- 1Department of Pharmacology and Pharmacotherapy, Medical School, University of Pécs, Pécs, Hungary
- 2Szentágothai Research Centre and Centre for Neuroscience, University of Pécs, Pécs, Hungary
- 3MTA-PTE Chronic Pain Research Group, Pécs, Hungary
- 4Section of Molecular Medicine, Rush University Medical Center, Chicago, IL, United States
- 5Bioinformatics and Scientific Computing, Vienna Biocenter Core Facilities, Vienna, Austria
Orofacial pain and headache disorders are among the most debilitating pain conditions. While the pathophysiological basis of these disorders may be diverse, it is generally accepted that a common mechanism behind the arising pain is the sensitization of extra- and intracranial trigeminal primary afferents. In the present study we investigated gene expression changes in the trigeminal ganglia (TRG), trigeminal nucleus caudalis (TNC) and peripheral blood mononuclear cells (PBMC) evoked by Complete Freund's Adjuvant (CFA)-induced orofacial inflammation in rats, as a model of trigeminal sensitization. Microarray analysis revealed 512 differentially expressed genes between the ipsi- and contralateral TRG samples 7 days after CFA injection. Time-dependent expression changes of G-protein coupled receptor 39 (Gpr39), kisspeptin-1 receptor (Kiss1r), kisspeptin (Kiss1), as well as synaptic plasticity-associated Lkaaear1 (Lkr) and Neurod2 mRNA were described on the basis of qPCR results. The greatest alterations were observed on day 3 ipsilaterally, when orofacial mechanical allodynia reached its maximum. This corresponded well with patterns of neuronal (Fosb), microglia (Iba1), and astrocyte (Gfap) activation markers in both TRG and TNC, and interestingly also in PBMCs. This is the first description of up- and downregulated genes both in primary and secondary sensory neurones of the trigeminovascular system that might play important roles in neuroinflammatory activation mechanisms. We are the first to show transcriptomic alterations in the PBMCs that are similar to the neuronal changes. These results open new perspectives and initiate further investigations in the research of trigeminal pain disorders.
Introduction
Orofacial pain and headache disorders are among the most debilitating pain conditions. While the pathophysiological basis of these disorders may be diverse, it is generally accepted that a common mechanism behind the arising pain is the sensitization of extra- and intracranial trigeminal primary afferents. The trigeminal nerve provides most of the sensory innervation to the face and oral cavity as well as the meninges where the nociceptive primary afferents are closely associated with the vasculature. The cell bodies of these neurons are located in the trigeminal ganglion (TRG) and their central projections terminate in the trigeminal nucleus caudalis (TNC). It has been described that there is convergence of extra- and intracranial primary afferents in the TNC (Burstein et al., 1998). Sensitization of these secondary nociceptive neurons might be responsible for the phenomenon of the facial allodynia developing in primary headaches (Burstein et al., 2000). A similar mechanism could induce the headache associated with disorders of extracranial structures. Inflammation of the temporal artery, temporomandibular joint, sinuses or orbit can induce headache which could have the same characteristics as the primary disorders. Co-morbidity of migraine and temporomandibular disorders has also been reported (Romero-Reyes and Uyanik, 2014).
Inflammatory pain models adapted to the orofacial area induce trigeminal sensitization and can constitute a possible way to understand the mechanisms of pain associated with orofacial disorders and headaches (Krzyzanowska et al., 2011; Krzyzanowska and Avendaño, 2012; Romero-Reyes et al., 2013). A commonly used model of peripheral inflammation in animals is injection of Complete Freund's Adjuvant (CFA) (Ren and Dubner, 1999; Takeda et al., 2007; Krzyzanowska and Avendaño, 2012; Gregory et al., 2013). Orofacial inflammation induces mechanical hyperalgesia/allodynia on the face by activation/sensitization of trigeminal primary and secondary sensory neurons (Iwata et al., 2017).
Since the mechanisms of trigeminal sensitization are not known, global transcriptomic analysis allows an unbiased approach to reveal key pathways responsible for the pathophysiological changes (Perrino et al., 2017). Gene expression changes in the trigeminal ganglion (TRG) had been assessed by microarray analysis after CFA injection in whisker pad (Okumura et al., 2010) or masseter muscle (Chung et al., 2016). However, no study has evaluated TRG gene expression changes in parallel with the central gene expression variances in the trigeminal nucleus caudalis (TNC) and correlate it with the time course and extent of facial allodynia. This comprehensive approach might facilitate the identification of differentially regulated genes with a relevant role in the cascade of events resulting in the sensitization of primary and secondary trigeminal neurons. Moreover, there is growing evidence that transcriptome changes in the central nervous system could be reflected in peripheral blood cells. Investigation of gene expression changes in migraine patients identified differential expression of major genes from the peripheral blood (Gardiner et al., 1998; Hershey et al., 2004, 2012; Du et al., 2006; Plummer et al., 2011; Gerring et al., 2017). Gene transcription changes of PBMCs have not been analysed in animal models of trigeminal sensitization, although it could provide a good opportunity to compare with human data.
The aim of the present study was to follow the temporal changes of facial mechanonociceptive thresholds and gene expression in TRG, TNC neurones and PBMCs after CFA inflammation using microarray and qPCR analyses in order to get a better insight into the mechanisms of trigeminal pain disorders.
Materials and Methods
Animals
Twenty male Wistar rats (Toxicoop Zrt., Hungary) weighing between 200–300 g were used. Animals were kept under standard light-dark cycle (12-h light/dark cycle) and temperature (24–25°C) conditions, food and water were provided ad libitum, in the local animal house of the Pécs University Department of Pharmacology and Pharmacotherapy. In order to minimise stress, all rats were habituated to handling and the light restraint used for the facial von Frey test for 3 days prior to the start of the experiments.
The study was carried out in accordance with the Ethical Codex of Animal Experiments of the University of Pécs and the 1998/XXVIII Act of the Hungarian Parliament on Animal Protection and Consideration Decree of Scientific Procedures of Animal Experiments (243/1988). The protocol was approved by the local Ethics Committee on Animal Research of University of Pécs (license No.: BA02/2000-9/2011).
CFA Injection
Orofacial inflammation was induced by unilateral s.c. injection of 50 μl complete Freund's adjuvant (CFA; Sigma-Aldrich, Saint Louis, USA; killed mycobacteria suspended in paraffin oil; 1 mg/ml) into the whisker pad of male rats, while under ketamine (72 mg/kg) and xylazine (8 mg/kg) anaesthesia. In the second series of experiments, a control group received the same volume of saline injection.
Microarray
Orofacial inflammation-associated gene expression was analysed using Agilent microarray platforms. Rat TRG tissue samples were collected from animals 7 days after receiving s.c. CFA injection (n = 8). Animals were anaesthetized with thiopental (100 mg/kg i.p.) and sacrificed by exsanguination. TRGs were excised and snap-frozen in liquid nitrogen. Contralateral sides of CFA-injected rats served as controls. Total RNA were isolated from snap-frozen samples using RNeasy Mini Kit (Qiagen, Carlsbad, CA) and high-quality samples (RIN > 8.0) were used for subsequent expression analyses. Sample labelling, array hybridization and primary data analysis was performed by ArrayStar Inc. (Rockville, MD, USA). Briefly, total RNA samples were amplified and labelled with Cy3-dCTP. Labeled amplicons were purified, fragmented and hybridized to rat LncRNA Array v1.0 (4 × 44 K, Arraystar Inc.) slides. One-color microarray-based gene expression analysis was used. After hybridization slides were washed, fixed and scanned. Gene expression data files were deposited to NCBI's Gene Expression Omnibus (Edgar et al., 2002) and are accessible through GEO Series accession number GSE111160.
Orofacial Pain Sensitivity Tested With Von Frey Filaments
In a second experiment, mechanical pain thresholds of the orofacial region were determined with a series of von Frey filaments. Tests were performed on days 0 (control day) before and 1, 3, 7 after CFA (n = 9)/saline (n = 3) injection. Animals were lightly restrained using a soft cotton glove in order to allow an easier habituation, then a set of calibrated nylon monofilaments (Stoelting, Wood Dale, Illinois, U.S.A) was used with increasing strengths (0.8–12 g) to measure facial mechanosensitivity. Filaments were applied in ascending order, starting from the 5.2 g filament during control measurements and the 0.8 g filament after CFA treatment. The mechanonociceptive threshold was defined as the lowest force evoking at least two withdrawal responses (face stroking with the forepaw or head shaking) out of five stimulations.
Experimental Setup of the Second Experiment
At each time point (1, 3, and 7 days) animals (n = 3) were anaesthetized with thiopental and blood was collected by cardiac puncture. Tissue samples (TRG, TNC) were quickly frozen in liquid nitrogen and stored at −80°C until RNA extraction and real-time PCR processing.
