- 1Department of Biomedical Engineering, National University of Singapore, Singapore, Singapore
- 2Singapore Institute for Neurotechnology (SINAPSE), National University of Singapore, Singapore, Singapore
- 3NUS Tissue Engineering Program, National University of Singapore, Singapore, Singapore
- 4Biomedical Institute for Global Health, Research and Technology, Singapore, Singapore
Neural crest cells (NCCs) are a multipotent and migratory cell population in the developing embryo that contribute to the formation of a wide range of tissues. Defects in the development, differentiation and migration of NCCs give rise to a class of syndromes and diseases that are known as neurocristopathies. NCC development has historically been studied in a variety of animal models, including xenopus, chick and mouse. In the recent years, there have been efforts to study NCC development and disease in human specific models, with protocols being established to derive NCCs from human pluripotent stem cells (hPSCs), and to further differentiate these NCCs to neural, mesenchymal and other lineages. These in vitro differentiation platforms are a valuable tool to gain a better understanding of the molecular mechanisms involved in human neural crest development. The use of induced pluripotent stem cells (iPSCs) derived from patients afflicted with neurocristopathies has also enabled the study of defective human NCC development using these in vitro platforms. Here, we review the various in vitro strategies that have been used to derive NCCs from hPSCs and to specify NCCs into cranial, trunk, and vagal subpopulations and their derivatives. We will also discuss the potential applications of these human specific NCC platforms, including the use of iPSCs for disease modeling and the potential of NCCs for future regenerative applications.
Introduction
Neural crest cells (NCCs) are transient, migratory stem cells that originate from the neural tube and migrate to different embryonic tissues to give rise to a wide variety of cell types (Le Douarin et al., 2004). They form ectodermal derivatives, such as sensory and enteric neurons, Schwann cells, as well as mesenchymal derivatives (Le Douarin and Dupin, 2003). Thus, NCCs have been widely studied in animal models to elucidate their role in a range of neurocristopathies involving the craniofacial skeleton and the peripheral nervous system. The advent of techniques for the derivation of human neural crest cells (hNCCs) from human pluripotent stem cells (hPSCs), has not only enabled the understanding of NCC development and disease in a human-specific context but also opened new opportunities for therapeutic applications. This review will highlight the state-of-the-art protocols used to derive hNCCs from hPSCs and discuss opportunities and challenges in the applications of hNCCs in disease modeling and tissue regeneration (Figure 1).
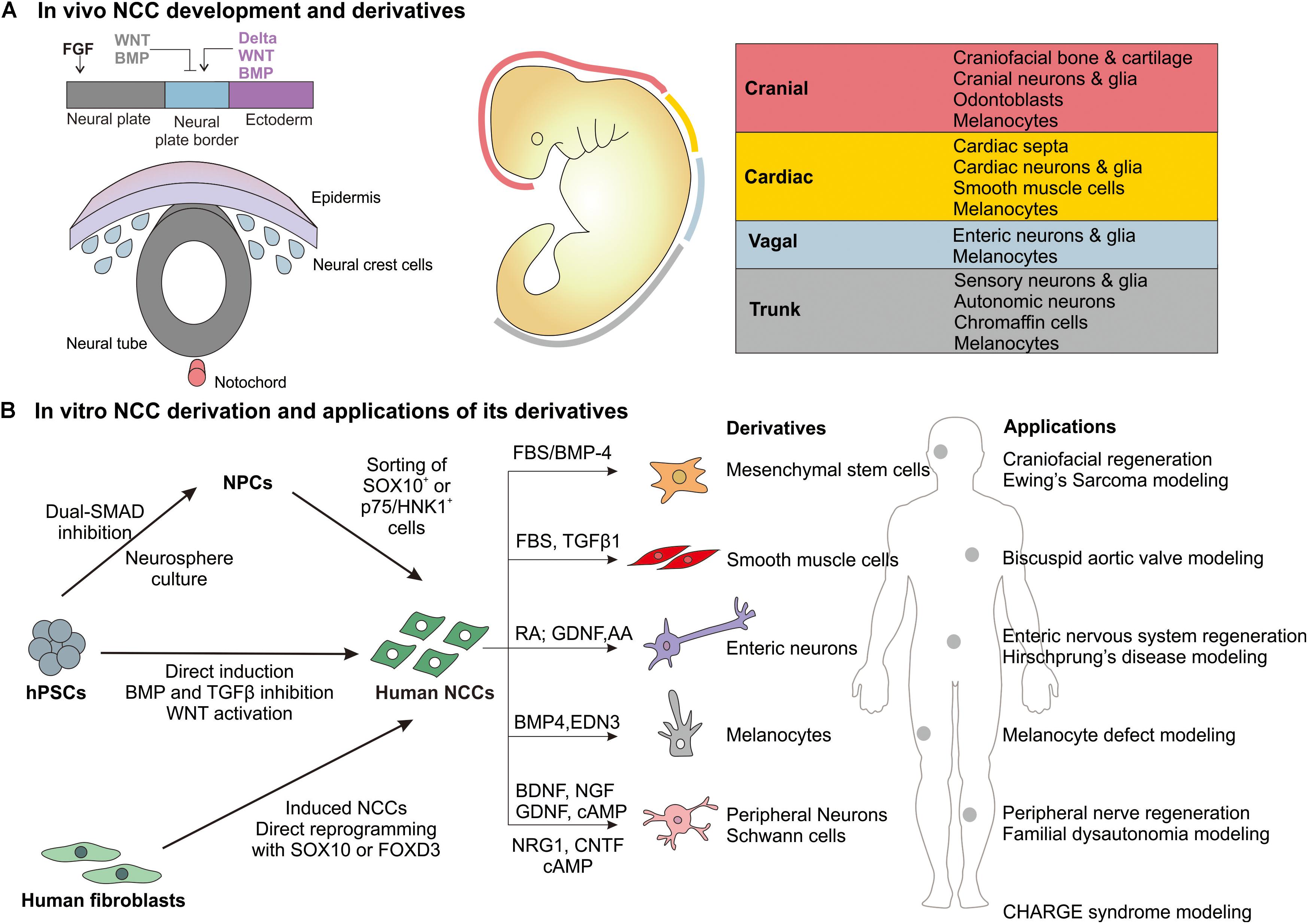
Figure 1. Overview of in vivo NCC development and derivatives and in vitro derivation of human NCCs and major applications. (A) During gastrulation, the neural plate border is specified by BMP, WNT, FGF, and Notch/Delta signaling from the surrounding neural plate, non-neural ectoderm and mesoderm. NCCs are specified at the neural plate border region and then reside in the dorsal portion of the neural tube. Following neural tube closure, they undergo an epithelial-mesenchymal transition and migrate along the anterior-posterior axis of the embryo to give rise to different derivatives based on the region (cranial, cardiac, vagal, or trunk) (Milet and Monsoro-Burq, 2012b; Simoes-Costa and Bronner, 2015; Gandhi and Bronner, 2018). (B) The major approaches by which human NCCs are derived in vitro from hPSCs and differentiated to selected derivatives. The potential applications of these derivatives in regenerative medicine and disease modeling.
