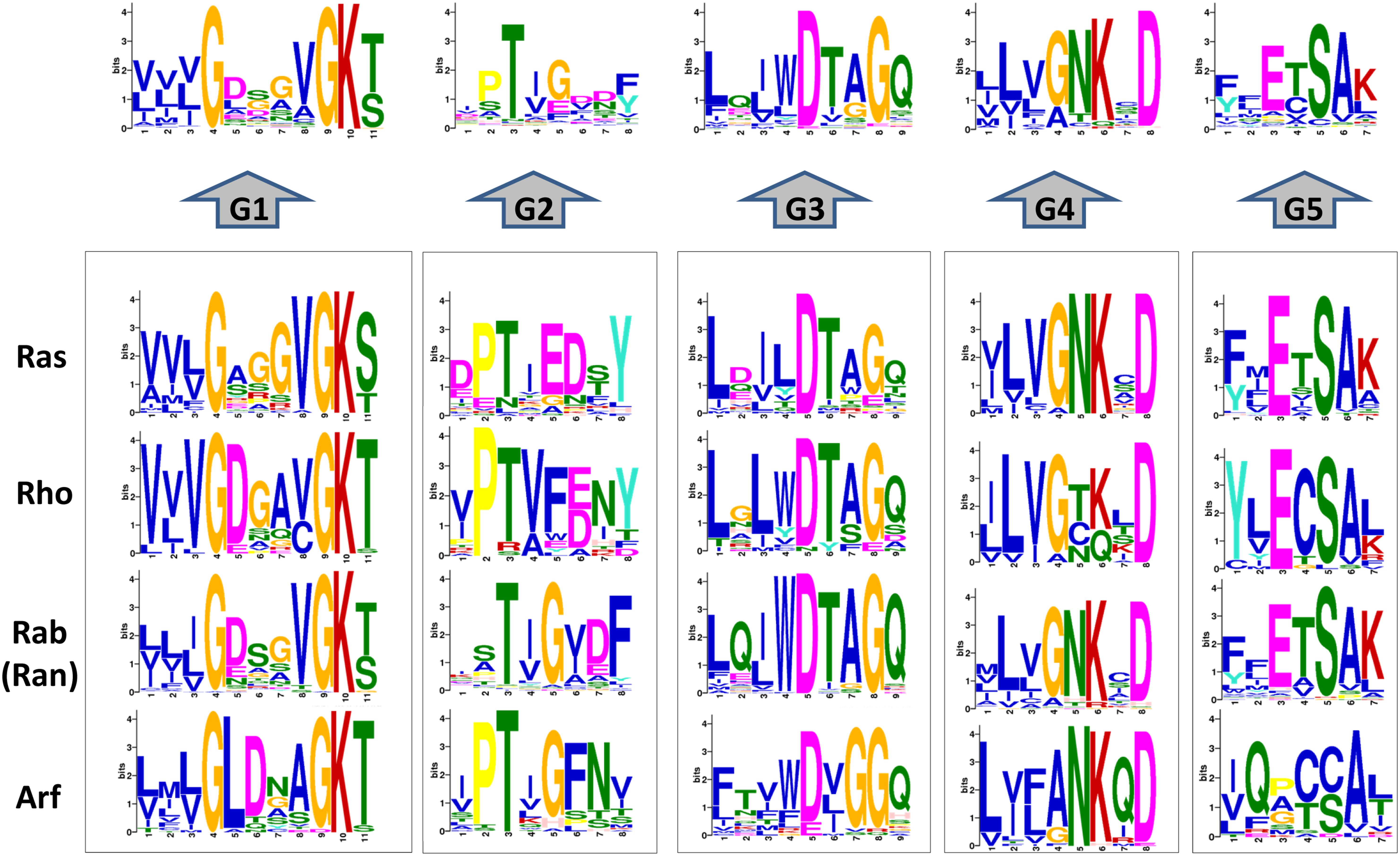
Figure 4. Conservation analysis of the 167 human G-domain of Ras superfamily and its subfamilies (http://meme-suite.org/). Considering that the Ran only has one sequence and is a branch of Rab subfamily, we incorporate Ran into Rab family.
Studies of the functions of Ras superfamily proteins in modulating the diverse signaling cascades have established many current fundamental paradigms of signal transduction, mainly based on decades of oncological investigations. The awareness of aberrant Ras-related signaling in the pathogenesis of other human disorders has also risen in recent years. Here we highlight the critical roles of each family of the Ras superfamily in non-neoplastic cerebral diseases (Tables 1–3), which in turn may suggest novel therapeutic targets for developmental diseases and cognitive impairments.
The Ras Family in Non-Neoplastic Cerebral Diseases
The Ras Family
As the first described and prototypical members of the small GTPase superfamily, Ras family proteins are almost universal components of signaling pathways in eukaryotic organisms, including vertebrates, invertebrates, and yeasts (Goitre et al., 2014). Ras genes were first identified as oncogenes, and were named after the Rat Sarcoma gene in the 1970s during the extensive study of acutely transforming retroviruses isolated from mice, rats, and other animals (Cox and Der, 2010). Following years of investigation, the widespread prevalence of Ras mutations in the context of carcinogenesis has now been widely recognized. This has inspired multiple attempts to find Ras inhibitors. However, as for the role of the Ras family in non-neoplastic diseases, we are still at the beginning stage of discovering how the related proteins and their precise mechanisms control and modulate non-oncogenic processes (Johnson and Chen, 2012).
According to their evolutionarily conservation in structural, biochemical, and functional levels, the Ras family is composed of over 36 members, with six major subfamilies: Ras subfamily (H-Ras, K-Ras, N-Ras, etc.), Ral (RalA, RalB), Rap (Rap1, Rap2), Rad (Rad, Gem, etc.), Rheb (Rheb1, and Rheb2 or RhebL1) and Rit (Rit1 or Rit, Rit2 or Rin, and Drosophila dRic) (Goitre et al., 2014).
Most Ras family proteins are predominantly localized on the intracellular surface of the plasma membrane as a result of C-terminal prenylation (Colicelli, 2004). They can be activated in response to multiple extracellular stimuli in the form of growth factors and small molecules, which thereby transduce signals to the intracellular environment to regulate cytoplasmic signaling networks (Ye and Carew, 2010).
The best-characterized downstream signaling cascade of Ras family proteins is the Mitogen-Activated Protein Kinase (MAPK) cascade, mainly the Extracellular signal-Regulated Kinase 1/2 (ERK) of the MAPK family (Ye and Carew, 2010). Ras family proteins can directly bind to the regulatory domain of Raf (c-Raf or b-Raf) and subsequently phosphorylate and dephosphorylate it at multiple sites for full activation. In turn, activated Raf phosphorylates and activates MAP Kinase or ERK Kinase (MEK), and finally targets transcription factors to induce gene expression (Lange-Carter and Johnson, 1994). Take Rap subfamily members for example. Rap1 can activate ERK by activating b-Raf (expressed predominantly in the brain) and p38MAPK, which implies a pivotal role in synaptic depression (Grewal et al., 2000; Kanda and Watanabe, 2007). Furthermore, Rap2 is involved in the activation of c-Jun N-terminal Kinase (JNK) (Machida et al., 2004). In addition to the Raf–MEK–ERK cascade, other well-known pathways include the phosphatidylinositol 3-kinase (PI3K), RalGEF-Ral, and phospholipase C epsilon (PLCε) pathways (Pulciani et al., 1982; Santos et al., 1984).
The Rit subfamily is composed of two classes, Rit and Rin (Ras like without CAAX2, Rit2), each containing a well-conserved GTPase core (G1–G5). Distinct patterns of developmental expression for both proteins were found, whereby Rit is widely expressed throughout development, while Rin shows delayed expressed limited to later stages of embryonic neuronal development (E14), with highest Rin expression found in the adult brain (Shi et al., 2013; Hamedani et al., 2017). Activated Rit was identified as playing a critical role in the regulation of neuronal morphogenesis, especially enhancing axonal growth, but with the opposite effect on dendritic growth (Lein et al., 2007).
Rin is involved in calcium-mediated processes in cells and binds to calmodulin through a C-terminal binding motif, regulating the Rin-mediated pathway (Hoshino and Nakamura, 2002; Daneshmandpour et al., 2018). Rin has an important role in intracellular signaling and interacts with DAT (dopamine active transporter) in lipid raft microdomains (Navaroli et al., 2011).
The Rheb subfamily (Ras homolog protein enriched in the brain) is a member of the Ras family that is highly conserved among different organisms (Urano et al., 2001; Schoneborn et al., 2018). Two different Rheb genes have been identified in mammalians, RHEB1 (from here on REHB), and RHEB2 (also as RHEBL1). RHEB is expressed in various human tissues, but RHEB2 is mainly expressed in the brain tissue, such as cerebral cortex, occipital pole, frontal, and temporal lobes (Saito et al., 2005; Schoneborn et al., 2018).
Mammalian target of rapamycin (mTOR) is a serine-threonine kinase that integrates signals to regulate cell growth and metabolism. In the brain, Rheb functions as a key activator of mTORC1, and deletion of RHEB causes decreased cortical thickness and defective myelination (Lee et al., 2015).
RalA and RalB are monomeric GTPases belonging to the Ral subclass, and both are expressed in the nervous system (Teodoro et al., 2013). Ral can be activated either by Ras indirectly via a Ral-GEF (Guanine nucleotide Exchange Factor) or by Ca2+/calmodulin binding, and it is inactivated by PKC phosphorylation of the effector Sec5 (Harvey et al., 2008; Chen et al., 2011). During brain development, it is considered that Ral is involved in the asymmetric division of neuroblasts, neuronal migration in the neocortex, neurite branching, and activity-dependent spine growth (Das et al., 2014). RalA regulates axon initiation in cortical neurons by promoting an interaction between the exocyst protein complex, which is one of its major effectors, and the Par3–Par6–aPKC complex, an evolutionarily conserved master regulator of cell polarity. However, in other cell migration process, such as tumor metastasis, it seems that RalB may play a more important role than RalA (Zago et al., 2017).
The Rap subfamily is composed of five related proteins, Rap1 (A and B) and Rap2 (A, B, and C), which have overlapping functions and expression patterns (Nakamura et al., 2013; Zhang L. et al., 2018). Similar to other members of the Ras family, Rap proteins work as molecular switches of multiple signal transduction cascades and are linked to several genetic defects related to mental, neurological and psychiatric disorders (Volk et al., 2015). Rap1 has been intensively investigated for its function in integrin-mediated cell adhesion and the regulation of cell–cell junction integrity (Jossin, 2011). A recent study indicates that Rap1 and Rap2 predominantly signal synaptic depression via the lysosomal p38MAPK and the bulk membrane JNK pathway, respectively, regulating different forms of synaptic plasticity (Zhang L. et al., 2018). Especially, Rap1B acts as a core molecule in the signaling network responsible for neuronal polarization (Nakamura et al., 2013).
Diseases Related to the Ras Family
Owing to the Ras family proteins’ essential role in modulating a wide range of cellular processes, several human diseases are caused by the dysregulation or dysfunction of related signaling pathways. These include cancer, developmental-, neurocognitive- and neurodegenerative disorders, as well as metabolic and cardiovascular diseases (Nakhaei-Rad et al., 2018). In this section, we reviewed some Ras family related developmental disorders and neurological diseases.
RASopathies
The RASopathies are the largest known group of rare human developmental disorders that are caused by germline mutations in genes that encode proteins of the RAS/MAPK pathway, resulting in its hyperactivation (Tajan et al., 2018). As mentioned above, the RAS/MAPK pathway is essential for the development of mammalian tissues, controlling a variety of cellular activities, such as cell cycle and growth, differentiation, metabolism and senescence (Slack, 2017). Although this signaling pathway is often summarized as a well-established cascade, there are still many gray areas and multilevel regulations, such as transcriptional control, posttranslational modifications, protein-protein interactions, and crosstalk with other signaling pathways. In this section, we mainly focus on individual symptoms, phenotypes and most evidenced gene mutations to the RASopathies. Further detailed information on comprehensive functional and pathophysiological consequences could be referred to suggested reviews (Atay and Skotheim, 2017; Simanshu et al., 2017).
The RASopathies includes neurofibromatosis type 1 (NF1), Legius syndrome (LS), capillary malformation–arterio-venous malformation syndrome (CM-AVM), Costello syndrome (CS), cardio-facio-cutaneous syndrome (CFC), Noonan syndrome (NS) and Noonan syndrome with multiple lentigines (NS-ML) (Simanshu et al., 2017; Dard et al., 2018). Although RASopathies exhibit unique phenotypes, they share many overlapping clinical characteristics due to the common pathway dysregulation, such as partial facial anomalies, cognitive impairment, and congenital heart defects (Nakhaei-Rad et al., 2018). As a whole, the RASopathies affect approximately 1 per 1,000 live births (Tajan et al., 2018). The initial diagnosis of a RASopathy patient is based on the clinical recognition of phenotypic features, and the clinical diagnosis is then confirmed by molecular genetic tests. To date, with the accumulation of genotype and phenotype correlation data, there are more than 20 gene mutations has been associated with RASopathies. Most mutations causing RASopathies occur at conserved positions within the RAS/MAPK pathway, providing a genetic foundation for their diagnosis and pathophysiology (Simanshu et al., 2017). Table 1 has listed several gene mutations related to small GTPases, especially the Ras family and RASopathies. However, considering the heterogeneity of a given syndrome and the pleiotropic roles during development and homeostasis maintenance, it is still difficult and fragmental to classify the causal genes and mutations involved with the genotype/phenotype correlations based on current progress.
NS was first described by Jacqueline Noonan about 50 years ago, and is also the most frequent RASopathy. Its estimated prevalence is between 1 in 2,000–2,500 newborns (Noonan, 1968; Tajan et al., 2018). It is an autosomal dominant disorder and the most genetically diverse RASopathy, which is clinically characterized by facial dysmorphic features, congenital heart defects, and growth retardation. More than half of patients with NS are identified as having mild to moderate developmental delay/learning disability, notably with social and communication difficulties, attention deficit, and language impairment (Cesarini et al., 2009). Most of the mutations responsible for NS affect familiar components of Ras pathways. The protein tyrosine phosphatase non-receptor type (PTPN11), encoding SHP2, is the first and major NS disease gene, which was found mutated in 50–60% of patients with NS (Atay and Skotheim, 2017). Other less common or rare mutation genes are related to the RAS/MAPK pathway as well, including SOS1, SOS2, H-Ras, K-Ras, R-Ras, N-Ras, M-Ras and Rit1, the GAP RasA2, the kinases CRAF, BRAF, MEK1, MEK2, and MAP3K8, CBL, MYST4, A2ML1, SHOC2, and its binding partner PP1 (Tidyman and Rauen, 2016b). SHOC2 mutations have been reported in combination with mutations in PTPN11 (SHP2) in NS (Ekvall et al., 2011). The diversity of the affected genes has determined the NS is the most genetically heterogeneous RASopathy. However, the role of these proteins in regulating the RAS/MAPK pathway is still not clear, and the underlying functional mechanisms and genotype/phenotype correlations remain to be elucidated (Tidyman and Rauen, 2016b; Simanshu et al., 2017).
NS-ML, formerly known as LEOPARD syndrome, is an autosomal dominant disorder that has a prevalence of fewer than 1 in 100,000 newborns (Sarkozy et al., 2008). However, considering that the phenotype of NS-ML is very close to that of NS, the diagnosis can be more difficult (Sarkozy et al., 2008). Distinctive clinical features for NS-ML are a high prevalence of hearing deficits (20%) and multiple pigmented skin lesions called lentigines, mostly starting at school age (90%) (Sarkozy et al., 2008). Missense mutations in PTPN11 (Legius et al., 1993; Digilio et al., 2006) and rarely in RAF1 (Pandit et al., 2007) and BRAF (Digilio et al., 2006) are associated with NSML. Loss of function mutations in the PTPN11 gene result in reduced SHP2 activity, which is not found in NS (Digilio et al., 2002; Kontaridis et al., 2006). A recent study implied that the Ras-related Akt-mTOR signaling pathway is implicated in NS-ML phenotypes (Marin et al., 2011).
