- 1Sorbonne Université, Paris, France
- 2Inserm, U1127, Paris, France
- 3CNRS, UMR 7225, Paris, France
- 4Institut du Cerveau et de la Moelle Epinière, Paris, France
- 5National Reference Center for Neurometabolic Diseases, Pitié-Salpêtrière University Hospital, Assistance Publique-Hôpitaux de Paris, Paris, France
- 6Equipe de Neurogénétique, Ecole Pratique des Hautes Etudes, PSL Research University, Paris, France
Hereditary spastic paraplegias (HSP) are a group of neurodegenerative diseases sharing spasticity in lower limbs as common symptom. There is a large clinical variability in the presentation of patients, partly underlined by the large genetic heterogeneity, with more than 60 genes responsible for HSP. Despite this large heterogeneity, the proteins with known function are supposed to be involved in a limited number of cellular compartments such as shaping of the endoplasmic reticulum or endolysosomal function. Yet, it is difficult to understand why alteration of such different cellular compartments can lead to degeneration of the axons of cortical motor neurons. A common feature that has emerged over the last decade is the alteration of lipid metabolism in this group of pathologies. This was first revealed by the identification of mutations in genes encoding proteins that have or are supposed to have enzymatic activities on lipid substrates. However, it also appears that mutations in genes affecting endoplasmic reticulum, mitochondria, or endolysosome function can lead to changes in lipid distribution or metabolism. The aim of this review is to discuss the role of lipid metabolism alterations in the physiopathology of HSP, to evaluate how such alterations contribute to neurodegenerative phenotypes, and to understand how this knowledge can help develop therapeutic strategy for HSP.
Introduction
Hereditary spastic paraplegias (HSP) are a group of rare neurodegenerative diseases characterized by weakness of lower limbs and spasticity (Harding, 1983). These symptoms are due to the degeneration of the long axons of the neurons from the motor cortex. Since the longest axons seemed to be more sensitive to neurodegeneration, it was proposed that altered intracellular trafficking could underlie the physiopathology of HSP (Soderblom and Blackstone, 2006). HSP are clinically heterogeneous, and complex forms of HSP are associated with various other neurological symptoms such as cognitive impairment or ataxia, due to degeneration of other neuronal populations. This clinical heterogeneity is partly underlined by the genetic heterogeneity of this group of diseases. Indeed, in the last decade, the emergence of new genetic tools allowed the identification of many genes responsible for HSP (Boutry et al., 2019a). Among the recently identified genes, many encode enzymes that are directly implicated in the metabolism of lipids. Since brain is mainly composed of lipids, identification of such lipid-related pathways opens new perspectives to evaluate the physiopathology of HSP. Importantly, beside the identification of genes encoding lipid-modifying enzymes, mutations in genes responsible for HSP and coding proteins involved in membrane trafficking can also alter lipid homeostasis in some subcellular compartments. The formation of myelin, the most prominent lipidic structure in the brain, also appears to play a critical role in the maintenance of axon in several forms of HSP. Therefore, it becomes evident that lipids are of critical importance for the physiopathology of almost all HSP. In this review, we propose in a first part an overview of the various metabolic pathways that are altered by mutations in genes responsible for HSP and HSP-related disorders such as leukodystrophies presenting with spasticity. In a second part, we examine the consequences of altered lipid metabolism on cellular functions to highlight various physiopathological pathways that could be altered in the different forms of HSP.
Alterations of Lipid Metabolism Due to HSP-Causing Mutations
The emergence of next-generation sequencing in the last decade allowed the identification of many genes responsible for HSP. Among all the newly identified genes, a quarter of them are encoding enzymes that are directly involved in the metabolism of lipids (Table 1). It appears that all the main classes of lipids could be implicated in the physiopathology of HSP.
Cholesterol
Cholesterol is a lipid highly enriched in the brain, as this organ contains about 20% of total body cholesterol (Dietschy and Turley, 2004). Most of the brain cholesterol enters in the composition of myelin, but cholesterol is also found in the membranes of glial and neuronal cells where it is actively trafficked.
Cholesterol Hydroxylation
The identification of mutations in cytochrome P450-7B1 (CYP7B1) in SPG5 patients (Tsaousidou et al., 2008) was the first indication that lipid metabolism could play a role in the physiopathology of HSP. SPG5 patients often present with pure HSP, but some of them may present with complicated forms of HSP and mild white matter abnormalities (Goizet et al., 2009; Marelli et al., 2018). CYP7B1 encodes a cytochrome P450 7α-hydroxylase responsible for the degradation of oxysterols. Consequently, loss of CYP7B1 leads to the accumulation of oxysterols such as 25-hydroxycholesterol, 26-hydroxycholesterol, 27-hydroxysterol, and 3β-hydroxy-5-cholestenoic acid (3β-CA) in serum and cerebrospinal fluid of SPG5 patients (Schüle et al., 2010; Theofilopoulos et al., 2014; Marelli et al., 2018). 3β-CA was demonstrated to exert toxic effects on rodent oculomotor neurons in vitro and zebrafish motor neurons in vivo via activation of liver X receptors (LXRs) (Theofilopoulos et al., 2014). In addition, an in vitro study using NSC-34 cell line and neurons derived from human induced pluripotent stem (iPS) cells showed that oxysterols and 3β-CA had a cytotoxic activity. In that study, 25-OHC and 27-OHC were harmful at concentrations comparable to levels measured in serum of SPG5 patients (Schöls et al., 2017). However, in both studies, the toxicity observed required levels of oxysterols that were much higher than the levels detected in the cerebrospinal fluid of SPG5 patients. The cytotoxic action of oxysterols are mainly due to their incorporation into the natural lipid bilayer, where they can change the interaction between molecules (Mitomo et al., 2009) and thus alter membrane properties. In both studies investigating the toxic accumulation of CYP7B1 substrates, oxysterols added to the medium likely partitioned between medium and membranes, and their real concentration in membranes were not analyzed. Furthermore, the concentration of oxysterols that need to be reached in the membranes to induce a deleterious effect are not known, and further investigations are required to evaluate the mechanisms underlying neurodegeneration in SPG5 patients. Based on the hypothesis that oxysterols are neurotoxic, two concomitant therapeutic trials were conducted in SPG5 patients with decreased plasma oxysterols as the primary outcome measure (Schöls et al., 2017; Marelli et al., 2018). In both short-term phase II trials, atorvastatin significantly decreased plasma 27-OHC (Schöls et al., 2017; Marelli et al., 2018). Furthermore, treatment with chenodeoxycholic acid (CDCA) restored bile acids profile in SPG5 patients (Marelli et al., 2018). However, the clinical benefit of such metabolic intervention remains to be established and surrogate markers are needed due to the very slowly progressive course and the rarity of this disease. Another clinical trial levels using monoclonal antibodies that inhibit proprotein convertase subtilisin-kexin type 9 (PCSK9) to reduce cholesterol levels has recently been initiated (Chen, 2019). It will monitor levels of 27-OHC as a primary outcome.
Another HSP related to defective cholesterol hydroxylation is cerebrotendinous xanthomatosis (CTX) due to CYP27A1 mutations, which encodes the mitochondrial cytochrome P450 enzyme sterol 27-hydroxylase. Deficiency in this enzyme interferes with sterol intermediates in the alternative bile acid pathway. More specifically, CTX is associated with reduced synthesis of 27-OHC and CDCA, as well as the shunting of sterol intermediates into the microsomal pathway for cholic acid formation (Salen et al., 1991). CTX is also characterized by high production of cholestanol, which accumulates in various tissues, as well as increased levels of bile alcohols in urine (Berginer et al., 1984). Evidence that cholestanol may be neurotoxic is supported by the finding of cholestanol deposition and apoptosis in neuronal cells in the cerebellum of rats fed a 1% cholestanol diet (Inoue et al., 1999). As the influx of 27-OHC may be involved in brain cholesterol homeostasis, the lack of 27-OHC may also impact cholesterol synthesis in the brain (Mignarri et al., 2016). About 60% of CTX patients (Wong et al., 2018) present with a complex form of HSP that includes systemic (infantile cholestasis, juvenile-onset cataracts, Achilles tendon xanthomas, chronic diarrhea, and osteoporosis) and/or neuropsychiatric symptoms (learning disability and/or autism spectrum disorder, cerebellar ataxia, peripheral neuropathy, parkinsonism, dementia, and psychiatric disturbances) (Nie et al., 2014). Importantly, there is a critical therapeutic window in most CTX patients before the onset of disabling neuropsychiatric symptoms (Yahalom et al., 2013). CDCA remains the treatment of choice in CTX as it down-regulates CYP27A1, restores the imbalance between CDCA and cholic acid, and is the only drug that has shown beneficial effects on neurological symptoms so far (Nie et al., 2014; Salen and Steiner, 2017). Several studies have emphasized that the response to treatment strongly depends on when CDCA is initiated (Amador et al., 2018; Duell et al., 2018; Stelten et al., 2019). In a cohort of 56 Dutch CTX patients treated by CDCA with a median follow-up time of 8 years, neurological symptoms, assessed by the modified Rankin Scale and Expanded Disability Status Scale (EDSS) scores, disappeared in all patients who were diagnosed before the age of 24 and treated since (Stelten et al., 2019). Furthermore, treatment prevented the development of new neurological symptoms during the follow-up period (Stelten et al., 2019).
Alteration of Cholesterol Synthesis or Trafficking in HSP
Oxysterols are present at very low levels compared to cholesterol (Wnętrzak et al., 2017), and alterations of cholesterol synthesis or trafficking are induced by the loss of function of several genes responsible for HSP.
For example, loss of function of Erlin1 and Erlin2, mutated in SPG62 and SPG18, respectively, are responsible for early onset autosomal recessive HSP associated with cognitive impairment (Alazami et al., 2011; Novarino et al., 2014). Autosomal dominant mutations in Erlin2, associated with SPG37, are also implicated in less severe forms of motor neuron diseases (Rydning et al., 2018; Stevanin et al., 2019). Erlin1 and Erlin2 form a heteromultimeric complex of ∼2 MDa (Pearce et al., 2009). This complex is localized in the lumen of the ER and is anchored to cholesterol-rich lipid domains by the N-terminus of the proteins (Browman, 2006). Downregulation of Erlin1 and 2 was shown to activate the sterol regulatory element binding protein (SREBP) transcription factors, leading to upregulation of their target genes. This increased cholesterol content in cells and cells containing cytoplasmic lipid inclusions stained with Oil Red O (Huber et al., 2013). However, the relevance of this function for the physiopathology of HSP has not been evaluated yet.
Loss of spatacsin, due to mutations in SPG11, is responsible for a complicated form of HSP where spasticity is often associated with cognitive impairment and lower motor neuron degeneration. White matter anomalies may be detected on brain MRI (Hehr et al., 2007; Stevanin et al., 2007, 2008). Spatacsin is implicated in the autophagic lysosome reformation, a mechanism allowing the formation of tubules in autolysosomes at the end of the autophagy process in order to recycle lysosome membrane (Chang et al., 2014). Recently, it was shown that the formation of tubules on lysosomes mediated by spatacsin allows the recycling of cholesterol from lysosomes. Loss of spatacsin led to an accumulation of cholesterol in lysosomes and decreased cholesterol content in plasma membrane (Boutry et al., 2019b), which could affect membrane properties and function. This highlights that impaired cholesterol trafficking and not only cholesterol overload in lysosomes could contribute to the pathology. However, the contribution of this mechanism to neuronal death is still not known.
Alteration of Phospholipid Synthesis and Degradation in Some HSP Entities
Phospholipids are the main components of cellular membranes. They are composed of a glycerol backbone to which two fatty acids are attached as esters. The third alcohol group of the glycerol is attached to a phosphoric acid that can associate various hydrophilic moieties, determining the nature of the phospholipid (phosphatidyl-choline, phosphatidyl inositol, etc.) (Figure 1). Several enzymes implicated in the metabolism of phospholipids have been associated with HSP or related disorders.