Derivation of Human NCCs and Its Derivatives From hPSCs
During embryonic development, NCCs are specified at the neural plate border (NPB) region before they undergo an epithelial-mesenchymal transition and migrate out of the neural tube (Figure 1A). It has been shown that NCC induction at the NPB relies on BMP, WNT, Notch/Delta, and FGF signaling emanating from the surrounding embryonic tissues (Rogers et al., 2012). Based on these molecular developmental programs, researchers have developed increasingly specific and efficient protocols to derive an NCC population from hPSCs in vitro as highlighted below.
Directed NCC Induction Using Small Molecules
Since NCCs are specified adjacent to neural plate cells in vivo, early strategies sought to derive a mixed neural precursor cell (NPC) population and enrich the NCC subpopulation. NPC induction methods include stromal cell co-culture (Pomp et al., 2005; Lee et al., 2007; Jiang et al., 2009), neurosphere culture (Brokhman et al., 2008; Bajpai et al., 2010) or defined monolayer induction of neural rosettes using dual-SMAD inhibition of BMP and Activin A/Nodal signaling (Chambers et al., 2009). Limited p75+/HNK-1+ NCCs could be purified from the periphery of these neural rosettes (Lee et al., 2010). These NPC-based protocols were highly inefficient and variable because NCCs represented a small subset of NPCs, and groups used different combinations of NCC markers such as p75, HNK-1, and SOX10 to isolate NCCs (Milet and Monsoro-Burq, 2012a). Also, the role of the stromal cells/cell aggregates/neural rosettes in inducing NCC formation was unclear, limiting their utility in the study of the molecular mechanisms of NCC development.
To overcome these limitations, researchers sought to achieve directed and specific NCC induction in adherent culture using a defined system. NCC development in vivo requires the activation of canonical WNT signaling and well as intermediate levels of BMP signaling, after an initial inhibition to establish neural plate identity (Pla and Monsoro-Burq, 2018). Mimicking this, researchers used combinations of small molecule activators of WNT signaling and inhibitors of BMP and Activin/Nodal signaling to achieve directed NCC differentiation from both hESCs and iPSCs (Menendez et al., 2011, 2013; Mica et al., 2013). While Mica et al. found a short pulse of BMP inhibition to be essential for NCC specification, Menendez et al. found it unnecessary in their hPSC culture system. This controversy on the requirement of BMP inhibition for NCC specification was addressed by Hackland et al., who demonstrated that the levels of endogenous BMP signaling differ across various hPSC cell lines and in vitro culture systems. They could achieve the precise intermediate BMP level required for robust NCC derivation by a top-down inhibition of BMP signaling in a completely defined culture system (Hackland et al., 2017). The directed induction approach is the most commonly used method to derive NCCs from hPSCs, and can generally achieve a high derivation efficiency, ranging from 40 to 90%. However, different groups employ different markers (e.g., SOX10+ or p75+/HNK1+) to identify NCCs and may not be isolating identical NCC populations.