Noonan-like syndrome with loose anagen hair (NSLAH), also known as Mazzanti syndrome, is phenotypically close to NS, but patients display distinctive hyperactive behavior and pathognomonic hair anomalies (Gripp et al., 2016). SHOC2 and PPP1CB have been indicated with the onset of the disease (Atay and Skotheim, 2017). NF1, the second-most frequent RASopathy, is an autosomal dominant genetic disorder that was first described in 1882 (Garcia-Romero et al., 2016; Simanshu et al., 2017). The incidence of NF1 is 1 per 2,500–3,000 in newborns, with approximately 50% of NF1 patients inheriting the mutation from a parent (Williams et al., 2009). The characteristic feature used for NF1 diagnosis is the presence of café-au-lait macules. Benign tumors (neurofibromas and optic pathway gliomas), iris Lisch nodules, bone malformations (limb pseudarthrosis), cardiac malformations, brain malformations, seizures, and mild neurocognitive impairment can aid the diagnosis (Huffmeier et al., 2006; Stevenson et al., 2006). NF1 is caused by mutations in the NF1 gene on chromosome 17q11.2, which encodes neurofibromin, a GAP that negatively regulates Ras (Cawthon et al., 1990; Legius et al., 1993). NF1 mutations result in the loss of function of neurofibromin, which in turn reduces Ras GTPase activity and finally increases the levels of active GTP-bound Ras. Studies have suggested that neurofibromin can act on M-Ras, R-Ras, and R-Ras2 (a.k.a. TC21) (Ohba et al., 2000). R-Ras and N-Ras activate PI3Kγ, while M-Ras recruits SHOC2/PP1c to the plasma membrane to regulate SCRIB activity (Rodriguez-Viciana et al., 2004; Young et al., 2013). As a consequence, the loss of neurofibromin may have broader impacts on cells than the activation of H-Ras, N-Ras, and K-Ras proteins themselves (Simanshu et al., 2017).
Legius syndrome is a milder form of neurofibromatosis type 1, which shares phenotypic features with NF1, albeit in less severe form (Tajan et al., 2018). However, the NF1 gene is intact and heterozygous inactivating mutations in the SPRED1 gene on chromosome 15q13.2 occur in LS (Brems et al., 2007). SPRED1 functions as a negative regulator of Ras by inhibiting the phosphorylation of Raf (Wakioka et al., 2001). The SPRED1 proteins are essential for the interaction of neurofibromin with Ras at the plasma membrane. It is suggested that SPRED2 and SPRED3 proteins can partially compensate the loss of SPRED1 function (Simanshu et al., 2017). Although SPRED1 binding does not affect neurofibromin’s GAP activity, it nevertheless plays an important role in enabling neurofibromin to downregulate Ras activity at the plasma membrane.
CFCS is a rare autosomal dominant disease with multiple congenital anomalies that affects 1/800,000 newborns (Pierpont et al., 2014). It shares many overlapping features with NS (e.g., heart defects, short stature, and facial features) but is additionally characterized by thick scaly skin, delayed growth and cardiac malformations (Rauen, 2007). Affected individuals frequently have severe neurological disturbances and mental retardation (Yoon et al., 2007). CFCS is caused by heterozygous mutations in BRAF (75% of cases), and less frequently in the MAP2K1 (MEK1), MAP2K2 (MEK2) (20% of cases) and k-ras genes (Rodriguez-Viciana et al., 2006). BRAF is a downstream effector of Ras, and activating BRAF can increase the activation of the MAPK pathway by CRAF (Heidorn et al., 2010).
Costello syndrome is a multiple congenital anomaly syndrome caused by heterozygous activating germline mutations in HRAS. A common and distinctive feature of CS among RASopathies is an increased risk of developing cancers such as rhabdomyosarcomas and neuroblastomas. A recent study has found an increased energy expenditure (EE) in patients with CS, resulting in growth failure (Leoni et al., 2016).
CM-AVM is associated with arteriovenous malformations and fistulas, and is caused by heterozygous inactivating mutations in RASA1 (Eerola et al., 2003). RASA1 encodes p120-RasGAP, which is a negative regulator of the RAS/MAPK signal transduction pathway. RASA1 mutations have also been associated with the related condition known as Parkes Weber syndrome (Banzic et al., 2017).
From the functional perspective, the global relationship for different syndromes could emerged as this: NS and NS-ML are mainly related with activators of the RAS/MAPK cascade (i.e., RAS or RAF activators), but NF and LS are associated to RAS inhibitors. In addition, CS and CFCS mutations hit the backbone of the pathway, while CS being centered on RAS and CFCS on downstream kinases (Atay and Skotheim, 2017).
Future prospective
With the continuous investigation and further understanding of causal mutations and functional analysis of pathophysiological consequences of RASopathies, tremendous advances have been made in the past 30 years. Whereas, given the current fragmentary view, the complexity of RASopathies determined that more issues and challenges lie ahead, such as unidentified causal genes in patients with RASopathies, further functional analyses of the newly discovered mutations, the precise mechanisms underlying the RASopathies (similarities and differences between RASopathies), and the variable expression of a gene mutation (Atay and Skotheim, 2017). In addition, we should take into account of the endocrine and metabolic prospective to interrogate the interactions and contributions of different mutations to the homeostasis imbalance and global phenotype. Moreover, additional factors, such as environmental, age-related and sex-related modifiers, may multifold the difficulty to decipher its pathophysiological process.
Neurological and Psychiatric Disorders
Alzheimer’s disease (AD) is the most common progressive neurodegenerative disorder, affecting more than 30 million people worldwide (Stornetta and Zhu, 2011). The disorder is characterized by early deficits in learning and memory followed by loss of other higher cognitive functions, which is correlated with synaptic depression and then neuronal degeneration (Haass and Selkoe, 2007). It is still unclear that what factors determined the age of onset and how the selective dysfunction of neurons in the brain been affected. A hallmark of AD pathology is the generation of amyloid beta (Aβ) from the amyloid precursor protein (APP) by APP-cleaving enzyme 1 (β-secretase, BACE1) (Schoneborn et al., 2018). Studies showed the underlying mechanism for physiological regulating BACE1 stability and activity in its GTP-bound state was Rheb GTPase, which induced mammalian target of rapamycin (mTOR) activity. Protein levels of BACE1 and Aβ generation are suppressed upon Rheb overexpression (Schoneborn et al., 2018). The interaction of GTP-activated Rheb with BACE1 stimulates its degradation via the proteasomal and lysosomal pathways (Shahani et al., 2014). Recently correlation study implied that the nutrient signaling might regulate cognitive functions in mammals by regulating Rheb–BACE1 and Rheb–mTOR pathways activity, which also orchestrated a potential new therapeutic target for Alzheimer’s-associated memory dysfunction (Shahani et al., 2017).
Parkinson’s disease (PD) is the second most common neurodegenerative disorder following Alzheimer’s disease, affecting 1–2% of the population above 60 years of age (Karimi-Moghadam et al., 2018). Although most PD cases occur sporadically, mutations in several genes, such as SNCA (α-synuclein), PARK2 (parkin), DJ-1, PINK1, ATP13A2, VPS35 (vacuolar protein sorting 35), EIF4G1 (eukaryotic initiation factor 4G1) and LRRK2 (leucine-rich repeat kinase 2), have been identified in hereditary PD (Tsika and Moore, 2013). LRRK2 is a large, multifunctional protein with a central catalytic GTPase/kinase core flanked by several protein-binding domains. Seven missense mutations, clustered in the Ras-of-complex (ROC) GTPase domain, C-terminal-of-ROC (COR) and kinase domains, segregate with PD in affected families (Atashrazm et al., 2018; Pfeffer, 2018). Dopaminergic signaling also plays a critical role in the pathogenesis of PD, and dopamine transporter (DAT) serves as a primary mechanism for terminating dopaminergic signaling (Shi et al., 2013). Rit2 (Rin) was recently identified as a protein that interacts with the DAT C-terminal endocytic domain, implying a role of Rin signaling in the regulation of DAT trafficking (Navaroli et al., 2011). In addition, studies from the perspective of immunity have also indicated that Rit2 polymorphisms affect the innate immune system, which in turn is responsible for some PD symptoms (Chan et al., 2016). Recent genome wide association study (GWAS) and meta-analysis results introduced Rit2 as a novel susceptibility locus, in association with decreased Rin expression in the substantia nigra pars compacta (SNc) of PD patients (Bossers et al., 2009; Latourelle et al., 2012; Pankratz et al., 2012). However, the results of RIT2 polymorphisms studies in different populations are controversial, which may be due to genetic context difference and environmental factors (Daneshmandpour et al., 2018). Therefore, further functional study, animal study and larger study with various population samples might give more detailed role of RIT2 in cells and groups in PD pathogenesis.
Huntington’s disease (HD) is a fatal autosomal-dominant neurodegenerative disease caused by CAG repeat expansion in exon 1 of huntingtin, which encodes the protein huntingtin (Htt). HD results in early loss of medium spiny neurons in the striatum, which impairs motor and cognitive functions (MacDonald et al., 1993). In HD, Htt contains an expanded poly-glutamine (poly-Q) tract. Under healthy conditions, Htt promotes signaling through mTORC1 (mammalian target of rapamycin complex 1). In the case of HD, the poly-Q tract potentiates the signaling by promoting the formation of a ternary complex of Htt-Rheb-mTOR, leading to enhanced mTORC1 activity (Sathasivam et al., 2013). In the striatum, Rhes (Ras homolog enriched in the striatum) serves as a key activator of mTORC1 (Pryor et al., 2014). Knockout of Rhes reduces mTORC1 activity and attenuates the adverse responses of L-DOPA-induced dyskinesia (Subramaniam et al., 2011; Lee et al., 2015). In addition, Rhes facilitates SUMOylation, a process implicated in HD pathogenesis (Subramaniam et al., 2009). Because Rhes is highly expressed in the striatum (Spano et al., 2004), it has been proposed that Rhes-Mutant Htt interactions may underlie the prominent striatal degeneration observed in HD. There is evidence that impaired Rhes/mTORC1 activity is relevant to the notable striatal pathogenesis in HD, which suggests that impaired mTORC1 function may represent a fundamental mechanism underlying the complex disease phenotypes of HD (Lee et al., 2015).
Autism spectrum disorders (ASD) are a continuum of complex neurological disorders interfering with normal social behavior and cognitive development (Hamedani et al., 2017). They are common neurodevelopmental diseases with onset prior to the age of three, affecting almost 1% of individuals worldwide (Liu et al., 2016). Children with ASD often show a large head circumference and develop epilepsy, and nearly half display severe intellectual disability (Stornetta and Zhu, 2011). ASD has a strong but complex genetic component. A recent study identified an increase in RIT1 transcript levels in patients with autism, implying that Rit may represent an ASD susceptibility gene (Garbett et al., 2008). Considering that several factors, such as abnormal assembly of synapses and dendritic spines, contribute to autism’s pathogenesis, the Rit signal pathway that regulates synaptic development and function might be a novel potential therapeutic target (Ebert and Greenberg, 2013).
Schizophrenia is a devastating psychiatric disorder characterized by reality distortion, with onset in late adolescence and unclear etiology (Glessner et al., 2010). Common features are positive symptoms, such as hallucinations, delusions, disorganized speech, and negative symptoms, such as social deficits, lack of motivation, anhedonia, and impaired emotion processing, as well as cognitive deficits with occupational dysfunction (Arajarvi et al., 2005). Previous studies have reported various copy number variations (CNVs) related to schizophrenia (Piluso et al., 2017; Zhuo et al., 2017). Several recent studies have suggested that the rit2 gene might be involved in the pathogenesis of schizophrenia (Glessner et al., 2010). When compared with control subjects, patients with schizophrenia present a significant enrichment of common interstitial deletions (Bouquillon et al., 2011). Among the deleted genes, rit2 was identified as a candidate gene for language delay, mental retardation, and behavioral abnormalities (Bouquillon et al., 2011). Evidence indicates that ret and rit2, both Ras-related genes important for neural crest development, are significantly affected by CNVs (Glessner et al., 2010). Rit2 is involved in the ubiquitin E3 ligase growth factor pathway that affects mitochondrial dysfunction, which is linked with both schizophrenia and autism (Prabakaran et al., 2004).
The protein interactions in the cells are common. Malfunction or alterations in the protein-protein interaction may influence vast biological functions. Selected members of Ras subfamily and their related possible interacting proteins are listed in the Table 2. Specifically for RIT2, evidence suggests that proteins interacting with RIT2 may cause or relate to similar clinical signs or diseases, which implied that RIT2 might be a potential candidate gene underlying several neurological diseases, such as PD, schizophrenia and autism (Daneshmandpour et al., 2018).
Considering the new insight of the role of members of Ras subfamily in neuro- psychiatric disorders and metabolic regulation, substantial novel therapies might be developed or repurposed based on the extensive studies of Ras subfamily signaling in the context of cancer. For example, evidence suggests trametinib, a small molecule inhibitor targets the MEK kinase with high specificity, extends lifespan in Drosophila and protects against the malfunction of genetic induced obesity in mice (Slack et al., 2015).
The Rho Subfamily in Non-Neoplastic Cerebral Diseases
In the mid-1980s, Madaule and Axel (1985) serendipitously identified the first Ras homolog in Aplysia sea slugs, and named it Rho. In recent decades, the Rho subfamily of small GTPases has been shown to regulate many aspects of basic cellular processes such as cell polarity, cell movement, cell–cell interaction, cell proliferation and differentiation, cell morphology, secretion, adhesion, gene expression and survival (Watabe-Uchida et al., 2006; Boureux et al., 2007; Cingolani and Goda, 2008; Peru Y Colón de Portugal et al., 2012; Goitre et al., 2014). More than 20 Rho members, which are structured into six subclasses, are found in all eukaryotic cells as a molecular switch for actin cytoskeleton reorganization (Hall, 2012; Narumiya and Thumkeo, 2018). The Rho subfamily of small GTPases plays essential roles from changes in intracellular cytoskeleton dynamics to extracellular message exchange (Zamboni et al., 2018). In particular, Rho GTPases regulate dendritic arborization, spine morphogenesis, growth cone development, and axon guidance (Stankiewicz and Linseman, 2014). The Rho subclass, including RhoA, RhoB, and RhoC, predominantly promotes the formation of actin stress fibers and focal adhesion (Aspenstrom et al., 2004; Fernandez-Sauze et al., 2009). The Rac subclass (Rac1, Rac2, Rac3, and RhoG) promotes actin chain formation during the branching of lamellipodia, and the cell division cycle. 42 GTP-binding protein (Cdc42) subclass (Cdc42, TC10/RhoQ and TCL/RhoJ) mainly controls the formation of actin microspikes and filopodia (Azzarelli et al., 2014; Goitre et al., 2014).
Rho signaling is involved in several cerebral diseases, including intellectual disability (ID), epilepsy, drug addiction, HD, amyotrophic lateral sclerosis (ALS), and AD, and it acts by regulating axonogenesis, neuronal migration and synaptic plasticity (Table 3) (Mendoza-Naranjo et al., 2007; Locke et al., 2009; Zhang Y. et al., 2015; Li et al., 2016; Wang et al., 2017; Zimering, 2018; Costain et al., 2019). Rac1 and Rac3 are associated with intelligence by regulating key cellular functions in the central nervous system (Reijnders et al., 2017; Costain et al., 2019). Moreover, it was confirmed that missense mutations in Rac3 cause severe intellectual disability and brain malformations in humans (Costain et al., 2019). Previous studies have shown that X-linked ID is related to mutations in genes that code for regulators of the small-GTPase family such as Rac/Cdc42 guanine nucleotide exchange factor 6 (αPIX), RhoGEF and trio Rho guanine nucleotide exchange factor (TRIO) (Lelieveld et al., 2016; Reijnders et al., 2017; Zamboni et al., 2018).