Isolation of Peripheral Blood Mononuclear Cells
Mononuclear cells were purified from fresh peripheral blood according to Ficoll-PaquePREMIUM (Cat. No. 17-5446-02, GE Healthcare, Budapest, Hungary) manufacturer's instructions. Fresh anticoagulant-treated blood and an equal volume of balanced salt solution (final volume of 8 ml) were transferred to 15 ml sterile centrifuge tubes. The mixture was carefully overlaid on 5 ml Ficoll-PaquePREMIUM and centrifuged 40 min at 2,100 RPM, 20°C. The mononuclear layer was transferred into a new 15 ml centrifuge tube, suspended with approximately 6 ml of salt solution and centrifuged 15 min at 2300 RPM, 20°C. The supernatant was removed and the pellet was resuspended in another 6 ml of salt solution, followed by another centrifugation (10 min, 2300 RPM, 20°C). After the removal of the supernatant the cells were resuspended with 1 ml of TRI Reagent (Molecular Research Center, Inc., Cincinnati, OH, USA) and transferred to Eppendorf and stored at −80°C until use.
Quantitative Real-Time RT-PCR (qRT-PCR)
Purification of total RNA was carried out according to the TRI Reagent manufacturer's (Molecular Research Center, Inc., Cincinnati, OH, USA) protocol up to the step of acquiring the aqueous phase. Briefly, tissue samples were homogenized in 1 ml of TRI Reagent, and then, 200 μl of bromo-chloro-propane (Sigma-Aldrich, Saint Louis, USA) was added. RNA was purified from the aqueous phase using the Direct-zol RNA MiniPrep kit (Cat. No. R2052; Zymo Research, Irvine, CA, USA) according to the manufacturer's protocol. Briefly, 400 μl of the aqueous phase was mixed with 400 μl absolute ethanol, the mixture was loaded onto the column, washed, and the RNA was eluted in 50 μl of RNase-free water. The quantity and purity of the extracted RNA were assessed on Nanodrop ND-1000 Spectrophotometer V3.5 (Nano-Drop Technologies, Inc., Wilmington, DE, USA). 200 ng of PBMCs/TRG and 250 ng of TNC total RNA was reverse transcribed using Maxima First Strand cDNA Synthesis Kit (Cat. No. K1642, ThermoScientific, Santa Clara, CA, USA) according to the manufacturer's instructions. qRT-PCR was performed on a Stratagene Mx3000P qPCR System (Agilent Technologies, Santa Clara, USA). PCR amplification was performed using SensiFast SYBR Lo-ROX Kit (Cat. No. BIO-94020). Transcripts of the reference genes glyceraldehyde 3-phosphate dehydrogenase (Gapdh), hypoxanthine phosphoribosyltransferase 1 (Hprt1), beta-2-microglobulin (β2m) and Peptidyl-prolyl cis-trans isomerase (Ppia) were detected in all samples. Ppia and Hprt1 for PBMCs and β2m, Hprt1 for TRG and TNC samples were eventually chosen as internal controls, the geometric mean of their Cq values was calculated. Primers of similar efficiencies were used and 2−ΔΔCq fold change values were calculated. Sequences of primers used for qRT-PCR are given in Supplementary Table 1.
Statistical Analysis
The raw microarray data were analysed using R and Bioconductor (Gentleman et al., 2004; R Development Core Team, 2008) The data were quantile normalised to reduce technical noise with Limma package (Ritchie et al., 2015). The statistical testing for differential expression was also performed using Limma, which applies linear modeling with a modified t-test to calculate the p-values and fold change values. One-way analysis of variance (ANOVA) followed by Tukeys' multiple comparison tests on RT-PCR data and in case of mechanical pain threshold detection two-way ANOVA with repeated measures followed by Bonferroni's post-test for time-matching samples were performed using GraphPad Prism software (GraphPad Software, Inc., La Jolla, CA, USA). Probability values p ≤ 0.05 were accepted as significant. Results are presented as the mean ± standard error of the mean (SEM). Log2 mRNA fold change data measured by qPCR were further analysed by hierarchical cluster analysis (1-Pearson correlation and average linkage method) and then visualised by heat map using the free web tool Morpheus (Morpheus)1.
Functional Classification of Differentially Regulated Genes
The functional enrichment analyses against Gene Ontology (GO) (Ashburner et al., 2000; The Gene Ontology Consortium, 2017), KEGG (Kanehisa and Goto, 2000) and Reactome (Fabregat et al., 2018) databases were performed using the topGO (Alexa and Rahnenführer, 2016) and gage (Luo et al., 2009) packages in R.
Result
Microarray Analysis
The microarray data analysis identified 512 differentially expressed (319 up- and 191 downregulated) transcripts between the control (contralateral) and 7-day CFA (ipsilateral) samples from TRG at a statistically significant level (p ≤ 0.05) and with fold change |FC|> 2 (Supplementary Table 2; Supplementary Figures 1, 2). All but 15 of these have absolute fold change values below 4. Original data files have been uploaded to the NCBI GEO Database. The top 25 up- and downregulated genes are included in Table 1. The most upregulated (5.20 fold) transcript was found to be a lncRNA (MRAK049104) with unknown function. The most downregulated transcript (−9.20 fold), Neurod2, is involved in neuronal differentiation. Figure 1 shows the 44 differentially expressed genes at a significance level p ≤ 0.001 and |FC|> 2, including a number of olfactory, taste and pheromone receptors, as well as the chemokine receptor (Ccr7) and the estrogen receptor 1 (Esr1) genes as well as long non-coding RNAs.
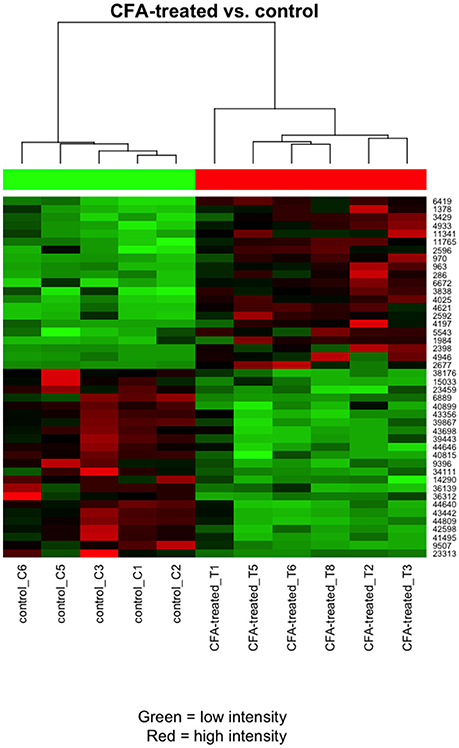
Figure 1. Heat map clustering of the most differentially expressed transcripts for the comparison between CFA-treated and contralateral side TRG samples. Pearson's metrics has been used in hierarchical clustering of the samples and filtered features. The clustering is based on the general expression measurement similarity. Red colour means high expression and green low expression. Each row represents one differentially expressed (DE) feature (microarray feature identifiers) and each column represents one sample.
Gene Ontology
Gene set enrichment analysis was performed on the microarray data to find common features of genes. The most differentially expressed genes (|FC|>2, p ≤ 0.001) between the control (contralateral) and 7-day CFA (ipsilateral) samples from TRG were functionally annotated based on gene ontology (GO), KEGG Pathway and Reactome terms to gain an overview of the affected biological processes and pathways (Table 2). The identified enriched GO terms include steroid and carbohydrate metabolism, sensory perception and olfactory transduction.
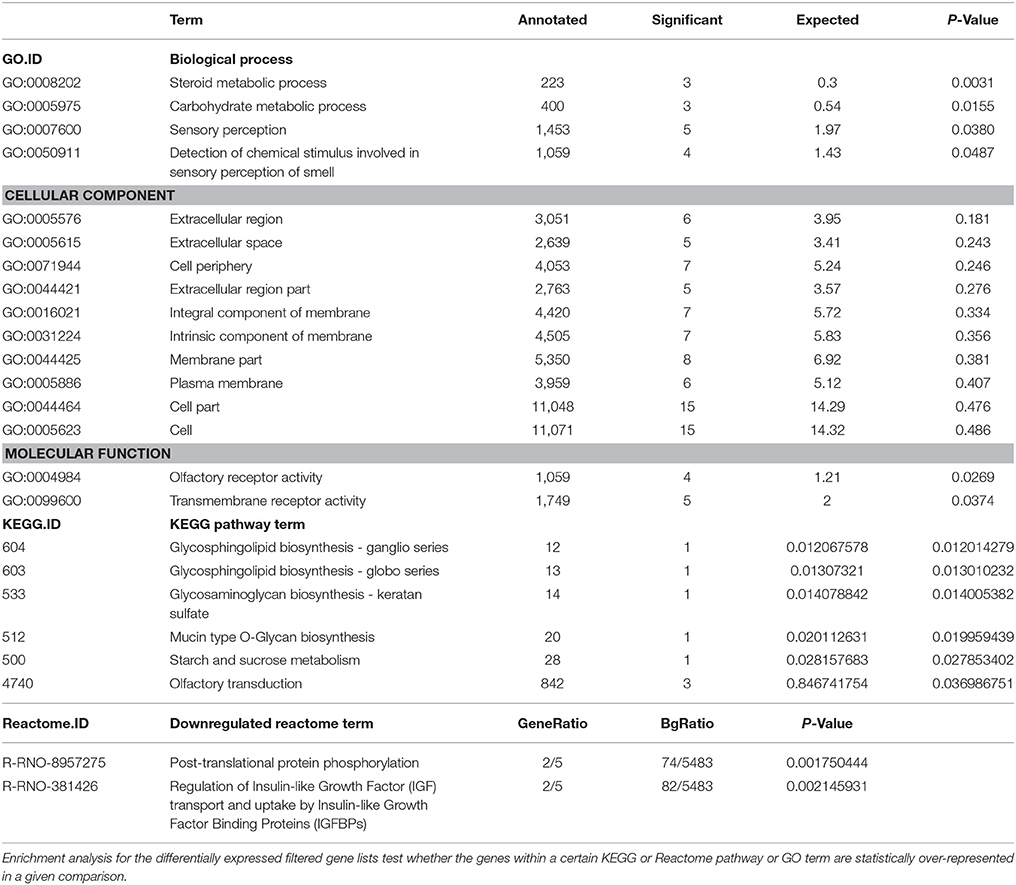
Table 2. Results of gene set enrichment analysis of a subset of genes differentially expressed between the control (contralateral) and 7-day CFA (ipsilateral) samples from TRG as detected by microarray.