Transcription Factor-Based Reprogramming
Neural crest cells have also been directly induced from fibroblasts by reprogramming with a single transcription factor SOX10, in the presence of environmental cues including a WNT activator (Kim et al., 2014). This system could be used to derive induced NCCs (iNCCs) directly from human patient fibroblasts. Direct reprogramming from fibroblasts allows for the generation of patient-specific NCCs as well as potentially reducing the length of in vitro culture before the iNCCs can be administered clinically. Another method to induce NCCs from fibroblasts involves the use of a chitosan substrate to effect the gene transfer of FOXD3, leading to increased NCC marker expression and the ability to rescue impaired neural function in a zebrafish model (Tseng et al., 2016). While this system provides a non-viral alternative for reprogramming to iNCCs, the exact nature of the induced cells was not fully defined due to incomplete characterization and lack of in vitro differentiation studies. Further work is required to reprogram iNCCs using non-viral methods to enable their use in clinical applications.
Regional Specification of Derived NCCs
Neural crest cells arise from four distinct regions of the anterior-posterior axis of the neural tube: cranial, cardiac, trunk, and vagal (Achilleos and Trainor, 2012). These NCC sub-populations express specific markers and have distinct differentiation potentials (Figure 1A). The dual-SMAD inhibition/WNT activation protocols tend to derive a HOX-negative NCC population that is disposed toward anterior (cranial) over posterior (vagal) identity (Mica et al., 2013). While early inhibition of BMP signaling is necessary to establish NCC identity, late supplementation of BMP-4 during NCC derivation can enhance cranial identity, as indicated by the upregulation of cranial-specific DLX genes (Mimura et al., 2016). These cranial NCCs also expressed pharyngeal mesenchymal genes and showed osteogenic and chondrogenic differentiation potential in vitro. This can be advantageous as a defined culture system to generate mesenchymal derivatives from hNCCs as compared to the use of undefined serum to drive NCCs into mesenchymal lineages.
Retinoic acid (RA) and FGF-2 are known to be caudalizing factors during neural development (Stuhlmiller and García-Castro, 2012), and thus were utilized to posteriorize hPSC-derived NCCs as well. Vagal NCCs could be specified by the addition of RA or FGF-2 to a NC induction protocol (Mica et al., 2013). Multiple studies then showed that RA could posteriorize hPSC-derived NCCs to enteric NCCs (ENCCs), which expressed vagal-specific HOXB genes and could differentiate into various enteric neuron subtypes (Fattahi et al., 2016; Schlieve et al., 2017). Sequential treatment with RA and BMP could generate PHOX2B+ trunk NCCs, which could differentiate into sympathoadrenal cells (Huang et al., 2016). This mimicked the in vivo induction of sympathoadrenal trunk NCCs by BMP signaling from the dorsal aorta (Saito et al., 2012). The emergence of these protocols to define regional NCC identity will be useful in determining intermediate cell populations during differentiation to NCC derivatives. Currently, it appears that many groups use either FBS/BMP-4 or RA to direct anterior or posterior NCC specification before differentiating NCCs to end-point derivatives (Figure 1B) using protocols optimized for other cell types. Widespread adoption and further development of defined protocols to drive and characterize region-specific NCCs will be beneficial to create more defined culture systems. This will enable the future use of NCC derivatives for tissue regeneration applications.
Applications of hPSC-Derived NCCs for Disease Modeling
Human-specific NCCs provide an invaluable resource to complement animal models in the understanding of neurocristopathies. Therefore, a major application of hPSC-derived NCCs to date is in the modeling of various neurocristopathies (Table 1). The following section highlights notable neurocristopathies whereby the generation of either patient-specific iPSC-derived NCCs or genetically modified hESCs bearing specific genetic mutations have been used to mimic clinically relevant NCC dysfunctions and discover novel molecular mediators.
CHARGE Syndrome
CHARGE syndrome is an acronym for a complex combination of congenital abnormalities including malformations of the craniofacial skeleton, peripheral nervous system, eyes, ears, and heart. It is often associated with mutations in the CHD7 gene, which is postulated to cause NCC dysfunction (Sanlaville and Verloes, 2007). CHD7 knockdown in hESCs affects the formation of multipotent, migratory NCCs by diminishing the expression of NC specifiers TWIST1, SOX9, and SLUG (Bajpai et al., 2010). Another study derived NCCs from CHARGE patient-derived iPSCs, which also showed defective delamination, migration and motility in vitro and defective migration in vivo when implanted into a chick embryo (Okuno et al., 2017). Therefore, CHD7 mutations in CHARGE syndrome result in defects in NCC migration, although the underpinning molecular mechanism remains to be elucidated.
Ewing’s Sarcoma
Ewing’s sarcoma family tumors (ESFT) are common malignant bone and soft tissue tumors, whose genetic hallmark involves the expression of an EWS-FLI1 fusion gene due to chromosome translocation (Paulussen et al., 2001). While their cellular origin remains elusive, one popular hypothesis implicates NCCs as the source of these cells (Tu et al., 2017). Von Levetzow et al. demonstrated that hESC-derived NCCs and NCC-derived MSCs were permissive for EWS-FLI using lentiviral transduction. Expression of EWS-FLI1 pushed NCC-derived MSCs to a more primitive NCC state and led to the loss of cellular senescence and repression of p16 (von Levetzow et al., 2011). They found that ESFT are genetically closely related to NCCs, supporting the hypothesis that some malignant ESFT cells may develop from NCC-derived cells. The use of this hESC-derived NCC model also helped to delineate the mechanism of oncogene tolerance in these cells.