Rac1 is essential for diverse forms of learning, and contributes to extinction of an established memory during drug withdrawal or alcohol use disorders (Peru Y Colón de Portugal et al., 2012; Wang et al., 2017). A recent study provided evidence that Rac1-dependent GABA-A receptor endocytosis plays a crucial role in the extinction of aversive memories (Wang et al., 2017). Furthermore, Rho signaling is involved in regulating neuronal synaptic plasticity in the nucleus accumbens (NAc) and ventral tegmental area (VTA), which both play crucial roles in the reward circuitry (Deguchi et al., 2016). These actions of Rho-associated kinase (ROCK) signaling might also be related to synaptic plasticity in the lateral amygdala and prelimbic prefrontal cortex, which were also identified as regulators in reward circuits (Lamprecht et al., 2002; Swanson et al., 2017). Furthermore, these studies have shown that Rac1 plays a role in drug addiction by regulating synaptic plasticity and the neuronal projection network. It has also been observed that cocaine reduces the phosphorylation of Ezrin/Radixin/Moesin proteins (ERM) in the NAc by downregulating RhoA-Rho kinase signaling, which may importantly contribute to the initiation of synaptic changes in this site, leading to drug addiction (Kim et al., 2009).
Cdc42 is a small GTPase of the Rho-subfamily that acts as a multifaceted key regulator of neuronal structure and function (Bustelo et al., 2007; Govek et al., 2011; Zhang Y. et al., 2015). Cdc42 plays an important role in regulating epileptic seizures (Zhang Y. et al., 2015). Cdc42 regulates the availability of presynaptic sites for CaV2.1 calcium channel incorporation (Chen et al., 2003; Frank et al., 2009), and presynaptic activation of Cdc42 can mimic the effects of electrical activity that promotes synaptic maturation and plasticity (Shen et al., 2006). Cdc42 is over-expressed in the human cortex of the temporal lobe and in the hippocampus of intractable epilepsy patients after an anterior temporal lobectomy (Xiao et al., 2007). Zhang Y. et al. (2015) demonstrated the effect of Cdc42 on the function of hippocampal CA1 pyramidal neurons and revealed that blocking Cdc42 decreases the spontaneous action potentials (APs), and increases both the miniature inhibitory postsynaptic current (mIPSC) and evoked inhibitory postsynaptic current (eIPSC) (Zhang Y. et al., 2015).
Although Rho subfamily has gained the most attention for its putative role in numerous neurodegenerative diseases, the precise mechanisms as a therapeutic target remain controversial and uncertain. In PD, Rho signaling pathway is a promising therapeutic target (Labandeira-Garcia et al., 2015). Some studies also suggest that mutations in LRRK2 can lead to a decrease in activation of Rac1 related Rho signaling, which causes disassembly of actin filaments leading to modulate cytoskeletal outgrowth and vesicular dynamics, including autophagy (Boon et al., 2014). These functions likely impact modulation of α-synuclein aggregation and associated toxicity in the pathophysiology of PD (Konno et al., 2012). A previous study revealed that ROCK inhibition protects against neuronal death induced by neurotoxins (Borrajo et al., 2014). ROCK regulation may provide a new neuroprotective strategy for neurodegenerative diseases, and it has to be settled urgently to develop more potent and selective ROCK regulators.
The Rab Subfamily in Non-Neoplastic Cerebral Diseases
The Rab subfamily, which comprises more than 60 members, is currently the largest branch of the Ras superfamily of small GTPases (Wennerberg et al., 2005; Diekmann et al., 2011). This protein family is primarily related to various aspects of membrane and protein traffic in the endocytic and secretory pathways (Wennerberg et al., 2005; Cherfils and Zeghouf, 2013; Goitre et al., 2014; Gao et al., 2018). Therefore, dysregulation of Rab GTPases may lead to the pathogenesis of some diseases (Table 3). Many neurodegenerative diseases are characterized by dysfunction of membrane and protein traffic in neurons (Zhang X. et al., 2018). The Rab subfamily has also been related to neurodegenerative disorders such as Alzheimer’s disease and PD (Wilson et al., 2014; Saridaki et al., 2018; Yan et al., 2018). Previous studies have shown that Rab3A, Rab6, Rab8A/Rab8, Rab23, and Rab27b are highly expressed in the brain and participate in synaptic vesicle exocytosis, postsynaptic glutamate receptor dynamics, neurite growth and neural development (Takamori et al., 2006; Ng and Tang, 2008; Uno et al., 2016). Rab3 is the most abundant Rab protein in the brain. It is localized in synaptic vesicles and participates in their fusion and neurotransmitter release (Ng and Tang, 2008; Shin, 2014).
Alzheimer’s disease is clinically characterized by progressive cognitive impairment and memory loss (Bejanin et al., 2017). Two classical pathological features of AD are aberrant phosphorylated forms of tau protein and pathologically generated Aβ peptides (Lee et al., 2001; Alavi Naini and Soussi-Yanicostas, 2015; Bejanin et al., 2017; Bourdenx et al., 2017; Sun et al., 2018). Rab GTPases are implicated in multiple pathological mechanisms, including Aβ production and accumulation in AD (Li, 2011; Ridge et al., 2017; Rodriguez et al., 2017; Yan et al., 2018). Rab1B was found to play a key role in APP trafficking from the ER to the Golgi (Dugan et al., 1995). A study has shown that downregulation of Rab1B blocked APP transport in the ER/Golgi and significantly inhibited Aβ secretion (Dugan et al., 1995). Upregulation of either the early endosome protein Rab5 or the late endosome protein Rab7 increased Aβ trafficking from the cytoplasm to the lysosomes (Li et al., 2012). Previous post mortem studies have shown that the levels of Rab7A are elevated in the brains of AD model mice. Furthermore, some recent studies have shown that Rab7A may regulate tau secretion and tangle propagation in AD (Rodriguez et al., 2017; Zafar et al., 2017). Moreover, Rab11A-positive recycling vesicles accelerated cellular Aβ accumulation (Li et al., 2012).
A large amount of literature has reported the importance of the interaction between Rab GTPases and LRRK2 in PD (Steger et al., 2016; Alessi and Sammler, 2018; Eguchi et al., 2018; Jeong et al., 2018; Madero-Perez et al., 2018; Mir et al., 2018; Pfeffer, 2018). It has been reported that autosomal dominant missense mutations within the LRRK2 gene account for 1–2% of all cases of PD (Paisan-Ruiz et al., 2013). Similarly, variations at the LRRK2 locus also mildly increase the risk for idiopathic PD (Paisan-Ruiz et al., 2013; Domingo and Klein, 2018). A previous study verified that LRRK2 phosphorylates a subgroup of Rab GTPases which includes Rab7, Rab8A, and Rab10, and plays a crucial role in membrane and protein traffic (Gomez-Suaga et al., 2014; Roosen and Cookson, 2016; Steger et al., 2016, 2017). Consistently with the importance of LRRK2 for PD, Rab subfamily members can be seen as regulators of membrane trafficking. Furthermore, Rab phosphorylation was found to be altered in vivo in all the related pathogenic processes (Steger et al., 2016, 2017). Some reports showed that Rab35 and Rab39B may be implied in the pathogenesis of PD (Chiu et al., 2016; Lin et al., 2017). There is increasing evidence that mutations in Rab29 or Rab39B are related to the impairment of membrane trafficking relevant for PD (Ben-David and Tu, 2015; Domingo and Klein, 2018; Purlyte et al., 2018).
Hearing loss often results in plastic changes in the central auditory pathways, which are also related to members of the Rab family of small GTPase (Dong et al., 2010; Mulders et al., 2014). Gene expression of Rab3A and Rab3GAP1 fas found to be decreased in the paraflocculus after acoustic or mechanical cochlear trauma (Dong et al., 2010; Mulders et al., 2014). Moreover, early modulation of Rab GTPase gene expression in the paraflocculus may affect auditory processing by regulating the release of neurotransmitters (Mulders et al., 2014).
Cone-rod dystrophy (CRD) is an inherited retinal dystrophy that belongs to the group of pigmentary retinopathies. It is characterized by primary loss of cone photoreceptors and subsequent or simultaneous loss of rod photoreceptors (Hamel, 2007; Roosing et al., 2013). A previous study has shown that mutations in Rab28 are associated with autosomal-recessive cone-rod dystrophy (Roosing et al., 2013). Rab28 is located in the basal body and ciliary rootlet, where it plays a crucial role in ciliary transport. Other studies have shown that primary ciliogenesis is associated Rab3A, Rab6A, Rab8, and Rab11, which play an indirect role in rhodopsin transport from the photoreceptors’ inner to the outer segments through the connecting cilium (Moritz et al., 2001; Satoh et al., 2005; Knodler et al., 2010).
The Arf Subfamily in Non-Neoplastic Cerebral Diseases
Arf GTPases are a subfamily of proteins in the Ras superfamily that were identified as cargo displacement factors (Ismail et al., 2011). The roles of Arf GTPases include protein trafficking, lipid metabolism and trafficking, as well as actin remodeling in eukaryotic cells via their regulated GTP cycle (D’souza-Schorey and Chavrier, 2006; Jackson and Bouvet, 2014). According to sequence homology, there are four main classes of Arf proteins in mammals, namely Class I (Arf1–3), Class II (Arf4–5), Class III (Arf6), and unclassified members (Rojas et al., 2012; Jackson and Bouvet, 2014). Arf1 is the first member of this small GTPase subfamily, which is viewed as key regulators of eukaryotic cell organization (Kahn and Gilman, 1986; Jackson and Bouvet, 2014). Mutations in Arf1 have been shown to be related to autosomal recessive periventricular heterotopia, a disorder that leads to severe malformation of the cerebral cortex (Sheen et al., 2004).
In the last two decades, a series of cerebral diseases associated with Arf GTPases have been studied (Table 3). Previous studies have shown that many Arf-regulated ER–Golgi trafficking processes are defective in ALS (Saxena et al., 2009; Wang et al., 2011; Zhai et al., 2015; Atkin et al., 2017). Moreover, a study has shown that regulation of Arf signaling reverses mutant protein toxicity in ALS by decreasing ER stress and stimulating various types of autophagy in cell lines and animal models (Zhai et al., 2015). Recent studies have shown that Arl proteins which belong to Arf GTPases are associated with retinitis pigmentosa (Veltel et al., 2008a; Zhang H. et al., 2015; Hanke-Gogokhia et al., 2016b). In the pathogenetic process of retinitis pigmentosa, Arl2/Arl3 signaling plays important roles in photoreceptor function by regulating lipid-modified membrane-associated proteins (Hanke-Gogokhia et al., 2016b). The formation of a ternary complex between Arl3, its cognate GAP RP2 and its retinal effector HRG4 is also of great importance for photoreceptor function (Veltel et al., 2008b). In a rodent model, regulating the activities of Arl3 GAP can reduce the severity of photoreceptor disease (Zhang H. et al., 2015).
The Ran Subfamily and Unclassified Ras Superfamily Members in Non-Neoplastic Cerebral Diseases
Ran (Ras-related Nuclear protein) is generally encoded by a single ortholog in eukaryotes (Reiner and Lundquist, 2018). The classic function of Ran is to regulate the cycle of nuclear import and export (Reiner and Lundquist, 2018). There are few literature reports on the relationship between Ran and cerebral diseases (Table 3). A study indicated that the expression of Ran is reduced in AD, and Ran is a pivotal molecule in nucleocytoplasmic transport in AD pathophysiology (Mastroeni et al., 2013).
Ran also plays crucial roles in frontotemporal lobe degeneration (FTLD) by regulating TDP-43 induced retinal neurodegeneration (Ward et al., 2014). FTLD comprises a group of disorders, and is clinically characterized by behavioral and personality changes, language impairment, as well as deficits in executive functioning, and is pathologically associated with degeneration of frontal and temporal lobes (Morris et al., 2012; Pottier et al., 2016). The expression of Ran is found to be reduced by nuclear depletion of TDP-43 in a Grn-KO induced rodent model of retinal neuronal loss (Ward et al., 2014). Retinal neurodegeneration as a new phenotype involves the reciprocal loss of Ran in progranulin-deficient FTLD via an underlying mechanism related to nuclear TDP-43 (Ward et al., 2014). There are also some unclassified members in the Ras superfamily of small GTPases (Rojas et al., 2012), and these also play vital roles in cerebral diseases. Gispert et al. (2015) studied synucleinopathy-induced expression changes in the mouse brain and identified 49 midbrain/brainstem-specific transcriptional dysregulations, including Rabl2A downregulation. Mitochondrial Rho GTPase 1 (MIRO1), which is encoded by the rhoT1 gene (Fransson et al., 2003; Tada et al., 2016; Lahiri and Klionsky, 2017), is involved in mitochondrial homeostasis and apoptosis, as well as PD and cancer (Anvret et al., 2012; Jiang et al., 2012; Schwarz, 2013; Sheng, 2014; Van Der Merwe et al., 2015; Lahiri and Klionsky, 2017). A recent study showed that MIRO1 is a potential adaptor for microtubule based peroxisome motility in mammalian cells (Castro et al., 2018). Another study has revealed that Rab20 is related to a genetic mechanism of longitudinal cognitive changes during the transition period from mild cognitive impairment to AD (Lee et al., 2017). Liang et al. (2012) found that Rab20 is substantially upregulated during the acute phase of brain inflammation, and also plays crucial roles in the subsequent inflammatory responses in the brain.
Conclusion
Knowledge about the roles of the Ras superfamily of small GTPases in cerebral diseases has considerably grown in the past 30 years. Currently, many research teams all over the world are also trying to identify novel small GTPases, which can be viewed as new regulators and effectors that control the crucial structure and biological functions affected by cerebral diseases. In particular, these important small GTPases have improved the development and applications of cellular and animal disease models. In this review, we mainly discussed how Ras-superfamily GTPases contribute to a range of human cerebral diseases as specific effectors in a series of complex mechanisms. Finally, a helpful summary of all described small GTPases from the Ras superfamily that play a role in cerebral diseases is shown schematically in Tables 1–3. Therefore, seeking treatment solutions for the related diseases by identifying the as-yet unknown physiological or pathological functions of more than 167 Ras superfamily members will open new research directions and fields in the following decades.
Author Contributions
X-LW and YW designed the study. LQ and CP collected, analyzed data, and wrote the manuscript. S-MH and BL interpreted the data and revised the manuscript. YW edited and polished the manuscript. G-DG and X-LW finalized the manuscript. All authors critically reviewed content and approved final version for publication.
Funding
This work was supported by the National Natural Science Foundation of China (Grant Number 81671366, awarded to X-LW and Grant Number 81572470, awarded to S-MH), the National Science Foundation of China (Grant Number 81601100, awarded to YW), and Science and Technology Innovation Foundation of Tangdu Hospital, Air Force Military Medical University (Grant Number 2017LCYJ002, awarded to X-LW).
Conflict of Interest Statement
The authors declare that the research was conducted in the absence of any commercial or financial relationships that could be construed as a potential conflict of interest.
Acknowledgments
Special thanks to Dr. Bolin Liu and Dr. Lei Chen for their contributions in the management and coordination of the study. Dr. Yafei Xue, Dr. Tao Zheng, and Dr. Lanfu Zhao have make great effort to the literature service in this study.
Supplementary Material
The Supplementary Material for this article can be found online at: https://www.frontiersin.org/articles/10.3389/fnmol.2019.00121/full#supplementary-material
TABLE S1 | G-domin regions of 167 small GTPases found in humans.
References
Ahearn, I. M., Haigis, K., Bar-Sagi, D., and Philips, M. R. (2011). Regulating the regulator: post-translational modification of RAS. Nat. Rev. Mol. Cell Biol. 13, 39–51. doi: 10.1038/nrm3255
Alavi Naini, S. M., and Soussi-Yanicostas, N. (2015). Tau hyperphosphorylation and oxidative stress, a critical vicious circle in neurodegenerative tauopathies? Oxid. Med. Cell. Longev. 2015:151979.
