- Department of Neurobiology and Anatomy, Wake Forest School of Medicine, Winston-Salem, NC, United States
The primate prefrontal cortex (PFC) is critical for executive functions including working memory, task switching and response selection. The functional organization of this area has been a matter of debate over a period of decades. Early models proposed segregation of spatial and object information represented in working memory in the dorsal and ventral PFC, respectively. Other models emphasized the integrative ability of the entire PFC depending on task demands, not necessarily tied to working memory. An anterior-posterior hierarchy of specialization has also been speculated, in which progressively more abstract information is represented more anteriorly. Here we revisit this debate, updating these arguments in light of recent evidence in non-human primate neurophysiology studies. We show that spatial selectivity is predominantly represented in the posterior aspect of the dorsal PFC, regardless of training history and task performed. Objects of different features excite both dorsal and ventral prefrontal neurons, however neurons highly specialized for feature information are located predominantly in the posterior aspect of the ventral PFC. In accordance with neuronal selectivity, spatial working memory is primarily impaired by inactivation or lesion of the dorsal PFC and object working memory by ventral inactivation or lesion. Neuronal responses are plastic depending on task training but training too has dissociable effects on ventral and dorsal PFC, with the latter appearing to be more plastic. Despite the absence of an overall topography, evidence exists for the orderly localization of stimulus information at a sub-millimeter scale, within the dimensions of a cortical column. Unresolved questions remain, regarding the existence or not of a functional map at the areal and columnar scale, and the link between behavior and neuronal activity for different prefrontal subdivisions.
Introduction
The functional organization of the prefrontal cortex (PFC) in humans and non-human primates has been a matter of long-standing debate (Riley and Constantinidis, 2016). Neurophysiological studies in non-human primates in the 1990s described physiological correlates of functional specialization across the dorsal-ventral axis, proposing that the dorsolateral PFC is responsible for spatial working memory, and the ventrolateral PFC for object working memory (Wilson et al., 1993; Ó Scalaidhe et al., 1997, 1999). These conclusions were challenged by other influential studies, which demonstrated that individual neurons in the PFC can represent both spatial and object information, thus suggesting that PFC is the site of integration of these streams in the primate brain (Rao et al., 1997; Rainer et al., 1998a). Human imaging studies at the time were also equivocal about the dorso-ventral organization of the PFC, with some supporting the idea that specialized processing occurs within the two prefrontal subdivisions (Adcock et al., 2000; Leung et al., 2002; Sala and Courtney, 2007), whereas others suggesting an organization in terms of cognitive operations rather than type of information (Owen et al., 1996, 1998; Stern et al., 2000). An anterior-posterior axis of functional specialization has also been speculated, suggesting a hierarchical organization progressing towards anterior areas (Badre and D’Esposito, 2009). How such a hierarchy might intersect with a dorso-ventral specialization added to the confusion.
A number of recent studies allow a re-evaluation of this debate, resolution of some of the questions and deeper insights on the representation of information in prefrontal networks. Here, we review results from non-human primate neurophysiology. We discuss the anatomical organization of the PFC, the evidence for specialization of stimulus representation within the PFC, the capacity for plasticity based on task being executed, the evidence for functional specialization drawn from inactivation experiments, and finally, the evidence for orderly representation of stimulus information within the cortical microcircuits.
Anatomical Organization
The PFC can be divided into a lateral, a medial and an orbital aspect. The lateral aspect can be further distinguished into a dorsal and ventral subdivision. The terms ventral and dorsal have not been used consistently in the literature, however (Constantinidis and Procyk, 2004). Walker initially identified multiple cytoarchitectonic areas on the lateral aspect of the macaque brain: areas 8a (encompassing the Frontal Eye Field) and 45 lining the superior and inferior banks of the arcuate sulcus respectively, area 8b just medial to the arcuate, areas 9 and 12 in the superior and inferior convexities of the cortex respectively, area 46 lining either banks of the principal sulcus and area 10 covering the frontal pole (Walker, 1940). Area 46 has been additionally subdivided along its mediolateral aspect, into areas 46dr, 46d, 46v and 46vr, lining the medial rim, the medial and the lateral banks of the principal sulcus and the lateral rim of the principal sulcus, respectively (Preuss and Goldman-Rakic, 1991). There is also evidence of a specialization in the anterior-posterior aspect, with the caudal aspect of area 46 shown to be functionally dissociable from the anterior one; the former is sometimes referred to as area 9/46, whereas the most anterior one as area 46 (Petrides, 2000). More areas yet may be present based on the evidence provided by fMRI studies probing functional connectivity at rest (Goulas et al., 2017).
Anatomical studies point to a relative segregation of projections from the posterior parietal cortex, which terminate mostly to the dorsal PFC (areas 8 and 46, including both banks of the principal sulcus), and from the inferior temporal cortex, which terminate on areas 12 and 45 of the ventral PFC (Petrides and Pandya, 1984; Selemon and Goldman-Rakic, 1988; Cavada and Goldman-Rakic, 1989). This specificity of inputs is not only limited to visual afferents; auditory connections representing sound localization information also target the dorsal PFC, whereas those representing auditory features terminate in the ventral PFC (Romanski et al., 1999). A relative segregation of inputs has also been observed across the anterior-posterior axis, with areas higher in the sensory and limbic hierarchies projecting to more anterior prefrontal subdivisions (Gerbella et al., 2013; Barbas, 2015; Borra et al., 2017).
Based on anatomical and physiological evidence, we have recently proposed a functional division of the lateral PFC into a dorsolateral region that encompasses the area between the arcuate and principal sulcus and both banks of the principal sulcus, and a ventral region that comprises the inferior limb of the arcuate and the inferior convexity (Riley et al., 2017). These are further subdivided along the anterior/posterior axis. A fronto-polar aspect completes the lateral surface (Figure 1).
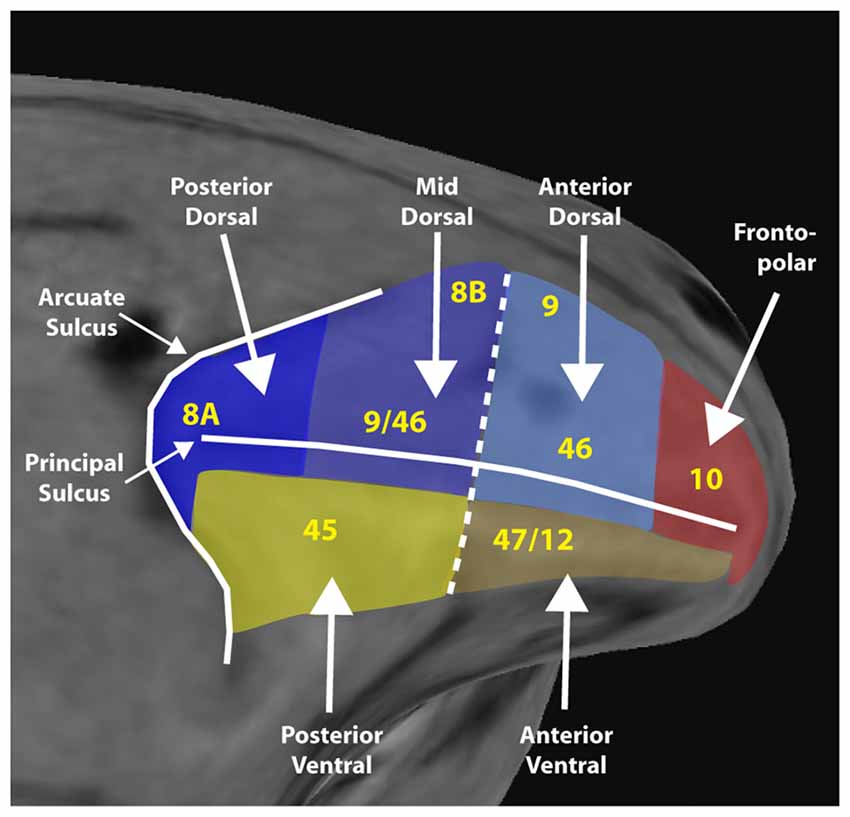
Figure 1. Diagram of a macaque monkey brain, with the lateral prefrontal cortex (PFC) divided into six regions, as indicated. Yellow letters denote approximate location anatomical areas, based on the Petrides and Pandya nomenclature. Adapted from Riley et al. (2017); with permission.