Hirschsprung’s Disease
Hirschsprung’s disease (HSCR) is caused by the defective migration of ENCCs in the gut, leading to loss of peristaltic activity, causing bowel obstruction and megacolon. The severity of the phenotype is determined by the length of the aganglionic segment- short (S-HSCR), long (L-HSCR) or total colonic aganglionosis (Amiel et al., 2008). While HSCR is genetically heterogeneous, mutations in the receptor tyrosine kinase RET are implicated in many cases. Lai et al. generated iPSCs from HSCR patients as well as CRISPR-Cas9 edited RET mutant iPSC lines, and demonstrated that both HSCR and RET-mutant ENCCs showed defective neuronal differentiation and migration compared to control ENCCs (Lai et al., 2017). They identified a novel mutation in the vinculin gene associated with S-HSCR, and corrected this mutation using CRISPR/Cas9 to restore ENCC function. This study demonstrates the great potential of hPSC-based in vitro assays to identify novel disease-associated mutations with high power. This will be useful in the study of HSCR as its genetic etiology is still not completely known.
Familial Dysautonomia
Familial dysautonomia (FD) is a rare but fatal, hereditary sensory and autonomic neuropathy usually caused due to a point mutation in the IKBKAP gene (Slaugenhaupt et al., 2001). FD is known to affect NCCs and cause degeneration of peripheral neurons. Lee et al. first reprogrammed iPSCs from FD patient fibroblasts and differentiated them to NCCs, while Kim et al. directly reprogrammed FD patient fibroblasts to iNCCs by SOX10 overexpression (Lee et al., 2009; Kim et al., 2014). In both studies, FD NCCs showed lower levels of normal IKBKAP transcripts, reduced migration and lower neuronal differentiation efficiency compared to control NCCs. Lee et al. went on to identify kinetin as a candidate drug to rescue aberrant IKBKAP splicing, while Kim et al. shed light on a previously unknown aspect of FD pathogenesis- aberrant splicing in other genes such as PAX3 and MEF2C in FD iNCCs.
Hermansky-Pudlak Syndrome and Chediak-Higashi Syndrome
Pigment producing melanocytes in the skin arise from NCCs during development. Mica et al. developed a protocol involving timed exposure to WNTs, BMPs, and EDN3s for the sequential specification of NCCs, melanoblasts and mature melanocytes (Mica et al., 2013). They then derived iPSCs from patients with Hermansky-Pudlak syndrome (HP) and Chediak-Higashi syndrome (CH), both of which cause defects in melanocyte vesicle formation and trafficking. Melanocytes derived from HP and CH NCCs showed different degrees of pigmentation loss and reduction in melanosome number and size, corresponding to the expected disease phenotype.
Applications of hPSC-Derived NCCs in Regenerative Medicine
Due to their wide differentiation potential, unlimited numbers, and developmental relevance to many tissues, hPSC-derived NCCs are a promising stem cell source for tissue regeneration and as therapies for neurocristopathies. iPSC-derived NCCs are a potentially autologous cell source that can overcome immune-compatibility issues. The preliminary investigations that have been done to assess the regenerative potential of hPSC-derived NCCs are discussed below (Table 1).
Bone, Cartilage, and Tendon
Currently, mesenchymal stem cells (MSCs) derived from bone marrow and adipose tissues are the paradigm cell source for the regeneration of craniofacial bone and cartilage (Yamano et al., 2012; Tollemar et al., 2016). However, since a significant portion of craniofacial mesenchymal tissues originate from NCCs, hPSC-derived NCCs are a promising alternative cell source for craniofacial bone and cartilage tissue engineering. hPSC-derived NCCs can be induced into MSCs either by fetal bovine serum (FBS) or by BMP-4 treatment (Mimura et al., 2016). Although FBS treatment is more prevalent, the use of undefined serum in MSC induction medium will limit future clinical applications. Mechanical cues such as substrate stiffness have recently been shown to modulate the differentiation potential of NCC-derived MSCs (Srinivasan et al., 2018). To date, only a handful of studies have evaluated the regenerative potential of NCC-derived MSCs. Chijimatsu et al. showed that although MSC-like cells differentiated from iPSC-derived NCCs showed chondrogenic ability in vitro, they had very limited repair efficiency in a rat osteochondral defect model (Chijimatsu et al., 2017). On the other hand, Xu et al. demonstrated that iPSC-derived NCCs exhibited enhanced tendon healing compared to the acellular control group in a rat patellar tendon defect model (Xu et al., 2013). Such differences in the regenerative efficiencies of NCC-derived MSCs are likely due to variations in the differentiation protocols for NCCs and MSCs as well as the choice of defect models. In both studies, the use of a single marker p75 to isolate NCCs is problematic, as studies have shown that p75 is not exclusive to NCCs and is widely expressed in the embryonic tissues (Betters et al., 2010). Further work is required to develop chemically defined protocols to drive human NCCs into different mesenchymal lineages, and comprehensively benchmark their regenerative potential to mesodermal MSC sources.