Anvret, A., Ran, C., Westerlund, M., Sydow, O., Willows, T., Olson, L., et al. (2012). Genetic screening of the mitochondrial Rho GTPases MIRO1 and MIRO2 in Parkinson’s disease. Open Neurol. J. 6, 1–5. doi: 10.2174/1874205x01206010001
Aoki, Y., Niihori, T., Banjo, T., Okamoto, N., Mizuno, S., Kurosawa, K., et al. (2013). Gain-of-function mutations in RIT1 cause Noonan syndrome, a RAS/MAPK pathway syndrome. Am. J. Hum. Genet. 93, 173–180. doi: 10.1016/j.ajhg.2013.05.021
Arajarvi, R., Suvisaari, J., Suokas, J., Schreck, M., Haukka, J., Hintikka, J., et al. (2005). Prevalence and diagnosis of schizophrenia based on register, case record and interview data in an isolated Finnish birth cohort born 1940-1969. Soc. Psychiatry Psychiatr. Epidemiol. 40, 808–816. doi: 10.1007/s00127-005-0951-9
Arun, V., Wiley, J. C., Kaur, H., Kaplan, D. R., and Guha, A. (2013). A novel neurofibromin (NF1) interaction with the leucine-rich pentatricopeptide repeat motif-containing protein links neurofibromatosis type 1 and the French Canadian variant of Leigh’s syndrome in a common molecular complex. J. Neurosci. Res. 91, 494–505. doi: 10.1002/jnr.23189
Aspenstrom, P., Fransson, A., and Saras, J. (2004). Rho GTPases have diverse effects on the organization of the actin filament system. Biochem. J. 377, 327–337. doi: 10.1042/bj20031041
Atashrazm, F., Hammond, D., Perera, G., Bolliger, M. F., Matar, E., Halliday, G. M., et al. (2018). LRRK2-mediated Rab10 phosphorylation in immune cells from Parkinson’s disease patients. Mov. Disord. 34, 406–415.
Atay, O., and Skotheim, J. M. (2017). Spatial and temporal signal processing and decision making by MAPK pathways. J. Cell Biol. 216, 317–330. doi: 10.1083/jcb.201609124
Atkin, J. D., Farg, M. A., Turner, B. J., Tomas, D., Lysaght, J. A., Nunan, J., et al. (2017). Induction of the unfolded protein response in familial amyotrophic lateral sclerosis and association of protein-disulfide isomerase with superoxide dismutase 1. J. Biol. Chem. 292:12007. doi: 10.1074/jbc.a117.603393
Azzarelli, R., Kerloch, T., and Pacary, E. (2014). Regulation of cerebral cortex development by Rho GTPases: insights from in vivo studies. Front. Cell. Neurosci. 8:445. doi: 10.3389/fncel.2014.00445
Banzic, I., Brankovic, M., Maksimovic, Z., Davidovic, L., Markovic, M., and Rancic, Z. (2017). Parkes Weber syndrome-Diagnostic and management paradigms: a systematic review. Phlebology 32, 371–383. doi: 10.1177/0268355516664212
Bejanin, A., Schonhaut, D. R., La Joie, R., Kramer, J. H., Baker, S. L., Sosa, N., et al. (2017). Tau pathology and neurodegeneration contribute to cognitive impairment in Alzheimer’s disease. Brain 140, 3286–3300.
Ben-David, E., and Tu, R. (2015). Genetics of Parkinson disease. AJNR Am. J. Neuroradiol. 36, 445–447.
Bertola, D., Buscarilli, M., Stabley, D. L., Baker, L., Doyle, D., Bartholomew, D. W., et al. (2017). Phenotypic spectrum of Costello syndrome individuals harboring the rare HRAS mutation p.Gly13Asp. Am. J. Med. Genet. A 173, 1309–1318. doi: 10.1002/ajmg.a.38178
Besga, A., Chyzhyk, D., Gonzalez-Ortega, I., Echeveste, J., Grana-Lecuona, M., Grana, M., et al. (2017). White matter tract integrity in Alzheimer’s disease vs. late onset bipolar disorder and its correlation with systemic inflammation and oxidative stress biomarkers. Front. Aging Neurosci. 9:179. doi: 10.3389/fnagi.2017.00179
Bongmba, O. Y. N., Martinez, L. A., Elhardt, M. E., Butler, K., and Tejada-Simon, M. V. (2011). Modulation of dendritic spines and synaptic function by Rac1: a possible link to Fragile X syndrome pathology. Brain Res. 1399, 79–95. doi: 10.1016/j.brainres.2011.05.020
Boon, J. Y., Dusonchet, J., Trengrove, C., and Wolozin, B. (2014). Interaction of LRRK2 with kinase and GTPase signaling cascades. Front. Mol. Neurosci. 7:64. doi: 10.3389/fnmol.2014.00064
Borrajo, A., Rodriguez-Perez, A. I., Villar-Cheda, B., Guerra, M. J., and Labandeira-Garcia, J. L. (2014). Inhibition of the microglial response is essential for the neuroprotective effects of Rho-kinase inhibitors on MPTP-induced dopaminergic cell death. Neuropharmacology 85, 1–8. doi: 10.1016/j.neuropharm.2014.05.021
Bossers, K., Meerhoff, G., Balesar, R., Van Dongen, J. W., Kruse, C. G., Swaab, D. F., et al. (2009). Analysis of gene expression in Parkinson’s disease: possible involvement of neurotrophic support and axon guidance in dopaminergic cell death. Brain Pathol. 19, 91–107. doi: 10.1111/j.1750-3639.2008.00171.x
Bouquillon, S., Andrieux, J., Landais, E., Duban-Bedu, B., Boidein, F., Lenne, B., et al. (2011). A 5.3Mb deletion in chromosome 18q12.3 as the smallest region of overlap in two patients with expressive speech delay. Eur. J. Med. Genet. 54, 194–197. doi: 10.1016/j.ejmg.2010.11.009
Bourdenx, M., Koulakiotis, N. S., Sanoudou, D., Bezard, E., Dehay, B., and Tsarbopoulos, A. (2017). Protein aggregation and neurodegeneration in prototypical neurodegenerative diseases: examples of amyloidopathies, tauopathies and synucleinopathies. Prog. Neurobiol. 155, 171–193. doi: 10.1016/j.pneurobio.2015.07.003
Boureux, A., Vignal, E., Faure, S., and Fort, P. (2007). Evolution of the Rho family of Ras-like GTPases in eukaryotes. Mol. Biol. Evol. 24, 203–216. doi: 10.1093/molbev/msl145
Bourne, H. R., Sanders, D. A., and Mccormick, F. (1991). The GTPase superfamily: conserved structure and molecular mechanism. Nature 349, 117–127. doi: 10.1038/349117a0
Brems, H., Chmara, M., Sahbatou, M., Denayer, E., Taniguchi, K., Kato, R., et al. (2007). Germline loss-of-function mutations in SPRED1 cause a neurofibromatosis 1-like phenotype. Nat. Genet. 39, 1120–1126. doi: 10.1038/ng2113
Buday, L., and Downward, J. (2008). Many faces of Ras activation. Biochim. Biophys. Acta 1786, 178–187. doi: 10.1016/j.bbcan.2008.05.001
Buggia-Prevot, V., Fernandez, C. G., Riordan, S., Vetrivel, K. S., Roseman, J., Waters, J., et al. (2014). Axonal BACE1 dynamics and targeting in hippocampal neurons: a role for Rab11 GTPase. Mol. Neurodegener. 9:1. doi: 10.1186/1750-1326-9-1
Bustelo, X. R., Sauzeau, V., and Berenjeno, I. M. (2007). GTP-binding proteins of the Rho/Rac family: regulation, effectors and functions in vivo. Bioessays 29, 356–370. doi: 10.1002/bies.20558
Caliskan, M., Chong, J. X., Uricchio, L., Anderson, R., Chen, P., Sougnez, C., et al. (2011). Exome sequencing reveals a novel mutation for autosomal recessive non-syndromic mental retardation in the TECR gene on chromosome 19p13. Hum. Mol. Genet. 20, 1285–1289. doi: 10.1093/hmg/ddq569
Castro, I. G., Richards, D. M., Metz, J., Costello, J. L., Passmore, J. B., Schrader, T. A., et al. (2018). A role for Mitochondrial Rho GTPase 1 (MIRO1) in motility and membrane dynamics of peroxisomes. Traffic 19, 229–242. doi: 10.1111/tra.12549
Cawthon, R. M., Weiss, R., Xu, G. F., Viskochil, D., Culver, M., Stevens, J., et al. (1990). A major segment of the neurofibromatosis type 1 gene: cDNA sequence, genomic structure, and point mutations. Cell 62, 193–201. doi: 10.1016/0092-8674(90)90253-b
Cesarini, L., Alfieri, P., Pantaleoni, F., Vasta, I., Cerutti, M., Petrangeli, V., et al. (2009). Cognitive profile of disorders associated with dysregulation of the RAS/MAPK signaling cascade. Am. J. Med. Genet. A 149A, 140–146. doi: 10.1002/ajmg.a.32488
Chaldakov, G. N., Tonchev, A. B., and Aloe, L. (2009). NGF and BDNF: from nerves to adipose tissue, from neurokines to metabokines. Riv. Psichiatr. 44, 79–87.
Chan, G., White, C. C., Winn, P. A., Cimpean, M., Replogle, J. M., Glick, L. R., et al. (2016). Trans-pQTL study identifies immune crosstalk between Parkinson and Alzheimer loci. Neurol. Genet. 2:e90. doi: 10.1212/nxg.0000000000000090
Chen, N., Furuya, S., Doi, H., Hashimoto, Y., Kudo, Y., and Higashi, H. (2003). Ganglioside/calmodulin kinase II signal inducing cdc42-mediated neuronal actin reorganization. Neuroscience 120, 163–176. doi: 10.1016/s0306-4522(03)00259-8
Chen, X. W., Leto, D., Xiao, J., Goss, J., Wang, Q., Shavit, J. A., et al. (2011). Exocyst function is regulated by effector phosphorylation. Nat. Cell Biol. 13, 580–588. doi: 10.1038/ncb2226
Cherfils, J., and Zeghouf, M. (2013). Regulation of small GTPases by GEFs, GAPs, and GDIs. Physiol. Rev. 93, 269–309. doi: 10.1152/physrev.00003.2012
Chiu, C. C., Yeh, T. H., Lai, S. C., Weng, Y. H., Huang, Y. C., Cheng, Y. C., et al. (2016). Increased Rab35 expression is a potential biomarker and implicated in the pathogenesis of Parkinson’s disease. Oncotarget 7, 54215–54227.
Chong, J. X., Caputo, V., Phelps, I. G., Stella, L., Worgan, L., Dempsey, J. C., et al. (2016). Recessive inactivating mutations in TBCK, encoding a Rab GTPase-activating protein, cause severe infantile syndromic encephalopathy. Am. J. Hum. Genet. 98, 772–781. doi: 10.1016/j.ajhg.2016.01.016
Chutna, O., Goncalves, S., Villar-Pique, A., Guerreiro, P., Marijanovic, Z., Mendes, T., et al. (2014). The small GTPase Rab11 co-localizes with alpha-synuclein in intracellular inclusions and modulates its aggregation, secretion and toxicity. Hum. Mol. Genet. 23, 6732–6745. doi: 10.1093/hmg/ddu391
Cingolani, L. A., and Goda, Y. (2008). Actin in action: the interplay between the actin cytoskeleton and synaptic efficacy. Nat. Rev. Neurosci. 9, 344–356. doi: 10.1038/nrn2373
Claing, A. (2013). beta-Arrestins: modulators of small GTPase activation and function. Prog. Mol. Biol. Transl. Sci. 118, 149–174.
Costain, G., Callewaert, B., Gabriel, H., Tan, T. Y., Walker, S., Christodoulou, J., et al. (2019). De novo missense variants in RAC3 cause a novel neurodevelopmental syndrome. Genet. Med. 21, 1021–1026.
Cox, A. D., and Der, C. J. (2002). Ras family signaling: therapeutic targeting. Cancer Biol. Ther. 1, 599–606. doi: 10.4161/cbt.306
Cox, A. D., and Der, C. J. (2010). Ras history: the saga continues. Small GTPases 1, 2–27. doi: 10.4161/sgtp.1.1.12178
Daneshmandpour, Y., Darvish, H., and Emamalizadeh, B. (2018). RIT2: responsible and susceptible gene for neurological and psychiatric disorders. Mol. Genet. Genomics 293, 785–792. doi: 10.1007/s00438-018-1451-4
Dard, L., Bellance, N., Lacombe, D., and Rossignol, R. (2018). RAS signalling in energy metabolism and rare human diseases. Biochim. Biophys. Acta Bioenerg. 1859, 845–867. doi: 10.1016/j.bbabio.2018.05.003
Das, A., Gajendra, S., Falenta, K., Oudin, M. J., Peschard, P., Feng, S., et al. (2014). RalA promotes a direct exocyst-Par6 interaction to regulate polarity in neuronal development. J. Cell Sci. 127, 686–699. doi: 10.1242/jcs.145037
De Cicco, M., Kiss, L., and Dames, S. A. (2018). NMR analysis of the backbone dynamics of the small GTPase Rheb and its interaction with the regulatory protein FKBP38. FEBS Lett. 592, 130–146. doi: 10.1002/1873-3468.12925
Deguchi, Y., Harada, M., Shinohara, R., Lazarus, M., Cherasse, Y., Urade, Y., et al. (2016). mDia and ROCK mediate actin-dependent presynaptic remodeling regulating synaptic efficacy and anxiety. Cell Rep. 17, 2405–2417. doi: 10.1016/j.celrep.2016.10.088
Dentici, M. L., Sarkozy, A., Pantaleoni, F., Carta, C., Lepri, F., Ferese, R., et al. (2009). Spectrum of MEK1 and MEK2 gene mutations in cardio-facio-cutaneous syndrome and genotype-phenotype correlations. Eur. J. Hum. Genet. 17, 733–740. doi: 10.1038/ejhg.2008.256
Diekmann, Y., Seixas, E., Gouw, M., Tavares-Cadete, F., Seabra, M. C., and Pereira-Leal, J. B. (2011). Thousands of Rab GTPases for the cell biologist. PLoS Comput. Biol. 7:e1002217. doi: 10.1371/journal.pcbi.1002217
Digilio, M. C., Conti, E., Sarkozy, A., Mingarelli, R., Dottorini, T., Marino, B., et al. (2002). Grouping of multiple-lentigines/LEOPARD and Noonan syndromes on the PTPN11 gene. Am. J. Hum. Genet. 71, 389–394. doi: 10.1086/341528
Digilio, M. C., Sarkozy, A., De Zorzi, A., Pacileo, G., Limongelli, G., Mingarelli, R., et al. (2006). LEOPARD syndrome: clinical diagnosis in the first year of life. Am. J. Med. Genet. A 140, 740–746. doi: 10.1002/ajmg.a.31156
Domingo, A., and Klein, C. (2018). Genetics of Parkinson disease. Handb. Clin. Neurol. 147, 211–227.
Dong, S., Mulders, W. H., Rodger, J., Woo, S., and Robertson, D. (2010). Acoustic trauma evokes hyperactivity and changes in gene expression in guinea-pig auditory brainstem. Eur. J. Neurosci. 31, 1616–1628.
D’souza-Schorey, C., and Chavrier, P. (2006). ARF proteins: roles in membrane traffic and beyond. Nat. Rev. Mol. Cell Biol. 7, 347–358. doi: 10.1038/nrm1910
Dugan, J. M., Dewit, C., Mcconlogue, L., and Maltese, W. A. (1995). The Ras-related GTP-binding protein, Rab1B, regulates early steps in exocytic transport and processing of beta-amyloid precursor protein. J. Biol. Chem. 270, 10982–10989. doi: 10.1074/jbc.270.18.10982
Ebert, D. H., and Greenberg, M. E. (2013). Activity-dependent neuronal signalling and autism spectrum disorder. Nature 493, 327–337. doi: 10.1038/nature11860
Eerola, I., Boon, L. M., Mulliken, J. B., Burrows, P. E., Dompmartin, A., Watanabe, S., et al. (2003). Capillary malformation-arteriovenous malformation, a new clinical and genetic disorder caused by RASA1 mutations. Am. J. Hum. Genet. 73, 1240–1249. doi: 10.1086/379793
Eguchi, T., Kuwahara, T., Sakurai, M., Komori, T., Fujimoto, T., Ito, G., et al. (2018). LRRK2 and its substrate Rab GTPases are sequentially targeted onto stressed lysosomes and maintain their homeostasis. Proc. Natl. Acad. Sci. U.S.A. 115, E9115–E9124.