Localization of Stimulus Selectivity
As noted in the “Introduction” section, neurophysiological studies initially proposed that dorsolateral PFC subserves primarily spatial working memory whereas ventrolateral, object working memory (Wilson et al., 1993; Ó Scalaidhe et al., 1997, 1999). This conclusion was based on the finding that a greater proportion of neurons selective for the spatial location of peripheral stimuli were observed in the dorsolateral than in the ventrolateral PFC, in monkeys trained to perform spatial working memory tasks (Wilson et al., 1993). On the other hand, neurons selective for highly specialized images such as faces were almost exclusively observed in the ventrolateral PFC, at least in monkeys just required to view such stimuli passively (Ó Scalaidhe et al., 1997, 1999). This “domain-specific” organization can be thought of as an extension of the dorsal and ventral visual streams (Ungerleider and Mishkin, 1982; Felleman and Van Essen, 1991).
An opposing view posited that prefrontal neurons selective for both the location of stimuli and their identity can be encountered throughout the PFC, after monkeys have been trained in tasks that required them to remember both the location and identity of a stimulus (Rao et al., 1997; Rainer et al., 1998a). The implication drawn was that the functional specialization observed in the earlier studies was the result of task requirements (Rao et al., 1997; Rainer et al., 1998a). This “integrative” model suggested a plastic prefrontal organization that is shaped by cognitive demands imposed by the task, instead. Related models suggest that the PFC is primarily organized based on cognitive process rather than stimulus presentation (Owen et al., 1998). Some studies have also suggested that lesions of the ventral PFC do not impair working memory for stimulus shape and color (Rushworth et al., 1997).
Spatial Selectivity
A number of recent studies in our own and other laboratories have reexamined the selectivity of PFC for different properties of stimuli by comparing responses of dorsal and ventral prefrontal neurons to the same stimuli, in the same animals. Spatial selectivity proved a strong predictor of whether a neuron was recorded in the dorsal or the ventral PFC (Meyer et al., 2011). More neurons in the dorsolateral PFC responded to white square stimuli presented at varying spatial locations; a higher percentage of these neurons were selective for spatial location; and a greater spatial selectivity was observed among those neurons, compared to ventrolateral prefrontal neurons. This difference in spatial selectivity between dorsal and ventral PFC was true for monkeys naïve to any training that viewed stimuli presented at different locations passively; for monkeys trained to perform only spatial working memory tasks; as well as for monkeys that were explicitly trained to perform tasks that required simultaneous maintenance of spatial and object information in memory (Meyer et al., 2007, 2011; Riley et al., 2017). This is not to say that ventral prefrontal neurons exhibit no selectivity for spatial location. Neurons with well localized receptive fields and significant selectivity for spatial location were observed in the ventral PFC (Meyer et al., 2011; Riley et al., 2017).
Spatial selectivity is dependent on the position of neurons along the anterior-posterior axis (Figure 2). Neuronal selectivity was found to decrease along the anterior posterior axis, so that the most highly selective neurons for stimulus properties were located more posteriorly in the PFC (Riley et al., 2017). Conversely, neurons in more anterior areas exhibited little selectivity to stimuli per se but were more likely to represent task variables. Thus, the functional specialization between dorsal and ventral PFC became less meaningful, particularly as task demands molded responses of neurons to different stimuli.
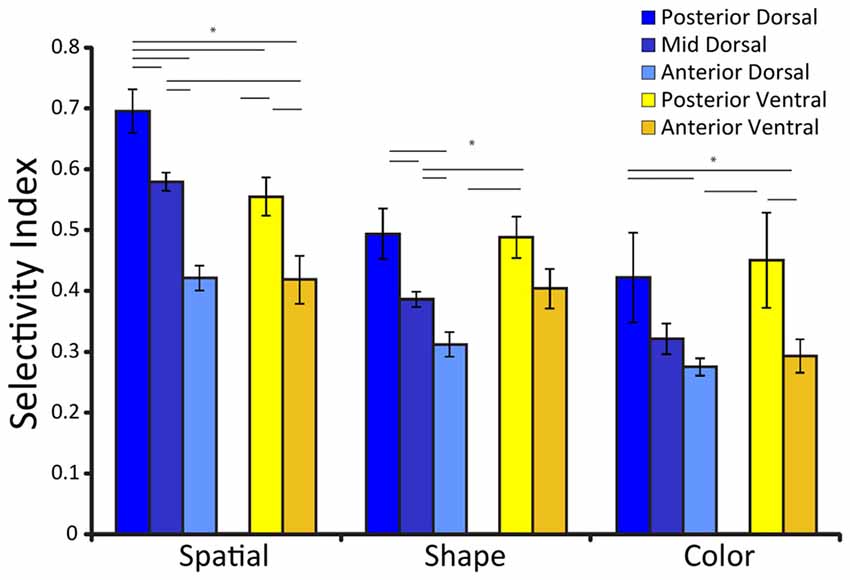
Figure 2. Average selectivity index for three different stimulus sets (defined as the difference between the maximum and minimum firing rate for the stimuli of the set, divided by their sum), among neurons with significant responses to stimuli for each prefrontal subdivisions. Error bars represent standard error of the mean. Adapted from Riley et al. (2017), with permission. Horizontal lines with stars * indicate significant differences (p < 0.05) between conditions, determined based on a 2-way ANOVA and post hoc Tukey test.
Stimulus selectivity also appears to have a strong temporal component in the PFC. In our experiments, neurons were highly selective for spatial location early in the trial, and response latency itself differentiated the highly selective posterior areas from the less selective anterior areas (Riley et al., 2017). Other studies too have determined a stronger contralateral bias in neuronal responses shortly after the appearance of the stimulus, which dissipated later in time (Kadohisa et al., 2015).
Finally, spatial selectivity was most evident for peripheral stimuli. In our experiments, stimuli were positioned 10–14° away from the fovea. Studies that have tested stimuli within 4–6° degrees from the fovea have found little or no differentiation between dorsal and ventral PFC (Rao et al., 1997; Kadohisa et al., 2015). We note that properties of ventral prefrontal neurons are similar to inferior temporal neurons, which project to the ventral PFC, and which can be highly selective for spatial position, particularly in perifoveal locations (DiCarlo and Maunsell, 2003). The difference in spatial selectivity between dorsal and ventral PFC is therefore quantitative rather than qualitative, yet clearly distinguishing between the two.