Mechanonociceptive Threshold
The facial mechanonociceptive threshold of CFA-injected rats was significantly decreased compared to the contralateral side starting from day 1 after injection. The allodynia reached its maximum on day 3 (p ≤ 0.001), as the threshold change was lower on day 7 (Figure 2). No significant changes in the contralateral threshold were observed in the whisker pad area.
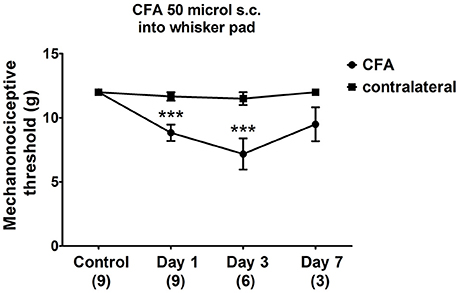
Figure 2. Changes in mechanical threshold in response to von Frey filaments before and 1, 3, 7 days after CFA (50 μl s.c. complete Freund's adjuvant) inflammation. Orofacial thresholds in both ipsilateral and contralateral sides were measured. Data are means ± S.E.M. (n = 9 at control and day 1; n = 6 at day 3; n = 3 at day 7). Asterisks denote statistically significant differences between contralateral (CT) and ipsilateral (CFA) sides (***p ≤ 0.001) as analysed by two-way ANOVA followed by Bonferroni's post-test.
RT-PCR Analysis
Validation of Differentially Expressed mRNAs in TRG by Real-Time RT-PCR
To validate the microarray results, the transcription levels of five differentially expressed, microarray-identified genes were further determined using quantitative real-time RT-PCR. The following genes were chosen for validation: Lkaaear1, Neurod2 (Table 1), as well as G-protein coupled receptor 39 (Gpr39), kisspeptin (Kiss1) and kisspeptin-1 receptor (Kiss1r) (microarray data not shown). The relative fold changes (up-regulated) of Gpr39 and Lkaaear1 for CFA TRG samples were 3.04 and 4.01 respectively, while the relative fold changes (down-regulated) of Kiss1, Kiss1r and Neurod2 were −1.74, −2.63, and −9.2 (Table 1, Supplementary Table 2). On day 7, Gpr39 and Kiss1r alterations were similar to the microarray data (Figure 3). PCR results could not confirm microarray data on Lkaaear1, Neurod2 and Kiss1. Lkaaear1 presented decreased mRNA levels on day 7 compared to contralateral CT side. In addition, we were unable to detect Neurod2 expression changes in TRG with our PCR protocol. We also chose to investigate the time course of neuronal and activation marker expressions. Although Fosb, Ionized calcium binding adaptor molecule 1 (Iba1), Glial fibrillary acidic protein (Gfap) and Calcitonin gene-related peptide (Cgrp) were not listed in microarray data, meaning no significant changes between the two groups of interest, we analysed the variation of these mRNA levels as well. No significant differences were detected on day 7 related to the mentioned genes which further confirmed the consistency and reliability of the microarray data.
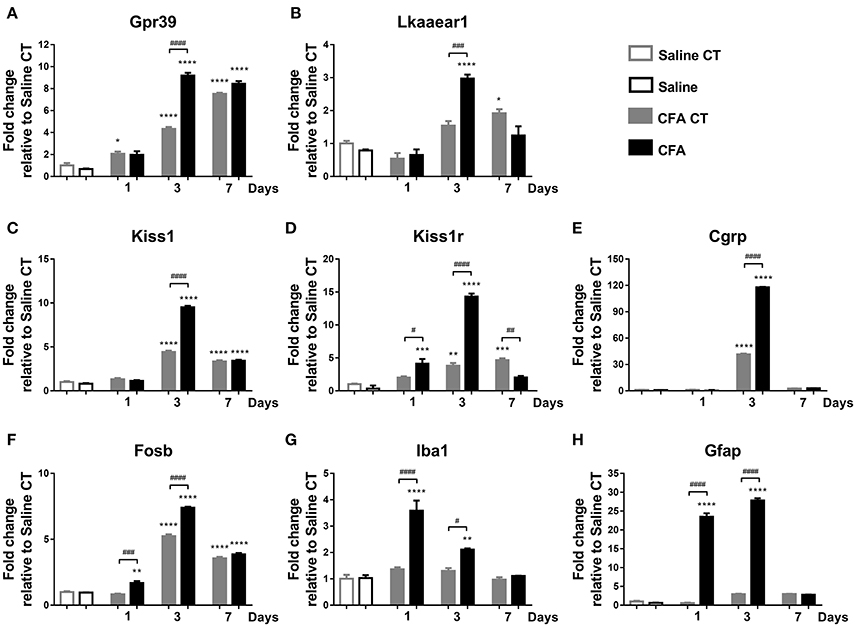
Figure 3. Time course of normalized fold changes in the trigeminal ganglia of Gpr39 (A), Lkaaear1 (B), Kiss1 (C), Kiss1r (D), Cgrp (E), Fosb (F), Iba1 (G), and Gfap (H) mRNA expression one, 3 and 7 days after CFA injection. The mRNA levels were normalised to β2m and Hprt1, as detailed in materials and methods. Data are means ± S.E.M. (n = 3 at each time point). Asterisks denote statistically significant differences between CT Saline and CT/CFA groups (*p ≤ 0.05, **p ≤ 0.01, ***p ≤ 0.001, ****p ≤ 0.0001), while hash marks label statistically significant differences between respective CT and CFA groups (#p ≤ 0.05, ##p ≤ 0.01, ###p ≤ 0.001, ####p ≤ 0.0001) as analysed by one-way ANOVA followed by Tukeys' multiple comparison tests.
Gene Expression Analysis in TRG Tissues
We measured mRNA levels of eight genes in TRG tissues on three different time points after CFA injection. On day 1, CFA-induced significant up-regulation of Kiss1r, as well as of neuronal (Fosb), glial (Iba1), and astrocyte (Gfap) activation markers compared to saline-treated control group. By day 3, seven genes reached their maximum at a level of 9.18- (Gpr39), 2.97- (Lkaaear1), 9.51- (Kiss1), 14.31- (Kiss1r), 117.82- (Cgrp), 7.40- (Fosb), and 27.80- fold (Gfap). Iba1 reached a 3.6-fold peak at day 1 before declining. mRNA levels of Lkaaear1, Kiss1r, Iba1 gradually decreased at last time point until reaching a non-significant level compared to saline-treated control side (Figure 3).
Gene Expression Analysis in TNC Tissues
Briefly, main changes in the relative gene expression were observed directly 1, 3 and 7 days post-CFA treatment. All measured mRNA levels, except Kiss1r, showed significantly altered temporal change in TNC of CFA-injected samples when compared to both CFA CT and Saline CT, presenting a maximum at day 3 (p ≤ 0.001 or p ≤ 0.0001). There was no significant difference in mRNA abundance of Kiss1r on different time points (Figure 4).