Enteric Nervous System
As the enteric nervous system (ENS) is derived from the neural crest (Iwashita et al., 2003), NCC-derived enteric neurons are an obvious choice of cell source for ENS regeneration. ENCC precursors derived from hPSCs were able to colonize postnatal and adult mouse colons upon in vivo engraftment and showed extensive migration (Fattahi et al., 2016). The ENCCs were also able to rescue disease-related mortality in a genetic mouse model of HSCR (Edrnbs-l/s-l mice). Two recent studies demonstrated the use of hPSC-derived ENCCs to populate human intestinal organoids with an ENS (Schlieve et al., 2017; Workman et al., 2017). Schlieve et al. demonstrated that the implantation of ENCCs into their tissue-engineered small intestine derived from human intestinal organoids (HIO-TESI) led to the repopulation of an ENS in the HIO-TESI system and the establishment of neuron-dependent motility. Another study showed that upon transplantation of NCC spheres into E5 chick embryonic hindgut, they showed ganglial organization within submucosal and myenteric regions and longitudinal migration (Li et al., 2018). Taken together, these studies suggest that hPSC-derived ENCCs are a promising cell source for treating human ENS disorders.
Peripheral Nerves
Peripheral nerve regeneration using primary Schwann cells is very difficult due to limited cell numbers, long culturing times and invasive harvesting techniques (Walsh and Midha, 2009). Thus, stem cell sources such as NCCs that can differentiate into Schwann cells have emerged as a promising alternative. Multiple studies have demonstrated the ability of NCCs to differentiate into Schwann cells and repair peripheral nerve defects when implanted as a nerve graft including a scaffold. Using rat or mice sciatic nerve injury models, these studies showed that grafted NCCs survived and promoted axonal regeneration in the artificial nerve conduits (Du et al., 2018; Jones et al., 2018; Kimura et al., 2018). While Jones et al. derived NCCs from hESCs that were only p75+, Kimura et al. derived a LNGFR+ (p75), THY1+ (CD90, a common MSC marker) NCC population from iPSCs, likely selecting for an MSC sub-population. Thus, the different markers used in these studies probably led to the isolation of two different cell populations, which may affect regeneration via different mechanisms. The in vitro culture duration of hPSC-derived NCCs, as indicated by the passage number, is also found to impact of their therapeutic efficacy as indicated by Schwann cell differentiation, survival and axonal growth (Du et al., 2018). This finite expansion window presents a practical constraint in the application of hPSC-derived NCCs in peripheral neuronal regeneration applications.
Conclusion and Future Perspectives
This extensive body of work to induce the formation of NCCs and its derivatives in vitro has enabled the use of hPSC-derived NCCs for applications including disease modeling and tissue regeneration. Studies involving patient-derived and genetically modified NCCs have already broadened our understanding of NCC development and disease. The use of iNCCs reprogrammed directly from patient fibroblasts will likely advance this process further. So far, most of these studies have used 2-D monolayer culture systems. As signaling from surrounding tissues is so critical in NCC development in vivo, the development of 3-D organotypic models containing multiple cell types would better replicate the in vivo environment. This will help us gain a better understanding of NCC disease development in human-specific models. Moving forward, the use of human NCC-based models to test developmental toxicity and screen for possible human teratogens is a likely prospect. This can be enabled by the development of scalable, cost-effective and biomimetic models of NCC development.
The preliminary studies on the use of hPSC-derived NCCs for tissue regeneration show the great promise of these cells due to their wide differentiation potential and large cell numbers. However, more defined differentiation regimes optimized for specifying NCCs into specific lineages are needed to reliably produce purified cell populations. These should be characterized not only by marker expression, but also transplantation to test functional capability. The chief issue that impedes the clinical translation of NCC-derived cells for regeneration is the safety concern with the use of hPSC-derived cells. While a few studies have shown that the implantation of hPSC-derived NCCs in animal models did not cause teratoma formation (Wang et al., 2011; Chijimatsu et al., 2017), thorough strategies to prevent uncontrolled proliferation are required to prevent any risk. Also, there are still unanswered questions regarding the relevance of a cell source’s developmental origin in regenerative medicine. It remains to be seen whether, for example, the use of developmentally relevant NCC-derived MSCs for craniofacial regeneration improves the therapeutic efficacy over other MSC sources, such as mesodermal derived bone marrow MSCs.
Author Contributions
AS and Y-CT conceived and wrote this manuscript.
Funding
This work was supported by National University of Singapore (R-397-000-192-133, R-397-000-211-133, R-397-000-299-114, and R-397-000-242-112), Singapore Ministry of Education (R-397-000-215-112), and Singapore Institute for Neurotechnology (R-719-004-100-305).
Conflict of Interest Statement
The authors declare that the research was conducted in the absence of any commercial or financial relationships that could be construed as a potential conflict of interest.