Feature Selectivity
Our experiments probed further the selectivity of prefrontal neurons for object features by testing neuronal responses in the two areas with a set of eight white geometric objects. Feature selectivity provided a less straightforward picture of the two prefrontal subdivisions. A larger proportion of dorsolateral prefrontal neurons responded to any stimulus appearing in their receptive fields. Many of these neurons exhibited broad but significant selectivity for shape (Meyer et al., 2011). These results are in agreement with findings from other laboratories, which have found broad but significant selectivity for stimulus shape in the dorsolateral PFC, including the Frontal Eye Fields (Peng et al., 2008; Clark et al., 2012). Such shape selectivity has also been reported in the posterior parietal cortex (the main afferent input to dorsolateral PFC), where broad but significant tuning for stimulus shape is present, when probed with very similar geometric shapes (Sereno and Maunsell, 1998; Janssen et al., 2008). On the other hand, ventrolateral prefrontal neurons were less likely to be driven by any stimulus, at least among the limited set of geometric shapes. When we quantified shape selectivity among responsive neurons, this was higher in the ventrolateral than dorsolateral PFC prior to training (Meyer et al., 2011). However, the percentages of selective neurons and selectivity magnitude values after training in working memory tasks were not found to be significantly different between the two prefrontal subdivisions (Meyer et al., 2011). This result essentially replicated the findings of the earlier studies that failed to detect a difference in feature selectivity between dorsal and ventral prefrontal neurons, in monkeys that were trained to perform a combined spatial and object working memory task (Rao et al., 1997). Newer studies have also found little evidence for greater prevalence of object coding in ventral compared to dorsal PFC, when each neuron is tested with a few stimuli (Kadohisa et al., 2015).
Although a limited stimulus set does not allow detection of a clear-cut dichotomy in shape selectivity between dorsal and ventral PFC, the lack of overall responsiveness in the ventrolateral PFC is not incompatible with high specialization for stimulus features. Neurons that are highly selective for object features, will only respond vigorously to a limited set of stimuli and are likely to produce uniformly weak responses to stimuli drawn from a small set, failing to differentiate between them. Such a response pattern has precisely been reported for inferior temporal neurons (Gross et al., 1972; Desimone et al., 1984; Tanaka et al., 1991; Fujita et al., 1992). In contrast, when neurons were tested with stimulus sets requiring very narrow shape selectivity, such as faces and complex objects, neurons distinguishing between such stimuli were localized exclusively in the ventral PFC (Ó Scalaidhe et al., 1997, 1999).
Our experiments also evaluated functional specialization for color, relying on eight iso-luminant colored squares presented over the fovea. The results of this analysis mirrored that of feature selectivity. A large proportion of dorsal PFC responded to colored squares, with a small percentage of neurons exhibiting weak but significant selectivity (Riley et al., 2017). Other studies have also observed color selectivity in only a small proportion of prefrontal neurons, in the order of 5%–15% (Lara and Wallis, 2014). Similarly, approximately 15% of posterior parietal neurons show selectivity for color of stimuli (Constantinidis and Steinmetz, 2001). A smaller percentage of ventral prefrontal neurons responded to the colored squares, and their overall selectivity for color was not significantly different from that of the dorsal PFC (Riley et al., 2017). Combined fMRI studies and neurophysiological studies in the temporal lobe have suggested that neurons selective for faces, other shapes, and colors are clustered at distinct patches of cortex (Tsao et al., 2006; Popivanov et al., 2014; Chang et al., 2017). A handful of studies have explored the PFC as well, for example suggesting that color-selective neurons are concentrated in specific “patches” (Lafer-Sousa and Conway, 2013). These results argue strongly for precise localization of function within the PFC.
As was the case for spatial stimuli, feature selectivity depended upon a second anatomical dimension, the position of neurons along the anterior-posterior axis (Figure 2). Neuronal selectivity for shape and color too was found to decrease along the anterior posterior axis, so that the most highly selective neurons for stimulus properties were located more posteriorly in the PFC (Riley et al., 2017). Object representation is also time dependent; early responses represent objects, whereas more abstract information such as stimulus category has been documented later in prefrontal responses (Meyers et al., 2008; Kadohisa et al., 2015).
Plasticity of Stimulus Representations
The activity of prefrontal neurons is well known to be modulated by task demands. Neuronal activity has been shown to represent the abstract rules of the cognitive tasks subjects are trained to perform (White and Wise, 1999; Wallis et al., 2001), as well as categories (Freedman et al., 2001; Shima et al., 2007), and numerical quantities (Nieder et al., 2002). Responses to stimuli are also modulated by perceptual decisions (Kim and Shadlen, 1999; Barraclough et al., 2004), reward expectation (Leon and Shadlen, 1999), and sequences of events or actions (Averbeck et al., 2002; Inoue and Mikami, 2006; Sigala et al., 2008; Berdyyeva and Olson, 2010). Activity of single neurons can represent stimulus features and task variables simultaneously, including nonlinear combinations of these factors (Rigotti et al., 2013; Parthasarathy et al., 2017). Additionally, prefrontal neurons respond more strongly to stimuli when these are part of a task and the monkey is required to attend to them, than the same stimuli when they are not necessary for performing the task or are even distracting in the context of the task (Rainer et al., 1998b; Everling et al., 2002; Lennert and Martinez-Trujillo, 2011). The stimulus dimension being represented in neuronal activity may dynamically change during the course of a single trial (Mante et al., 2013). The representation of task variables may reasonably be assumed to be the result of training, as these differ between trained tasks. Considering such plasticity of neuronal representations exists, an extreme view of plasticity may lead to the conclusion that all stimulus properties represented in neuronal responses are the effect of training rather than inherent specialization for different types of stimuli. It is important therefore to consider how training in a behavioral task alters stimulus representations within the PFC. Experiments in our laboratory directly addressed this question by recording neuronal activity in monkeys when they were naïve to cognitive task training, and after they were trained to perform tasks that required them to maintain the properties of the same stimuli in working memory (Qi et al., 2011; Qi and Constantinidis, 2013). The effects of training varied between areas. Posterior dorsal PFC exhibited the weakest effects of plasticity, with robust selectivity for spatial stimuli being present both before and after training in the working memory tasks (Meyer et al., 2011; Riley et al., 2017). On the other hand, the ventral subdivisions of the PFC in general, were more affected by the effects of training, such that more neurons were responsive to stimuli when the monkey was performing a working memory task (Meyer et al., 2011; Riley et al., 2017).
Considering this difference in plasticity between ventral and dorsal PFC, is it possible to entirely reverse the relative selectivity for spatial information between the two subdivisions, and have ventral PFC exhibit strong spatial selectivity if this is relevant for a trained task and tightly coupled to reward? Results from one series of studies demonstrated such a reversal; a systematic difference in spatial selectivity between dorsal and ventral PFC was found, but higher spatial selectivity was present in the ventral rather than the dorsal PFC (Kennerley and Wallis, 2009). Recordings in these experiments were localized in the anterior PFC, using a task which signaled the magnitude of reward. It is likely that neuronal responses in anterior and ventral areas are more sensitive to task variables and cognitive factors rather than stimulus properties per se, so that robust selectivity to the location of stimuli may emerge as a result of training in task that requires tracking of reward. Ventral PFC has greater sensitivity to learning of new, rewarded conditions likely due to the action of dopamine D1R receptors (Puig and Miller, 2012).