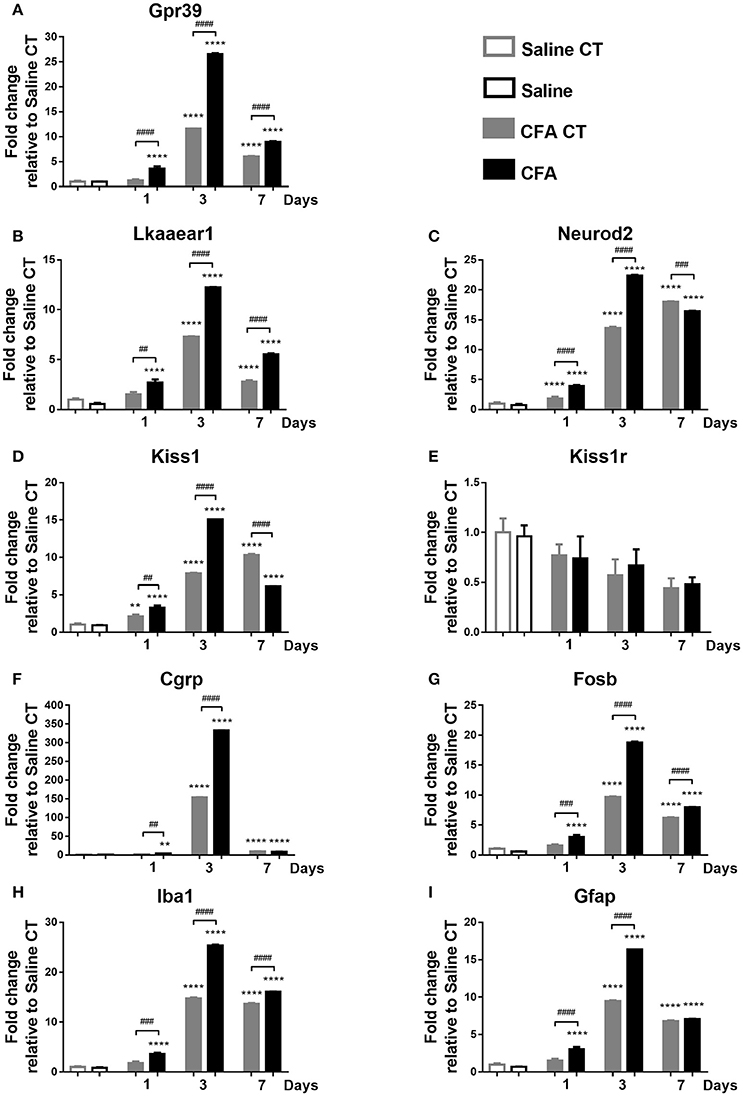
Figure 4. Time course of normalized fold changes in the trigeminal nucleus caudalis of Gpr39 (A), Lkaaear1 (B), Neurod2 (C), Kiss1 (D), Kiss1r (E), Cgrp (F), Fosb (G), Iba1 (H), and Gfap (I) mRNA expression one, 3 and 7 days after CFA injection. The mRNA levels were normalised to β2m and Hprt1, as detailed in materials and methods. Data are means ± S.E.M. (n = 3 at each time point). Asterisks denote statistically significant differences between CT Saline and CT/CFA groups (**p ≤ 0.01, ****p ≤ 0.0001), while hash marks label statistically significant differences between respective CT and CFA groups (##p ≤ 0.01, ###p ≤ 0.001, ####p ≤ 0.0001) as analysed by one-way ANOVA followed by Tukeys' multiple comparison tests.
Gene Expression Analysis in PBMCs
Finally, low but significant expressional changes of Lkaaear1 and Kiss1r gene mRNA in peripheral blood from CFA-treated rats have been observed. Lkaaear1 displayed a gene expression pattern similar to Kiss1r, where Lkaaear1 presented a maximum of 2.33 and Kiss1r a 3.86 fold change at day one. We noted no significant changes in Gpr39 mRNA levels of PBMCs after CFA exposure. Interestingly, Fosb and Iba1 seem to be up-regulated (p ≤ 0.01 or p ≤ 0.001) at each time point due to CFA treatment, while Gfap only on day 7 (Figure 5).
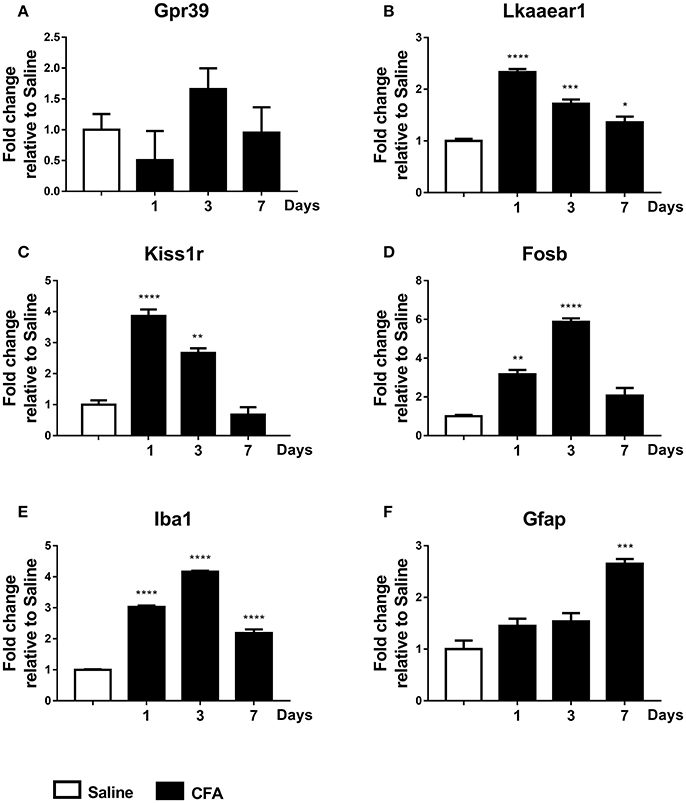
Figure 5. Time course of normalized fold changes in PBMC of Gpr39 (A), Lkaaear1 (B), Kiss1r (C), Fosb (D), Iba1 (E), and Gfap (F) mRNA expression one, 3 and 7 days after CFA injection. The mRNA levels were normalised to Ppia and Hprt1, as detailed in materials and methods. Data are means ± S.E.M. (n = 3 at each time point). Asterisks denote statistically significant differences between respective Saline and CT/CFA groups (*p ≤ 0.05, **p ≤ 0.01, ***p ≤ 0.001, ****p ≤ 0.0001) as analysed by one-way ANOVA followed by Tukeys' multiple comparison tests.
Heat Map Plotting
Fold change data were plotted on a heat map to summarize changes in mRNA levels measured by qPCR (Figure 6). Genes with a similar level of expression were grouped into three major clusters in TRG samples: 1. Kiss1r, Gpr39; 2. Kiss, Fosb, Lkr, Cgrp; 3. Gfap, Iba1. In the TNC, all but one gene fall into a large cluster with highly similar temporal patterns, except for Kiss1r that changed the opposite way, however, its alterations were not found to be significant. Group 3 genes distinctly upregulated in CFA-treated TRG samples on days 1 and 3 but not on respective contralateral sides while they were substantially upregulated in TNC samples from both sides on these days, as well as on day 7. Genes upregulated in TRG and TNC were also elevated in PBMCs, although starting at an earlier time point (day 1) for most genes.
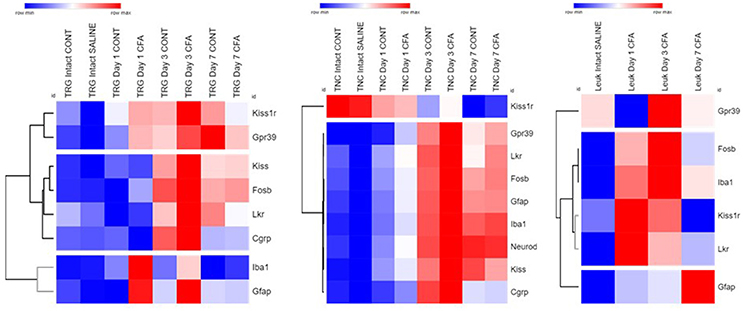
Figure 6. Summary of all qPCR results in TRG, TNC and PBMCs one, 3 and 7 days after CFA injection and in saline-treated controls. Fold change values were log2 transformed and plotted on a heat map. Rows: genes; columns: sample groups; scale: blue (low) to red (high). Hierarchical cluster analysis (1-Pearson correlation and average linkage method) was performed. Dendrograms on the left side of each figure demonstrate similarities between expression patterns of genes.
Discussion
To our knowledge, this is the first comprehensive study which compared gene expression changes in the TRG, TNC and peripheral blood leukocytes in an inflammatory orofacial pain model. Simultaneous measurement of the transcriptional changes of PBMCs had been suggested to reflect alterations in the CNS (Arosio et al., 2014; Gerring et al., 2016; Srinivasan et al., 2017). We described up- and downregulation of distinct genes that are likely to be involved in the activation and sensitization of primary and secondary trigeminal neurons.