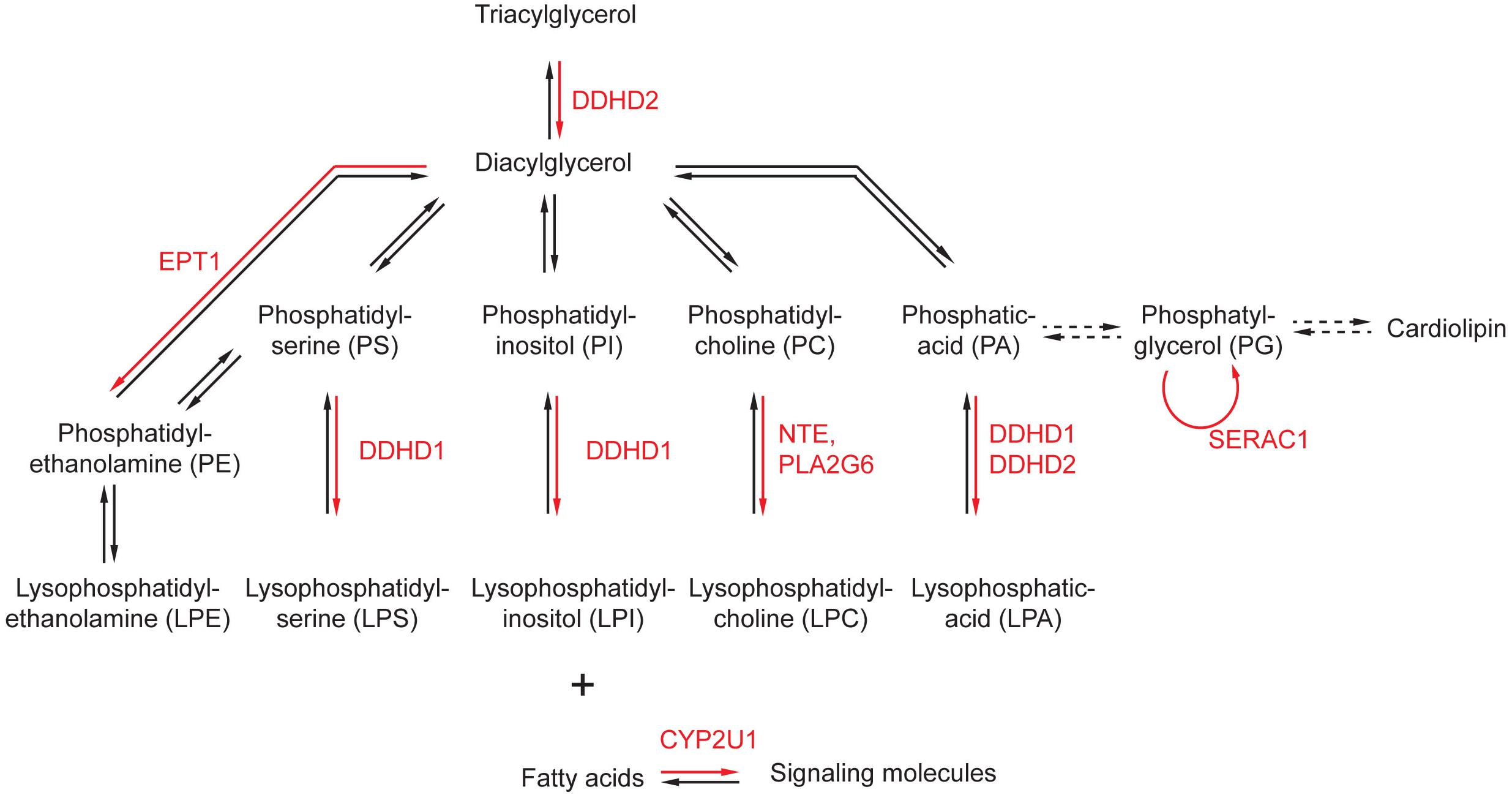
Figure 1. Alteration of phospholipid metabolism by genes implicated in the physiopathology of HSP. The main metabolic pathways affecting phospholipids and phospholipid-related molecules are represented. The enzymes affected by mutations in genes responsible for HSP are highlighted in red. Dashed arrows represent conversion requiring more than one enzymatic reaction.
Altered Synthesis of Phospholipids
Mutations in EPT1/SELENOI have been found in patients presenting an early onset complicated HSP with white matter abnormalities detected on brain MRI (Ahmed et al., 2017; Horibata et al., 2018). EPT1 is an ethanolamine transferase implicated in the synthesis of phosphatidylethanolamine (PE), the second most abundant phospholipid in membranes. Analysis of a patient’s fibroblasts as well as EPT1 knockout Hela cells showed decreased PE biosynthesis, as well as an imbalance in plasmalogens (increased plasmanyl-phosphatidylcholine and decreased plasmenyl-phosphatidylethanolamine) (Horibata et al., 2018). Of note, plasmenyl-phosphatidylethanolamine represents more than 50% of ethanolamine glycerophospholipids in the brain (Braverman and Moser, 2012), which highlights why the loss of EPT1 activity may lead to such severe brain dysfunction.
Mutations in SERAC1 were known to cause the autosomal recessive MEGDEL syndrome, characterized by infantile onset symptoms including feeding problem, liver failure, deafness, dystonia, encephalopathy, spasticity, and Leigh-like syndrome (Wortmann et al., 2012). Recently, mutations in SERAC1 were found in a family presenting juvenile onset complicated HSP (Roeben et al., 2018). SERAC1 is a phospholipid remodeling enzyme that converts phosphatidylglycerol 34:1 (PG34:1) into phosphatidylglycerol 36:1 (PG36:1). Families presenting with an HSP phenotype had a milder change in the PG34:1/PG36:1 ratio compared to patients with MEGDEL syndrome (Roeben et al., 2018), suggesting phenotype–genotype correlation.
Mutations in PGAP1 have been identified in one family of complex HSP (SPG67) (Novarino et al., 2014), but also in cases of intellectual disability and encephalopathy (Murakami et al., 2014). PGAP1 encodes an ER protein that promotes the deacylation of inositol in the glycosylphosphatidylinositol (GPI)-anchored proteins (Tanaka et al., 2004). During their synthesis, GPI-anchored proteins are localized in the lumen of the ER. The remodeling of GPI anchor by PGAP1 is required for the sorting of GPI-anchored proteins to ER exit sites, allowing their export out of the ER (Fujita et al., 2011).
Degradation of Glycerophospholipids
Several enzymes with phospholipase activity have been associated with HSP. Mutations in the genes encoding two calcium-independent phospholipases, NTE/PNPLA6 and PLA2G6, are responsible for a variety of neurodegenerative disorders. Mutations in neuropathy target esterase (NTE/PNPLA6) are responsible for autosomal recessive HSP (SPG39) (Rainier et al., 2008), but also other neurological syndromes combining ataxia, spasticity, retinal degeneration, and/or hypogonadism (Synofzik et al., 2014). PLA2G6 associated neurodegeneration is a group of heterogeneous neurodegenerative disorders, including infantile neuroaxonal dystrophy, neurodegeneration with brain iron accumulation, parkinsonism, and HSP. Both enzymes hydrolyze the sn-2 acyl chain of phospholipids. NTE/PNPLA6 seems to have a privileged affinity for phosphatidylcholine, as its levels were increased in the brain of nestin-cre:NTEfl/fl mice (Read et al., 2009). The product of PLA2G6 mediates the release of docosahexaenoic acid from brain phospholipids (Green et al., 2008), suggesting that different actions of phospholipases can contribute to the physiopathology of HSP.
Another group of phospholipases associated with the physiopathology of HSP are DDHD containing enzymes DDHD1 and DDHD2 (Schuurs-Hoeijmakers et al., 2012; Tesson et al., 2012). Mutations in DDHD1 are responsible for pure or complex HSP (SPG28), sometimes associated with brain iron accumulation (Dard et al., 2017). DDHD2 is mutated in SGP54, which is usually characterized by very early onset spastic paraplegia, intellectual disability, and white matter abnormalities (Schuurs-Hoeijmakers et al., 2012), but adult onset forms have been reported for both DDHD1 and DDHD2 (Doi et al., 2015; Dard et al., 2017). As a unique finding in DDHD2, proton magnetic resonance spectroscopy revealed an abnormal lipid peak in the basal ganglia and thalamus area (Schuurs-Hoeijmakers et al., 2012). In vitro experiments proposed that DDHD1 and DDHD2 have a phosphatidic acid-preferring phospholipase A1 action, hydrolyzing the sn-1 acyl chain (Higgs et al., 1998; Inoue et al., 2012). These results were challenged by lipidomic analysis of knockout mouse models for these two genes. Knockout of Ddhd2 led to accumulation of triacylglycerol, and recombinant DDHD2 was revealed to have a triacylglycerol-lipase activity (Inloes et al., 2014). Recently, lipidomic analysis of the brain of Ddhd1 knockout mice showed that phosphatidylinositol and phosphatidylserine are the main physiological substrates of DDHD1 (Inloes et al., 2018). It is conceivable that DDHD1 and DDHD2 have different substrates depending on the cell type that is investigated.
Metabolism of Phospholipid and Cellular Signaling
The metabolism of phospholipids can contribute to signaling pathways by modifying various lipid species. One of the most studied lipid signaling pathway relies on the phosphorylation state of phosphoinositides (Wang et al., 2019). Interestingly, loss of Ddhd1 in mice led to striking remodeling of phosphoinositides in the brain (Inloes et al., 2018). Furthermore, spastizin (ZFYVE26) mutated in SPG15 (Hanein et al., 2008) has a FYVE domain that mediates interaction with phosphatidylinositol-3-phosphate (PI3P). The ability of the spastizin FYVE domain to bind PI3P is required for addressing protein to lysosomes (Chang et al., 2014), even if the content of PI3P in this subcellular compartment is poorly characterized. Deletion of the FYVE domain or point mutation abolishing the interaction with PI3P impaired the localization of spastizin to lysosomes (Chang et al., 2014). Loss of phosphatidylinositol 4-kinase 2alpha activity in mouse caused neurological symptoms resembling HSP (Simons et al., 2009). In this mouse model, the authors revealed neurodegeneration that was associated to accumulation of lipofuscin-like material, similar to the observation made in Spg15 knockout mice (Khundadze et al., 2013). Although mutations in phosphatidylinositol 4-kinase 2 alpha have not been identified in HSP patients so far, this illustrates the role of signaling phosphoinositides in the physiopathology of HSP.
It is important to note that the phospholipases A1 or A2 may release lysosphospholipids such as lysophosphatidic acid or lysophosphatidyl-inositol and some of them play an important a role in cellular signaling (Yung et al., 2015). The phospholipases A2 hydrolyze phospholipids in the sn-2 position, releasing a fatty acid that is often unsaturated. Such fatty acid can be hydroxylated by the cytochrome P450 CYP2U1 that is mutated in the early onset form of complex HSP SPG49 (HUGO) or SPG56 (OMIM) (Chuang et al., 2004; Tesson et al., 2012). Oxidation of unsaturated fatty acids can produce a variety of signaling molecules, but their identities and roles are still to be uncovered. The role of such signaling molecules in the physiopathology of HSP has not been investigated so far, and it may open new avenues to identify targets of therapeutic interest for these diseases.
Alteration of Sphingolipid Metabolism in HSP
Sphingolipids are a group of lipids derived from the sphingosine backbone. They are synthesized in the ER and the Golgi apparatus, and are degraded by lysosomes (Figure 2). Alteration of both biosynthetic and degradative pathways of sphingolipids have been associated with HSP phenotype.