Our experiments also tested the idea that task demands that require memory of both location and identity of an object modifies the properties of neuronal firing so as to exhibit selectivity for both spatial and object information, as was speculated in early studies that reported integration of these types of information (Rao et al., 1997). This was clearly not the case. Neurons with selectivity for both identity and location were present prior to training, in both the dorsal and ventral PFC (Meyer et al., 2011). Among neurons responding to stimuli, the average strength of selectivity for either spatial location or shape actually declined after training in the task that required working memory for both (Meyer et al., 2011). However, since more neurons were active, the information about stimulus location and shape that could be decoded from neuronal populations after training were no less than that before training (Meyers et al., 2012).
These results suggest that while PFC has a great capacity for plasticity and representation of abstract relationships for behaviorally relevant stimuli, plasticity does not entirely erase pre-existing functional specialization for spatial and object information between dorsal and ventral PFC, particularly in their posterior aspects. The gradients of spatial and object selectivity are depicted schematically in Figure 3. Training does not affect all areas of the PFC in the same manner, either. Neuronal properties detected in trained monkeys thus depend on the anatomical location of the neurons.
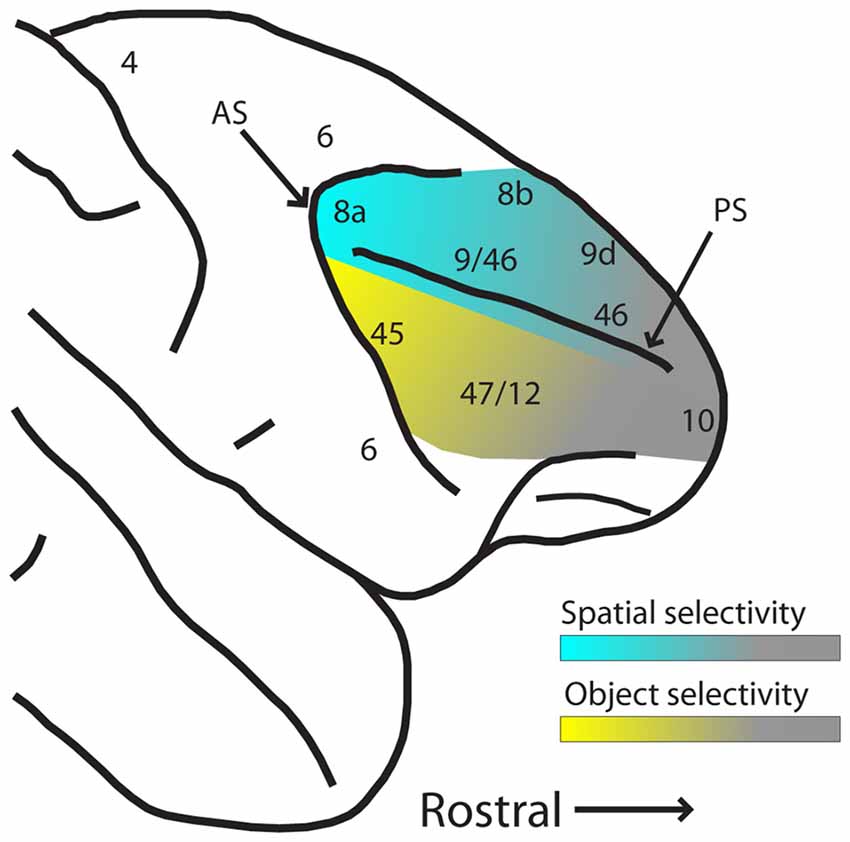
Figure 3. Schematic diagram of hypothesized spatial and object selectivity gradients in the dorsal and ventral PFC. The posterior aspects of dorsal and ventral PFC are most selective for spatial locations and object features (indicated by blue and yellow hues, respectively). Selectivity declines more anteriorly (indicated by gray hue), and effects of task variables and training history become more dominant. Abbreviations: AS, arcuate sulcus; PS, principal sulcus.
Functional Implications of Dorsal and Ventral Prefrontal Inactivation
Although selectivity to different types of stimuli is revealing, the ultimate functional role of an area can be probed by examining the consequences of its activity on performance of working memory tasks. Thus, experiments manipulating neuronal activity can be very informative on the functional roles of prefrontal subdivisions. Temporary inactivation experiments e.g. injections of the GABAA agonist muscimol, or lidocaine, or by cooling of the underlying cortex during working memory are consistent with the specialized stimulus selectivity of dorsal and ventral PFC neurons. Inactivation of dorsal prefrontal areas, including the Frontal Eye Field, decreases performance during spatial working memory tasks (Sommer and Tehovnik, 1997; Dias and Segraves, 1999; Chafee and Goldman-Rakic, 2000; Suzuki and Gottlieb, 2013; Noudoost et al., 2014). Similarly, limited injections of muscimol in the dorsal PFC produce spatial working memory deficits that localize in the contralateral visual field as do small, focal lesions, a phenomenon that has been termed a “mnemonic scotoma” (Funahashi et al., 1993; Sawaguchi and Iba, 2001).
Inactivation of a dorsal area, the Frontal Eye Field, has negligible effects on object working memory (Clark et al., 2014), even when monkeys are tested with objects for which Frontal Eye Field neurons were shown to exhibit broad but significant selectivity in the same object-working memory task (Clark et al., 2012). In contrast, inactivation of the ventral PFC impairs the ability to locate objects based on remembered features, but not on spatial location (Bichot et al., 2015). Location along the anterior-posterior axis is also critical for the effects of lesions. It was lesions in the anterior aspect of the ventral PFC (area 47/12) that failed to produce deficits of feature working memory (Rushworth et al., 1997), and inactivation of the posterior ventral PFC that did (Bichot et al., 2015).
Although our review focuses on spatial and object working memory, we do not wish to suggest that these are the only functions of the dorsal and ventral PFC, respectively. The representation of task rules and associations reviewed in the neurophysiological studies of the previous section fares prominently on the functional consequences of lesion studies. In this case too, the effects of lesions are dissociable between dorsal and ventral PFC. Lesions in the posterior-dorsal PFC impair tasks relying on learnt associations (Petrides, 2005). In contrast, lesions of the mid-dorsal PFC result in deficits in tasks involving presentation of a stimulus and after a delay period, the same stimulus plus a new one, requiring selection of the newly added item, particularly if this is not a novel object but it is one the monkey is familiar with (Petrides, 2005). Damage to the ventral PFC does not produce impairments in recognition or simple recall; its effects become apparent in free-recall tasks (Petrides, 1996). The ventral PFC also appears to be essential for learning a task by trial and error, and reversal learning, which requires learning new associations within a session (Rushworth et al., 1997; Buckley et al., 2009; Rygula et al., 2010).
Prefrontal Microcircuit Organization
In order to understand the organization of spatial and object information in the PFC, it is necessary to delve into the representation of stimulus properties at the scale of cortical micro-circuits. Anatomical evidence reveals a regular pattern of axonal terminations into prefrontal neurons, originating either from within the PFC or from association cortices, forming repeating, interdigitated stripes (Kritzer and Goldman-Rakic, 1995; Pucak et al., 1996). More recent studies also reveal a systematic relationship of correlated spiking as a function of distance between neurons and of their spatial tuning, which also points to a regular organization of synaptic inputs (Leavitt et al., 2013). The principles of anatomical input organization with respect to their functional content remain elusive, though the available evidence raises some possible alternatives.