Ekvall, S., Hagenas, L., Allanson, J., Anneren, G., and Bondeson, M. L. (2011). Co-occurring SHOC2 and PTPN11 mutations in a patient with severe/complex Noonan syndrome-like phenotype. Am. J. Med. Genet. A 155A, 1217–1224. doi: 10.1002/ajmg.a.33987
Ekvall, S., Wilbe, M., Dahlgren, J., Legius, E., Van Haeringen, A., Westphal, O., et al. (2015). Mutation in NRAS in familial Noonan syndrome–case report and review of the literature. BMC Med. Genet. 16:95. doi: 10.1186/s12881-015-0239-1
Emamalizadeh, B., Jamshidi, J., Movafagh, A., Ohadi, M., Khaniani, M. S., Kazeminasab, S., et al. (2017). RIT2 polymorphisms: is there a differential association? Mol. Neurobiol. 54, 2234–2240. doi: 10.1007/s12035-016-9815-4
Fan, Y., Esmail, M. A., Ansley, S. J., Blacque, O. E., Boroevich, K., Ross, A. J., et al. (2004). Mutations in a member of the Ras superfamily of small GTP-binding proteins causes Bardet-Biedl syndrome. Nat. Genet. 36, 989–993. doi: 10.1038/ng1414
Fernandez-Sauze, S., Grall, D., Cseh, B., and Van Obberghen-Schilling, E. (2009). Regulation of fibronectin matrix assembly and capillary morphogenesis in endothelial cells by Rho family GTPases. Exp. Cell Res. 315, 2092–2104. doi: 10.1016/j.yexcr.2009.03.017
Flex, E., Jaiswal, M., Pantaleoni, F., Martinelli, S., Strullu, M., Fansa, E. K., et al. (2014). Activating mutations in RRAS underlie a phenotype within the RASopathy spectrum and contribute to leukaemogenesis. Hum. Mol. Genet. 23, 4315–4327. doi: 10.1093/hmg/ddu148
Frank, C. A., Pielage, J., and Davis, G. W. (2009). A presynaptic homeostatic signaling system composed of the Eph receptor, ephexin, Cdc42, and CaV2.1 calcium channels. Neuron 61, 556–569. doi: 10.1016/j.neuron.2008.12.028
Fransson, A., Ruusala, A., and Aspenstrom, P. (2003). Atypical Rho GTPases have roles in mitochondrial homeostasis and apoptosis. J. Biol. Chem. 278, 6495–6502. doi: 10.1074/jbc.m208609200
Gao, Y., Wilson, G. R., Stephenson, S. E. M., Bozaoglu, K., Farrer, M. J., and Lockhart, P. J. (2018). The emerging role of Rab GTPases in the pathogenesis of Parkinson’s disease. Mov. Disord. 33, 196–207. doi: 10.1002/mds.27270
Garbett, K., Ebert, P. J., Mitchell, A., Lintas, C., Manzi, B., Mirnics, K., et al. (2008). Immune transcriptome alterations in the temporal cortex of subjects with autism. Neurobiol. Dis. 30, 303–311. doi: 10.1016/j.nbd.2008.01.012
Garcia-Romero, M. T., Parkin, P., and Lara-Corrales, I. (2016). Mosaic neurofibromatosis type 1: a systematic review. Pediatr. Dermatol. 33, 9–17. doi: 10.1111/pde.12673
Ginsberg, S. D., Alldred, M. J., Counts, S. E., Cataldo, A. M., Neve, R. L., Jiang, Y., et al. (2010a). Microarray analysis of hippocampal CA1 neurons implicates early endosomal dysfunction during Alzheimer’s disease progression. Biol. Psychiatry 68, 885–893. doi: 10.1016/j.biopsych.2010.05.030
Ginsberg, S. D., Mufson, E. J., Counts, S. E., Wuu, J., Alldred, M. J., Nixon, R. A., et al. (2010b). Regional selectivity of rab5 and rab7 protein upregulation in mild cognitive impairment and Alzheimer’s disease. J. Alzheimers Dis. 22, 631–639. doi: 10.3233/jad-2010-101080
Ginsberg, S. D., Mufson, E. J., Alldred, M. J., Counts, S. E., Wuu, J., Nixon, R. A., et al. (2011). Upregulation of select Rab GTPases in cholinergic basal forebrain neurons in mild cognitive impairment and Alzheimer’s disease. J. Chem. Neuroanat. 42, 102–110. doi: 10.1016/j.jchemneu.2011.05.012
Gispert, S., Kurz, A., Brehm, N., Rau, K., Walter, M., Riess, O., et al. (2015). Complexin-1 and Foxp1 expression changes are novel brain effects of alpha-synuclein pathology. Mol. Neurobiol. 52, 57–63. doi: 10.1007/s12035-014-8844-0
Glessner, J. T., Reilly, M. P., Kim, C. E., Takahashi, N., Albano, A., Hou, C., et al. (2010). Strong synaptic transmission impact by copy number variations in schizophrenia. Proc. Natl. Acad. Sci. U.S.A. 107, 10584–10589.
Goitre, L., Trapani, E., Trabalzini, L., and Retta, S. F. (2014). The Ras superfamily of small GTPases: the unlocked secrets. Methods Mol. Biol. 1120, 1–18. doi: 10.1007/978-1-62703-791-4_1
Gomez-Suaga, P., Rivero-Rios, P., Fdez, E., Blanca Ramirez, M., Ferrer, I., Aiastui, A., et al. (2014). LRRK2 delays degradative receptor trafficking by impeding late endosomal budding through decreasing Rab7 activity. Hum. Mol. Genet. 23, 6779–6796. doi: 10.1093/hmg/ddu395
Govek, E. E., Hatten, M. E., and Van Aelst, L. (2011). The role of Rho GTPase proteins in CNS neuronal migration. Dev. Neurobiol. 71, 528–553. doi: 10.1002/dneu.20850
Grewal, S. S., Horgan, A. M., York, R. D., Withers, G. S., Banker, G. A., and Stork, P. J. (2000). Neuronal calcium activates a Rap1 and B-Raf signaling pathway via the cyclic adenosine monophosphate-dependent protein kinase. J. Biol. Chem. 275, 3722–3728. doi: 10.1074/jbc.275.5.3722
Gripp, K. W., Aldinger, K. A., Bennett, J. T., Baker, L., Tusi, J., Powell-Hamilton, N., et al. (2016). A novel rasopathy caused by recurrent de novo missense mutations in PPP1CB closely resembles Noonan syndrome with loose anagen hair. Am. J. Med. Genet. A 170, 2237–2247. doi: 10.1002/ajmg.a.37781
Grosshans, B. L., Ortiz, D., and Novick, P. (2006). Rabs and their effectors: achieving specificity in membrane traffic. Proc. Natl. Acad. Sci. U.S.A. 103, 11821–11827. doi: 10.1073/pnas.0601617103
Guvenc, H., Pavlyukov, M. S., Joshi, K., Kurt, H., Banasavadi-Siddegowda, Y. K., Mao, P., et al. (2013). Impairment of glioma stem cell survival and growth by a novel inhibitor for Survivin-Ran protein complex. Clin. Cancer Res. 19, 631–642. doi: 10.1158/1078-0432.ccr-12-0647
Haass, C., and Selkoe, D. J. (2007). Soluble protein oligomers in neurodegeneration: lessons from the Alzheimer’s amyloid beta-peptide. Nat. Rev. Mol. Cell Biol. 8, 101–112. doi: 10.1038/nrm2101
Hamedani, S. Y., Gharesouran, J., Noroozi, R., Sayad, A., Omrani, M. D., Mir, A., et al. (2017). Ras-like without CAAX 2 (RIT2): a susceptibility gene for autism spectrum disorder. Metab. Brain Dis. 32, 751–755. doi: 10.1007/s11011-017-9969-4
Hancock, J. F. (2003). Ras proteins: different signals from different locations. Nat. Rev. Mol. Cell Biol. 4, 373–384.
Hanke-Gogokhia, C., Wu, Z., Gerstner, C. D., Frederick, J. M., Zhang, H., and Baehr, W. (2016a). Arf-like protein 3 (ARL3) regulates protein trafficking and ciliogenesis in mouse photoreceptors. J. Biol. Chem. 291, 7142–7155. doi: 10.1074/jbc.m115.710954
Hanke-Gogokhia, C., Zhang, H., Frederick, J. M., and Baehr, W. (2016b). The function of Arf-like proteins ARL2 and ARL3 in photoreceptors. Adv. Exp. Med. Biol. 854, 655–661. doi: 10.1007/978-3-319-17121-0_87
Harms, F. L., Kloth, K., Bley, A., Denecke, J., Santer, R., Lessel, D., et al. (2018). Activating mutations in PAK1, encoding p21-activated kinase 1, cause a neurodevelopmental disorder. Am. J. Hum. Genet. 103, 579–591. doi: 10.1016/j.ajhg.2018.09.005
Harvey, C. D., Yasuda, R., Zhong, H., and Svoboda, K. (2008). The spread of Ras activity triggered by activation of a single dendritic spine. Science 321, 136–140. doi: 10.1126/science.1159675
Heidorn, S. J., Milagre, C., Whittaker, S., Nourry, A., Niculescu-Duvas, I., Dhomen, N., et al. (2010). Kinase-dead BRAF and oncogenic RAS cooperate to drive tumor progression through CRAF. Cell 140, 209–221. doi: 10.1016/j.cell.2009.12.040
Heo, W. D., and Meyer, T. (2003). Switch-of-function mutants based on morphology classification of Ras superfamily small GTPases. Cell 113, 315–328. doi: 10.1016/s0092-8674(03)00315-5
Hirata, Y., Brems, H., Suzuki, M., Kanamori, M., Okada, M., Morita, R., et al. (2016). Interaction between a domain of the negative regulator of the Ras-ERK pathway, SPRED1 protein, and the GTPase-activating protein-related domain of neurofibromin is implicated in legius syndrome and neurofibromatosis type 1. J. Biol. Chem. 291, 3124–3134. doi: 10.1074/jbc.m115.703710
Hoshino, M., and Nakamura, S. (2002). The Ras-like small GTP-binding protein Rin is activated by growth factor stimulation. Biochem. Biophys. Res. Commun. 295, 651–656. doi: 10.1016/s0006-291x(02)00731-3
Houlden, H., King, R. H., Hashemi-Nejad, A., Wood, N. W., Mathias, C. J., Reilly, M., et al. (2001). A novel TRK A (NTRK1) mutation associated with hereditary sensory and autonomic neuropathy type V. Ann. Neurol. 49, 521–525. doi: 10.1002/ana.103
Huffmeier, U., Zenker, M., Hoyer, J., Fahsold, R., and Rauch, A. (2006). A variable combination of features of Noonan syndrome and neurofibromatosis type I are caused by mutations in the NF1 gene. Am. J. Med. Genet. A 140, 2749–2756. doi: 10.1002/ajmg.a.31547
Indo, Y. (2001). Molecular basis of congenital insensitivity to pain with anhidrosis (CIPA): mutations and polymorphisms in TRKA (NTRK1) gene encoding the receptor tyrosine kinase for nerve growth factor. Hum. Mutat. 18, 462–471. doi: 10.1002/humu.1224
Ismail, S. A., Chen, Y. X., Rusinova, A., Chandra, A., Bierbaum, M., Gremer, L., et al. (2011). Arl2-GTP and Arl3-GTP regulate a GDI-like transport system for farnesylated cargo. Nat. Chem. Biol. 7, 942–949. doi: 10.1038/nchembio.686
Jackson, C. L., and Bouvet, S. (2014). Arfs at a glance. J. Cell Sci. 127, 4103–4109. doi: 10.1242/jcs.144899
Jeong, G. R., Jang, E. H., Bae, J. R., Jun, S., Kang, H. C., Park, C. H., et al. (2018). Dysregulated phosphorylation of Rab GTPases by LRRK2 induces neurodegeneration. Mol. Neurodegener. 13:8.
Jiang, H., He, C., Geng, S., Sheng, H., Shen, X., Zhang, X., et al. (2012). RhoT1 and Smad4 are correlated with lymph node metastasis and overall survival in pancreatic cancer. PLoS One 7:e42234. doi: 10.1371/journal.pone.0042234
Johnson, D. S., and Chen, Y. H. (2012). Ras family of small GTPases in immunity and inflammation. Curr. Opin. Pharmacol. 12, 458–463. doi: 10.1016/j.coph.2012.02.003
Jossin, Y. (2011). Polarization of migrating cortical neurons by Rap1 and N-cadherin: revisiting the model for the Reelin signaling pathway. Small GTPases 2, 322–328. doi: 10.4161/sgtp.18283
Jurnak, F. (1985). Structure of the GDP domain of EF-Tu and location of the amino acids homologous to ras oncogene proteins. Science 230, 32–36. doi: 10.1126/science.3898365
Kahn, R. A., and Gilman, A. G. (1986). The protein cofactor necessary for ADP-ribosylation of Gs by cholera toxin is itself a GTP binding protein. J. Biol. Chem. 261, 7906–7911.