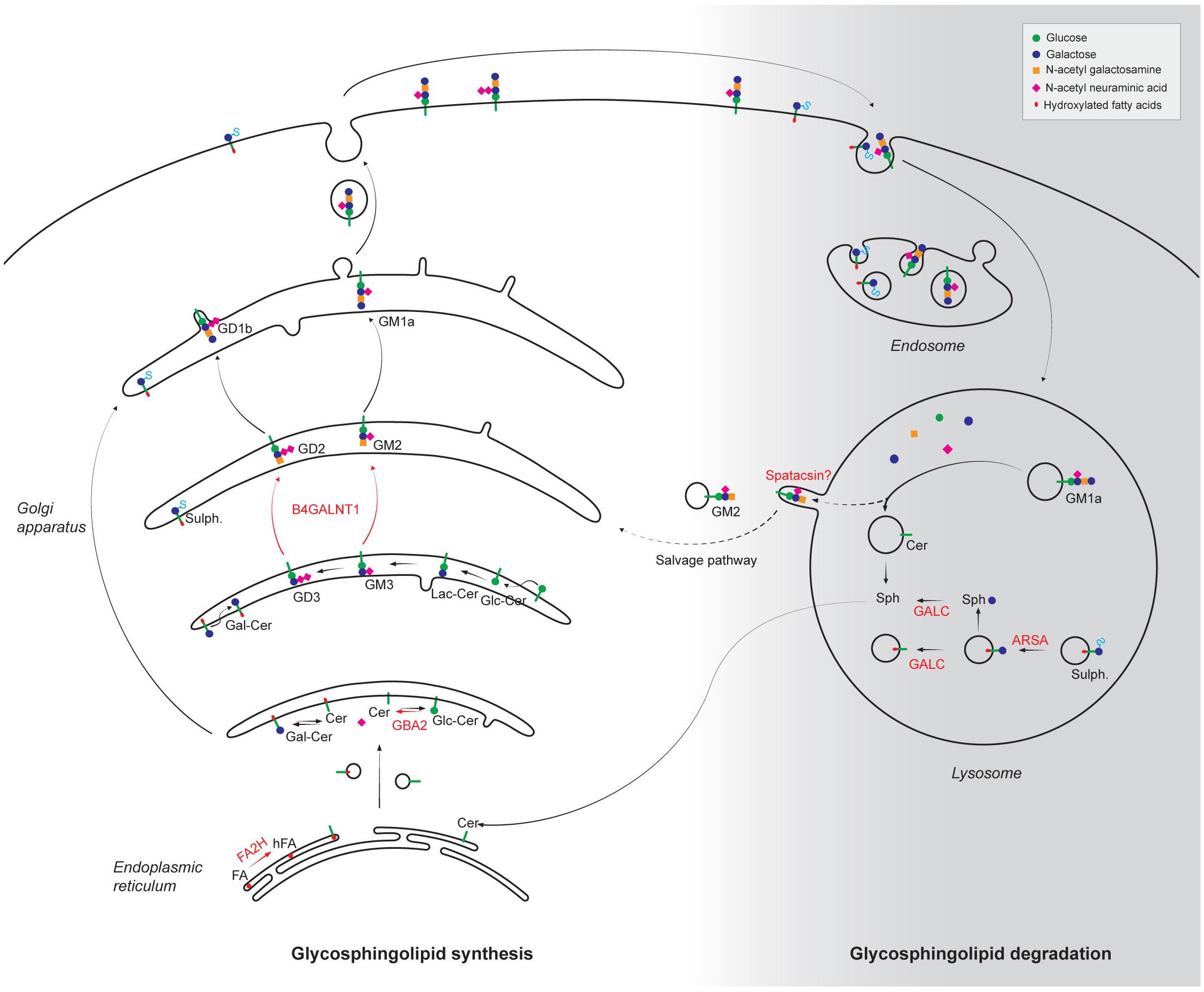
Figure 2. Alteration of synthesis and degradation pathways of glycosphingolipids in the physiopathology of HSP. The different steps of glycosphingolipid biosynthesis occur in the endoplasmic reticulum and the Golgi apparatus by progressive addition of the molecules indicated in the top right panel. Following internalization by endocytosis, plasma membrane glycosphinglipids are progressively degraded in lysosome into basic building blocks. The products of HSP-mutated genes that are implicated in the metabolism of glycosphingolipids are highlighted in red. FA, fatty acids; hFA, hydroxylated fatty acids; Cer, Ceramide; Gal-Cer, Galatosyl-ceramide; Glc-Cer, Glucosyl-ceramide; Lac-Cer, Lactosyl-ceramide; Sulf: Sulfatides; Sph, Sphingosine; FA2H, fatty acid-2-hydroxylase; GBA2, β-glucosidase 2; B4GALNT1, β-1,4-N-acetyl-galactosaminyltransferase; GALC, Galactosyl-cerebrosidase; ARSA, Arylsulfatase A. It has been proposed that some gangliosides could be recycled from the lysosomes using a salvage pathway. Whether spatacsin could be implicated in such pathway is still unclear.
Sphingolipid Biosynthesis
Loss-of-function mutations in fatty acid-2-hydroxylase (FA2H) are responsible for the complex form of autosomal recessive HSP (SPG35) (Edvardson et al., 2008) and allelic neurological disorders. A recent study showed that patients with nonsense or missense mutations presented a rather homogeneous phenotype, with a spastic tetraparesis frequently associated with cognitive deficits. Symptoms onset is in early childhood and all patients presented white matter abnormalities on brain MRI (Rattay et al., 2019). FA2H is the enzyme responsible for the formation of 2-hydroxy-fatty acids (Alderson et al., 2004) that are frequently incorporated as N-acyl chain in galactosylceramide (Bowen and Radin, 1968). In mouse models, the loss of function of Fa2h was associated with the absence of sphingolipids containing 2-hydroxylated fatty acids and increased non-hydroxylated galactosylceramide (Zoller et al., 2008).
Gangliosides are synthesized in the ER. These glycosphingolipids are highly enriched at the plasma membranes of neurons and glial cells, notably complex gangliosides such as GM1 and GD1. These molecules serve as coreceptors for many factors. B4GALNT1 is mutated in SPG26 that is characterized by complex HSP frequently associated with intellectual disability (Boukhris et al., 2013). B4GALNT1 encodes β-1,4-N-acetyl-galactosaminyl transferase 1 or GM2/GD2 synthase that converts GM3 into GM2 and GD3 into GD2. Accordingly, analysis in fibroblasts of SPG26 patients showed a lack of GM2 associated with increased levels of its precursor GM3 (Harlalka et al., 2013). Knockout of the GM2 synthase in mouse leads to a progressive demyelination and axonal loss that lead to motor dysfunction (Sheikh et al., 1999; Chiavegatto et al., 2000), similar to the symptoms observed in patients.
Other forms of HSP are putatively linked to sphingolipid metabolism, although the roles of the mutated gene products are still elusive. Deficiency in carnitine palmitoyltransferase 1C (CPT1C) accounts for SPG73 and has been associated with sphingolipid metabolism (Rinaldi et al., 2015). This enzyme belongs to a family mediating the trans-esterification of palmitoyl-coA and carnitine, to allow the formation of a molecule that can be transported through membranes, in contrast to palmitoyl-CoA. While CPT1A and CPT1B are enriched in mitochondria and allow the transport of acyl-CoA into mitochondria to undergo β-oxidation, CPT1C is localized in the ER and its carnitine palmitoyl transferase activity is low compared to CPT1A and CPT1B (Casals et al., 2016). In contrast, a lipidomic analysis in the brain of fasted Cpt1c knockout mice showed lower levels of ceramide and sphingosine (Carrasco et al., 2012). The molecular mechanisms leading to this change in sphingolipid metabolism are however unknown.
A missense mutation in SLC33A1 (p. S113R) has been observed in a large family of patients presenting with an autosomal dominant spastic paraplegia (SPG42) (Lin et al., 2008). SLC33A1 encodes the acetyl-CoA transporter 1 (AT-1) localized in the ER, which has been proposed to play a key role in the acetylation of proteins but also of gangliosides such as O-Ac-GD3 or O-Ac-GT3 (Kanamori et al., 1997). The p. S113R mutant is supposed to disrupt the second transmembrane domain of the protein, thus blocking its function (Lin et al., 2008). A knock-in mouse model expressing the p.S113R mutant at the heterozygous state presents impaired locomotion at the age of 12 months (Liu et al., 2017). This phenotype is associated with a demyelination and alteration of ER and mitochondria in the cell body of neurons. However, whether the synthesis of O-Ac-GD3 or O-Ac-GT3 was decreased in this mouse model has not been investigated. Acetyl-coA has also been associated with fatty acid metabolism (Yoshii et al., 2015), but it has not been investigated whether fatty acid metabolism is impaired in SPG42 patients or in the Spg42 mouse model.
Sphingolipid Degradation
Krabbe disease and metachromatic leukodystrophy are two autosomal recessive diseases due to impaired lysosomal degradation of sphingolipids. Both are progressive diseases, usually characterized by spasticity, often related to brain white matter lesions detected on brain MRI—peripheral neuropathy and cognitive impairment (Orsini et al., 2000; Gomez-Ospina, 2006). Krabbe disease is caused by loss of function of galactosylcerebrosidase (GALC) (Rafi et al., 1995). GALC degrades galactosylceramide, the major sphingolipid of myelin, as well as other sphingolipids containing galactose such as psychosine (D-galactosylsphingosine). In the absence of a functional GALC, galactosylceramide can be degraded by GM1 ganglioside β-galactosidase (Kobayashi et al., 1985) and thus do not accumulate in cells of Krabbe disease patients. In contrast, psychosine cannot be metabolized by GM1 ganglioside β-galactosidase and thus accumulates in the brain of Krabbe patients (Svennerholm et al., 1980). In the Twitcher mouse, a natural model of Krabbe disease, a biochemical analysis revealed decreased levels of galactosylceramide and sulfatides at early stages, as well as strong accumulation of psychosine (Igisu et al., 1983; Igisu and Suzuki, 1984). Metachromatic leukodystrophy is due to a deficiency in arylsulfatase A (Gomez-Ospina, 2006). This enzyme mediates the degradation of a class of glycosphingolipids, sulfatides (Mehl and Jatzkewitz, 1968). Consequently, metachromatic leukodystrophy patients present high levels of sulfatides in many tissues, including the brain, and they excrete high levels of sulfatides in urines (Gomez-Ospina, 2006). A mouse model deficient in arylsulfatase presents with early onset accumulation of sulfatides in lysosomes of neurons and oligodendrocytes (Wittke et al., 2004). In both Krabbe disease and metachromatic leukodystrophy, allogenic hematopoietic stem cell transplantation (HSCT) can stabilize/slow-down cerebral demyelinating lesions for pre- or early-symptomatic juvenile or adult patients (van Rappard et al., 2016; Laule et al., 2018).
Krabbe disease and metachromatic leukodystrophy belong to the group of lysosomal storage disorders. However, accumulation of some glycosphingolipids in lysosomes has also been observed in SPG11 models of HSP (Boutry et al., 2018) that is not classically considered as a lysosomal storage disorder. Accumulation of gangliosides in SPG11 was likely not due to impaired function of ganglioside-degrading enzymes, suggesting that the accumulation of gangliosides has another cause. SPG11 is due to the loss of function of spatacsin, a protein that has been implicated in the autophagic lysosome reformation, a mechanism allowing formation of tubule in autolysosomes at the end of the autophagy process to recycle lysosome membrane (Chang et al., 2014). It has been proposed that inhibition of the tubulation in absence of spatacsin could lead to accumulation of gangliosides in lysosomes, which could contribute to the motor dysfunction observed in the mouse model (Branchu et al., 2017; Boutry et al., 2018). Some SPG11 patients are diagnosed as cases of amyotrophic lateral sclerosis or Charcot-Marie tooth disease (Orlacchio et al., 2010; Montecchiani et al., 2016). Of note, the levels of several glycosphingolipids, including gangliosides, were upregulated in the spinal cord of ALS patients, suggesting that they could participate to neurodegeneration in this disease as well (Dodge et al., 2015). SPG11 patients also frequently present parkinsonian features (Anheim et al., 2009). It is interesting to note that the main risk factor identified so far for Parkinson’s disease is the heterozygous loss of GBA1 (Aharon-Peretz et al., 2004). This gene encodes lysosomal glucosylceramidase, which is a lysosomal enzyme implicated in the degradation of glycosphingolipids downstream the degradation of gangliosides.