It is well understood that the PFC is not organized in a retinotopic fashion, with only a coarse bias towards peripheral locations in dorsal subdivisions and foveal locations in ventral subdivisions (Suzuki and Azuma, 1983). The lack of a retinotopic organization across the surface of the PFC should not be surprising given that prefrontal neurons are modulated by a wide array of sensory and cognitive factors. Theoretical studies have formally demonstrated the advantage of schemes that contain multiple representations of the same sensory stimulus, mapped to multiple outcomes but each modulated in a different fashion by contextual factors (Salinas, 2004; Rigotti et al., 2013). The lack of a retinotopic map has been readily demonstrated in studies that sampled systematically the surface of the dorsolateral PFC. The same retinal location was found to be represented at multiple electrode tracks, scattered across the surface of cortex (Constantinidis et al., 2001; Meyer et al., 2011). More recent studies, using chronic implants with a regular grid of electrodes to sample a small area of the PFC, also found no obvious organization; neurons representing any spatial location of the contralateral hemifield are observed within the ~3 × 3 mm surface of such an array with electrodes spaced at 0.4 mm apart (Kiani et al., 2015; Bullock et al., 2017). Wider grids of electrodes spaced at 1.5 mm of each other, revealed no obvious pattern of retinal location represented at this larger spatial scale, either (Markowitz et al., 2015).
Even though the surface of the PFC does not correspond to a topographic map of visual space, organization of receptive fields is not random. Evidence suggests a systematic organization of spatial information, at a finer scale, particularly in the posterior dorsal PFC. Simultaneous recordings from closely spaced electrodes have indicated that neurons in proximity of each other (laterally separated by 0.2–0.3 mm) most often represent adjacent spatial locations (Constantinidis et al., 2001). Clustering of neurons with preference for similar spatial locations and similar motion direction tuning was also detected for prefrontal neurons located up to 0.7 mm apart from each other (Masse et al., 2017), as well as in the grid recordings mentioned above Markowitz et al., 2015;Bullock et al. (2017). Anatomical reconstruction of neurons recorded in neurophysiological experiments as electrodes descent into the principal sulcus also indicate a regular transition of memory field location in adjacent micro-columns (Arnsten, 2013). These results raise the possibility that the entire visual hemifield is represented in repeating cortical modules corresponding to the (0.2–0.8 mm) dimensions of the stripe-like zones of axonal terminations (Levitt et al., 1993; Kritzer and Goldman-Rakic, 1995; Pucak et al., 1996).
Conclusions and Unresolved Questions
A number of conclusions can be drawn from the results that were reviewed here. Anatomical inputs to the PFC are relatively segregated along a dorsal-ventral axis and modulated along an anterior-posterior axis. Functional specialization reflects this anatomical organization so that spatial information is represented predominantly (though not exclusively) in the dorsal PFC. This dominance of spatial information in the dorsal PFC is unaltered by training in working memory tasks, whether they require spatial or object working memory, or combination of both. However, this specialization is highly dependent on: (a) location across the anterior-posterior axis, as higher stimulus selectivity was observed more posteriorly in the dorsolateral PFC and greater dependence to task demands, more anteriorly; (b) time course of responses, with greater sensitivity to spatial location appearing earlier in trials; and (c) representation of peripheral as opposed to perifoveal stimuli, with the former being more distinguishing between dorsal and ventral PFC.
Object working memory has been more difficult to localize with neurophysiological methods. Dorsal prefrontal neurons have broad selectivity for shape and color, and more dorsal prefrontal neurons respond to any given object. On the other hand, the ventral PFC appears better suited for the representation of highly specialized objects like faces. Object selectivity also declines towards more anterior areas and is time-dependent.
A number of unanswered questions remain. One category of questions has to do with the organization of the PFC at the scale of cortical areas and the scale of cortical columns. Is there a functional map of some sort across the surface of prefrontal areas? If so, which are the functional variables that are mapped systematically? What is the role of a prefrontal column within such a map? A combination of fMRI, optical imaging and neurophysiological recordings in non-human primates has made progress in revealing the functional organization of other cortical areas and will likely be instrumental for addressing this question in the PFC.
A second series of questions has to do with the functional implications of activity in different areas of the PFC. The functional role of the different divisions of the PFC will be definitively revealed when a direct link between neuronal activity and behavior can be established. In this context, the question that needs to be addressed is which areas and neurons determine behavior in different working memory and other cognitive tasks? Modern methods of neuronal inactivation and excitation, including through optogenetic manipulation combined with large scale recordings that allow prediction of trial-to-trial deviations of behavior depending on neuronal activity offer promise in answering these questions definitively.
Author Contributions
CC and X-LQ conceptualized this article, reviewed the literature and composed the text.
Funding
This research reported in this article was supported by the National Eye Institute of the National Institutes of Health under award numbers R01 EY017077 and R01 EY016773 to CC and by the Wake Forest Clinical and Translational Science Institute.
Conflict of Interest Statement
The authors declare that the research was conducted in the absence of any commercial or financial relationships that could be construed as a potential conflict of interest.
The reviewer SJC and handling Editor declared their shared affiliation.
References
Adcock, R. A., Constable, R. T., Gore, J. C., and Goldman-Rakic, P. S. (2000). Functional neuroanatomy of executive processes involved in dual-task performance. Proc. Natl. Acad. Sci. U S A 97, 3567–3572. doi: 10.1073/pnas.060588897
Arnsten, A. F. (2013). The neurobiology of thought: the groundbreaking discoveries of Patricia Goldman-Rakic 1937–2003. Cereb. Cortex 23, 2269–2281. doi: 10.1093/cercor/bht195
Averbeck, B. B., Chafee, M. V., Crowe, D. A., and Georgopoulos, A. P. (2002). Parallel processing of serial movements in prefrontal cortex. Proc. Natl. Acad. Sci. U S A 99, 13172–13177. doi: 10.1073/pnas.162485599
Badre, D., and D’Esposito, M. (2009). Is the rostro-caudal axis of the frontal lobe hierarchical? Nat. Rev. Neurosci. 10, 659–669. doi: 10.1038/nrn2667
Barbas, H. (2015). General cortical and special prefrontal connections: principles from structure to function. Annu. Rev. Neurosci. 38, 269–289. doi: 10.1146/annurev-neuro-071714-033936
Barraclough, D. J., Conroy, M. L., and Lee, D. (2004). Prefrontal cortex and decision making in a mixed-strategy game. Nat. Neurosci. 7, 404–410. doi: 10.1038/nn1209
Berdyyeva, T. K., and Olson, C. R. (2010). Rank signals in four areas of macaque frontal cortex during selection of actions and objects in serial order. J. Neurophysiol. 104, 141–159. doi: 10.1152/jn.00639.2009
Bichot, N. P., Heard, M. T., DeGennaro, E. M., and Desimone, R. (2015). A source for feature-based attention in the prefrontal cortex. Neuron 88, 832–844. doi: 10.1016/j.neuron.2015.10.001
Borra, E., Ferroni, C. G., Gerbella, M., Giorgetti, V., Mangiaracina, C., Rozzi, S., et al. (2017). Rostro-caudal connectional heterogeneity of the dorsal part of the macaque prefrontal area 46. Cereb. Cortex doi: 10.1093/cercor/bhx332 [Epub ahead of print].