Kametani, F., Usami, M., Tanaka, K., Kume, H., and Mori, H. (2004). Mutant presenilin (A260V) affects Rab8 in PC12D cell. Neurochem. Int. 44, 313–320. doi: 10.1016/s0197-0186(03)00176-1
Kanda, Y., and Watanabe, Y. (2007). Adrenaline increases glucose transport via a Rap1-p38MAPK pathway in rat vascular smooth muscle cells. Br. J. Pharmacol. 151, 476–482. doi: 10.1038/sj.bjp.0707247
Karimi-Moghadam, A., Charsouei, S., Bell, B., and Jabalameli, M. R. (2018). Parkinson disease from mendelian forms to genetic susceptibility: new molecular insights into the neurodegeneration process. Cell. Mol. Neurobiol. 38, 1153–1178. doi: 10.1007/s10571-018-0587-4
Karnoub, A. E., and Weinberg, R. A. (2008). Ras oncogenes: split personalities. Nat. Rev. Mol. Cell Biol. 9, 517–531. doi: 10.1038/nrm2438
Kim, J. K., Lee, S. Y., Park, C. W., Park, S. H., Yin, J., Kim, J., et al. (2014). Rab3a promotes brain tumor initiation and progression. Mol. Biol. Rep. 41, 5903–5911. doi: 10.1007/s11033-014-3465-2
Kim, W. Y., Shin, S. R., Kim, S., Jeon, S., and Kim, J. H. (2009). Cocaine regulates ezrin-radixin-moesin proteins and RhoA signaling in the nucleus accumbens. Neuroscience 163, 501–505. doi: 10.1016/j.neuroscience.2009.06.067
Knihtila, R., Holzapfel, G., Weiss, K., Meilleur, F., and Mattos, C. (2015). Neutron crystal structure of RAS GTPase puts in question the protonation state of the GTP gamma-phosphate. J. Biol. Chem. 290, 31025–31036. doi: 10.1074/jbc.m115.679860
Knodler, A., Feng, S., Zhang, J., Zhang, X., Das, A., Peranen, J., et al. (2010). Coordination of Rab8 and Rab11 in primary ciliogenesis. Proc. Natl. Acad. Sci. U.S.A. 107, 6346–6351. doi: 10.1073/pnas.1002401107
Konno, M., Hasegawa, T., Baba, T., Miura, E., Sugeno, N., Kikuchi, A., et al. (2012). Suppression of dynamin GTPase decreases alpha-synuclein uptake by neuronal and oligodendroglial cells: a potent therapeutic target for synucleinopathy. Mol. Neurodegener. 7:38. doi: 10.1186/1750-1326-7-38
Kontaridis, M. I., Swanson, K. D., David, F. S., Barford, D., and Neel, B. G. (2006). PTPN11 (Shp2) mutations in LEOPARD syndrome have dominant negative, not activating, effects. J. Biol. Chem. 281, 6785–6792. doi: 10.1074/jbc.m513068200
Labandeira-Garcia, J. L., Rodriguez-Perez, A. I., Villar-Cheda, B., Borrajo, A., Dominguez-Meijide, A., and Guerra, M. J. (2015). Rho kinase and dopaminergic degeneration: a promising therapeutic target for Parkinson’s disease. Neuroscientist 21, 616–629. doi: 10.1177/1073858414554954
Lahiri, V., and Klionsky, D. J. (2017). Functional impairment in RHOT1/Miro1 degradation and mitophagy is a shared feature in familial and sporadic Parkinson disease. Autophagy 13, 1259–1261. doi: 10.1080/15548627.2017.1327512
Lamprecht, R., Farb, C. R., and Ledoux, J. E. (2002). Fear memory formation involves p190 RhoGAP and ROCK proteins through a GRB2-mediated complex. Neuron 36, 727–738. doi: 10.1016/s0896-6273(02)01047-4
Lange-Carter, C. A., and Johnson, G. L. (1994). Ras-dependent growth factor regulation of MEK kinase in PC12 cells. Science 265, 1458–1461. doi: 10.1126/science.8073291
Latourelle, J. C., Dumitriu, A., Hadzi, T. C., Beach, T. G., and Myers, R. H. (2012). Evaluation of Parkinson disease risk variants as expression-QTLs. PLoS One 7:e46199. doi: 10.1371/journal.pone.0046199
Lee, E., Giovanello, K. S., Saykin, A. J., Xie, F., Kong, D., Wang, Y., et al. (2017). Single-nucleotide polymorphisms are associated with cognitive decline at Alzheimer’s disease conversion within mild cognitive impairment patients. Alzheimers Dement. 8, 86–95. doi: 10.1016/j.dadm.2017.04.004
Lee, J., Lee, J., and Ju, B. G. (2011). Drosophila arf72A acts as an essential regulator of endoplasmic reticulum quality control and suppresses autosomal-dominant retinopathy. Int. J. Biochem. Cell Biol. 43, 1392–1401. doi: 10.1016/j.biocel.2011.06.004
Lee, J. H., Tecedor, L., Chen, Y. H., Monteys, A. M., Sowada, M. J., Thompson, L. M., et al. (2015). Reinstating aberrant mTORC1 activity in Huntington’s disease mice improves disease phenotypes. Neuron 85, 303–315. doi: 10.1016/j.neuron.2014.12.019
Lee, M. N., Ha, S. H., Kim, J., Koh, A., Lee, C. S., Kim, J. H., et al. (2009). Glycolytic flux signals to mTOR through glyceraldehyde-3-phosphate dehydrogenase-mediated regulation of Rheb. Mol. Cell Biol. 29, 3991–4001. doi: 10.1128/mcb.00165-09
Lee, V. M., Goedert, M., and Trojanowski, J. Q. (2001). Neurodegenerative tauopathies. Annu. Rev. Neurosci. 24, 1121–1159. doi: 10.1146/annurev.neuro.24.1.1121
Legius, E., Marchuk, D. A., Collins, F. S., and Glover, T. W. (1993). Somatic deletion of the neurofibromatosis type 1 gene in a neurofibrosarcoma supports a tumour suppressor gene hypothesis. Nat. Genet. 3, 122–126. doi: 10.1038/ng0293-122
Lein, P. J., Guo, X., Shi, G. X., Moholt-Siebert, M., Bruun, D., and Andres, D. A. (2007). The novel GTPase Rit differentially regulates axonal and dendritic growth. J. Neurosci. 27, 4725–4736. doi: 10.1523/jneurosci.5633-06.2007
Lelieveld, S. H., Reijnders, M. R., Pfundt, R., Yntema, H. G., Kamsteeg, E. J., De Vries, P., et al. (2016). Meta-analysis of 2,104 trios provides support for 10 new genes for intellectual disability. Nat. Neurosci. 19, 1194–1196. doi: 10.1038/nn.4352
Leoni, C., Onesimo, R., Giorgio, V., Diamanti, A., Giorgio, D., Martini, L., et al. (2016). Understanding growth failure in costello syndrome: increased resting energy expenditure. J. Pediatr. 170, 322–324. doi: 10.1016/j.jpeds.2015.11.076
Li, G. (2011). Rab GTPases, membrane trafficking and diseases. Curr. Drug Targets 12, 1188–1193. doi: 10.2174/138945011795906561
Li, J., Kanekiyo, T., Shinohara, M., Zhang, Y., Ladu, M. J., Xu, H., et al. (2012). Differential regulation of amyloid-beta endocytic trafficking and lysosomal degradation by apolipoprotein E isoforms. J. Biol. Chem. 287, 44593–44601. doi: 10.1074/jbc.m112.420224
Li, J., Xing, H., Jiang, G., Su, Z., Wu, Y., Zhang, Y., et al. (2016). Increased expression of Rac1 in epilepsy patients and animal models. Neurochem. Res. 41, 836–843. doi: 10.1007/s11064-015-1759-y
Liang, Y., Lin, S., Zou, L., Zhou, H., Zhang, J., Su, B., et al. (2012). Expression profiling of Rab GTPases reveals the involvement of Rab20 and Rab32 in acute brain inflammation in mice. Neurosci. Lett. 527, 110–114. doi: 10.1016/j.neulet.2012.08.039
Lim, K. C., and Crino, P. B. (2013). Focal malformations of cortical development: new vistas for molecular pathogenesis. Neuroscience 252, 262–276. doi: 10.1016/j.neuroscience.2013.07.037
Lin, H. H., Wu, R. M., Lin, H. I., Chen, M. L., Tai, C. H., and Lin, C. H. (2017). Lack of RAB39B mutations in early-onset and familial Parkinson’s disease in a Taiwanese cohort. Neurobiol. Aging 50, 169.e3–169.e4. doi: 10.1016/j.neurobiolaging.2016.10.021
Linford, A., Yoshimura, S., Nunes Bastos, R., Langemeyer, L., Gerondopoulos, A., Rigden, D. J., et al. (2012). Rab14 and its exchange factor FAM116 link endocytic recycling and adherens junction stability in migrating cells. Dev. Cell 22, 952–966. doi: 10.1016/j.devcel.2012.04.010
Liu, W. N., Yan, M., and Chan, A. M. (2017). A thirty-year quest for a role of R-Ras in cancer: from an oncogene to a multitasking GTPase. Cancer Lett. 403, 59–65. doi: 10.1016/j.canlet.2017.06.003
Liu, X., Shimada, T., Otowa, T., Wu, Y. Y., Kawamura, Y., Tochigi, M., et al. (2016). Genome-wide association study of autism spectrum disorder in the east asian populations. Autism Res. 9, 340–349. doi: 10.1002/aur.1536
Liu, Z. H., Guo, J. F., Wang, Y. Q., Li, K., Sun, Q. Y., Xu, Q., et al. (2015). Assessment of RIT2 rs12456492 association with Parkinson’s disease in Mainland China. Neurobiol. Aging 36, 1600.e9–1600.e11. doi: 10.1016/j.neurobiolaging.2014.12.012
Locke, C. J., Kautu, B. B., Berry, K. P., Lee, S. K., Caldwell, K. A., and Caldwell, G. A. (2009). Pharmacogenetic analysis reveals a post-developmental role for Rac GTPases in Caenorhabditis elegans GABAergic neurotransmission. Genetics 183, 1357–1372. doi: 10.1534/genetics.109.106880
Ma, Y., Gong, Y., Cheng, Z., Loganathan, S., Kao, C., Sarkaria, J. N., et al. (2015). Critical functions of RhoB in support of glioblastoma tumorigenesis. Neuro. Oncol. 17, 516–525. doi: 10.1093/neuonc/nou228
MacDonald, M. E., Ambrose, C. M., Duyao, M. P., Myers, R. H., Lin, C., Srinidhi, L., et al. (1993). A novel gene containing a trinucleotide repeat that is expanded and unstable on Huntington’s disease chromosomes. The Huntington’s Disease Collaborative Research Group. Cell 72, 971–983. doi: 10.1016/0092-8674(93)90585-e
Machida, N., Umikawa, M., Takei, K., Sakima, N., Myagmar, B. E., Taira, K., et al. (2004). Mitogen-activated protein kinase kinase kinase kinase 4 as a putative effector of Rap2 to activate the c-Jun N-terminal kinase. J. Biol. Chem. 279, 15711–15714. doi: 10.1074/jbc.c300542200
Madaule, P., and Axel, R. (1985). A novel Ras-related gene family. Cell 41, 31–40. doi: 10.1016/0092-8674(85)90058-3
Madero-Perez, J., Fdez, E., Fernandez, B., Lara Ordonez, A. J., Blanca Ramirez, M., Gomez-Suaga, P., et al. (2018). Parkinson disease-associated mutations in LRRK2 cause centrosomal defects via Rab8a phosphorylation. Mol. Neurodegener. 13:3.
Marin, T. M., Keith, K., Davies, B., Conner, D. A., Guha, P., Kalaitzidis, D., et al. (2011). Rapamycin reverses hypertrophic cardiomyopathy in a mouse model of LEOPARD syndrome-associated PTPN11 mutation. J. Clin. Invest. 121, 1026–1043. doi: 10.1172/jci44972
Mastroeni, D., Chouliaras, L., Grover, A., Liang, W. S., Hauns, K., Rogers, J., et al. (2013). Reduced RAN expression and disrupted transport between cytoplasm and nucleus; a key event in Alzheimer’s disease pathophysiology. PLoS One 8:e53349. doi: 10.1371/journal.pone.0053349
Melser, S., Chatelain, E. H., Lavie, J., Mahfouf, W., Jose, C., Obre, E., et al. (2013). Rheb regulates mitophagy induced by mitochondrial energetic status. Cell Metab. 17, 719–730. doi: 10.1016/j.cmet.2013.03.014
Mendoza-Naranjo, A., Gonzalez-Billault, C., and Maccioni, R. B. (2007). Abeta1-42 stimulates actin polymerization in hippocampal neurons through Rac1 and Cdc42 Rho GTPases. J. Cell Sci. 120, 279–288. doi: 10.1242/jcs.03323
Mir, R., Tonelli, F., Lis, P., Macartney, T., Polinski, N. K., Martinez, T. N., et al. (2018). The Parkinson’s disease VPS35[D620N] mutation enhances LRRK2-mediated Rab protein phosphorylation in mouse and human. Biochem. J. 475, 1861–1883. doi: 10.1042/bcj20180248
Mohamed, N. V., Desjardins, A., and Leclerc, N. (2017). Tau secretion is correlated to an increase of Golgi dynamics. PLoS One 12:e0178288. doi: 10.1371/journal.pone.0178288
Moritz, O. L., Tam, B. M., Hurd, L. L., Peranen, J., Deretic, D., and Papermaster, D. S. (2001). Mutant Rab8 Impairs docking and fusion of rhodopsin-bearing post-Golgi membranes and causes cell death of transgenic Xenopus rods. Mol. Biol. Cell 12, 2341–2351. doi: 10.1091/mbc.12.8.2341
Morris, H. R., Waite, A. J., Williams, N. M., Neal, J. W., and Blake, D. J. (2012). Recent advances in the genetics of the ALS-FTLD complex. Curr. Neurol. Neurosci. Rep. 12, 243–250. doi: 10.1007/s11910-012-0268-5
Moshiri, A., Humpal, D., Leonard, B. C., Imai, D. M., Tham, A., Bower, L., et al. (2017). Arap1 deficiency causes photoreceptor degeneration in mice. Invest. Ophthalmol. Vis. Sci. 58, 1709–1718.
Mulders, W. H., Rodger, J., Yates, C. G., and Robertson, D. (2014). Modulation of gene expression in guinea pig paraflocculus after induction of hearing loss. F1000Res. 3:63.
Nakamura, T., Yasuda, S., Nagai, H., Koinuma, S., Morishita, S., Goto, A., et al. (2013). Longest neurite-specific activation of Rap1B in hippocampal neurons contributes to polarity formation through RalA and Nore1A in addition to PI3-kinase. Genes Cells 18, 1020–1031. doi: 10.1111/gtc.12097
Nakhaei-Rad, S., Haghighi, F., Nouri, P., Rezaei Adariani, S., Lissy, J., Kazemein Jasemi, N. S., et al. (2018). Structural fingerprints, interactions, and signaling networks of RAS family proteins beyond RAS isoforms. Crit. Rev. Biochem. Mol. Biol. 53, 130–156. doi: 10.1080/10409238.2018.1431605
Narumiya, S., and Thumkeo, D. (2018). Rho signaling research: history, current status and future directions. FEBS Lett. 592, 1763–1776. doi: 10.1002/1873-3468.13087
Navaroli, D. M., Stevens, Z. H., Uzelac, Z., Gabriel, L., King, M. J., Lifshitz, L. M., et al. (2011). The plasma membrane-associated GTPase Rin interacts with the dopamine transporter and is required for protein kinase C-regulated dopamine transporter trafficking. J. Neurosci. 31, 13758–13770. doi: 10.1523/jneurosci.2649-11.2011
Nelson, N., and Clark, G. J. (2016). Rheb may complex with RASSF1A to coordinate Hippo and TOR signaling. Oncotarget 7, 33821–33831.
Ng, E. L., and Tang, B. L. (2008). Rab GTPases and their roles in brain neurons and glia. Brain Res. Rev. 58, 236–246. doi: 10.1016/j.brainresrev.2008.04.006
Noonan, J. A. (1968). Hypertelorism with Turner phenotype. A new syndrome with associated congenital heart disease. Am. J. Dis. Child 116, 373–380.
Ohba, Y., Mochizuki, N., Yamashita, S., Chan, A. M., Schrader, J. W., Hattori, S., et al. (2000). Regulatory proteins of R-Ras, TC21/R-Ras2, and M-Ras/R-Ras3. J. Biol. Chem. 275, 20020–20026. doi: 10.1074/jbc.m000981200
Paduch, M., Jelen, F., and Otlewski, J. (2001). Structure of small G proteins and their regulators. Acta Biochim. Pol. 48, 829–850.
Paisan-Ruiz, C., Lewis, P. A., and Singleton, A. B. (2013). LRRK2: cause, risk, and mechanism. J. Parkinsons Dis. 3, 85–103.
Pandit, B., Sarkozy, A., Pennacchio, L. A., Carta, C., Oishi, K., Martinelli, S., et al. (2007). Gain-of-function RAF1 mutations cause Noonan and LEOPARD syndromes with hypertrophic cardiomyopathy. Nat. Genet. 39, 1007–1012. doi: 10.1038/ng2073
Pankratz, N., Beecham, G. W., Destefano, A. L., Dawson, T. M., Doheny, K. F., Factor, S. A., et al. (2012). Meta-analysis of Parkinson’s disease: identification of a novel locus, RIT2. Ann. Neurol. 71, 370–384. doi: 10.1002/ana.22687
Pereira-Leal, J. B., and Seabra, M. C. (2001). Evolution of the Rab family of small GTP-binding proteins. J. Mol. Biol. 313, 889–901. doi: 10.1006/jmbi.2001.5072
Peru Y Colón de Portugal, R. L., Acevedo, S. F., Rodan, A. R., Chang, L. Y., Eaton, B. A., and Rothenfluh, A. (2012). Adult neuronal Arf6 controls ethanol-induced behavior with Arfaptin downstream of Rac1 and RhoGAP18B. J. Neurosci. 32, 17706–17713. doi: 10.1523/jneurosci.1944-12.2012
Pfeffer, S. R. (2018). LRRK2 and Rab GTPases. Biochem. Soc. Trans. 46, 1707–1712. doi: 10.1042/bst20180470
Pierpont, M. E., Magoulas, P. L., Adi, S., Kavamura, M. I., Neri, G., Noonan, J., et al. (2014). Cardio-facio-cutaneous syndrome: clinical features, diagnosis, and management guidelines. Pediatrics 134, e1149–e1162. doi: 10.1542/peds.2013-3189
Piluso, G., Monteleone, P., Galderisi, S., Giugliano, T., Bertolino, A., Rocca, P., et al. (2017). Assessment of de novo copy-number variations in Italian patients with schizophrenia: detection of putative mutations involving regulatory enhancer elements. World J. Biol. Psychiatry 20, 126–136. doi: 10.1080/15622975.2017.1395072
Pottier, C., Ravenscroft, T. A., Sanchez-Contreras, M., and Rademakers, R. (2016). Genetics of FTLD: overview and what else we can expect from genetic studies. J. Neurochem. 138(Suppl. 1), 32–53. doi: 10.1111/jnc.13622
Prabakaran, S., Swatton, J. E., Ryan, M. M., Huffaker, S. J., Huang, J. T., Griffin, J. L., et al. (2004). Mitochondrial dysfunction in schizophrenia: evidence for compromised brain metabolism and oxidative stress. Mol. Psychiatry 9, 684–697, 643.