Mutations in GBA1 have not been found in HSP patients, but loss of its paralog GBA2 is responsible for spastic ataxia with cognitive impairment (SPG46) (Hammer et al., 2013; Martin et al., 2013). GBA2 is a non-lysosomal glucosylceramidase, hydrolyzing glucosylceramide into glucose and ceramide. Most mutations impair the enzymatic function of GBA2 and can thus be considered from an enzymatic perspective as null mutants (Sultana et al., 2015). Gba2 knockout mice exhibit gait abnormalities (Woeste et al., 2019) and fertility impairment due to abnormal sperm morphology (Raju et al., 2015). This is consistent with male infertility reported in SPG46 patients (Martin et al., 2013). Lipidomic analysis in Sertoli cells confirmed that loss of GBA2 promotes accumulation of glucosylceramide (Raju et al., 2015). In cerebellum, no difference was observed in the total levels of glucosylceramide between control and Gba2 knockout mice. However, levels of the gangliosides GM1a, GT1b, GD1b, and GM3 were slightly increased in the cerebellum of Gba2 knockout mice compared to wild-type mice (Woeste et al., 2019). Yet, the link between accumulation of glucosylceramide and neurodegeneration is not known. It was proposed that accumulation of glucosylceramide could alter the membrane fluidity, which could lead to increased actin polymerization (Raju et al., 2015).
Alteration of Fatty Acid Metabolism in Syndromes Presenting Spasticity
Several rare neurodegenerative diseases presenting spasticity are implicated in the metabolism of fatty acids. Fatty acids are building blocks for other lipids such as phospholipids, ceramide, cholesterol esters, and thus change in fatty acid metabolism can have an impact on many classes of lipids as illustrated below.
X-linked adrenoleukodystrophy (X-ALD) is an X-linked syndrome caused by mutations in the ABCD1 gene that codes for the adrenoleukodystrophy protein (ALDP) (Mosser et al., 1993). First, symptoms may occur from childhood to adulthood, leading to progressive demyelination of the central and peripheral nervous system. The earlier the illness begins, the more severe it will be and childhood forms of X-ALD are characterized by a devastating cerebral demyelination, leading to rapid cognitive and motor decline, vegetative state within a few months, and premature death. The clinical spectrum in males with X-ALD ranges from childhood cerebral ALD (CALD: onset 5–12 years, 35–40% of affected males) to slowly progressive spastic paraplegia in adulthood (adrenomyeloneuropathy: onset 20–30 years, 60% of affected males) (Kemp et al., 2012). Approximately 20% of males with adrenomyeloneuropathy will also develop CALD later in life with the same poor prognosis as in children. The only available therapy for CALD is allogenic HSCT (Raymond et al., 2019; Waldhüter et al., 2019). If performed in patients with minimal brain lesions and no/minor clinical symptoms, it may arrest the progression of demyelinating lesions. To overcome the limitations of allogeneic transplantation (lack of donor, graft versus host reaction, high mortality rate), autologous transplantation of HSC corrected ex vivo with a lentiviral vector expressing the ABCD1 gene is currently under clinical trial in children with CALD with very encouraging results (Eichler et al., 2017). Women carriers of ABCD1 mutations may develop milder forms of the disease, usually with spastic paraplegia, and sometimes peripheral neuropathy, in adulthood (Huffnagel et al., 2019). However, it is increasingly recognized that ABCD1-mutated women develop a wide spectrum of neurological diseases with age (Engelen et al., 2014). All male patients present elevated plasma levels of very long chain fatty acids (VLCFA) but they may be normal in female carriers. The accumulation of VLCFA is also detected in lipid molecules or proteins complexing fatty acids. The myelin of X-ALD patients showed a strong enrichment in VLCFA complexed in cholesterol esters and sphingomyelin (Brown et al., 1983), an increased proportion of proteolipid protein (PLP) complexed to monounsaturated VLCFA (Bizzozero et al., 1991), and increased amount of VLCFA in glycerophospholipids (Theda et al., 1992). VLCFA accumulation is also associated with clinically significant microglial activation (Eichler et al., 2008). These observations highlight the variety of effects that can result from the accumulation of VLCFA. This accumulation in X-ALD patients is the consequence of the impaired β-oxidation of VLCFA by peroxisomes. The ABCD1 product, ALDP, is a membrane transporter of the ATP-binding cassette family of proteins and is localized in peroxisomes (Watkins et al., 1995). It was shown that ALDP can transport the VLCFA from the cytosol across the peroxisome membrane, allowing their β-oxidation (van Roermund et al., 2008). VLCFA accumulation in X-ALD patients is the consequence of the absence of functional ALDP protein.
VLCFA can be obtained from food, but they can also be synthesized in the ER by elongases that are enzymes allowing the elongation of fatty acids (Jakobsson et al., 2006). Heterozygous mutations in the gene encoding the elongase ELOVL4 was associated with autosomal dominant juvenile macular degeneration (Zhang et al., 2001), or spinocerebellar ataxia SCA34 (Ozaki et al., 2015). However, recessive mutations in ELOVL4 were found in patients presenting ichthyosis, seizures, mental retardation, and spastic paraplegia (Aldahmesh et al., 2011). Deficiency in ELOVL4 in mice led to perinatal death and was associated with a reduction in VLCFA (Cameron et al., 2007), as well as decreased levels of ceramide with omega-hydroxy VLCFA (Li et al., 2007). Recently, a dominant mutation in ELOVL1 was found in two pediatric patients presenting ichthyosis, spasticity, mild hypomyelination detected on brain MRI, and dysmorphic features (Kutkowska-Kaźmierczak et al., 2018). Expression of the pathogenic variant in cell lines reduced the production of VLCFA (Kutkowska-Kaźmierczak et al., 2018). Analysis of patient cells also revealed a moderate decrease in the levels of ceramide with fatty acids longer than 24 carbons (Mueller et al., 2019). Consistently, knockout of Elovl1 in mice resulted in decreased levels of ceramide with VLCFA (Sassa et al., 2013). The X-ALD and elongase-linked syndromes highlight the importance of the balance between synthesis and degradation of VLCFA for proper brain function. The interplay between the two cellular function was also demonstrated by the beneficial action of downregulating ELOVL1 in fibroblasts of X-ALD patients (Ofman et al., 2010).
Sjogren Larsson syndrome (SLS) is an autosomal recessive disorder characterized by ichthyosis, spastic paraplegia, and intellectual disability (Sjogren and Larsson, 1957). This syndrome results from the accumulation of fatty aldehydes (Rizzo and Craft, 1991). Consistently, mutations in ALDH3A2 that encodes a fatty aldehyde dehydrogenase were identified as responsible for SLS (Laurenzi et al., 1996). It was demonstrated that fibroblasts of SLS patients are more sensitive to cell death induced by fatty aldehydes (James and Zoeller, 1997). This effect could be due to reactions between accumulating fatty aldehydes and proteins or lipids such as phosphatidylethanolamine that would disrupt their biochemical functions (James and Zoeller, 1997).
Mutations in genes encoding enzymes involved in the metabolism of various lipid molecules highlight the role of lipids in the physiopathology of HSP. It is important to note that both synthesis and degradation of most classes of lipids are responsible for diseases with overlapping presentation. This highlights the importance of lipid homeostasis for the normal function of polarized cells like neurons or oligodendrocytes. However, it is not clear how the change in lipid metabolism can lead to axon loss or neurodegeneration. Some functional links have been established from the study of other forms of HSP, as discussed in the next part of this review.
Functional Consequences of the Alteration of Lipid Metabolism
Lipids being major membrane constituents, alteration of their metabolism is likely to alter various membrane-related functions. We will see that alterations of lipid metabolism can affect various cellular functions (Table 2), which could contribute to neurodegeneration. However, it has also emerged in the last few years that mutations in genes affecting various subcellular compartments can secondarily lead to alteration of lipid metabolism, reinforcing its implication in the physiopathology of HSP.
Lipid Droplets
Lipid droplets (LD) are storage organelles that are formed by a core of neutral lipids surrounded by a monolayer of phospholipids. The main lipids stored in LDs are triacylglycerols (TAG) and esterified cholesterol. LDs formation occurs in the membrane of the ER. They are dynamic organelles, playing a key role in lipid metabolism and energy homeostasis (Olzmann and Carvalho, 2019).
Consistent with the formation of LD in the ER membrane, several proteins implicated in the morphogenesis of the ER network have been associated with LD biogenesis or have been shown to modify their dynamics. When overexpressed, atlastin and REEP1 are localized at the rim of LD, and the coexpression of both proteins increases the size of LD (Klemm et al., 2013). Similarly, M1 spastin, but not M87 spastin, was colocalized with LD, and overexpression of the M1 spastin isoform increased the size of LD in HeLa cells, as well as in Drosophila fat body, muscle, and nerve tissues (Papadopoulos et al., 2015). Conversely, downregulation of spastin in Drosophila or Caenorhabditis elegans decreased the number of LD and TAG levels (Papadopoulos et al., 2015). Atlastin loss of function in C. elegans or Drosophila reduced LD size and TAG levels (Klemm et al., 2013). Reep1 knockout mice also presented with a lipoatrophy, associated with reduced levels of serum cholesterol and TAG. The number of LD was also reduced in neurons of Reep1 knockout mice (Renvoisé et al., 2016). Since atlastin-1, spastin, and Reep1 are ER-shaping proteins (Park et al., 2010), these studies nicely show a correlation between ER morphology and accumulation of LD. CPT1C, an atlastin binding protein, has not been shown to alter ER morphology, but its knockout in mouse also decreased the number and size of LD (Rinaldi et al., 2015). It is noteworthy that SPG3 (atlastin-1), SPG4 (spastin), SPG31 (REEP1), and SPG73 (CPT1C) are rather pure forms of HSP. Yet, it is not known whether the alteration of ER morphology or the alteration of LD metabolism is responsible for patients’ phenotype. Recently, it was proposed that M1 spastin promotes the docking of LD to peroxisomes, allowing the transfer of fatty acids from LD to peroxisomes. Expression of a mutant spastin prevented this transfer and led to accumulation of peroxided lipids, which could contribute to cell death (Chang et al., 2019).
Spartin and seipin, which are mutated in the complex forms of HSP SPG20 and SPG17, respectively, were also found in contact with LD (Szymanski et al., 2007; Eastman et al., 2009). Spartin interacts with the E3 ubiquitin ligases AIP4 and AIP5 (Edwards et al., 2009), and it was proposed to promote the ubiquitylation of lipid droplet proteins (Hooper et al., 2010). Downregulation of spartin leads to increased LD number and size (Eastman et al., 2009). Seipin is also implicated in the regulation of ER-LD contact and loss of seipin function impaired the formation of LD in fibroblasts (Szymanski et al., 2007; Salo et al., 2016). However, SPG17 is due to gain-of-function mutation in the BSCL2 gene, altering glycosylation sites of the protein, and the consequences of these mutations on LD metabolism have not yet been investigated.
Another complex form of HSP associated with the accumulation of LD is SPG54. LD accumulated prominently in neurons when the main brain TAG lipase, DDHD2, is knocked out (Inloes et al., 2014). The loss of Ddhd2 in mice was also responsible for motor and cognitive impairment. In this mouse model, LD accumulated in neurons, including axons and dendrites (Inloes et al., 2014). It was proposed that accumulation of LD in neuronal processes can lead to swellings and disturb intraneuronal trafficking, which could contribute to neurodegeneration. Ddhd2 knockdown in Drosophila reduced the number of active zones at synaptic terminals (Schuurs-Hoeijmakers et al., 2012). LD may thus disrupt trafficking of proteins and lipids required for synapse assembly (Pennetta and Welte, 2018).