Buckley, M. J., Mansouri, F. A., Hoda, H., Mahboubi, M., Browning, P. G., Kwok, S. C., et al. (2009). Dissociable components of rule-guided behavior depend on distinct medial and prefrontal regions. Science 325, 52–58. doi: 10.1126/science.1172377
Bullock, K. R., Pieper, F., Sachs, A. J., and Martinez-Trujillo, J. C. (2017). Visual and presaccadic activity in area 8Ar of the macaque monkey lateral prefrontal cortex. J. Neurophysiol. 118, 15–28. doi: 10.1152/jn.00278.2016
Cavada, C., and Goldman-Rakic, P. S. (1989). Posterior parietal cortex in rhesus monkey: II. Evidence for segregated corticocortical networks linking sensory and limbic areas with the frontal lobe. J. Comp. Neurol. 287, 422–445. doi: 10.1002/cne.902870403
Chafee, M. V., and Goldman-Rakic, P. S. (2000). Inactivation of parietal and prefrontal cortex reveals interdependence of neural activity during memory guided-saccades. J. Neurophysiol. 83, 1550–1566. doi: 10.1152/jn.2000.83.3.1550
Chang, L., Bao, P., and Tsao, D. Y. (2017). The representation of colored objects in macaque color patches. Nat. Commun. 8:2064. doi: 10.1038/s41467-017-01912-7
Clark, K. L., Noudoost, B., and Moore, T. (2012). Persistent spatial information in the frontal eye field during object-based short-term memory. J. Neurosci. 32, 10907–10914. doi: 10.1523/jneurosci.1450-12.2012
Clark, K. L., Noudoost, B., and Moore, T. (2014). Persistent spatial information in the FEF during object-based short-term memory does not contribute to task performance. J. Cogn. Neurosci. 26, 1292–1299. doi: 10.1162/jocn_a_00599
Constantinidis, C., Franowicz, M. N., and Goldman-Rakic, P. S. (2001). Coding specificity in cortical microcircuits: a multiple electrode analysis of primate prefrontal cortex. J. Neurosci. 21, 3646–3655. doi: 10.1523/jneurosci.21-10-03646.2001
Constantinidis, C., and Procyk, E. (2004). The primate working memory networks. Cogn. Affect. Behav. Neurosci. 4, 444–465. doi: 10.3758/cabn.4.4.444
Constantinidis, C., and Steinmetz, M. A. (2001). Neuronal responses in area 7a to multiple stimulus displays: I. Neurons encode the location of the salient stimulus. Cereb. Cortex 11, 581–591. doi: 10.1093/cercor/11.7.581
Desimone, R., Albright, T. D., Gross, C. G., and Bruce, C. (1984). Stimulus-selective properties of inferior temporal neurons in the macaque. J. Neurosci. 4, 2051–2062. doi: 10.1523/jneurosci.04-08-02051.1984
Dias, E. C., and Segraves, M. A. (1999). Muscimol-induced inactivation of monkey frontal eye field: effects on visually and memory-guided saccades. J. Neurophysiol. 81, 2191–2214. doi: 10.1152/jn.1999.81.5.2191
DiCarlo, J. J., and Maunsell, J. H. (2003). Anterior inferotemporal neurons of monkeys engaged in object recognition can be highly sensitive to object retinal position. J. Neurophysiol. 89, 3264–3278. doi: 10.1152/jn.00358.2002
Everling, S., Tinsley, C. J., Gaffan, D., and Duncan, J. (2002). Filtering of neural signals by focused attention in the monkey prefrontal cortex. Nat. Neurosci. 5, 671–676. doi: 10.1038/nn874
Felleman, D. J., and Van Essen, D. C. (1991). Distributed hierarchical processing in the primate cerebral cortex. Cereb. Cortex 1, 1–47. doi: 10.1093/cercor/1.1.1
Freedman, D. J., Riesenhuber, M., Poggio, T., and Miller, E. K. (2001). Categorical representation of visual stimuli in the primate prefrontal cortex. Science 291, 312–316. doi: 10.1126/science.291.5502.312
Fujita, I., Tanaka, K., Ito, M., and Cheng, K. (1992). Columns for visual features of objects in monkey inferotemporal cortex. Nature 360, 343–346. doi: 10.1038/360343a0
Funahashi, S., Bruce, C. J., and Goldman-Rakic, P. S. (1993). Dorsolateral prefrontal lesions and oculomotor delayed-response performance: evidence for mnemonic “scotomas”. J. Neurosci. 13, 1479–1497. doi: 10.1523/jneurosci.13-04-01479.1993
Gerbella, M., Borra, E., Tonelli, S., Rozzi, S., and Luppino, G. (2013). Connectional heterogeneity of the ventral part of the macaque area 46. Cereb. Cortex 23, 967–987. doi: 10.1093/cercor/bhs096
Goulas, A., Stiers, P., Hutchison, R. M., Everling, S., Petrides, M., and Margulies, D. S. (2017). Intrinsic functional architecture of the macaque dorsal and ventral lateral frontal cortex. J. Neurophysiol. 117, 1084–1099. doi: 10.1152/jn.00486.2016
Gross, C. G., Rocha-Miranda, C. E., and Bender, D. B. (1972). Visual properties of neurons in inferotemporal cortex of the Macaque. J. Neurophysiol. 35, 96–111. doi: 10.1152/jn.1972.35.1.96
Inoue, M., and Mikami, A. (2006). Prefrontal activity during serial probe reproduction task: encoding, mnemonic and retrieval processes. J. Neurophysiol. 95, 1008–1041. doi: 10.1152/jn.00552.2005
Janssen, P., Srivastava, S., Ombelet, S., and Orban, G. A. (2008). Coding of shape and position in macaque lateral intraparietal area. J. Neurosci. 28, 6679–6690. doi: 10.1523/jneurosci.0499-08.2008
Kadohisa, M., Kusunoki, M., Petrov, P., Sigala, N., Buckley, M. J., Gaffan, D., et al. (2015). Spatial and temporal distribution of visual information coding in lateral prefrontal cortex. Eur. J. Neurosci. 41, 89–96. doi: 10.1111/ejn.12754
Kennerley, S. W., and Wallis, J. D. (2009). Reward-dependent modulation of working memory in lateral prefrontal cortex. J. Neurosci. 29, 3259–3270. doi: 10.1523/jneurosci.5353-08.2009
Kiani, R., Cueva, C. J., Reppas, J. B., Peixoto, D., Ryu, S. I., and Newsome, W. T. (2015). Natural grouping of neural responses reveals spatially segregated clusters in prearcuate cortex. Neuron 85, 1359–1373. doi: 10.1016/j.neuron.2015.02.014
Kim, J. N., and Shadlen, M. N. (1999). Neural correlates of a decision in the dorsolateral prefrontal cortex of the macaque. Nat. Neurosci. 2, 176–185. doi: 10.1038/5739
Kritzer, M. F., and Goldman-Rakic, P. S. (1995). Intrinsic circuit organization of the major layers and sublayers of the dorsolateral prefrontal cortex in the rhesus monkey. J. Comp. Neurol. 359, 131–143. doi: 10.1002/cne.903590109
Lafer-Sousa, R., and Conway, B. R. (2013). Parallel, multi-stage processing of colors, faces and shapes in macaque inferior temporal cortex. Nat. Neurosci. 16, 1870–1878. doi: 10.1038/nn.3555
Lara, A. H., and Wallis, J. D. (2014). Executive control processes underlying multi-item working memory. Nat. Neurosci. 17, 876–883. doi: 10.1038/nn.3702