References
Achilleos, A., and Trainor, P. A. (2012). Neural crest stem cells: discovery, properties and potential for therapy. Cell Res. 22, 288–304. doi: 10.1038/cr.2012.11
Amiel, J., Sproat-Emison, E., Garcia-Barcelo, M., Lantieri, F., Burzynski, G., Borrego, S., et al. (2008). Hirschsprung disease, associated syndromes and genetics: a review. J. Med. Genet. 45, 1–14.
Bajpai, R., Chen, D. A., Rada-Iglesias, A., Zhang, J., Xiong, Y., Helms, J., et al. (2010). CHD7 cooperates with PBAF to control multipotent neural crest formation. Nature 463, 958–962. doi: 10.1038/nature08733
Betters, E., Liu, Y., Kjaeldgaard, A., Sundstrom, E., and Garcia-Castro, M. I. (2010). Analysis of early human neural crest development. Dev. Biol. 344, 578–592. doi: 10.1016/j.ydbio.2010.05.012
Brokhman, I., Gamarnik-Ziegler, L., Pomp, O., Aharonowiz, M., Reubinoff, B. E., and Goldstein, R. S. (2008). Peripheral sensory neurons differentiate from neural precursors derived from human embryonic stem cells. Differentiation 76, 145–155.
Chambers, S. M., Fasano, C. A., Papapetrou, E. P., Tomishima, M., Sadelain, M., and Studer, L. (2009). Highly efficient neural conversion of human ES and iPS cells by dual inhibition of SMAD signaling. Nat. Biotechnol. 27, 275–280. doi: 10.1038/nbt.1529
Chijimatsu, R., Ikeya, M., Yasui, Y., Ikeda, Y., Ebina, K., Moriguchi, Y., et al. (2017). Characterization of mesenchymal stem cell-like cells derived from human iPSCs via neural crest development and their application for osteochondral repair. Stem Cells Int. 2017:1960965. doi: 10.1155/2017/1960965
Du, J., Chen, H., Zhou, K., and Jia, X. (2018). Quantitative multimodal evaluation of passaging human neural crest stem cells for peripheral nerve regeneration. Stem Cell Rev. 14, 92–100. doi: 10.1007/s12015-017-9758-9
Fattahi, F., Steinbeck, J. A., Kriks, S., Tchieu, J., Zimmer, B., Kishinevsky, S., et al. (2016). Deriving human ENS lineages for cell therapy and drug discovery in hirschsprung disease. Nature 531, 105–109. doi: 10.1038/nature16951
Gandhi, S., and Bronner, M. E. (2018). Insights into neural crest development from studies of avian embryos. Int. J. Dev. Biol. 62, 183–194. doi: 10.1387/ijdb.180038sg
Hackland, J. O. S., Frith, T. J. R., Thompson, O., Marin Navarro, A., Garcia-Castro, M. I., Unger, C., et al. (2017). Top-down inhibition of BMP signaling enables robust induction of hPSCs into neural crest in fully defined. Xeno-free Conditions. Stem Cell Rep. 9, 1043–1052. doi: 10.1016/j.stemcr.2017.08.008
Huang, M., Miller, M. L., Mchenry, L. K., Zheng, T., Zhen, Q., Ilkhanizadeh, S., et al. (2016). Generating trunk neural crest from human pluripotent stem cells. Sci. Rep. 6:19727. doi: 10.1038/srep19727
Iwashita, T., Kruger, G. M., Pardal, R., Kiel, M. J., and Morrison, S. J. (2003). Hirschsprung disease is linked to defects in neural crest stem cell function. Science 301, 972–976.
Jiang, X., Gwye, Y., Mckeown, S. J., Bronner-Fraser, M., Lutzko, C., and Lawlor, E. R. (2009). Isolation and characterization of neural crest stem cells derived from in vitro-differentiated human embryonic stem cells. Stem Cells Dev. 18, 1059–1070. doi: 10.1089/scd.2008.0362
Jiao, J., Xiong, W., Wang, L., Yang, J., Qiu, P., Hirai, H., et al. (2016). Differentiation defect in neural crest-derived smooth muscle cells in patients with aortopathy associated with bicuspid aortic valves. EBioMedicine 10, 282–290. doi: 10.1016/j.ebiom.2016.06.045
Jones, I., Novikova, L. N., Novikov, L. N., Renardy, M., Ullrich, A., Wiberg, M., et al. (2018). Regenerative effects of human embryonic stem cell-derived neural crest cells for treatment of peripheral nerve injury. J. Tissue Eng. Regen Med. 12, e2099–e2109. doi: 10.1002/term.2642
Kim, Y. J., Lim, H., Li, Z., Oh, Y., Kovlyagina, I., Choi, I. Y., et al. (2014). Generation of multipotent induced neural crest by direct reprogramming of human postnatal fibroblasts with a single transcription factor. Cell Stem Cell 15, 497–506. doi: 10.1016/j.stem.2014.07.013
Kimura, H., Ouchi, T., Shibata, S., Amemiya, T., Nagoshi, N., Nakagawa, T., et al. (2018). Stem cells purified from human induced pluripotent stem cell-derived neural crest-like cells promote peripheral nerve regeneration. Sci. Rep. 8:10071. doi: 10.1038/s41598-018-27952-7
Lai, F. P., Lau, S. T., Wong, J. K., Gui, H., Wang, R. X., Zhou, T., et al. (2017). Correction of hirschsprung-associated mutations in human induced pluripotent stem cells via clustered regularly interspaced short palindromic repeats/Cas9, restores neural crest cell function. Gastroenterology 153, 139–153.e8. doi: 10.1053/j.gastro.2017.03.014
Le Douarin, N. M., Creuzet, S., Couly, G., and Dupin, E. (2004). Neural crest cell plasticity and its limits. Development 131, 4637–4650.