Interestingly, these studies suggest that mutations leading to reduced LD are associated with rather pure forms of HSP. In contrast, other forms of HSP that were associated with accumulation of LD are rather complex HSP. However, it is not clear whether the severity of the phenotype is only due to alteration of LD. Indeed, LD are in interaction with other subcellular compartments such as mitochondria or lysosomes that can also be affected and could contribute to the physiopathology of HSP.
Mitochondria Dysfunction
Mitochondria play a key role in lipid metabolism, as they can degrade lipids through β-oxidation, or contribute to synthesis of TAG (Benador et al., 2019). It is interesting to note that loss of DDHD2 or spartin that are associated with accumulation of LD also leads to mitochondrial dysfunction (Joshi and Bakowska, 2011; Maruyama et al., 2018). Yet, neurons are not known to utilize lipids as an energy source for mitochondria, raising the question of other possible roles of lipids in the regulation of mitochondrial function. Alternatively, motor neuron dysfunction could also result from altered energy supply from glial cells that use lipids as energetic substrates.
DDHD1 and DDHD2 were initially described as phosphatidic acid (PA)-preferring phospholipases A1 (Higgs et al., 1998; Inoue et al., 2012). The content of PA in outer mitochondrial membrane can regulate the dynamics of mitochondria fusion and fission (Kameoka et al., 2018). Knockdown of DDHD1 or DDHD2 in some cells types, but not all of them, promoted elongation of mitochondria, probably by enhancing their fusion (Baba et al., 2014). The equilibrium between fusion and fission of mitochondria is required to clear damaged mitochondria (Twig et al., 2008). Loss of DDHD2 in mouse embryonic fibroblasts increased mitochondrial membrane potential and reduced ATP production and O2 consumption. These mitochondrial dysfunction were associated with production of reactive oxygen species (Maruyama et al., 2018). Similar to loss of DDHD2, lymphoblasts of SPG28 patients devoid of DDHD1 also presented higher ROS production and lower ATP production compared to control cells (Tesson et al., 2012). Excessive fusion observed in the absence of DDHD1 or DDHD2 could explain the accumulation of dysfunctional mitochondria and thus impaired ATP production, which could contribute to neurodegeneration.
Importantly, the alteration of mitochondrial function in DDHD2 knockout fibroblasts was not associated with the accumulation of LD or increased levels of TAG, but it was associated with decreased levels of cardiolipin, a mitochondria-specific lipid class (Maruyama et al., 2018). Cardiolipin can regulate the fusion of mitochondria and the formation of mitochondrial respiratory supercomplexes (Mileykovskaya and Dowhan, 2014; Kameoka et al., 2018). In fibroblasts of patients with SERAC1 mutations (MEGDEL syndrome), the levels of cardiolipin were increased compared to controls. This was associated with a dysfunction of the mitochondrial respiratory chain (Wortmann et al., 2012). It has not been investigated whether mitochondrial function was altered in fibroblasts of HSP patients with SERAC1 mutations. Spartin, implicated in SPG20, was also shown to interact with cardiolipins and was present on the outer mitochondrial membrane. Downregulation of spartin decreased mitochondrial membrane potential (Joshi and Bakowska, 2011), but it is not clear whether this effect is caused by a change in the levels of mitochondria lipid composition.
In vitro experiments showed that exposition to VLCFA induced death of astrocytes and oligodendrocytes (Hein et al., 2008). VLCFA exposition deregulated intracellular calcium homeostasis in neurons, astrocytes, and oligodendrocytes, but the latter were the most affected cell type (Hein et al., 2008). VLCFA exposition induced mitochondrial depolarization and reactive oxygen species production (Hein et al., 2008), which could contribute to oligodendrocyte death. Consistently, astrocytes derived from Abcd1 knockout mice exhibited impaired energy metabolism and increased ROS production when exposed to supraphysiological concentration of VLCFA (Kruska et al., 2015).
Change in mitochondrial function was also observed in fibroblasts and lymphoblasts from SPG49/56 patients. In the absence of functional CYP21U, the oxygen consumption was reduced and was associated with increased oxidative stress (Tesson et al., 2012). The subcellular localization of CYP2U1 is still not clear. This cytochrome was proposed to metabolize arachidonic acid into 19- and 20-HETE (Chuang et al., 2004), or to oxidize the endocannabinoid N-arachidonoylserotonin to downregulate its action (Siller et al., 2014). However, it is not known whether these molecules can regulate mitochondrial function directly or whether this is mediated by other metabolites, not yet identified, of this cytochrome P450.
Lysosomal Dysfunction
The clearest link between alteration of lysosome function and spasticity is provided by Krabbe and metachromatic leukodystrophy patients. Indeed, these two pathologies belong to the group of lysosomal storage disorders. Both diseases result from the loss of activity of a lysosomal enzyme leading to abnormal accumulation of substrates: psychosine (Krabbe) and sulfatides (Metachromatic leukodystrophy). Why such accumulation of substrates leads to spasticity is not clear.
Several other forms of HSP have also been proposed to be associated with impaired lysosomal function. Mutations of ATP13A2 were found in families with complex hereditary spastic paraplegia (SPG78) (Estrada-Cuzcano et al., 2017). ATP13A2 is a P5ATPase that is mainly localized in the membrane of lysosomes (Ramirez et al., 2006), and dysfunction of this protein was shown to be responsible for impaired lysosomal degradation (Dehay et al., 2012). Consistently, homozygous mutations in fibroblasts of SPG78 patients increased the number of lysosomes and impaired lysosomal degradative activity, and electron microscopy analysis revealed that lysosomes accumulated abnormal material consisting of whirls and stacks of membranes (Estrada-Cuzcano et al., 2017). This was reminiscent of neuronal ceroid lipofuscinosis, also found in patients mutated in this gene (Bras et al., 2012). Similar accumulation of lysosomal membranes was also observed in fibroblasts of SPG48 patients with loss-of-function mutation in the ζ subunit of the AP-5 complex (Hirst et al., 2015). Electron microscopy analysis of fibroblasts of SPG15 patients and neurons of Spg15 knockout mice showed the accumulation of zebra or fingerprint bodies (Khundadze et al., 2013; Renvoisé et al., 2014), which are lysosomes with accumulation of membranes similar to those found in some lysosomal storage disorders (Parkinson-Lawrence et al., 2010). Analysis of SPG11 patient fibroblasts, in contrast, did not reveal accumulation of lamellar structures by electron microscopy. However, in brains of Spg15, Spg11, and Spg48 knockout mice, ultrastructural analysis showed the presence of electron dense deposits that looked like lipofuscin (Khundadze et al., 2013, 2019; Varga et al., 2015; Branchu et al., 2017). In all three models, the presence of these deposits was proposed to result from the accumulation of autolysosomes or impaired autophagic clearance. In the case of Spg11 knockout mice, the lysosomal deposits were shown to contain lipids, and a lipidomic analysis revealed that the lysosomes accumulated simple gangliosides (Boutry et al., 2018). Whether gangliosides accumulate upon loss of function of spastizin or AP-5ζ is not known. Likewise, it is not clear why gangliosides accumulate in absence of spatacsin, the product of SPG11. It was proposed that such accumulation could be a consequence of impaired lysosomal membrane recycling (Boutry et al., 2018), but other mechanisms could contribute to this accumulation. For example, the levels of bis-monoacylglycerol-phosphate (BMP), which facilitates the degradation of GM2 ganglioside (Anheuser et al., 2015), were reduced in fibroblasts of patients with SERAC1 mutations compared to controls (Wortmann et al., 2012). Importantly, mutations in SPG11, SPG15, SPG48, ATP13A2, and SERAC1 have been associated with parkinsonism in some patients (Ramirez et al., 2006; Anheim et al., 2009; Schicks et al., 2011; Hirst et al., 2016; Ma et al., 2018). The clinical overlap between these patients, as well as the similarities observed in the lysosomal dysfunction suggest that these HSP entities may share some physiopathological pathways, although some differences may exist between them (Vantaggiato et al., 2019). Accumulation of membrane structures in lysosomes has also been observed in cells derived from Spg4 or Spg31 knockout mice. These defects resulted from impaired ER-mediated endosomal tubule fission (Allison et al., 2017). Yet, it is not clear whether these lysosomal structures share similarities in composition with the lysosomes accumulating membranes observed in fibroblasts of SPG11, SPG15, SPG48, or ATP13A2-deficient patients.
In SPG11 models, decreased gangliosides synthesis prevented neuronal death in vitro and improved the motor phenotype in a zebrafish model, suggesting that accumulation of gangliosides could contribute to neurodegeneration (Boutry et al., 2018). These results were notably obtained using miglustat, a non-specific inhibitor of glucosylceramide synthase that is used in the treatment of Gaucher disease. However, miglustat poorly crosses the blood–brain barrier and is not efficient for symptoms of neuropathic Gaucher Disease (Schiffmann et al., 2008). Furthermore, in a mouse model of Sandhoff disease, miglustat increased the levels of brain glycosphingolipids (Ashe et al., 2011), questioning its efficacy in the central nervous system. Decreasing ganglioside synthesis is thus of therapeutic interest for SPG11 patients, but it is still unsure whether miglustat is a good therapeutic option for these patients. Further studies are thus required to validate this therapeutic strategy. If such a therapy was efficient in SPG11, it would be worth validating whether a similar strategy could also be efficient in other forms of HSP presenting alteration of lysosomal function and a phenotypic presentation overlapping with SPG11 patients. Besides synthesis of gangliosides, other studies have shown a beneficial effect of clinically used GSK3 blocker tideglusib on the neurodevelopmental phenotype observed in SPG11 models (Mishra et al., 2016; Pérez-Brangulí et al., 2019). However, it is not known yet whether such a treatment would affect the metabolism of gangliosides or act on a different pathway. It has also not been evaluated whether restoration of normal development in SPG11 could prevent neurodegeneration that occurs later in life of SPG11 patients.
Alteration of Synaptic Function
Neuropathological investigations on autopsic cases of HSP have proposed a “dying back” mechanism of axonal degeneration (DeLuca et al., 2004). Such mechanisms would be compatible with a primary alteration of synaptic function. Exocytosis and endocytosis of synaptic vesicles have been thought to be mainly mediated by proteins, but it has emerged that lipid composition of membranes and lipid mediators also play a key role in these processes (Darios et al., 2007; Mochel, 2018). It is therefore tempting to speculate that alteration of lipid metabolism observed in some HSP would impair synaptic function and underlie some symptoms of the disease. For example, the product of PLA2G6, the calcium-independent phospholipase A2 β (iPLA2β), is localized in axon terminals and dendritic spines of neurons (Ong et al., 2005) where it releases polyunsaturated fatty acids (PUFAs) from brain phospholipids (Green et al., 2008). Accordingly, brains of Pla2g6 knockout mice present alteration of some PUFAs (Beck et al., 2011). Ultrastructural analyses of the posterior horn in this model showed the presence of loose presynaptic membranes containing synaptic vesicles (Beck et al., 2011). This insufficient membrane remodeling was proposed to contribute to the generation of axonal spheroids (Beck et al., 2011). Synaptic dysfunction could also underlie early behavioral symptoms in these mice, before the onset of neurodegeneration. Similarly, deficiency in NTE, another phospholipase associated with HSP, is associated with increased levels of phosphatidylcholine in brain—as described in the first part of the review—and a 20% decrease of protein secretion (Read et al., 2009). Alteration of the secretory pathway could affect synaptic vesicles and contribute to neuronal dysfunction long before neurodegeneration. Yet, iPLA2β and NTE have different lipid targets and may act by different mechanisms to regulate synaptic function. DDHD2, which was proposed to have a PLA1 action, also leads to synaptic defects in a drosophila model (Schuurs-Hoeijmakers et al., 2012). It would be interesting to further analyze how the loss of function of these enzymes contributes to synaptic dysfunction and underlie some early symptoms of HSP.