Leavitt, M. L., Pieper, F., Sachs, A., Joober, R., and Martinez-Trujillo, J. C. (2013). Structure of spike count correlations reveals functional interactions between neurons in dorsolateral prefrontal cortex area 8a of behaving primates. PLoS One 8:e61503. doi: 10.1371/journal.pone.0061503
Lennert, T., and Martinez-Trujillo, J. (2011). Strength of response suppression to distracter stimuli determines attentional-filtering performance in primate prefrontal neurons. Neuron 70, 141–152. doi: 10.1016/j.neuron.2011.02.041
Leon, M. I., and Shadlen, M. N. (1999). Effect of expected reward magnitude on the response of neurons in the dorsolateral prefrontal cortex of the macaque. Neuron 24, 415–425. doi: 10.1016/s0896-6273(00)80854-5
Leung, H. C., Gore, J. C., and Goldman-Rakic, P. S. (2002). Sustained mnemonic response in the human middle frontal gyrus during on-line storage of spatial memoranda. J. Cogn. Neurosci. 14, 659–671. doi: 10.1162/08989290260045882
Levitt, J. B., Lewis, D. A., Yoshioka, T., and Lund, J. S. (1993). Topography of pyramidal neuron intrinsic connections in macaque monkey prefrontal cortex (areas 9 and 46). J. Comp. Neurol. 338, 360–376. doi: 10.1002/cne.903380304
Mante, V., Sussillo, D., Shenoy, K. V., and Newsome, W. T. (2013). Context-dependent computation by recurrent dynamics in prefrontal cortex. Nature 503, 78–84. doi: 10.1038/nature12742
Markowitz, D. A., Curtis, C. E., and Pesaran, B. (2015). Multiple component networks support working memory in prefrontal cortex. Proc. Natl. Acad. Sci. U S A 112, 11084–11089. doi: 10.1073/pnas.1517476112
Masse, N. Y., Hodnefield, J. M., and Freedman, D. J. (2017). Mnemonic encoding and cortical organization in parietal and prefrontal cortices. J. Neurosci. 37, 6098–6112. doi: 10.1523/jneurosci.3903-16.2017
Meyer, T., Qi, X. L., and Constantinidis, C. (2007). Persistent discharges in the prefrontal cortex of monkeys naive to working memory tasks. Cereb. Cortex 17, i70–i76. doi: 10.1093/cercor/bhm063
Meyer, T., Qi, X. L., Stanford, T. R., and Constantinidis, C. (2011). Stimulus selectivity in dorsal and ventral prefrontal cortex after training in working memory tasks. J. Neurosci. 31, 6266–6276. doi: 10.1523/jneurosci.6798-10.2011
Meyers, E. M., Freedman, D. J., Kreiman, G., Miller, E. K., and Poggio, T. (2008). Dynamic population coding of category information in inferior temporal and prefrontal cortex. J. Neurophysiol. 100, 1407–1419. doi: 10.1152/jn.90248.2008
Meyers, E. M., Qi, X. L., and Constantinidis, C. (2012). Incorporation of new information into prefrontal cortical activity after learning working memory tasks. Proc. Natl. Acad. Sci. U S A 109, 4651–4656. doi: 10.1073/pnas.1201022109
Nieder, A., Freedman, D. J., and Miller, E. K. (2002). Representation of the quantity of visual items in the primate prefrontal cortex. Science 297, 1708–1711. doi: 10.1126/science.1072493
Noudoost, B., Clark, K. L., and Moore, T. (2014). A distinct contribution of the frontal eye field to the visual representation of saccadic targets. J. Neurosci. 34, 3687–3698. doi: 10.1523/jneurosci.3824-13.2014
Ó Scalaidhe, S. P., Wilson, F. A., and Goldman-Rakic, P. S. (1997). Areal segregation of face-processing neurons in prefrontal cortex. Science 278, 1135–1138. doi: 10.1126/science.278.5340.1135
Ó Scalaidhe, S. P., Wilson, F. A., and Goldman-Rakic, P. S. (1999). Face-selective neurons during passive viewing and working memory performance of rhesus monkeys: evidence for intrinsic specialization of neuronal coding. Cereb. Cortex 9, 459–475. doi: 10.1093/cercor/9.5.459
Owen, A. M., Doyon, J., Petrides, M., and Evans, A. C. (1996). Planning and spatial working memory: a positron emission tomography study in humans. Eur. J. Neurosci. 8, 353–364. doi: 10.1111/j.1460-9568.1996.tb01219.x
Owen, A. M., Stern, C. E., Look, R. B., Tracey, I., Rosen, B. R., and Petrides, M. (1998). Functional organization of spatial and nonspatial working memory processing within the human lateral frontal cortex. Proc. Natl. Acad. Sci. U S A 95, 7721–7726. doi: 10.1073/pnas.95.13.7721
Parthasarathy, A., Herikstad, R., Bong, J. H., Medina, F. S., Libedinsky, C., and Yen, S. C. (2017). Mixed selectivity morphs population codes in prefrontal cortex. Nat. Neurosci. 20, 1770–1779. doi: 10.1038/s41593-017-0003-2
Peng, X., Sereno, M. E., Silva, A. K., Lehky, S. R., and Sereno, A. B. (2008). Shape selectivity in primate frontal eye field. J. Neurophysiol. 100, 796–814. doi: 10.1152/jn.01188.2007
Petrides, M. (1996). Specialized systems for the processing of mnemonic information within the primate frontal cortex. Philos. Trans. R. Soc. Lond. B Biol. Sci. 351, 1455–1461; discussion 1461–1462. doi: 10.1098/rstb.1996.0130
Petrides, M. (2000). The role of the mid-dorsolateral prefrontal cortex in working memory. Exp. Brain Res. 133, 44–54. doi: 10.1007/s002210000399
Petrides, M. (2005). Lateral prefrontal cortex: architectonic and functional organization. Philos. Trans. R. Soc. Lond. B Biol. Sci. 360, 781–795. doi: 10.1098/rstb.2005.1631
Petrides, M., and Pandya, D. N. (1984). Projections to the frontal cortex from the posterior parietal region in the rhesus monkey. J. Comp. Neurol. 228, 105–116. doi: 10.1002/cne.902280110
Popivanov, I. D., Jastorff, J., Vanduffel, W., and Vogels, R. (2014). Heterogeneous single-unit selectivity in an fMRI-defined body-selective patch. J. Neurosci. 34, 95–111. doi: 10.1523/jneurosci.2748-13.2014
Preuss, T. M., and Goldman-Rakic, P. S. (1991). Architectonics of the parietal and temporal association cortex in the strepsirhine primate Galago compared to the anthropoid primate Macaca. J. Comp. Neurol. 310, 475–506. doi: 10.1002/cne.903100403
Pucak, M. L., Levitt, J. B., Lund, J. S., and Lewis, D. A. (1996). Patterns of intrinsic and associational circuitry in monkey prefrontal cortex. J. Comp. Neurol. 376, 614–630. doi: 10.1002/(sici)1096-9861(19961223)376:4<614::aid-cne9>3.0.co;2-4
Puig, M. V., and Miller, E. K. (2012). The role of prefrontal dopamine D1 receptors in the neural mechanisms of associative learning. Neuron 74, 874–886. doi: 10.1016/j.neuron.2012.04.018
Qi, X. L., and Constantinidis, C. (2013). Neural changes after training to perform cognitive tasks. Behav. Brain Res. 241, 235–243. doi: 10.1016/j.bbr.2012.12.017
Qi, X. L., Meyer, T., Stanford, T. R., and Constantinidis, C. (2011). Changes in prefrontal neuronal activity after learning to perform a spatial working memory task. Cereb. Cortex 21, 2722–2732. doi: 10.1093/cercor/bhr058