Le Douarin, N. M., and Dupin, E. (2003). Multipotentiality of the neural crest. Curr. Opin. Genet. Dev. 13, 529–536.
Lee, G., Chambers, S. M., Tomishima, M. J., and Studer, L. (2010). Derivation of neural crest cells from human pluripotent stem cells. Nat. Protoc. 5, 688–701. doi: 10.1038/nprot.2010.35
Lee, G., Kim, H., Elkabetz, Y., Al Shamy, G., Panagiotakos, G., Barberi, T., et al. (2007). Isolation and directed differentiation of neural crest stem cells derived from human embryonic stem cells. Nat. Biotechnol. 25, 1468–1475.
Lee, G., Papapetrou, E. P., Kim, H., Chambers, S. M., Tomishima, M. J., Fasano, C. A., et al. (2009). Modelling pathogenesis and treatment of familial dysautonomia using patient-specific iPSCs. Nature 461, 402–406. doi: 10.1038/nature08320
Li, W., Huang, L., Zeng, J., Lin, W., Li, K., Sun, J., et al. (2018). Characterization and transplantation of enteric neural crest cells from human induced pluripotent stem cells. Mol. Psychiatry 23, 499–508. doi: 10.1038/mp.2016.191
Menendez, L., Kulik, M. J., Page, A. T., Park, S. S., Lauderdale, J. D., Cunningham, M. L., et al. (2013). Directed differentiation of human pluripotent cells to neural crest stem cells. Nat. Protoc. 8, 203–212. doi: 10.1038/nprot.2012.156
Menendez, L., Yatskievych, T. A., Antin, P. B., and Dalton, S. (2011). Wnt signaling and a Smad pathway blockade direct the differentiation of human pluripotent stem cells to multipotent neural crest cells. Proc. Natl. Acad. Sci. U.S.A. 108, 19240–19245. doi: 10.1073/pnas.1113746108
Mica, Y., Lee, G., Chambers, S. M., Tomishima, M. J., and Studer, L. (2013). Modeling neural crest induction, melanocyte specification, and disease-related pigmentation defects in hESCs and patient-specific iPSCs. Cell Rep. 3, 1140–1152. doi: 10.1016/j.celrep.2013.03.025
Milet, C., and Monsoro-Burq, A. H. (2012a). Embryonic stem cell strategies to explore neural crest development in human embryos. Dev. Biol. 366, 96–99. doi: 10.1016/j.ydbio.2012.01.016
Milet, C., and Monsoro-Burq, A. H. (2012b). Neural crest induction at the neural plate border in vertebrates. Dev. Biol. 366, 22–33. doi: 10.1016/j.ydbio.2012.01.013
Mimura, S., Suga, M., Okada, K., Kinehara, M., Nikawa, H., and Furue, M. K. (2016). Bone morphogenetic protein 4 promotes craniofacial neural crest induction from human pluripotent stem cells. Int. J. Dev. Biol. 60, 21–28. doi: 10.1387/ijdb.160040mk
Okuno, H., Renault Mihara, F., Ohta, S., Fukuda, K., Kurosawa, K., Akamatsu, W., et al. (2017). CHARGE syndrome modeling using patient-iPSCs reveals defective migration of neural crest cells harboring CHD7 mutations. eLife 6:e21114. doi: 10.7554/eLife.21114
Paulussen, M., Frohlich, B., and Jurgens, H. (2001). Ewing tumour: incidence, prognosis and treatment options. Paediatr. Drugs 3, 899–913.
Pla, P., and Monsoro-Burq, A. H. (2018). The neural border: induction, specification and maturation of the territory that generates neural crest cells. Dev. Biol. doi: 10.1016/j.ydbio.2018.05.018 [Epub ahead of print].
Pomp, O., Brokhman, I., Ben-Dor, I., Reubinoff, B., and Goldstein, R. S. (2005). Generation of peripheral sensory and sympathetic neurons and neural crest cells from human embryonic stem cells. Stem Cells 23, 923–930.
Rogers, C. D., Jayasena, C. S., Nie, S., and Bronner, M. E. (2012). Neural crest specification: tissues, signals, and transcription factors. Wiley Interdiscip. Rev. Dev. Biol. 1, 52–68. doi: 10.1002/wdev.8
Saito, D., Takase, Y., Murai, H., and Takahashi, Y. (2012). The dorsal aorta initiates a molecular cascade that instructs sympatho-adrenal specification. Science 336, 1578–1581. doi: 10.1126/science.1222369
Sanlaville, D., and Verloes, A. (2007). CHARGE syndrome: an update. Eur. J. Hum. Genet. 15, 389–399.