Alteration of synaptic function has also been observed upon expression of N88S seipin mutant in neurons, which decreased the number of synaptic vesicles docked to the plasma membrane. This alteration decreased the amplitude of evoked postsynaptic response (Wei et al., 2014). Yet, the link between the function of seipin in LD and the alteration of synaptic vesicles docking is unknown. Similarly, loss of spastin or atlastin, which regulates LD formation as illustrated above, affects synaptic functions (Trotta et al., 2004; De Gregorio et al., 2017). Whether this is related to the change in ER morphology due to loss of spastin or atlastin, or to the action of these proteins on LD remains to be established.
Importance of Myelin for Axon Maintenance
The most prominent lipid component of the brain is myelin that surrounds axons and promotes fast propagation of membrane depolarization. Most, if not all, forms of complex HSP are associated with white matter abnormalities detected on brain MRI. On the other hand, most, if not all, forms of leukodystrophies can present with spastic paraplegia such as Krabbe disease and metachromatic leukodystrophy. Likewise, it becomes increasingly difficult to make a distinction between HSP and leukodystrophy genes as illustrated by FA2H (Kruer et al., 2010).
Mutations in proteolipid protein gene (PLP1), GJC2, and MAG are responsible for a variety of phenotype ranging from hereditary spastic paraplegias (SPG2, SPG44, and SPG75, respectively) to neurodevelopmental disorders including Pelizaeus-Merzbacher disease, a hypomyelinating disorder of the central nervous system (Saugier-Veber et al., 1994; Orthmann-Murphy et al., 2009; Novarino et al., 2014; Lossos et al., 2015). The proteins encoded by these genes are essential for the maintenance of the myelin and axon integrity. Furthermore, loss of PLP1 in an oligodendrocyte cell line led to decreased levels of ethanolamine plasmalogen (Wood et al., 2011), highlighting the link between lipid metabolism and myelin maintenance. PLP1 encodes two proteins, PLP and DM20, that are essential constituents of myelin. An autopsic case of SPG2 showed widespread white matter pallor in the central nervous system. Demyelination was associated with loss of axons and remaining fibers contained spheroids (Suzuki et al., 2011). Similarly, Plp1 knockout mice assembled compact myelin sheaths, but subsequently showed progressive degeneration of axons (Griffiths, 1998), suggesting that maintenance of myelin is required to sustain neuronal function. This was confirmed by the specific deletion of Plp1 in oligodendroglial lineage, demonstrating that PLP is essential in oligodendrocytes to sustain axonal function and prevent axonal degeneration (Lüders et al., 2017). Loss of PLP was shown to alter fast axonal transport, leading to accumulation of membranous organelles and formation of axonal swellings (Edgar et al., 2004).
Similarly to loss of Plp, Mag knockout mice did not show impaired myelination, but when they became older than 8 months, they presented alteration of the maintenance of axon-myelin units, resulting in both axon and myelin degeneration (Fruttiger et al., 1995). MAG is expressed on the myelin membrane wrap that is directly apposed to the membrane of neurons, and it binds to receptors on axons (Schnaar and Lopez, 2009). Among the putative receptors on axon membranes, GD1a and GT1b were identified as potent MAG receptors (Yang et al., 1996). B4galnt1 knockout mice, which are deficient in a key enzyme for ganglioside extension, lack GD1a and GT1b and present progressive axon degeneration (Sheikh et al., 1999; Chiavegatto et al., 2000). Expression of B4GALNT1 specifically in neurons in the B4galnt1–/– mice prevented axon degeneration, suggesting that neuronal gangliosides are critical to maintain axon integrity (Yao et al., 2014). Interestingly, comparison of Mag, B4galnt1, and double knockout mice in the same genetic background showed similar axon degeneration, suggesting that oligodendroglial MAG binding to neuronal gangliosides contributes to maintain axon function (Pan et al., 2005).
Beside mutations affecting proteins that are myelin constituents, other mutations affect the lipid composition of myelin. Myelin is highly enriched in sphingolipids containing 2-hydroxylated fatty acids that require the FA2H enzyme for their synthesis, as illustrated in the first section of the review. The absence of FA2H in mouse did not prevent the formation of myelin sheaths. However, as mice were aging, axon and myelin sheath degeneration was observed (Zoller et al., 2008). Similar to the observations made in mice devoid of PLP or MAG, this suggests that axon degeneration is a consequence of impaired support provided by myelin. Specific deletion of FA2H in Schwann cells and oligodendrocytes showed demyelination similar to that observed in a constitutive Fa2h knockout mouse model (Potter et al., 2011). However, these mice did not show impaired cognitive function as observed in constitutive knockout, suggesting that FA2H could also have important function outside myelinating cells (Potter et al., 2011). Levels of 2-hydroxygalactosylceramide are also strongly reduced in the Aldh3a2 knockout mouse model of Sjorgren–Larsson syndrome, due to inactivation of FA2H (Kanetake et al., 2019). Consistent with the Fa2h knockout, the formation of myelin was not impaired in Aldh3a2 knockout mice (Kanetake et al., 2019).
As already discussed, X-ALD patients present progressive demyelination. In these patients and in a mouse model of the disease, the composition of myelin is altered (Brown et al., 1983; Bizzozero et al., 1991; Theda et al., 1992; Hama et al., 2018), but it is still not known whether this change in lipid composition contributes to demyelination. Patients affected by Krabbe disease or metachromatic leukodystrophy also present a strong demyelination, although the molecular mechanism underlying this phenomenon is still unclear. Loss of arylsulfatase A activity both in metachromatic leukodystrophy patients and in a mouse model of the disease leads to a strong accumulation of sulfatides, a class of lipids enriched in myelin. Yet, the mouse model of this disease did not present any gross white matter defect (Hess et al., 1996). In Krabbe disease, defective myelin formation as well as demyelination at a late disease stage have been observed in the natural Twitcher mouse model of the disease (Takahashi and Suzuki, 1984; Inamura et al., 2018). The alterations were proposed to result from impaired differentiation and survival of oligodendrocytes, due to accumulation of psychosine (Inamura et al., 2018).
Abnormal white matter has been detected on brain MRI in many HSP subtypes. Therefore, the loss of support provided by myelin to maintain axon function could contribute to the physiopathology in many forms of HSP. However, the mechanism of myelin loss in many forms of HSP is not known, and it will be important to determine whether loss of myelin is the primary defect or whether it is secondary or concomitant to axon degeneration.
Conclusion
HSP can be due to various mutations in genes encoding proteins involved in lipid metabolism, affecting most classes of lipids (sterols, fatty acids, phospholipids, and sphingolipids). This review of the literature highlights that alteration of both synthesis and degradation of the various classes of lipids can lead to HSP or HSP-related disorders, highlighting the importance of lipid homeostasis for the brain physiology. Based on current knowledge, the products of some genes can be connected within metabolic pathways, but many gaps exist between these products. Since the genetic cause is still unknown in about 50% of HSP patients, the identification of new causative genes may help to fill this gap. However, the identification of genes responsible for diseases has become challenging, as the main genes have likely been identified. Combination of genomic with lipidomic analysis could help us to uncover new genes and complete the map of genes responsible for HSP that are implicated in lipid metabolism.
As illustrated here, various steps of the metabolism of lipid molecules can be affected, leading to alteration in various subcellular compartments. Alteration of mitochondrial functions, oxidative stress or impairment of axonal transport can occur in some forms of HSP associated with alteration of lipid metabolism. Yet, the link between alteration of lipid metabolism and neurodegeneration is still unclear in most forms of HSP. Indeed, many studies have been performed in cell lines or cells derived from patients to demonstrate which lipid class was primarily affected. As shown by the investigation of myelin–axon interaction, studies modulating lipid metabolism in animal models, or at least cultured neuronal models, are now required to evaluate the role of lipids in neurodegeneration in HSP. This kind of study will be critical to develop therapeutic strategies. Indeed, metabolic processes, including lipid metabolism, are in theory druggable and understanding the role of lipids in neurodegeneration could help to identify therapeutic targets.
Author Contributions
All authors listed have made a substantial, direct and intellectual contribution to the work, and approved it for publication.
Funding
This work was supported by the “Investissements d’avenir” program grants (ANR-10-IAIHU-06) and (ANR-11-INBS-0011) and received funding from the European Union’s Horizon 2020 Research and Innovation Programme under grant agreement No. 779257-SOLVE-RD (to GS), the GIS-Maladies Rares Foundation (to GS), the E-Rare program (Neurolipid and Prepare consortia, to GS), and the European Research Council (European Research Council Starting [grant No. 311149] to FD).
Conflict of Interest
The authors declare that the research was conducted in the absence of any commercial or financial relationships that could be construed as a potential conflict of interest.
Acknowledgments
The authors thank B. Gurchenkov for his help with illustrations.