Rainer, G., Asaad, W. F., and Miller, E. K. (1998a). Memory fields of neurons in the primate prefrontal cortex. Proc. Natl. Acad. Sci. U S A 95, 15008–15013. doi: 10.1073/pnas.95.25.15008
Rainer, G., Asaad, W. F., and Miller, E. K. (1998b). Selective representation of relevant information by neurons in the primate prefrontal cortex. Nature 393, 577–579. doi: 10.1038/31235
Rao, S. C., Rainer, G., and Miller, E. K. (1997). Integration of what and where in the primate prefrontal cortex. Science 276, 821–824. doi: 10.1126/science.276.5313.821
Rigotti, M., Barak, O., Warden, M. R., Wang, X. J., Daw, N. D., Miller, E. K., et al. (2013). The importance of mixed selectivity in complex cognitive tasks. Nature 497, 585–590. doi: 10.1038/nature12160
Riley, M. R., and Constantinidis, C. (2016). Role of prefrontal persistent activity in working memory. Front. Syst. Neurosci. 9:181. doi: 10.3389/fnsys.2015.00181
Riley, M. R., Qi, X. L., and Constantinidis, C. (2017). Functional specialization of areas along the anterior-posterior axis of the primate prefrontal cortex. Cereb. Cortex 27, 3683–3697. doi: 10.1093/cercor/bhw190
Romanski, L. M., Tian, B., Fritz, J., Mishkin, M., Goldman-Rakic, P. S., and Rauschecker, J. P. (1999). Dual streams of auditory afferents target multiple domains in the primate prefrontal cortex. Nature Neurosci. 2, 1131–1136. doi: 10.1038/16056
Rushworth, M. F., Nixon, P. D., Eacott, M. J., and Passingham, R. E. (1997). Ventral prefrontal cortex is not essential for working memory. J. Neurosci. 17, 4829–4838. doi: 10.1523/jneurosci.17-12-04829.1997
Rygula, R., Walker, S. C., Clarke, H. F., Robbins, T. W., and Roberts, A. C. (2010). Differential contributions of the primate ventrolateral prefrontal and orbitofrontal cortex to serial reversal learning. J. Neurosci. 30, 14552–14559. doi: 10.1523/jneurosci.2631-10.2010
Sala, J. B., and Courtney, S. M. (2007). Binding of what and where during working memory maintenance. Cortex 43, 5–21. doi: 10.1016/s0010-9452(08)70442-8
Salinas, E. (2004). Fast remapping of sensory stimuli onto motor actions on the basis of contextual modulation. J. Neurosci. 24, 1113–1118. doi: 10.1523/jneurosci.4569-03.2004
Sawaguchi, T., and Iba, M. (2001). Prefrontal cortical representation of visuospatial working memory in monkeys examined by local inactivation with muscimol. J. Neurophysiol. 86, 2041–2053. doi: 10.1152/jn.2001.86.4.2041
Selemon, L. D., and Goldman-Rakic, P. S. (1988). Common cortical and subcortical targets of the dorsolateral prefrontal and posterior parietal cortices in the rhesus monkey: evidence for a distributed neural network subserving spatially guided behavior. J. Neurosci. 8, 4049–4068. doi: 10.1523/jneurosci.08-11-04049.1988
Sereno, A. B., and Maunsell, J. H. (1998). Shape selectivity in primate lateral intraparietal cortex. Nature 395, 500–503. doi: 10.1038/26752
Shima, K., Isoda, M., Mushiake, H., and Tanji, J. (2007). Categorization of behavioural sequences in the prefrontal cortex. Nature 445, 315–318. doi: 10.1038/nature05470
Sigala, N., Kusunoki, M., Nimmo-Smith, I., Gaffan, D., and Duncan, J. (2008). Hierarchical coding for sequential task events in the monkey prefrontal cortex. Proc. Natl. Acad. Sci. U S A 105, 11969–11974. doi: 10.1073/pnas.0802569105
Sommer, M. A., and Tehovnik, E. J. (1997). Reversible inactivation of macaque frontal eye field. Exp. Brain Res. 116, 229–249. doi: 10.1007/pl00005752
Stern, C. E., Owen, A. M., Tracey, I., Look, R. B., Rosen, B. R., and Petrides, M. (2000). Activity in ventrolateral and mid-dorsolateral prefrontal cortex during nonspatial visual working memory processing: evidence from functional magnetic resonance imaging. Neuroimage 11, 392–399. doi: 10.1006/nimg.2000.0569
Suzuki, H., and Azuma, M. (1983). Topographic studies on visual neurons in the dorsolateral prefrontal cortex of the monkey. Exp. Brain Res. 53, 47–58. doi: 10.1007/bf00239397
Suzuki, M., and Gottlieb, J. (2013). Distinct neural mechanisms of distractor suppression in the frontal and parietal lobe. Nat. Neurosci. 16, 98–104. doi: 10.1038/nn.3282
Tanaka, K., Saito, H., Fukada, Y., and Moriya, M. (1991). Coding visual images of objects in the inferotemporal cortex of the macaque monkey. J. Neurophysiol. 66, 170–189. doi: 10.1152/jn.1991.66.1.170
Tsao, D. Y., Freiwald, W. A., Tootell, R. B., and Livingstone, M. S. (2006). A cortical region consisting entirely of face-selective cells. Science 311, 670–674. doi: 10.1126/science.1119983
Ungerleider, L. G., and Mishkin, M. (1982). “Two cortical visual systems,” in Analysis of Visual Behavior, eds D. J. Ingle, M. A. Goodale and R. J. W. Mansfield (Cambridge, MA: MIT Press), 549–586.
Walker, A. E. (1940). A cytoarchitectural study of the prefrontal area of the macaque monkey. J. Comp. Neurol. 73, 59–86. doi: 10.1002/cne.900730106
Wallis, J. D., Anderson, K. C., and Miller, E. K. (2001). Single neurons in prefrontal cortex encode abstract rules. Nature 411, 953–956. doi: 10.1038/35082081
White, I. M., and Wise, S. P. (1999). Rule-dependent neuronal activity in the prefrontal cortex. Exp. Brain Res. 126, 315–335. doi: 10.1007/s002210050740
Keywords: prefrontal cortex, monkey, neurophysiology, fMRI, neurons
Citation: Constantinidis C and Qi X-L (2018) Representation of Spatial and Feature Information in the Monkey Dorsal and Ventral Prefrontal Cortex. Front. Integr. Neurosci. 12:31. doi: 10.3389/fnint.2018.00031
Received: 17 April 2018; Accepted: 17 July 2018;
Published: 07 August 2018.
Edited by:
Ioan Opris, University of Miami, United StatesReviewed by:
Emmanuel Procyk, Institut National de la Santé et de la Recherche Médicale (INSERM), FranceStephano Jin Chang, University of Miami, United States
Copyright © 2018 Constantinidis and Qi. This is an open-access article distributed under the terms of the Creative Commons Attribution License (CC BY). The use, distribution or reproduction in other forums is permitted, provided the original author(s) and the copyright owner(s) are credited and that the original publication in this journal is cited, in accordance with accepted academic practice. No use, distribution or reproduction is permitted which does not comply with these terms.
*Correspondence: Christos Constantinidis, Y2NvbnN0YW5Ad2FrZWhlYWx0aC5lZHU=