Schlieve, C. R., Fowler, K. L., Thornton, M., Huang, S., Hajjali, I., Hou, X., et al. (2017). Neural crest cell implantation restores enteric nervous system function and alters the gastrointestinal transcriptome in human tissue-engineered small intestine. Stem Cell Rep. 9, 883–896. doi: 10.1016/j.stemcr.2017.07.017
Simoes-Costa, M., and Bronner, M. E. (2015). Establishing neural crest identity: a gene regulatory recipe. Development 142, 242–257. doi: 10.1242/dev.105445
Slaugenhaupt, S. A., Blumenfeld, A., Gill, S. P., Leyne, M., Mull, J., Cuajungco, M. P., et al. (2001). Tissue-specific expression of a splicing mutation in the IKBKAP gene causes familial dysautonomia. Am. J. Hum. Genet. 68, 598–605.
Srinivasan, A., Chang, S. Y., Zhang, S., Toh, W. S., and Toh, Y. C. (2018). Substrate stiffness modulates the multipotency of human neural crest derived ectomesenchymal stem cells via CD44 mediated PDGFR signaling. Biomaterials 167, 153–167. doi: 10.1016/j.biomaterials.2018.03.022
Stuhlmiller, T. J., and García-Castro, M. I. (2012). Current perspectives of the signaling pathways directing neural crest induction. Cell. Mol. Life Sci. 69, 3715–3737. doi: 10.1007/s00018-012-0991-8
Tollemar, V., Collier, Z. J., Mohammed, M. K., Lee, M. J., Ameer, G. A., and Reid, R. R. (2016). Stem cells, growth factors and scaffolds in craniofacial regenerative medicine. Genes Dis. 3, 56–71.
Tseng, T. C., Hsieh, F. Y., Dai, N. T., and Hsu, S. H. (2016). Substrate-mediated reprogramming of human fibroblasts into neural crest stem-like cells and their applications in neural repair. Biomaterials 102, 148–161. doi: 10.1016/j.biomaterials.2016.06.020
Tu, J., Huo, Z., Gingold, J., Zhao, R., Shen, J., and Lee, D.-F. (2017). The Histogenesis of Ewing Sarcoma. Cancer Rep. Rev. 1. doi: 10.15761/CRR.1000111
von Levetzow, C., Jiang, X., Gwye, Y., Von Levetzow, G., Hung, L., Cooper, A., et al. (2011). Modeling initiation of ewing sarcoma in human neural crest cells. PLoS One 6:e19305. doi: 10.1371/journal.pone.0019305
Walsh, S., and Midha, R. (2009). Practical considerations concerning the use of stem cells for peripheral nerve repair. Neurosurg. Focus 26:E2. doi: 10.3171/FOC.2009.26.2.E2
Wang, A., Tang, Z., Park, I.-H., Zhu, Y., Patel, S., Daley, G. Q., et al. (2011). Induced pluripotent stem cells for neural tissue engineering. Biomaterials 32, 5023–5032. doi: 10.1016/j.biomaterials.2011.03.070
Workman, M. J., Mahe, M. M., Trisno, S., Poling, H. M., Watson, C. L., Sundaram, N., et al. (2017). Engineered human pluripotent-stem-cell-derived intestinal tissues with a functional enteric nervous system. Nat. Med. 23, 49–59. doi: 10.1038/nm.4233
Xu, W., Wang, Y., Liu, E., Sun, Y., Luo, Z., Xu, Z., et al. (2013). Human iPSC-derived neural crest stem cells promote tendon repair in a rat patellar tendon window defect model. Tissue Eng. Part A 19, 2439–2451. doi: 10.1089/ten.TEA.2012.0453
Keywords: neural crest, disease model, tissue regeneration, pluripotent stem cell, neurocristopathy
Citation: Srinivasan A and Toh Y-C (2019) Human Pluripotent Stem Cell-Derived Neural Crest Cells for Tissue Regeneration and Disease Modeling. Front. Mol. Neurosci. 12:39. doi: 10.3389/fnmol.2019.00039
Received: 25 October 2018; Accepted: 01 February 2019;
Published: 22 February 2019.
Edited by:
Sabine Wislet, University of Liège, BelgiumReviewed by:
Virginie Neirinckx, Luxembourg Institute of Health (LIH), LuxembourgShyam Gajavelli, University of Miami, United States
Gabriele Zanirati, Pontifícia Universidade Católica do Rio Grande do Sul, Brazil
Copyright © 2019 Srinivasan and Toh. This is an open-access article distributed under the terms of the Creative Commons Attribution License (CC BY). The use, distribution or reproduction in other forums is permitted, provided the original author(s) and the copyright owner(s) are credited and that the original publication in this journal is cited, in accordance with accepted academic practice. No use, distribution or reproduction is permitted which does not comply with these terms.
*Correspondence: Yi-Chin Toh, YmlldHlAbnVzLmVkdS5zZw==