References
Aharon-Peretz, J., Rosenbaum, H., and Gershoni-Baruch, R. (2004). Mutations in the glucocerebrosidase gene and Parkinson’s disease in ashkenazi jews. N. Engl. J. Med. 351, 1972–1977. doi: 10.1056/NEJMoa033277
Ahmed, M. Y., Al-Khayat, A., Al-Murshedi, F., Al-Futaisi, A., Chioza, B. A., Pedro Fernandez-Murray, J., et al. (2017). A mutation of EPT1 (SELENOI) underlies a new disorder of Kennedy pathway phospholipid biosynthesis. Brain 140, 547–554. doi: 10.1093/brain/aww318
Alazami, A. M., Adly, N., Al Dhalaan, H., and Alkuraya, F. S. (2011). A nullimorphic ERLIN2 mutation defines a complicated hereditary spastic paraplegia locus (SPG18) Neurogenetics 12, 333–336. doi: 10.1007/s10048-011-02918
Aldahmesh, M. A., Mohamed, J. Y., Alkuraya, H. S., Verma, I. C., Puri, R. D., Alaiya, A. A., et al. (2011). Recessive mutations in ELOVL4 cause ichthyosis, intellectual disability, and spastic quadriplegia. Am. J. Hum. Genet. 89, 745–750. doi: 10.1016/j.ajhg.2011.10.011
Alderson, N. L., Rembiesa, B. M., Walla, M. D., Bielawska, A., Bielawski, J., and Hama, H. (2004). The human FA2H gene encodes a fatty acid 2-hydroxylase. J. Biol. Chem. 279, 48562–48568. doi: 10.1074/jbc.M406649200
Allison, R., Edgar, J. R., Pearson, G., Rizo, T., Newton, T., Günther, S., et al. (2017). Defects in ER–endosome contacts impact lysosome function in hereditary spastic paraplegia. J. Cell Biol. 216, 1337–1355. doi: 10.1083/jcb.201609033
Amador, M. D. M., Masingue, M., Debs, R., Lamari, F., Perlbarg, V., Roze, E., et al. (2018). Treatment with chenodeoxycholic acid in cerebrotendinous xanthomatosis: clinical, neurophysiological, and quantitative brain structural outcomes. J. Inherit. Metab. Dis. 41, 799–807. doi: 10.1007/s10545-018-01627
Anheim, M., Lagier-Tourenne, C., Stevanin, G., Fleury, M., Durr, A., Namer, I. J., et al. (2009). SPG11 spastic paraplegia. A new cause of juvenile parkinsonism. J. Neurol. 256, 104–108. doi: 10.1007/s00415-009-0083-3
Anheuser, S., Breiden, B., Schwarzmann, G., and Sandhoff, K. (2015). Membrane lipids regulate ganglioside GM2 catabolism and GM2 activator protein activity. J. Lipid Res. 56, 1747–1761. doi: 10.1194/jlr.M061036
Ashe, K. M., Bangari, D., Li, L., Cabrera-Salazar, M. A., Bercury, S. D., Nietupski, J. B., et al. (2011). Iminosugar-based inhibitors of glucosylceramide synthase increase brain glycosphingolipids and survival in a mouse model of sandhoff disease. PLoS ONE 6:e21758. doi: 10.1371/journal.pone.0021758
Baba, T., Kashiwagi, Y., Arimitsu, N., Kogure, T., Edo, A., Maruyama, T., et al. (2014). Phosphatidic acid (PA)-preferring phospholipase A1 regulates mitochondrial dynamics. J. Biol. Chem. 289, 11497–11511. doi: 10.1074/jbc.M113.531921
Beck, G., Sugiura, Y., Shinzawa, K., Kato, S., Setou, M., Tsujimoto, Y., et al. (2011). Neuroaxonal dystrophy in calcium-independent phospholipase A2 deficiency results from insufficient remodeling and degeneration of mitochondrial and presynaptic membranes. J. Neurosci. 31, 11411–11420. doi: 10.1523/JNEUROSCI.0345-11.2011
Benador, I. Y., Veliova, M., Liesa, M., and Shirihai, O. S. (2019). Mitochondria bound to lipid droplets: where mitochondrial dynamics regulate lipid storage and utilization. Cell Metab. 29, 827–835. doi: 10.1016/j.cmet.2019.02.011
Berginer, V. M., Salen, G., and Shefer, S. (1984). Long-term treatment of cerebrotendinous xanthomatosis with chenodeoxycholic acid. N. Engl. J. Med. 311, 1649–1652. doi: 10.1056/NEJM198412273112601
Bizzozero, O. A., Zuñiga, G., and Lees, M. B. (1991). Fatty acid composition of human myelin proteolipid protein in peroxisomal disorders. J. Neurochem. 56, 872–878. doi: 10.1111/j.1471-4159.1991.tb02003.x
Boukhris, A., Schule, R., Loureiro, J. L., Lourenço, C. M., Mundwiller, E., Gonzalez, M. A., et al. (2013). Alteration of ganglioside biosynthesis responsible for complex hereditary spastic paraplegia. Am. J. Hum. Genet. 93, 118–123. doi: 10.1016/j.ajhg.2013.05.006
Boutry, M., Branchu, J., Lustremant, C., Pujol, C., Pernelle, J., Matusiak, R., et al. (2018). Inhibition of lysosome membrane recycling causes accumulation of gangliosides that contribute to neurodegeneration. Cell Rep. 23, 3813–3826. doi: 10.1016/j.celrep.2018.05.098
Boutry, M., Morais, S., and Stevanin, G. (2019a). Update on the genetics of spastic paraplegias. Curr. Neurol. Neurosci. Rep. 19:18. doi: 10.1007/s11910-019-09302
Boutry, M., Pierga, A., Matusiak, R., Branchu, J., Houllegatte, M., Ibrahim, Y., et al. (2019b). Loss of spatacsin impairs cholesterol trafficking and calcium homeostasis. Commun. Biol. 2:380. doi: 10.1038/s42003-019-0615-z
Bowen, D. M., and Radin, N. S. (1968). Hydroxy fatty acid metabolism in brain. Adv. Lipid Res. 6, 255–272. doi: 10.1016/b978-1-4831-9942-9.50013-9
Branchu, J., Boutry, M., Sourd, L., Depp, M., Leone, C., Corriger, A., et al. (2017). Loss of spatacsin function alters lysosomal lipid clearance leading to upper and lower motor neuron degeneration. Neurobiol. Dis. 102, 21–37. doi: 10.1016/j.nbd.2017.02.007
Bras, J., Verloes, A., Schneider, S. A., Mole, S. E., and Guerreiro, R. J. (2012). Mutation of the parkinsonism gene ATP13A2 causes neuronal ceroid-lipofuscinosis. Hum. Mol. Genet. 21, 2646–2650. doi: 10.1093/hmg/dds089
Braverman, N. E., and Moser, A. B. (2012). Functions of plasmalogen lipids in health and disease. Biochim. Biophys. Acta BBA – Mol. Basis Dis. 1822, 1442–1452. doi: 10.1016/j.bbadis.2012.05.008
Browman, D. T. (2006). Erlin-1 and erlin-2 are novel members of the prohibitin family of proteins that define lipid-raft-like domains of the ER. J. Cell Sci. 119, 3149–3160. doi: 10.1242/jcs.03060
Brown, F. R., Chen, W. W., Kirschner, D. A., Frayer, K. L., Powers, J. M., Moser, A. B., et al. (1983). Myelin membrane from adrenoleukodystrophy brain white matter? Biochemical properties. J. Neurochem. 41, 341–348. doi: 10.1111/j.1471-4159.1983.tb04748.x
Cameron, D. J., Tong, Z., Yang, Z., Kaminoh, J., Kamiyah, S., Chen, H., et al. (2007). Essential role of Elovl4 in very long chain fatty acid synthesis, skin permeability barrier function, and neonatal survival. Int. J. Biol. Sci. 3, 111–119. doi: 10.7150/ijbs.3.111
Carrasco, P., Sahún, I., McDonald, J., Ramírez, S., Jacas, J., Gratacós, E., et al. (2012). Ceramide levels regulated by carnitine palmitoyltransferase 1c control dendritic spine maturation and cognition. J. Biol. Chem. 287, 21224–21232. doi: 10.1074/jbc.M111.337493
Casals, N., Zammit, V., Herrero, L., Fadó, R., Rodríguez-Rodríguez, R., and Serra, D. (2016). Carnitine palmitoyltransferase 1C: from cognition to cancer. Prog. Lipid Res. 61, 134–148. doi: 10.1016/j.plipres.2015.11.004
Chang, C.-L., Weigel, A. V., Ioannou, M. S., Pasolli, H. A., Xu, C. S., Peale, D. R., et al. (2019). Spastin tethers lipid droplets to peroxisomes and directs fatty acid trafficking through ESCRT-III. J. Cell Biol. 218, 2583–2599. doi: 10.1083/jcb.201902061
Chang, J., Lee, S., and Blackstone, C. (2014). Spastic paraplegia proteins spastizin and spatacsin mediate autophagic lysosome reformation. J. Clin. Invest. 124, 5249–5262. doi: 10.1172/JCI77598
Chen, W.-J. (2019). PCSK9 Inhibitor Treatment for Patients With Hereditary Spastic Paraplegia Type 5. Available at: https://clinicaltrials.gov/ct2/show/NCT04101643.
Chiavegatto, S., Sun, J., Nelson, R. J., and Schnaar, R. L. (2000). A functional role for complex gangliosides: motor deficits in GM2/GD2 synthase knockout mice. Exp. Neurol. 166, 227–234. doi: 10.1006/exnr.2000.7504
Chuang, S. S., Helvig, C., Taimi, M., Ramshaw, H. A., Collop, A. H., Amad, M., et al. (2004). CYP2U1, a novel human thymus- and brain-specific cytochrome P450, Catalyzes ω- and (ω-1)-Hydroxylation of Fatty Acids. J. Biol. Chem. 279, 6305–6314. doi: 10.1074/jbc.M311830200
Dard, R., Meyniel, C., Touitou, V., Stevanin, G., Lamari, F., Durr, A., et al. (2017). Mutations in DDHD1, encoding a phospholipase A1, is a novel cause of retinopathy and neurodegeneration with brain iron accumulation. Eur. J. Med. Genet. 60, 639–642. doi: 10.1016/j.ejmg.2017.08.015
Darios, F., Connell, E., and Davletov, B. (2007). Phospholipases and fatty acid signalling in exocytosis: phospholipases and fatty acid signalling in exocytosis. J. Physiol. 585, 699–704. doi: 10.1113/jphysiol.2007.136812
De Gregorio, C., Delgado, R., Ibacache, A., Sierralta, J., and Couve, A. (2017). Drosophila Atlastin in motor neurons is required for locomotion and presynaptic function. J. Cell Sci. 130, 3507–3516. doi: 10.1242/jcs.201657
Dehay, B., Ramirez, A., Martinez-Vicente, M., Perier, C., Canron, M.-H., Doudnikoff, E., et al. (2012). Loss of P-type ATPase ATP13A2/PARK9 function induces general lysosomal deficiency and leads to Parkinson disease neurodegeneration. Proc. Natl. Acad. Sci. U.S.A. 109, 9611–9616. doi: 10.1073/pnas.1112368109
DeLuca, G. C., Ebers, G. C., and Esiri, M. M. (2004). The extent of axonal loss in the long tracts in hereditary spastic paraplegia. Neuropathol. Appl. Neurobiol. 30, 576–584. doi: 10.1111/j.1365-2990.2004.00587.x
Dietschy, J. M., and Turley, S. D. (2004). Thematic review series: brain lipids. Cholesterol metabolism in the central nervous system during early development and in the mature animal. J. Lipid Res. 45, 1375–1397. doi: 10.1194/jlr.R400004-JLR200
Dodge, J. C., Treleaven, C. M., Pacheco, J., Cooper, S., Bao, C., Abraham, M., et al. (2015). Glycosphingolipids are modulators of disease pathogenesis in amyotrophic lateral sclerosis. Proc. Natl. Acad. Sci. U.S.A. 112, 8100–8105. doi: 10.1073/pnas.1508767112
Doi, H., Ushiyama, M., Baba, T., Tani, K., Shiina, M., Ogata, K., et al. (2015). Late-onset spastic ataxia phenotype in a patient with a homozygous DDHD2 mutation. Sci. Rep. 4:7132. doi: 10.1038/srep07132
Duell, P. B., Salen, G., Eichler, F. S., DeBarber, A. E., Connor, S. L., Casaday, L., et al. (2018). Diagnosis, treatment, and clinical outcomes in 43 cases with cerebrotendinous xanthomatosis. J. Clin. Lipidol. 12, 1169–1178. doi: 10.1016/j.jacl.2018.06.008
Eastman, S. W., Yassaee, M., and Bieniasz, P. D. (2009). A role for ubiquitin ligases and Spartin/SPG20 in lipid droplet turnover. J. Cell Biol. 184, 881–894. doi: 10.1083/jcb.200808041
Edgar, J. M., McLaughlin, M., Yool, D., Zhang, S.-C., Fowler, J. H., Montague, P., et al. (2004). Oligodendroglial modulation of fast axonal transport in a mouse model of hereditary spastic paraplegia. J. Cell Biol. 166, 121–131. doi: 10.1083/jcb.200312012
Edvardson, S., Hama, H., Shaag, A., Gomori, J. M., Berger, I., Soffer, D., et al. (2008). Mutations in the fatty acid 2-hydroxylase gene are associated with leukodystrophy with spastic paraparesis and dystonia. Am. J. Hum. Genet. 83, 643–648. doi: 10.1016/j.ajhg.2008.10.010
Edwards, T. L., Clowes, V. E., Tsang, H. T. H., Connell, J. W., Sanderson, C. M., Luzio, J. P., et al. (2009). Endogenous spartin (SPG20) is recruited to endosomes and lipid droplets and interacts with the ubiquitin E3 ligases AIP4 and AIP5. Biochem. J. 423, 31–39. doi: 10.1042/BJ20082398
Eichler, F., Duncan, C., Musolino, P. L., Orchard, P. J., De Oliveira, S., Thrasher, A. J., et al. (2017). Hematopoietic stem-cell gene therapy for cerebral adrenoleukodystrophy. N. Engl. J. Med. 377, 1630–1638. doi: 10.1056/NEJMoa1700554
Eichler, F. S., Ren, J.-Q., Cossoy, M., Rietsch, A. M., Nagpal, S., Moser, A. B., et al. (2008). Is microglial apoptosis an early pathogenic change in cerebral X-linked adrenoleukodystrophy? Ann. Neurol. 63, 729–742. doi: 10.1002/ana.21391
Engelen, M., Barbier, M., Dijkstra, I. M. E., Schür, R., de Bie, R. M. A., Verhamme, C., et al. (2014). X-linked adrenoleukodystrophy in women: a cross-sectional cohort study. Brain 137, 693–706. doi: 10.1093/brain/awt361
Estrada-Cuzcano, A., Martin, S., Chamova, T., Synofzik, M., Timmann, D., Holemans, T., et al. (2017). Loss-of-function mutations in the ATP13A2/PARK9 gene cause complicated hereditary spastic paraplegia (SPG78). Brain 140, 287–305. doi: 10.1093/brain/aww307
Fruttiger, M., Montag, D., Schachner, M., and Martini, R. (1995). Crucial role for the myelin-associated glycoprotein in the maintenance of axon-myelin integrity. Eur. J. Neurosci. 7, 511–515. doi: 10.1111/j.1460-9568.1995.tb00347.x
Fujita, M., Watanabe, R., Jaensch, N., Romanova-Michaelides, M., Satoh, T., Kato, M., et al. (2011). Sorting of GPI-anchored proteins into ER exit sites by p24 proteins is dependent on remodeled GPI. J. Cell Biol. 194, 61–75. doi: 10.1083/jcb.201012074
Goizet, C., Boukhris, A., Durr, A., Beetz, C., Truchetto, J., Tesson, C., et al. (2009). CYP7B1 mutations in pure and complex forms of hereditary spastic paraplegia type 5. Brain 132, 1589–1600. doi: 10.1093/brain/awp073
Gomez-Ospina, N. (2006). “Arylsulfatase a deficiency,” in GeneReviews®, eds M. P. Adam, H. H. Ardinger, R. A. Pagon, S. E. Wallace, L. J. Bean, K. Stephens, et al. (Seattle, WA: University of Washington, Seattle).