The mechanisms of nociceptor sensitization after inflammation have been extensively studied in rodents by electrophysiological, histological and molecular biological approaches (Hucho and Levine, 2007; Coste et al., 2008; Matsumoto et al., 2010; Cady et al., 2011; Bernstein and Burstein, 2012; Weyer et al., 2016). Nevertheless, we cannot extrapolate all the findings to the trigeminovascular system, since it is considerably different from other regions of the somatosensory system. As mentioned before, the central terminals of extra- and intracranial trigeminal primary afferents converge considerably in the TNC. As a consequence, inflammatory sensitization of primary meningeal afferents and secondary trigeminal neurons resulted in an enhanced response to cutaneous stimulation of the face (Burstein et al., 1998; Levy et al., 2004). On the other hand, experimental data also confirm that noxious stimulation (e.g., intranasal capsaicin), inflammation or nerve lesion on the face can induce meningeal vasodilation or neurogenic inflammation (Kunkler et al., 2011; Filipović et al., 2012). Intriguingly, it was revealed that there are trigeminal afferents which project to both the meninges and extracranial tissues (Schueler et al., 2013). Both human and rodent data point out that gene expression of TRGs is distinct from DRGs (Manteniotis et al., 2013; Flegel et al., 2015; Kogelman et al., 2017; LaPaglia et al., 2017). Yet, there have only been few rodent studies investigating gene expression changes in the TRGs after chronic orofacial inflammation (Okumura et al., 2010; Chung et al., 2016).
Our microarray study revealed a high number of differentially expressed olfactory, taste and pheromone receptor genes between the ipsi- and contralateral sides 7 days after CFA treatment. A large number of transcripts of chemoreceptors had been detected in murine and human TRGs using next-generation sequencing (Manteniotis et al., 2013), however their involvement in trigeminal sensitisation is not known. It is appealing to draw parallels between the perturbation of TRG chemoreceptors in our model and the known phenomena of an odour or perfume-triggered migraine, as well as odour hypersensitivity, osmophobia, odour hallucination and taste abnormalities associated with migraine (Schreiber and Calvert, 1986; Kelman, 2004; Goadsby et al., 2017). The microarray analysis implicated thyroid hormone receptor beta which had been previously associated with migraine (Gormley et al., 2015), and chemokine signalling (Ccr7), among many others as well as long non-coding RNAs (lncRNA) putatively involved in gene regulation.
On the basis of these microarray results, we further investigated the time-dependent changes of one of the most upregulated genes (Lkaaear1) and the most downregulated (Neurod2) gene with qPCR. Transcripts of genes with possible roles in nociception, which also have the potential to be future drug targets, were also chosen to be studied, such as two G-protein-coupled receptors (Gpr39 and Kiss1r) and the neuropeptide kisspeptin (Kiss1). Lkaaear1 encodes an LKAAEAR motive containing protein with unclear function. It is highly expressed in the brain and testis and during organ development (NCBI Gene database)2 Neurod2 is involved in neuronal differentiation and has been implicated in synaptic plasticity (Bayam et al., 2015; Chen et al., 2016). Gpr39 is a Zn2+-sensing Gαq-coupled receptor which is expressed in a wide range of tissues including some areas of the brain. Activation of Gpr39 induces the release of Ca2+ via the IP3 pathway. The receptor may play a role in depression, and as a specific and direct sensor of Zn2+, in many physiological functions where the cation is involved such as synaptic transmission (Popovics and Stewart, 2011; Sato et al., 2016). Kisspeptin, encoded by the Kiss1 gene, is considered to have an emerging role in the neuroendocrine regulation of reproduction and puberty (de Roux et al., 2003; Seminara, 2006; Kauffman et al., 2007; Colledge, 2009). Kisspeptin-expressing neurons and Kiss1r are found in areas other than the hypothalamus: amygdala, hippocampus, periaqueductal grey (Oakley et al., 2009; Herbison et al., 2010). In addition, DRG and dorsal horns neurons of the spinal cord have been shown to express kisspeptin and Kiss1r, whose expression might be upregulated due to intra-articular injection of CFA (Mi et al., 2009). There are studies showing hyperalgesic effect of peripheral and intrathecal kisspeptin (Spampinato et al., 2011). Likewise, i.c.v. administration of kisspeptin-10 induces both hyperalgesia and opioid antagonistic activity (Elhabazi et al., 2013), suggesting its possible involvement in the regulation of pain sensitivity.
We successfully reproduced the changes detected with the microarray by qPCR in cases of Gpr39 and Kiss1r. Neurod2 transcripts were not detected in the TRG and Lkaaear1 expression was higher on day 3 but not on day 7 compared to the contralateral side. It is important to highlight that there was a delayed but considerable increase of mRNA levels on the contralateral side of CFA-treated animals when compared to saline-treated animals. This is consistent with earlier reports found after inflammation or nerve injury of the hind limbs in which structural and biochemical changes appeared both centrally and in the periphery on the contralateral side (Koltzenburg et al., 1999; Shenker et al., 2003) However, we did not only use the contralateral side of CFA injected animals as controls, but we also included a saline-injected group as well. We aimed at keeping the animal number at a minimum level and meanwhile taking into account the possible trauma caused by only the injection itself. In addition, there was no detectable allodynia on the contralateral side in our model, therefore the comparison to the contralateral side is still valid from a functional aspect and provides additional information based on this double comparison.
We added Cgrp to the list of investigated markers to validate the model, since it is a well-known mediator and even a novel pharmacological target of migraine (Durham, 2006; Doods et al., 2007; Benemei et al., 2009; Edvinsson et al., 2012; Bigal et al., 2013; Russo, 2015). Moreover, its expression was shown to be elevated in TRGs in rodent models of orofacial inflammation (Yasuda et al., 2012; Shinoda and Iwata, 2013; Kuzawinska et al., 2014). Our results are consistent with these previous findings, Cgrp transcripts were significantly increased in the TRG at day 3 after CFA treatment corresponding to the peak of the facial allodynia.
In addition to the TRG, we also examined the transcriptional changes in the TNC, reflecting mechanisms involved in central sensitization, as well as PBMCs in the peripheral blood. In the TNC, significant changes were observed for the examined genes with the exception of Kiss1r. Intriguingly, the Kiss1 expression in the TNC was mirroring the changes of the receptor expression in the TRG which suggests a presynaptic effect on primary afferents. Lkaaear1 and Kiss1r expression were also significantly increased in PBMCs with a similar time course.
Besides allodynia, as the main functional parameter, neuronal and glial activation markers were also assessed by comparing their gene expression profiles. Therefore, we determined the widely-used neuronal activation marker Fosb, Gfap for astrocytes and Iba1 for microglia (Nestler et al., 2001; Alibhai et al., 2007; Knight et al., 2011). Gfap has been shown to play a role in astrocyte migration, the function of the blood-brain barrier, signal transduction pathways and neuron-glia interactions (Middeldorp and Hol, 2011). Iba1, also known as AIF1 (Allograft Inflammatory Factor 1) expressed in various cells such as monocyte/macrophages and activated T lymphocytes, is mostly used as a microglia marker (Kelemen and Autieri, 2005; Pawlik et al., 2016). All the three activation markers were significantly increased already at day 1 of the inflammation in both TRGs and TNCs, peaked by day 3 and decreased by day 7 when allodynia was declining. Remarkably and most interestingly, a smaller but significant increase of expression was also detectable in PBMCs which highlights the relevance of blood transcriptomics data in CNS diseases. To our knowledge, this is the first study to determine these transcripts in the peripheral blood of experimental animals, however, there are relevant human data for Gfap as a blood biomarker. It was first presented in acute stroke diagnosis in adults (Niebrój-Dobosz et al., 1994) and head trauma (Missler et al., 1999). Recently, it has been suggested that Gfap might be a potential biomarker of intracerebral haemorrhage (IHC) with symptoms of acute stroke (Brunkhorst et al., 2010; Mayer et al., 2013; Foerch et al., 2015). It is also an early marker of traumatic brain injury (Bembea et al., 2011; Lei et al., 2015), during different phases of cardiopulmonary bypass (Vedovelli et al., 2017), with predictiveness of neurological outcome (Lei et al., 2015). It is clear that the measurement of Gfap changes at the periphery is not a specific diagnostic tool and it is too early to draw a final conclusion on its utility at this stage. However, it would be interesting to see in future studies whether it could have a prognostic value to predict the conversion of orofacial pain or headache conditions from episodic to chronic. In our model, Gfap expression remained high even at the end of the experiment which could reflect a persistent neuroinflammation.
In conclusion, the main novelty of the present findings is the description of some up- and downregulated genes at the levels of both primary and secondary sensory neurones of the trigeminovascular system that might play important roles in neuroinflammatory activation mechanisms. Furthermore, we are the first to show transcriptomic alterations in the PBMCs that are similar to the changes detected in the neuronal tissues. These results open new perspectives and initiate further investigations in the research of trigeminal pain disorders.
Author Contributions
ZH, KB, and ÉS: Study concept and design; KB, TA, and ÉS: Animal model, behavioural studies and sample collection conducted by; JK, SJ, AG, TR, TA: Microarray/qPCR analysis and interpretation of genetic data; ZH and TR: Funding. All authors contributed to the analysis of the results, drafting of manuscript and approved the final version.
Funding
The present study was supported by Hungarian Grants: National Brain Research Program 2(2017-1.2.1-NKP-2017-00002), EFOP-3.6.1.-16-2016-0004, GINOP 2.3.2-15-2016-00050 PEPSYS and University of Pécs, Medical School Grant: KA-2015-20. JK was supported by a scholarship from the European Union and the State of Hungary, co-financed by the European Social Fund in the framework of TAMOP-4.2.4.A/2-11/1-2012-0001 National Excellence Program Apáczai Scholarship.