Prekeris, R. (2012). The art of “cut and run”: the role of Rab14 GTPase in regulating N-cadherin shedding and cell motility. Dev. Cell 22, 909–910. doi: 10.1016/j.devcel.2012.05.002
Prior, I. A., and Hancock, J. F. (2012). Ras trafficking, localization and compartmentalized signalling. Semin. Cell Dev. Biol. 23, 145–153. doi: 10.1016/j.semcdb.2011.09.002
Pryor, W. M., Biagioli, M., Shahani, N., Swarnkar, S., Huang, W. C., Page, D. T., et al. (2014). Huntingtin promotes mTORC1 signaling in the pathogenesis of Huntington’s disease. Sci. Signal. 7:ra103. doi: 10.1126/scisignal.2005633
Pulciani, S., Santos, E., Lauver, A. V., Long, L. K., Aaronson, S. A., and Barbacid, M. (1982). Oncogenes in solid human tumours. Nature 300, 539–542. doi: 10.1038/300539a0
Purlyte, E., Dhekne, H. S., Sarhan, A. R., Gomez, R., Lis, P., Wightman, M., et al. (2018). Rab29 activation of the Parkinson’s disease-associated LRRK2 kinase. EMBO J. 37, 1–18. doi: 10.15252/embj.201798099
Rauen, K. A. (2007). HRAS and the Costello syndrome. Clin. Genet. 71, 101–108. doi: 10.1111/j.1399-0004.2007.00743.x
Rees, E., Walters, J. T., Chambert, K. D., O’dushlaine, C., Szatkiewicz, J., Richards, A. L., et al. (2014). CNV analysis in a large schizophrenia sample implicates deletions at 16p12.1 and SLC1A1 and duplications at 1p36.33 and CGNL1. Hum. Mol. Genet. 23, 1669–1676. doi: 10.1093/hmg/ddt540
Reijnders, M. R. F., Ansor, N. M., Kousi, M., Yue, W. W., Tan, P. L., Clarkson, K., et al. (2017). RAC1 missense mutations in developmental disorders with diverse phenotypes. Am. J. Hum. Genet. 101, 466–477. doi: 10.1016/j.ajhg.2017.08.007
Reiter, E., Ahn, S., Shukla, A. K., and Lefkowitz, R. J. (2012). Molecular mechanism of beta-arrestin-biased agonism at seven-transmembrane receptors. Annu. Rev. Pharmacol. Toxicol. 52, 179–197. doi: 10.1146/annurev.pharmtox.010909.105800
Ridge, P. G., Karch, C. M., Hsu, S., Arano, I., Teerlink, C. C., Ebbert, M. T. W., et al. (2017). Linkage, whole genome sequence, and biological data implicate variants in RAB10 in Alzheimer’s disease resilience. Genome Med. 9:100.
Rodriguez, L., Mohamed, N. V., Desjardins, A., Lippe, R., Fon, E. A., and Leclerc, N. (2017). Rab7A regulates tau secretion. J. Neurochem. 141, 592–605. doi: 10.1111/jnc.13994
Rodriguez-Viciana, P., Sabatier, C., and Mccormick, F. (2004). Signaling specificity by Ras family GTPases is determined by the full spectrum of effectors they regulate. Mol. Cell Biol. 24, 4943–4954. doi: 10.1128/mcb.24.11.4943-4954.2004
Rodriguez-Viciana, P., Tetsu, O., Tidyman, W. E., Estep, A. L., Conger, B. A., Cruz, M. S., et al. (2006). Germline mutations in genes within the MAPK pathway cause cardio-facio-cutaneous syndrome. Science 311, 1287–1290. doi: 10.1126/science.1124642
Rojas, A. M., Fuentes, G., Rausell, A., and Valencia, A. (2012). The Ras protein superfamily: evolutionary tree and role of conserved amino acids. J. Cell Biol. 196, 189–201. doi: 10.1083/jcb.201103008
Rolfe, B. E., Worth, N. F., World, C. J., Campbell, J. H., and Campbell, G. R. (2005). Rho and vascular disease. Atherosclerosis 183, 1–16. doi: 10.1016/j.atherosclerosis.2005.04.023
Roosen, D. A., and Cookson, M. R. (2016). LRRK2 at the interface of autophagosomes, endosomes and lysosomes. Mol. Neurodegener. 11:73.
Roosing, S., Rohrschneider, K., Beryozkin, A., Sharon, D., Weisschuh, N., Staller, J., et al. (2013). Mutations in RAB28, encoding a farnesylated small GTPase, are associated with autosomal-recessive cone-rod dystrophy. Am. J. Hum. Genet. 93, 110–117. doi: 10.1016/j.ajhg.2013.05.005
Saito, K., Araki, Y., Kontani, K., Nishina, H., and Katada, T. (2005). Novel role of the small GTPase Rheb: its implication in endocytic pathway independent of the activation of mammalian target of rapamycin. J. Biochem. 137, 423–430. doi: 10.1093/jb/mvi046
Saitou, N., and Nei, M. (1987). The neighbor-joining method: a new method for reconstructing phylogenetic trees. Mol. Biol. Evol. 4, 406–425.
Salyakina, D., Cukier, H. N., Lee, J. M., Sacharow, S., Nations, L. D., Ma, D., et al. (2011). Copy number variants in extended autism spectrum disorder families reveal candidates potentially involved in autism risk. PLoS One 6:e26049. doi: 10.1371/journal.pone.0026049
Santos, E., Martin-Zanca, D., Reddy, E. P., Pierotti, M. A., Della Porta, G., and Barbacid, M. (1984). Malignant activation of a K-ras oncogene in lung carcinoma but not in normal tissue of the same patient. Science 223, 661–664. doi: 10.1126/science.6695174
Saridaki, T., Nippold, M., Dinter, E., Roos, A., Diederichs, L., Fensky, L., et al. (2018). FYCO1 mediates clearance of alpha-synuclein aggregates through a Rab7-dependent mechanism. J. Neurochem. 146, 474–492. doi: 10.1111/jnc.14461
Sarkozy, A., Digilio, M. C., and Dallapiccola, B. (2008). Leopard syndrome. Orphanet J. Rare Dis. 3:13.
Sathasivam, K., Neueder, A., Gipson, T. A., Landles, C., Benjamin, A. C., Bondulich, M. K., et al. (2013). Aberrant splicing of HTT generates the pathogenic exon 1 protein in Huntington disease. Proc. Natl. Acad. Sci. U.S.A. 110, 2366–2370. doi: 10.1073/pnas.1221891110
Sato, T., Akasu, H., Shimono, W., Matsu, C., Fujiwara, Y., Shibagaki, Y., et al. (2015). Rheb protein binds CAD (carbamoyl-phosphate synthetase 2, aspartate transcarbamoylase, and dihydroorotase) protein in a GTP- and effector domain-dependent manner and influences its cellular localization and carbamoyl-phosphate synthetase (CPSase) activity. J. Biol. Chem. 290, 1096–1105. doi: 10.1074/jbc.m114.592402
Satoh, A. K., O’tousa, J. E., Ozaki, K., and Ready, D. F. (2005). Rab11 mediates post-Golgi trafficking of rhodopsin to the photosensitive apical membrane of Drosophila photoreceptors. Development 132, 1487–1497. doi: 10.1242/dev.01704
Saxena, S., Cabuy, E., and Caroni, P. (2009). A role for motoneuron subtype-selective ER stress in disease manifestations of FALS mice. Nat. Neurosci. 12, 627–636. doi: 10.1038/nn.2297
Scheper, W., Hoozemans, J. J., Hoogenraad, C. C., Rozemuller, A. J., Eikelenboom, P., and Baas, F. (2007). Rab6 is increased in Alzheimer’s disease brain and correlates with endoplasmic reticulum stress. Neuropathol. Appl. Neurobiol. 33, 523–532.
Schoneborn, H., Raudzus, F., Coppey, M., Neumann, S., and Heumann, R. (2018). Perspectives of RAS and RHEB GTPase signaling pathways in regenerating brain neurons. Int. J. Mol. Sci. 19:4052.
Schwartz, M. A., and Shattil, S. J. (2000). Signaling networks linking integrins and Rho family GTPases. Trends Biochem. Sci. 25, 388–391. doi: 10.1016/s0968-0004(00)01605-4
Schwarz, T. L. (2013). Mitochondrial trafficking in neurons. Cold Spring Harb. Perspect. Biol. 5:a011304.
Segev, N. (2001). Ypt/rab gtpases: regulators of protein trafficking. Sci. STKE 2001:re11. doi: 10.1126/stke.2001.100.re11
Shahani, N., Huang, W. C., Varnum, M., Page, D. T., and Subramaniam, S. (2017). Forebrain depletion of Rheb GTPase elicits spatial memory deficits in mice. Neurobiol. Aging 50, 134–143. doi: 10.1016/j.neurobiolaging.2016.11.006
Shahani, N., Pryor, W., Swarnkar, S., Kholodilov, N., Thinakaran, G., Burke, R. E., et al. (2014). Rheb GTPase regulates beta-secretase levels and amyloid beta generation. J. Biol. Chem. 289, 5799–5808. doi: 10.1074/jbc.m113.532713
Shao, H., and Andres, D. A. (2000). A novel RalGEF-like protein, RGL3, as a candidate effector for Rit and Ras. J. Biol. Chem. 275, 26914–26924.
Sheen, V. L., Ganesh, V. S., Topcu, M., Sebire, G., Bodell, A., Hill, R. S., et al. (2004). Mutations in ARFGEF2 implicate vesicle trafficking in neural progenitor proliferation and migration in the human cerebral cortex. Nat. Genet. 36, 69–76. doi: 10.1038/ng1276
Shen, W., Wu, B., Zhang, Z., Dou, Y., Rao, Z. R., Chen, Y. R., et al. (2006). Activity-induced rapid synaptic maturation mediated by presynaptic cdc42 signaling. Neuron 50, 401–414. doi: 10.1016/j.neuron.2006.03.017
Sheng, Z. H. (2014). Mitochondrial trafficking and anchoring in neurons: new insight and implications. J. Cell Biol. 204, 1087–1098. doi: 10.1083/jcb.201312123
Shenoy, S. K., and Lefkowitz, R. J. (2011). beta-Arrestin-mediated receptor trafficking and signal transduction. Trends Pharmacol. Sci. 32, 521–533. doi: 10.1016/j.tips.2011.05.002
Shi, G. X., and Andres, D. A. (2005). Rit contributes to nerve growth factor-induced neuronal differentiation via activation of B-Raf-extracellular signal-regulated kinase and p38 mitogen-activated protein kinase cascades. Mol. Cell Biol. 25, 830–846. doi: 10.1128/mcb.25.2.830-846.2005
Shi, G. X., Cai, W., and Andres, D. A. (2013). Rit subfamily small GTPases: regulators in neuronal differentiation and survival. Cell. Signal. 25, 2060–2068. doi: 10.1016/j.cellsig.2013.06.002
Shi, G. X., Jin, L., and Andres, D. A. (2011). A rit GTPase-p38 mitogen-activated protein kinase survival pathway confers resistance to cellular stress. Mol. Cell Biol. 31, 1938–1948. doi: 10.1128/mcb.01380-10
Shimohama, S., Kamiya, S., Taniguchi, T., Sumida, Y., and Fujimoto, S. (1999). Differential involvement of small G proteins in Alzheimer’s disease. Int. J. Mol. Med. 3, 597–600.
Shin, O. H. (2014). Exocytosis and synaptic vesicle function. Compr. Physiol. 4, 149–175. doi: 10.1002/cphy.c130021
Siegfried, A., Cances, C., Denuelle, M., Loukh, N., Tauber, M., Cave, H., et al. (2017). Noonan syndrome, PTPN11 mutations, and brain tumors. A clinical report and review of the literature. Am. J. Med. Genet. A 173, 1061–1065.
Simanshu, D. K., Nissley, D. V., and Mccormick, F. (2017). RAS proteins and their regulators in human disease. Cell 170, 17–33. doi: 10.1016/j.cell.2017.06.009
Slack, C. (2017). Ras signaling in aging and metabolic regulation. Nutr. Healthy Aging 4, 195–205. doi: 10.3233/nha-160021
Slack, C., Alic, N., Foley, A., Cabecinha, M., Hoddinott, M. P., and Partridge, L. (2015). The Ras-Erk-ETS-signaling pathway is a drug target for longevity. Cell 162, 72–83. doi: 10.1016/j.cell.2015.06.023
Sokolov, A. M., Seluzicki, C. M., Morton, M. C., and Feliciano, D. M. (2018). Dendrite growth and the effect of ectopic Rheb expression on cortical neurons. Neurosci. Lett. 671, 140–147. doi: 10.1016/j.neulet.2018.02.021
Sonmez, F. M., Uctepe, E., Aktas, D., and Alikasifoglu, M. (2017). Microdeletion of chromosome 1q21.3 in fraternal twins is associated with mental retardation, microcephaly, and epilepsy. Intractable Rare Dis. Res. 6, 61–64. doi: 10.5582/irdr.2016.01075
Spano, D., Branchi, I., Rosica, A., Pirro, M. T., Riccio, A., Mithbaokar, P., et al. (2004). Rhes is involved in striatal function. Mol. Cell Biol. 24, 5788–5796. doi: 10.1128/mcb.24.13.5788-5796.2004
Stankiewicz, T. R., and Linseman, D. A. (2014). Rho family GTPases: key players in neuronal development, neuronal survival, and neurodegeneration. Front. Cell. Neurosci. 8:314. doi: 10.3389/fncel.2014.00314
Steger, M., Diez, F., Dhekne, H. S., Lis, P., Nirujogi, R. S., Karayel, O., et al. (2017). Systematic proteomic analysis of LRRK2-mediated Rab GTPase phosphorylation establishes a connection to ciliogenesis. eLife 6:e31012.
Steger, M., Tonelli, F., Ito, G., Davies, P., Trost, M., Vetter, M., et al. (2016). Phosphoproteomics reveals that Parkinson’s disease kinase LRRK2 regulates a subset of Rab GTPases. eLife 5:e12813.
Stevenson, D. A., Viskochil, D. H., Rope, A. F., and Carey, J. C. (2006). Clinical and molecular aspects of an informative family with neurofibromatosis type 1 and Noonan phenotype. Clin. Genet. 69, 246–253. doi: 10.1111/j.1399-0004.2006.00576.x
Stornetta, R. L., and Zhu, J. J. (2011). Ras and Rap signaling in synaptic plasticity and mental disorders. Neuroscientist 17, 54–78. doi: 10.1177/1073858410365562
Subramaniam, S., Napolitano, F., Mealer, R. G., Kim, S., Errico, F., Barrow, R., et al. (2011). Rhes, a striatal-enriched small G protein, mediates mTOR signaling and L-DOPA-induced dyskinesia. Nat. Neurosci. 15, 191–193. doi: 10.1038/nn.2994
Subramaniam, S., Sixt, K. M., Barrow, R., and Snyder, S. H. (2009). Rhes, a striatal specific protein, mediates mutant-huntingtin cytotoxicity. Science 324, 1327–1330. doi: 10.1126/science.1172871
Sugiura, H., Yasuda, S., Katsurabayashi, S., Kawano, H., Endo, K., Takasaki, K., et al. (2015). Rheb activation disrupts spine synapse formation through accumulation of syntenin in tuberous sclerosis complex. Nat. Commun. 6:6842.
Sun, W., Samimi, H., Gamez, M., Zare, H., and Frost, B. (2018). Pathogenic tau-induced piRNA depletion promotes neuronal death through transposable element dysregulation in neurodegenerative tauopathies. Nat. Neurosci. 21, 1038–1048. doi: 10.1038/s41593-018-0194-1
Swanson, A. M., Depoy, L. M., and Gourley, S. L. (2017). Inhibiting Rho kinase promotes goal-directed decision making and blocks habitual responding for cocaine. Nat. Commun. 8:1861.