Conflict of Interest Statement
The authors declare that the research was conducted in the absence of any commercial or financial relationships that could be construed as a potential conflict of interest.
Acknowledgments
The authors wish to thank Mrs. Dóra Ömböli for expert technical assistance.
Supplementary Material
The Supplementary Material for this article can be found online at: https://www.frontiersin.org/articles/10.3389/fnmol.2018.00219/full#supplementary-material
Abbreviations
TRG, trigeminal ganglion; TNC, trigeminal nucleus caudalis; PBMC, peripheral blood mononuclear cells; CFA, Complete Freund's Adjuvant; Iba1, Ionized calcium binding adaptor molecule 1; Gfap, Glial fibrillary acidic protein.
Footnotes
1. ^https://software.broadinstitute.org/morpheus/ Broad Institute, Cambridge, MA, USA.
References
Alexa, A., and Rahnenführer, J. (2016). topGO: Enrichment Analysis for Gene Ontology. R package version 2.30.30. doi: 10.18129/B9.bioc.topGO
Alibhai, I. N., Green, T. A., Potashkin, J. A., and Nestler, E. J. (2007). Regulation of fosB and ΔfosB mRNA expression: in vivo and in vitro studies. Brain Res. 1143, 22–33. doi: 10.1016/j.brainres.2007.01.069
Arosio, B., D'Addario, C., Gussago, C., Casati, M., Tedone, E., Ferri, E., et al. (2014). Peripheral blood mononuclear cells as a laboratory to study dementia in the elderly. BioMed Res. Int. 2014:169203. doi: 10.1155/2014/169203
Ashburner, M., Ball, C. A., Blake, J. A., Botstein, D., Butler, H., Cherry, J. M., et al. (2000). Gene ontology: tool for the unification of biology. Gene Ontol. Consortium. Nat. Genet. 25, 25–29. doi: 10.1038/75556
Bayam, E., Sahin, G. S., Guzelsoy, G., Guner, G., Kabakcioglu, A., and Ince-Dunn, G. (2015). Genome-wide target analysis of NEUROD2 provides new insights into regulation of cortical projection neuron migration and differentiation. BMC Genomics 16:681. doi: 10.1186/s12864-015-1882-9
Bembea, M. M., Savage, W., Strouse, J. J., Schwartz, J. M., Graham, E., Thompson, C. B., et al. (2011). Glial fibrillary acidic protein as a brain injury biomarker in children undergoing extracorporeal membrane oxygenation. Pediatr. Crit. Care Med. J. Soc. Crit. Care Med. World Fed. Pediatr. Intensive Crit. Care Soc. 12, 572–579. doi: 10.1097/PCC.0b013e3181fe3ec7
Benemei, S., Nicoletti, P., Capone, J. G., and Geppetti, P. (2009). CGRP receptors in the control of pain and inflammation. Curr. Opin. Pharmacol. 9, 9–14. doi: 10.1016/j.coph.2008.12.007
Bernstein, C., and Burstein, R. (2012). Sensitization of the trigeminovascular pathway: perspective and implications to migraine pathophysiology. J. Clin. Neurol. Seoul Korea 8, 89–99. doi: 10.3988/jcn.2012.8.2.89
Bigal, M. E., Walter, S., and Rapoport, A. M. (2013). Calcitonin gene-related peptide (CGRP) and migraine current understanding and state of development. Headache 53, 1230–1244. doi: 10.1111/head.12179
Brunkhorst, R., Pfeilschifter, W., and Foerch, C. (2010). Astroglial proteins as diagnostic markers of acute intracerebral hemorrhage—pathophysiological background and clinical findings. Transl. Stroke Res. 1, 246–251. doi: 10.1007/s12975-010-0040-6
Burstein, R., Cutrer, M. F., and Yarnitsky, D. (2000). The development of cutaneous allodynia during a migraine attack Clinical evidence for the sequential recruitment of spinal and supraspinal nociceptive neurons in migraine. Brain 123, 1703–1709. doi: 10.1093/brain/123.8.1703
Burstein, R., Yamamura, H., Malick, A., and Strassman, A. M. (1998). Chemical stimulation of the intracranial dura induces enhanced responses to facial stimulation in brain stem trigeminal neurons. J. Neurophysiol. 79, 964–982. doi: 10.1152/jn.1998.79.2.964
Cady, R. J., Glenn, J. R., Smith, K. M., and Durham, P. L. (2011). Calcitonin gene-related peptide promotes cellular changes in trigeminal neurons and glia implicated in peripheral and central sensitization. Mol. Pain 7:94. doi: 10.1186/1744-8069-7-94
Chen, F., Moran, J. T., Zhang, Y., Ates, K. M., Yu, D., Schrader, L. A., et al. (2016). The transcription factor NeuroD2 coordinates synaptic innervation and cell intrinsic properties to control excitability of cortical pyramidal neurons. J. Physiol. 594, 3729–3744. doi: 10.1113/JP271953
Chung, M.-K., Park, J., Asgar, J., and Ro, J. Y. (2016). Transcriptome analysis of trigeminal ganglia following masseter muscle inflammation in rats. Mol. Pain 12:1. doi: 10.1177/1744806916668526
Colledge, W. H. (2009). Kisspeptins and GnRH neuronal signalling. Trends Endocrinol. Metab. 20, 115–121. doi: 10.1016/j.tem.2008.10.005
Coste, J., Voisin, D. L., Luccarini, P., and Dallel, R. (2008). A role for wind-up in trigeminal sensory processing: intensity coding of nociceptive stimuli in the rat. Cephalalgia Int. J. Headache 28, 631–639. doi: 10.1111/j.1468-2982.2008.01568.x
de Roux, N., Genin, E., Carel, J.-C., Matsuda, F., Chaussain, J.-L., and Milgrom, E. (2003). Hypogonadotropic hypogonadism due to loss of function of the KiSS1-derived peptide receptor GPR54. Proc. Natl. Acad. Sci. U.S.A. 100, 10972–10976. doi: 10.1073/pnas.1834399100
Doods, H., Arndt, K., Rudolf, K., and Just, S. (2007). CGRP antagonists: unravelling the role of CGRP in migraine. Trends Pharmacol. Sci. 28, 580–587. doi: 10.1016/j.tips.2007.10.005
Du, X., Tang, Y., Xu, H., Lit, L., Walker, W., Ashwood, P., et al. (2006). Genomic profiles for human peripheral blood T cells, B cells, natural killer cells, monocytes, and polymorphonuclear cells: comparisons to ischemic stroke, migraine, and Tourette syndrome. Genomics 87, 693–703. doi: 10.1016/j.ygeno.2006.02.003
Durham, P. L. (2006). Calcitonin Gene-Related Peptide (CGRP) and migraine. Headache 46, S3–S8. doi: 10.1111/j.1526-4610.2006.00483.x
Edgar, R., Domrachev, M., and Lash, A. E. (2002). Gene expression omnibus: NCBI gene expression and hybridization array data repository. Nucleic Acids Res. 30, 207–210. doi: 10.1093/nar/30.1.207
Edvinsson, L., Villalón, C. M., and MaassenVanDenBrink, A. (2012). Basic mechanisms of migraine and its acute treatment. Pharmacol. Ther. 136, 319–333. doi: 10.1016/j.pharmthera.2012.08.011
Elhabazi, K., Humbert, J.-P., Bertin, I., Schmitt, M., Bihel, F., Bourguignon, J.-J., et al. (2013). Endogenous mammalian RF-amide peptides, including PrRP, kisspeptin and 26RFa, modulate nociception and morphine analgesia via NPFF receptors. Neuropharmacology 75, 164–171. doi: 10.1016/j.neuropharm.2013.07.012
Fabregat, A., Jupe, S., Matthews, L., Sidiropoulos, K., Gillespie, M., Garapati, P., et al. (2018). The Reactome Pathway Knowledgebase. Nucleic Acids Res. 46, D649–D655. doi: 10.1093/nar/gkx1132
Filipović, B., Matak, I., Bach-Rojecky, L., and Lacković, Z. (2012). Central action of peripherally applied botulinum toxin Type A on pain and dural protein extravasation in rat model of trigeminal neuropathy. PLoS ONE 7:e29803. doi: 10.1371/journal.pone.0029803
Flegel, C., Schöbel, N., Altmüller, J., Becker, C., Tannapfel, A., Hatt, H., et al. (2015). RNA-Seq analysis of human trigeminal and dorsal root ganglia with a focus on chemoreceptors. PLoS ONE 10:e0128951. doi: 10.1371/journal.pone.0128951
Foerch, C., Luger, S., and Group, B. F. S. (2015). Glial fibrillary acidic protein (GFAP) plasma levels distinguish intracerebral hemorrhage from cerebral ischemia in the early phase of acute stroke. J. Neurol. Sci. 357:e430. doi: 10.1016/j.jns.2015.09.042
Gardiner, I., Ahmed, F., Steiner, T., McBain, A., Kennard, C., and de Belleroche, J. (1998). A study of adaptive responses in cell signaling in migraine and cluster headache: correlations between headache type and changes in gene expression. Cephalalgia 18, 192–196. doi: 10.1046/j.1468-2982.1998.1804192.x
Gentleman, R. C., Carey, V. J., Bates, D. M., Bolstad, B., Dettling, M., Dudoit, S., et al. (2004). Bioconductor: open software development for computational biology and bioinformatics. Genome Biol. 5:R80. doi: 10.1186/gb-2004-5-10-r80
Gerring, Z. F., Powell, J. E., Montgomery, G. W., and Nyholt, D. R. (2017). Genome-wide analysis of blood gene expression in migraine implicates immune-inflammatory pathways. Cephalalgia 38, 292–303. doi: 10.1177/0333102416686769
Gerring, Z., Rodriguez-Acevedo, A. J., Powell, J. E., Griffiths, L. R., Montgomery, G. W., and Nyholt, D. R. (2016). Blood gene expression studies in migraine: potential and caveats. Cephalalgia 36, 669–678. doi: 10.1177/0333102416628463
Goadsby, P. J., Holland, P. R., Martins-Oliveira, M., Hoffmann, J., Schankin, C., and Akerman, S. (2017). Pathophysiology of migraine: a disorder of sensory processing. Physiol. Rev. 97, 553–622. doi: 10.1152/physrev.00034.2015
Gormley, P., Anttila, V., Winsvold, B. S., Palta, P., Esko, T., Pers, T. H., et al. (2015). Meta-analysis of 375,000 individuals identifies 38 susceptibility loci for migraine. bioRxiv 030288. doi: 10.1101/030288
Gregory, N. S., Harris, A. L., Robinson, C. R., Dougherty, P. M., Fuchs, P. N., and Sluka, K. A. (2013). An overview of animal models of pain: disease models and outcome measures. J. Pain 14, 1255–1269. doi: 10.1016/j.jpain.2013.06.008
Herbison, A. E., d'Anglemont de Tassigny, X., Doran, J., and Colledge, W. H. (2010). Distribution and postnatal development of Gpr54 gene expression in mouse brain and gonadotropin-releasing hormone neurons. Endocrinology 151, 312–321. doi: 10.1210/en.2009-0552
Hershey, A. D., Tang, Y., Powers, S. W., Kabbouche, M. A., Gilbert, D. L., Glauser, T. A., et al. (2004). Genomic abnormalities in patients with migraine and chronic migraine: preliminary blood gene expression suggests platelet abnormalities. Headache 44, 994–1004. doi: 10.1111/j.1526-4610.2004.04193.x
Hershey, A., Horn, P., Kabbouche, M., O'Brien, H., and Powers, S. (2012). Genomic expression patterns in menstrually-related migraine in adolescents. Headache 52, 68–79. doi: 10.1111/j.1526-4610.2011.02049.x
Hucho, T., and Levine, J. D. (2007). Signaling pathways in sensitization: toward a nociceptor cell biology. Neuron 55, 365–376. doi: 10.1016/j.neuron.2007.07.008
Iwata, K., Takeda, M., Bae Oh, S., and Shinoda, M. (2017). “Neurophysiology of orofacial pain,” in Contemporary Oral Medicine, eds C. Farah, R. Balasubramaniam, and M. McCullough (Cham: Springer), 1–23.
Kanehisa, M., and Goto, S. (2000). KEGG: kyoto encyclopedia of genes and genomes. Nucleic Acids Res. 28, 27–30. doi: 10.1093/nar/28.1.27
Kauffman, A. S., Clifton, D. K., and Steiner, R. A. (2007). Emerging ideas about kisspeptin– GPR54 signaling in the neuroendocrine regulation of reproduction. Trends Neurosci. 30, 504–511. doi: 10.1016/j.tins.2007.08.001
Kelemen, S. E., and Autieri, M. V. (2005). Expression of Allograft Inflammatory Factor-1 in T Lymphocytes. Am. J. Pathol. 167, 619–626. doi: 10.1016/S0002-9440(10)63003-9
Kelman, L. (2004). Osmophobia and taste abnormality in migraineurs: a tertiary care study. Headache 44, 1019–1023. doi: 10.1111/j.1526-4610.2004.04197.x
Knight, W. D., Little, J. T., Carreno, F. R., Toney, G. M., Mifflin, S. W., and Cunningham, J. T. (2011). Chronic intermittent hypoxia increases blood pressure and expression of FosB/ FosB in central autonomic regions. Am. J. Physiol. Regul. Integr. Comp. Physiol. 301, R131–R139. doi: 10.1152/ajpregu.00830.2010
Kogelman, L. J. A., Christensen, R. E., Pedersen, S. H., Bertalan, M., Hansen, T. F., Jansen-Olesen, I., et al. (2017). Whole transcriptome expression of trigeminal ganglia compared to dorsal root ganglia in Rattus Norvegicus. Neuroscience 350, 169–179. doi: 10.1016/j.neuroscience.2017.03.027
Koltzenburg, M., Wall, P. D., and McMahon, S. B. (1999). Does the right side know what the left is doing? Trends Neurosci. 22, 122–127. doi: 10.1016/S0166-2236(98)01302-2
Krzyzanowska, A., and Avendaño, C. (2012). Behavioral testing in rodent models of orofacial neuropathic and inflammatory pain. Brain Behav. 2, 678–697. doi: 10.1002/brb3.85
Krzyzanowska, A., Pittolo, S., Cabrerizo, M., Sánchez-López, J., Krishnasamy, S., Venero, C., et al. (2011). Assessing nociceptive sensitivity in mouse models of inflammatory and neuropathic trigeminal pain. J. Neurosci. Methods 201, 46–54. doi: 10.1016/j.jneumeth.2011.07.006
Kunkler, P. E., Ballard, C. J., Oxford, G. S., and Hurley, J. H. (2011). TRPA1 receptors mediate environmental irritant-induced meningeal vasodilatation. Pain 152, 38–44. doi: 10.1016/j.pain.2010.08.021
Kuzawinska, O., Lis, K., Cudna, A., and Balkowiec-Iskra, E. (2014). Gender differences in the neurochemical response of trigeminal ganglion neurons to peripheral inflammation in mice. Acta Neurobiol Exp 74, 227–232.
LaPaglia, D. M., Sapio, M. R., Burbelo, P. D., Thierry-Mieg, J., Thierry-Mieg, D., Raithel, S. J., et al. (2017). RNA-Seq investigations of human post-mortem trigeminal ganglia. Cephalalgia 38, 912–932. doi: 10.1177/0333102417720216
Lei, J., Gao, G., Feng, J., Jin, Y., Wang, C., Mao, Q., et al. (2015). Glial fibrillary acidic protein as a biomarker in severe traumatic brain injury patients: a prospective cohort study. Crit. Care Lond. Engl. 19:362. doi: 10.1186/s13054-015-1081-8
Levy, D., Jakubowski, M., and Burstein, R. (2004). Disruption of communication between peripheral and central trigeminovascular neurons mediates the antimigraine action of 5HT1B/1D receptor agonists. Proc. Natl. Acad. Sci. U.S.A. 101, 4274–4279. doi: 10.1073/pnas.0306147101
Luo, W., Friedman, M. S., Shedden, K., Hankenson, K. D., and Woolf, P. J. (2009). GAGE: generally applicable gene set enrichment for pathway analysis. BMC Bioinformatics 10:161. doi: 10.1186/1471-2105-10-161
Manteniotis, S., Lehmann, R., Flegel, C., Vogel, F., Hofreuter, A., Schreiner, B. S. P., et al. (2013). Comprehensive RNA-Seq expression analysis of sensory ganglia with a focus on ion channels and GPCRs in trigeminal ganglia. PLoS ONE 8:e79523. doi: 10.1371/journal.pone.0079523
Matsumoto, S., Yoshida, S., Takahashi, M., Saiki, C., and Takeda, M. (2010). The roles of ID, IA and IK in the electrophysiological functions of small-diameter rat trigeminal ganglion neurons. Curr. Mol. Pharmacol. 3, 30–36. doi: 10.2174/1874467211003010030
Mayer, C. A., Brunkhorst, R., Niessner, M., Pfeilschifter, W., Steinmetz, H., and Foerch, C. (2013). Blood levels of glial fibrillary acidic protein (GFAP) in patients with neurological diseases. PLoS ONE 8:e62101. doi: 10.1371/journal.pone.0062101
Mi, W.-L., Mao-Ying, Q.-L., Liu, Q., Wang, X.-W., Li, X., Wang, Y.-Q., et al. (2009). The distribution of kisspeptin and its receptor GPR54 in rat dorsal root ganglion and up-regulation of its expression after CFA injection. Brain Res. Bull. 78, 254–260. doi: 10.1016/j.brainresbull.2008.12.003
Middeldorp, J., and Hol, E. M. (2011). GFAP in health and disease. Prog Neurobiol. 93, 421–443. doi: 10.1016/j.pneurobio.2011.01.005
Missler, U., Wiesmann, M., Wittmann, G., Magerkurth, O., and Hagenström, H. (1999). Measurement of glial fibrillary acidic protein in human blood: analytical method and preliminary clinical results. Clin. Chem. 45, 138–141.