Tada, H., Taira, Y., Morichika, K., and Kinoshita, T. (2016). Mitochondrial trafficking through Rhot1 is involved in the aggregation of germinal granule components during primordial germ cell formation in Xenopus embryos. Dev. Growth Differ. 58, 641–650. doi: 10.1111/dgd.12310
Tajan, M., De Rocca Serra, A., Valet, P., Edouard, T., and Yart, A. (2015). SHP2 sails from physiology to pathology. Eur. J. Med. Genet. 58, 509–525. doi: 10.1016/j.ejmg.2015.08.005
Tajan, M., Paccoud, R., Branka, S., Edouard, T., and Yart, A. (2018). The RASopathy family: consequences of germline activation of the RAS/MAPK pathway. Endocr. Rev. 39, 676–700. doi: 10.1210/er.2017-00232
Takai, Y., Sasaki, T., and Matozaki, T. (2001). Small GTP-binding proteins. Physiol. Rev. 81, 153–208.
Takamori, S., Holt, M., Stenius, K., Lemke, E. A., Gronborg, M., Riedel, D., et al. (2006). Molecular anatomy of a trafficking organelle. Cell 127, 831–846.
Tansey, K. E., Rees, E., Linden, D. E., Ripke, S., Chambert, K. D., Moran, J. L., et al. (2016). Common alleles contribute to schizophrenia in CNV carriers. Mol. Psychiatry 21:1153. doi: 10.1038/mp.2015.170
Tartaglia, M., Gelb, B. D., and Zenker, M. (2011). Noonan syndrome and clinically related disorders. Best Pract. Res. Clin. Endocrinol. Metab. 25, 161–179. doi: 10.1016/j.beem.2010.09.002
Teodoro, R. O., Pekkurnaz, G., Nasser, A., Higashi-Kovtun, M. E., Balakireva, M., Mclachlan, I. G., et al. (2013). Ral mediates activity-dependent growth of postsynaptic membranes via recruitment of the exocyst. EMBO J. 32, 2039–2055. doi: 10.1038/emboj.2013.147
Tidyman, W. E., and Rauen, K. A. (2016a). Expansion of the RASopathies. Curr. Genet. Med. Rep. 4, 57–64. doi: 10.1007/s40142-016-0100-7
Tidyman, W. E., and Rauen, K. A. (2016b). Pathogenetics of the RASopathies. Hum. Mol. Genet. 25, R123–R132.
Tsika, E., and Moore, D. J. (2013). Contribution of GTPase activity to LRRK2-associated Parkinson disease. Small GTPases 4, 164–170. doi: 10.4161/sgtp.25130
Tyagi, R., Shahani, N., Gorgen, L., Ferretti, M., Pryor, W., Chen, P. Y., et al. (2015). Rheb inhibits protein synthesis by activating the PERK-eIF2alpha signaling cascade. Cell Rep. 10, 684–693.
Udayar, V., Buggia-Prevot, V., Guerreiro, R. L., Siegel, G., Rambabu, N., Soohoo, A. L., et al. (2013). A paired RNAi and RabGAP overexpression screen identifies Rab11 as a regulator of beta-amyloid production. Cell Rep. 5, 1536–1551. doi: 10.1016/j.celrep.2013.12.005
Ullrich, M., Weber, M., Post, A. M., Popp, S., Grein, J., Zechner, M., et al. (2018). OCD-like behavior is caused by dysfunction of thalamo-amygdala circuits and upregulated TrkB/ERK-MAPK signaling as a result of SPRED2 deficiency. Mol. Psychiatry 23, 444–458. doi: 10.1038/mp.2016.232
Uno, T., Furutani, M., Watanabe, C., Sakamoto, K., Uno, Y., Kanamaru, K., et al. (2016). Rab proteins in the brain and corpus allatum of Bombyx mori. Histochem. Cell Biol. 146, 59–69. doi: 10.1007/s00418-016-1422-y
Urano, J., Ellis, C., Clark, G. J., and Tamanoi, F. (2001). Characterization of Rheb functions using yeast and mammalian systems. Methods Enzymol. 333, 217–231. doi: 10.1016/s0076-6879(01)33058-6
Van Der Merwe, C., Jalali Sefid Dashti, Z., Christoffels, A., Loos, B., and Bardien, S. (2015). Evidence for a common biological pathway linking three Parkinson’s disease-causing genes: parkin, PINK1 and DJ-1. Eur. J. Neurosci. 41, 1113–1125. doi: 10.1111/ejn.12872
Van Trier, D. C., Rinne, T., Noordam, K., Draaisma, J. M., and Van Der Burgt, I. (2017). Variable phenotypic expression in a large Noonan syndrome family segregating a novel SOS1 mutation. Am. J. Med. Genet. A 173, 2968–2972. doi: 10.1002/ajmg.a.38466
Veltel, S., Gasper, R., Eisenacher, E., and Wittinghofer, A. (2008a). The retinitis pigmentosa 2 gene product is a GTPase-activating protein for Arf-like 3. Nat. Struct. Mol. Biol. 15, 373–380. doi: 10.1038/nsmb.1396
Veltel, S., Kravchenko, A., Ismail, S., and Wittinghofer, A. (2008b). Specificity of Arl2/Arl3 signaling is mediated by a ternary Arl3-effector-GAP complex. FEBS Lett. 582, 2501–2507. doi: 10.1016/j.febslet.2008.05.053
Vigil, D., Cherfils, J., Rossman, K. L., and Der, C. J. (2010). Ras superfamily GEFs and GAPs: validated and tractable targets for cancer therapy? Nat. Rev. Cancer 10, 842–857. doi: 10.1038/nrc2960
Volk, L., Chiu, S. L., Sharma, K., and Huganir, R. L. (2015). Glutamate synapses in human cognitive disorders. Annu. Rev. Neurosci. 38, 127–149. doi: 10.1146/annurev-neuro-071714-033821
Wakioka, T., Sasaki, A., Kato, R., Shouda, T., Matsumoto, A., Miyoshi, K., et al. (2001). Spred is a Sprouty-related suppressor of Ras signalling. Nature 412, 647–651. doi: 10.1038/35088082
Wang, H., and Jiang, C. (2013). RAB38 confers a poor prognosis, associated with malignant progression and subtype preference in glioma. Oncol. Rep. 30, 2350–2356. doi: 10.3892/or.2013.2730
Wang, L., Popko, B., and Roos, R. P. (2011). The unfolded protein response in familial amyotrophic lateral sclerosis. Hum. Mol. Genet. 20, 1008–1015. doi: 10.1093/hmg/ddq546
Wang, W., Ju, Y. Y., Zhou, Q. X., Tang, J. X., Li, M., Zhang, L., et al. (2017). The small GTPase Rac1 contributes to extinction of aversive memories of drug withdrawal by facilitating GABAA receptor endocytosis in the vmPFC. J. Neurosci. 37, 7096–7110. doi: 10.1523/jneurosci.3859-16.2017
Ward, M. E., Taubes, A., Chen, R., Miller, B. L., Sephton, C. F., Gelfand, J. M., et al. (2014). Early retinal neurodegeneration and impaired Ran-mediated nuclear import of TDP-43 in progranulin-deficient FTLD. J. Exp. Med. 211, 1937–1945. doi: 10.1084/jem.20140214
Watabe-Uchida, M., Govek, E. E., and Van Aelst, L. (2006). Regulators of Rho GTPases in neuronal development. J. Neurosci. 26, 10633–10635. doi: 10.1523/jneurosci.4084-06.2006
Weis, K. (2003). Regulating access to the genome: nucleocytoplasmic transport throughout the cell cycle. Cell 112, 441–451. doi: 10.1016/s0092-8674(03)00082-5
Wennerberg, K., Rossman, K. L., and Der, C. J. (2005). The Ras superfamily at a glance. J. Cell Sci. 118, 843–846. doi: 10.1242/jcs.01660
Williams, V. C., Lucas, J., Babcock, M. A., Gutmann, D. H., Korf, B., and Maria, B. L. (2009). Neurofibromatosis type 1 revisited. Pediatrics 123, 124–133.
Wilson, G. R., Sim, J. C., Mclean, C., Giannandrea, M., Galea, C. A., Riseley, J. R., et al. (2014). Mutations in RAB39B cause X-linked intellectual disability and early-onset Parkinson disease with alpha-synuclein pathology. Am. J. Hum. Genet. 95, 729–735. doi: 10.1016/j.ajhg.2014.10.015
Xiao, F., He, M., Wang, X. F., Xi, Z. Q., Li, J. M., Wu, Y., et al. (2007). [Overexpression of the Cdc42 in the brain tissue of human with intractable temporal epilepsy]. Zhonghua Yi Xue Za Zhi 87, 2030–2032.
Xu, W., Tan, L., and Yu, J. T. (2015). The role of PICALM in Alzheimer’s disease. Mol. Neurobiol. 52, 399–413. doi: 10.1007/s12035-014-8878-3
Yan, T., Wang, L., Gao, J., Siedlak, S. L., Huntley, M. L., Termsarasab, P., et al. (2018). Rab10 phosphorylation is a prominent pathological feature in Alzheimer’s disease. J. Alzheimers Dis. 63, 157–165. doi: 10.3233/jad-180023
Yaoita, M., Niihori, T., Mizuno, S., Okamoto, N., Hayashi, S., Watanabe, A., et al. (2016). Spectrum of mutations and genotype-phenotype analysis in Noonan syndrome patients with RIT1 mutations. Hum. Genet. 135, 209–222. doi: 10.1007/s00439-015-1627-5
Ye, X., and Carew, T. J. (2010). Small G protein signaling in neuronal plasticity and memory formation: the specific role of ras family proteins. Neuron 68, 340–361. doi: 10.1016/j.neuron.2010.09.013
Yin, J. C., Platt, M. J., Tian, X., Wu, X., Backx, P. H., Simpson, J. A., et al. (2017). Cellular interplay via cytokine hierarchy causes pathological cardiac hypertrophy in RAF1-mutant Noonan syndrome. Nat. Commun. 8:15518. doi: 10.1038/ncomms15518
Yoon, G., Rosenberg, J., Blaser, S., and Rauen, K. A. (2007). Neurological complications of cardio-facio-cutaneous syndrome. Dev. Med. Child Neurol. 49, 894–899. doi: 10.1111/j.1469-8749.2007.00894.x
Young, L. C., Hartig, N., Boned Del Rio, I., Sari, S., Ringham-Terry, B., Wainwright, J. R., et al. (2018). SHOC2-MRAS-PP1 complex positively regulates RAF activity and contributes to Noonan syndrome pathogenesis. Proc. Natl. Acad. Sci. U.S.A. 115, E10576–E10585.
Young, L. C., Hartig, N., Munoz-Alegre, M., Oses-Prieto, J. A., Durdu, S., Bender, S., et al. (2013). An MRAS, SHOC2, and SCRIB complex coordinates ERK pathway activation with polarity and tumorigenic growth. Mol. Cell 52, 679–692. doi: 10.1016/j.molcel.2013.10.004
Zafar, S., Schmitz, M., Younus, N., Tahir, W., Shafiq, M., Llorens, F., et al. (2015). Creutzfeldt-jakob disease subtype-specific regional and temporal regulation of ADP ribosylation factor-1-dependent Rho/MLC pathway at pre-clinical stage. J. Mol. Neurosci. 56, 329–348. doi: 10.1007/s12031-015-0544-3
Zafar, S., Younas, N., Correia, S., Shafiq, M., Tahir, W., Schmitz, M., et al. (2017). Strain-specific altered regulatory response of Rab7a and tau in creutzfeldt-jakob disease and Alzheimer’s disease. Mol. Neurobiol. 54, 697–709. doi: 10.1007/s12035-016-9694-8
Zago, G., Biondini, M., Camonis, J., and Parrini, M. C. (2017). A family affair: a Ral-exocyst-centered network links Ras, Rac, Rho signaling to control cell migration. Small GTPases doi: 10.1080/21541248.2017 [Epub ahead of print].
Zamboni, V., Jones, R., Umbach, A., Ammoni, A., Passafaro, M., Hirsch, E., et al. (2018). Rho GTPases in intellectual disability: from genetics to therapeutic opportunities. Int. J. Mol. Sci. 19:E1821.
Zhai, J., Zhang, L., Mojsilovic-Petrovic, J., Jian, X., Thomas, J., Homma, K., et al. (2015). Inhibition of cytohesins protects against genetic models of motor neuron disease. J. Neurosci. 35, 9088–9105. doi: 10.1523/jneurosci.5032-13.2015
Zhang, H., Hanke-Gogokhia, C., Jiang, L., Li, X., Wang, P., Gerstner, C. D., et al. (2015). Mistrafficking of prenylated proteins causes retinitis pigmentosa 2. FASEB J. 29, 932–942. doi: 10.1096/fj.14-257915
Zhang, Y., Liu, J., Luan, G., and Wang, X. (2015). Inhibition of the small GTPase Cdc42 in regulation of epileptic-seizure in rats. Neuroscience 289, 381–391. doi: 10.1016/j.neuroscience.2014.12.059
Zhang, H., Petit, G. H., Gaughwin, P. M., Hansen, C., Ranganathan, S., Zuo, X., et al. (2013). NGF rescues hippocampal cholinergic neuronal markers, restores neurogenesis, and improves the spatial working memory in a mouse model of Huntington’s disease. J. Huntingtons Dis. 2, 69–82.
Zhang, L., Zhang, P., Wang, G., Zhang, H., Zhang, Y., Yu, Y., et al. (2018). Ras and Rap signal bidirectional synaptic plasticity via distinct subcellular microdomains. Neuron 98, 783–800.e4. doi: 10.1016/j.neuron.2018.03.049
Zhang, X., Huang, T. Y., Yancey, J., Luo, H., and Zhang, Y. W. (2018). Role of Rab GTPases in Alzheimer’s disease. ACS Chem. Neurosci. 10, 828–838.
Zhao, Z., Sagare, A. P., Ma, Q., Halliday, M. R., Kong, P., Kisler, K., et al. (2015). Central role for PICALM in amyloid-beta blood-brain barrier transcytosis and clearance. Nat. Neurosci. 18, 978–987. doi: 10.1038/nn.4025
Zheng, X., Liang, Y., He, Q., Yao, R., Bao, W., Bao, L., et al. (2014). Current models of mammalian target of rapamycin complex 1 (mTORC1) activation by growth factors and amino acids. Int. J. Mol. Sci. 15, 20753–20769. doi: 10.3390/ijms151120753
Zhu, W., Shi, D. S., Winter, J. M., Rich, B. E., Tong, Z., Sorensen, L. K., et al. (2017). Small GTPase ARF6 controls VEGFR2 trafficking and signaling in diabetic retinopathy. J. Clin. Invest. 127, 4569–4582. doi: 10.1172/jci91770
Zhuo, C., Hou, W., Lin, C., Hu, L., and Li, J. (2017). Potential value of genomic copy number variations in schizophrenia. Front. Mol. Neurosci. 10:204. doi: 10.3389/fnmol.2017.00204
Keywords: small GTPases, Ras superfamily, Rho subfamily, Rab subfamily, Arf subfamily, Ran subfamily, non-neoplastic cerebral diseases
Citation: Qu L, Pan C, He S-M, Lang B, Gao G-D, Wang X-L and Wang Y (2019) The Ras Superfamily of Small GTPases in Non-neoplastic Cerebral Diseases. Front. Mol. Neurosci. 12:121. doi: 10.3389/fnmol.2019.00121
Received: 29 January 2019; Accepted: 25 April 2019;
Published: 21 May 2019.
Edited by:
Jean-Marc Taymans, Institut National de la Santé et de la Recherche Médicale (INSERM), FranceReviewed by:
Michael E. Cahill, University of Wisconsin–Madison, United StatesSilvia Bassani, Institute of Neuroscience (IN), Italy
Copyright © 2019 Qu, Pan, He, Lang, Gao, Wang and Wang. This is an open-access article distributed under the terms of the Creative Commons Attribution License (CC BY). The use, distribution or reproduction in other forums is permitted, provided the original author(s) and the copyright owner(s) are credited and that the original publication in this journal is cited, in accordance with accepted academic practice. No use, distribution or reproduction is permitted which does not comply with these terms.
*Correspondence: Xue-Lian Wang, eHVlbGlhbndhbmczQDEyNi5jb20= Yuan Wang, YmxhZGVydW5uZXJ3YW5nQGdtYWlsLmNvbQ==
†These authors have contributed equally to this work