Nestler, E. J., Barrot, M., and Self, D. W. (2001). ΔFosB: a sustained molecular switch for addiction. Proc. Natl. Acad. Sci. U.S.A. 98, 11042–11046. doi: 10.1073/pnas.191352698
Niebrój-Dobosz, I., Rafałowska, J., Lukasiuk, M., Pfeffer, A., and Mossakowski, M. J. (1994). Immunochemical analysis of some proteins in cerebrospinal fluid and serum of patients with ischemic strokes. Folia Neuropathol. 32, 129–137.
Oakley, A. E., Clifton, D. K., and Steiner, R. A. (2009). Kisspeptin Signaling in the Brain. Endocr. Rev. 30, 713–743. doi: 10.1210/er.2009-0005
Okumura, M., Iwata, K., Yasuda, K., Inoue, K., Shinoda, M., Honda, K., et al. (2010). Alternation of Gene Expression in Trigeminal Ganglion Neurons Following Complete Freund's Adjuvant or Capsaicin Injection into the Rat Face. J. Mol. Neurosci. 42, 200–209. doi: 10.1007/s12031-010-9348-7
Pawlik, A., Kotrych, D., Paczkowska, E., Roginska, D., Dziedziejko, V., Safranow, K., et al. (2016). Expression of allograft inflammatory factor-1 in peripheral blood monocytes and synovial membranes in patients with rheumatoid arthritis. Hum. Immunol. 77, 131–136. doi: 10.1016/j.humimm.2015.11.008
Perrino, C., Barabási, A.-L., Condorelli, G., Davidson, S. M., De Windt, L., Dimmeler, S., et al. (2017). Epigenomic and transcriptomic approaches in the post-genomic era: path to novel targets for diagnosis and therapy of the ischaemic heart? Position Paper of the European Society of Cardiology Working Group on Cellular Biology of the Heart. Cardiovasc. Res. 113, 725–736. doi: 10.1093/cvr/cvx070
Plummer, P. N., Colson, N. J., Lewohl, J. M., MacKay, R. K., Fernandez, F., Haupt, L. M., et al. (2011). Significant differences in gene expression of GABA receptors in peripheral blood leukocytes of migraineurs. Gene 490, 32–36. doi: 10.1016/j.gene.2011.08.031
Popovics, P., and Stewart, A. J. (2011). GPR39: a Zn2+-activated G protein-coupled receptor that regulates pancreatic, gastrointestinal and neuronal functions. Cell. Mol. Life Sci. 68, 85–95. doi: 10.1007/s00018-010-0517-1
R Development Core Team (2008). R: A Language and Environment for Statistical Computing. Vienna: R Foundation for Statistical Computing.
Ren, K., and Dubner, R. (1999). Inflammatory models of pain and hyperalgesia. ILAR J. 40, 111–118. doi: 10.1093/ilar.40.3.111
Ritchie, M. E., Phipson, B., Wu, D., Hu, Y., Law, C. W., Shi, W., et al. (2015). limma powers differential expression analyses for RNA-sequencing and microarray studies. Nucleic Acids Res. 43:e47. doi: 10.1093/nar/gkv007
Romero-Reyes, M., Akerman, S., Nguyen, E., Vijjeswarapu, A., Hom, B., Dong, H.-W., et al. (2013). Spontaneous behavioral responses in the orofacial region: a model of trigeminal pain in mouse. Headache 53, 137–151. doi: 10.1111/j.1526-4610.2012.02226.x
Romero-Reyes, M., and Uyanik, J. M. (2014). Orofacial pain management: current perspectives. J. Pain Res. 7, 99–115. doi: 10.2147/JPR.S37593
Russo, A. F. (2015). Calcitonin Gene-Related Peptide (CGRP). Annu. Rev. Pharmacol. Toxicol. 55, 533–552. doi: 10.1146/annurev-pharmtox-010814-124701
Sato, S., Huang, X.-P., Kroeze, W. K., and Roth, B. L. (2016). Discovery and characterization of novel GPR39 agonists allosterically modulated by Zinc. Mol. Pharmacol. 90, 726–737. doi: 10.1124/mol.116.106112
Schreiber, A. O., and Calvert, P. C. (1986). Migrainous olfactory hallucinations. Headache 26, 513–514. doi: 10.1111/j.1526-4610.1986.hed2610513.x
Schueler, M., Messlinger, K., Dux, M., Neuhuber, W. L., and De, R. (2013). Extracranial projections of meningeal afferents and their impact on meningeal nociception and headache. Pain 154, 1622–1631. doi: 10.1016/j.pain.2013.04.040
Seminara, S. B. (2006). Mechanisms of Disease: the first kiss—a crucial role for kisspeptin-1 and its receptor, G-protein-coupled receptor 54, in puberty and reproduction. Nat. Rev. Endocrinol. 2, 328–334. doi: 10.1038/ncpendmet0139
Shenker, N., Haigh, R., Roberts, E., Mapp, P., Harris, N., and Blake, D. (2003). A review of contralateral responses to a unilateral inflammatory lesion. Rheumatol. Oxf. Engl. 42, 1279–1286. doi: 10.1093/rheumatology/keg397
Shinoda, M., and Iwata, K. (2013). Neural communication in the trigeminal ganglion contributes to ectopic orofacial pain. J. Oral Biosci. 55, 165–168. doi: 10.1016/j.job.2013.06.003
Spampinato, S., Trabucco, A., Biasiotta, A., Biagioni, F., Cruccu, G., Copani, A., et al. (2011). Hyperalgesic activity of kisspeptin in mice. Mol. Pain 7:90. doi: 10.1186/1744-8069-7-90
Srinivasan, S., Di Dario, M., Russo, A., Menon, R., Brini, E., Romeo, M., et al. (2017). Dysregulation of MS risk genes and pathways at distinct stages of disease. Neurol. - Neuroimmunol. Neuroinflammation 4:e337. doi: 10.1212/NXI.0000000000000337
Takeda, M., Tanimoto, T., Kadoi, J., Nasu, M., Takahashi, M., Kitagawa, J., et al. (2007). Enhanced excitability of nociceptive trigeminal ganglion neurons by satellite glial cytokine following peripheral inflammation. Pain 129, 155–166. doi: 10.1016/j.pain.2006.10.007
The Gene Ontology Consortium (2017). Expansion of the Gene Ontology knowledgebase and resources. Nucleic Acids Res. 45, D331–D338. doi: 10.1093/nar/gkw1108
Vedovelli, L., Padalino, M., D'Aronco, S., Stellin, G., Ori, C., Carnielli, V. P., et al. (2017). Glial fibrillary acidic protein plasma levels are correlated with degree of hypothermia during cardiopulmonary bypass in congenital heart disease surgery. Interact. Cardiovasc. Thorac. Surg. 24, 436–442. doi: 10.1093/icvts/ivw395
Weyer, A. D., Zappia, K. J., Garrison, S. R., O'Hara, C. L., Dodge, A. K., and Stucky, C. L. (2016). Nociceptor Sensitization Depends on Age and Pain Chronicity(1,2,3). eNeuro 3: ENEURO.0115-15.2015. doi: 10.1523/ENEURO.0115-15.2015
Keywords: orofacial pain, trigeminovascular system, Kisspeptin-1 receptor, Gpr39, Neurod2, differential gene expression data analysis
Citation: Aczél T, Kun J, Szőke É, Rauch T, Junttila S, Gyenesei A, Bölcskei K and Helyes Z (2018) Transcriptional Alterations in the Trigeminal Ganglia, Nucleus and Peripheral Blood Mononuclear Cells in a Rat Orofacial Pain Model. Front. Mol. Neurosci. 11:219. doi: 10.3389/fnmol.2018.00219
Received: 04 April 2018; Accepted: 06 June 2018;
Published: 26 June 2018.
Edited by:
Hermona Soreq, Hebrew University of Jerusalem, IsraelReviewed by:
Parisa Gazerani, Aalborg University, DenmarkHans-Georg Schaible, Friedrich-Schiller-Universität-Jena, Germany
Copyright © 2018 Aczél, Kun, Szőke, Rauch, Junttila, Gyenesei, Bölcskei and Helyes. This is an open-access article distributed under the terms of the Creative Commons Attribution License (CC BY). The use, distribution or reproduction in other forums is permitted, provided the original author(s) and the copyright owner are credited and that the original publication in this journal is cited, in accordance with accepted academic practice. No use, distribution or reproduction is permitted which does not comply with these terms.
*Correspondence: Zsuzsanna Helyes, zsuzsanna.helyes@aok.pte.hu
†These authors have contributed equally to this work.