Green, J. T., Orr, S. K., and Bazinet, R. P. (2008). The emerging role of group VI calcium-independent phospholipase A2 in releasing docosahexaenoic acid from brain phospholipids: Fig. 1. J. Lipid Res. 49, 939–944. doi: 10.1194/jlr.R700017-JLR200
Griffiths, I. (1998). Axonal swellings and degeneration in mice lacking the major proteolipid of myelin. Science 280, 1610–1613. doi: 10.1126/science.280.5369.1610
Hama, K., Fujiwara, Y., Morita, M., Yamazaki, F., Nakashima, Y., Takei, S., et al. (2018). Profiling and imaging of phospholipids in brains of Abcd1-deficient mice. Lipids 53, 85–102. doi: 10.1002/lipd.12022
Hammer, M. B., Eleuch-Fayache, G., Schottlaender, L. V., Nehdi, H., Gibbs, J. R., Arepalli, S. K., et al. (2013). Mutations in GBA2 cause autosomal-recessive cerebellar ataxia with spasticity. Am. J. Hum. Genet. 92, 245–251. doi: 10.1016/j.ajhg.2012.12.012
Hanein, S., Martin, E., Boukhris, A., Byrne, P., Goizet, C., Hamri, A., et al. (2008). Identification of the SPG15 gene, encoding spastizin, as a frequent cause of complicated autosomal-recessive spastic paraplegia, including kjellin syndrome. Am. J. Hum. Genet. 82, 992–1002. doi: 10.1016/j.ajhg.2008.03.004
Harding, A. E. (1983). Classification of the hereditary ataxias and paraplegias. Lancet Lond. Engl. 1, 1151–1155. doi: 10.1016/s0140-6736(83)928799
Harlalka, G. V., Lehman, A., Chioza, B., Baple, E. L., Maroofian, R., Cross, H., et al. (2013). Mutations in B4GALNT1 (GM2 synthase) underlie a new disorder of ganglioside biosynthesis. Brain 136, 3618–3624. doi: 10.1093/brain/awt270
Hehr, U., Bauer, P., Winner, B., Schule, R., Olmez, A., Koehler, W., et al. (2007). Long-term course and mutational spectrum of spatacsin-linked spastic paraplegia. Ann. Neurol. 62, 656–665. doi: 10.1002/ana.21310
Hein, S., Schonfeld, P., Kahlert, S., and Reiser, G. (2008). Toxic effects of X-linked adrenoleukodystrophy-associated, very long chain fatty acids on glial cells and neurons from rat hippocampus in culture. Hum. Mol. Genet. 17, 1750–1761. doi: 10.1093/hmg/ddn066
Hess, B., Saftig, P., Hartmann, D., Coenen, R., Lullmann-Rauch, R., Goebel, H. H., et al. (1996). Phenotype of arylsulfatase A-deficient mice: relationship to human metachromatic leukodystrophy. Proc. Natl. Acad. Sci. U.S.A. 93, 14821–14826. doi: 10.1073/pnas.93.25.14821
Higgs, H. N., Han, M. H., Johnson, G. E., and Glomset, J. A. (1998). Cloning of a phosphatidic acid-preferring phospholipase A1 from bovine testis. J. Biol. Chem. 273, 5468–5477. doi: 10.1074/jbc.273.10.5468
Hirst, J., Edgar, J. R., Esteves, T., Darios, F., Madeo, M., Chang, J., et al. (2015). Loss of AP-5 results in accumulation of aberrant endolysosomes: defining a new type of lysosomal storage disease. Hum. Mol. Genet. 24, 4984–4996. doi: 10.1093/hmg/ddv220
Hirst, J., Madeo, M., Smets, K., Edgar, J. R., Schols, L., Li, J., et al. (2016). Complicated spastic paraplegia in patients with AP5Z1 mutations (SPG48). Neurol. Genet. 2:e98. doi: 10.1212/NXG.0000000000000098
Hooper, C., Puttamadappa, S. S., Loring, Z., Shekhtman, A., and Bakowska, J. C. (2010). Spartin activates atrophin-1-interacting protein 4 (AIP4) E3 ubiquitin ligase and promotes ubiquitination of adipophilin on lipid droplets. BMC Biol. 8:72. doi: 10.1186/1741-7007-872
Horibata, Y., Elpeleg, O., Eran, A., Hirabayashi, Y., Savitzki, D., Tal, G., et al. (2018). EPT1 (selenoprotein I) is critical for the neural development and maintenance of plasmalogen in humans. J. Lipid Res. 59, 1015–1026. doi: 10.1194/jlr.P081620
Huber, M. D., Vesely, P. W., Datta, K., and Gerace, L. (2013). Erlins restrict SREBP activation in the ER and regulate cellular cholesterol homeostasis. J. Cell Biol. 203, 427–436. doi: 10.1083/jcb.201305076
Huffnagel, I. C., Dijkgraaf, M. G. W., Janssens, G. E., van Weeghel, M., van Geel, B. M., Poll-The, B. T., et al. (2019). Disease progression in women with X-linked adrenoleukodystrophy is slow. Orphanet J. Rare Dis. 14:30. doi: 10.1186/s13023-019-10086
Igisu, H., Shimomura, K., Kishimoto, Y., and Suzuki, K. (1983). Lipids of developing brain of twitcher mouse. An authentic murine model of human Krabbe disease. Brain J. Neurol. 106(Pt 2), 405–417. doi: 10.1093/brain/106.2.405
Igisu, H., and Suzuki, K. (1984). Progressive accumulation of toxic metabolite in a genetic leukodystrophy. Science 224, 753–755. doi: 10.1126/science.6719111
Inamura, N., Kito, M., Go, S., Kishi, S., Hosokawa, M., Asai, K., et al. (2018). Developmental defects and aberrant accumulation of endogenous psychosine in oligodendrocytes in a murine model of Krabbe disease. Neurobiol. Dis. 120, 51–62. doi: 10.1016/j.nbd.2018.08.023
Inloes, J. M., Hsu, K.-L., Dix, M. M., Viader, A., Masuda, K., Takei, T., et al. (2014). The hereditary spastic paraplegia-related enzyme DDHD2 is a principal brain triglyceride lipase. Proc. Natl. Acad. Sci. U.S.A. 111, 14924–14929. doi: 10.1073/pnas.1413706111
Inloes, J. M., Jing, H., and Cravatt, B. F. (2018). The spastic paraplegia-associated phospholipase DDHD1 is a primary brain phosphatidylinositol lipase. Biochemistry 57, 5759–5767. doi: 10.1021/acs.biochem.8b00810
Inoue, H., Baba, T., Sato, S., Ohtsuki, R., Takemori, A., Watanabe, T., et al. (2012). Roles of SAM and DDHD domains in mammalian intracellular phospholipase A1 KIAA0725p. Biochim. Biophys. Acta BBA – Mol. Cell Res. 1823, 930–939. doi: 10.1016/j.bbamcr.2012.02.002
Inoue, K., Kubota, S., and Seyama, Y. (1999). Cholestanol induces apoptosis of cerebellar neuronal cells. Biochem. Biophys. Res. Commun. 256, 198–203. doi: 10.1006/bbrc.1998.9497
Jakobsson, A., Westerberg, R., and Jacobsson, A. (2006). Fatty acid elongases in mammals: their regulation and roles in metabolism. Prog. Lipid Res. 45, 237–249. doi: 10.1016/j.plipres.2006.01.004
James, P. F., and Zoeller, R. A. (1997). Isolation of animal cell mutants defective in long-chain fatty aldehyde dehydrogenase: sensitivity to fatty aldehydes and schiff’s base modification of phospholipids: implications for sjögren-larsson syndrome. J. Biol. Chem. 272, 23532–23539. doi: 10.1074/jbc.272.38.23532
Joshi, D. C., and Bakowska, J. C. (2011). SPG20 protein spartin associates with cardiolipin via its plant-related senescence domain and regulates mitochondrial Ca2+ homeostasis. PLoS ONE 6:e19290. doi: 10.1371/journal.pone.0019290
Kameoka, S., Adachi, Y., Okamoto, K., Iijima, M., and Sesaki, H. (2018). Phosphatidic acid and cardiolipin coordinate mitochondrial dynamics. Trends Cell Biol. 28, 67–76. doi: 10.1016/j.tcb.2017.08.011
Kanamori, A., Nakayama, J., Fukuda, M. N., Stallcup, W. B., Sasaki, K., Fukuda, M., et al. (1997). Expression cloning and characterization of a cDNA encoding a novel membrane protein required for the formation of O-acetylated ganglioside: a putative acetyl-CoA transporter. Proc. Natl. Acad. Sci. U.S.A. 94, 2897–2902. doi: 10.1073/pnas.94.7.2897
Kanetake, T., Sassa, T., Nojiri, K., Sawai, M., Hattori, S., Miyakawa, T., et al. (2019). Neural symptoms in a gene knockout mouse model of Sjögren-Larsson syndrome are associated with a decrease in 2-hydroxygalactosylceramide. FASEB J. 33, 928–941. doi: 10.1096/fj.201800291R
Kemp, S., Berger, J., and Aubourg, P. (2012). X-linked adrenoleukodystrophy: clinical, metabolic, genetic and pathophysiological aspects. Biochim. Biophys. Acta BBA – Mol. Basis Dis. 1822, 1465–1474. doi: 10.1016/j.bbadis.2012.03.012
Khundadze, M., Kollmann, K., Koch, N., Biskup, C., Nietzsche, S., Zimmer, G., et al. (2013). A hereditary spastic paraplegia mouse model supports a role of ZFYVE26/SPASTIZIN for the endolysosomal system. PLoS Genet. 9:e1003988. doi: 10.1371/journal.pgen.1003988
Khundadze, M., Ribaudo, F., Hussain, A., Rosentreter, J., Nietzsche, S., Thelen, M., et al. (2019). A mouse model for SPG48 reveals a block of autophagic flux upon disruption of adaptor protein complex five. Neurobiol. Dis. 127, 419–431. doi: 10.1016/j.nbd.2019.03.026
Klemm, R. W., Norton, J. P., Cole, R. A., Li, C. S., Park, S. H., Crane, M. M., et al. (2013). A conserved role for atlastin GTPases in regulating lipid droplet size. Cell Rep. 3, 1465–1475. doi: 10.1016/j.celrep.2013.04.015
Kobayashi, T., Shinnoh, N., Goto, I., and Kuroiwa, Y. (1985). Hydrolysis of galactosylceramide is catalyzed by two genetically distinct acid beta-galactosidases. J. Biol. Chem. 260, 14982–14987.
Kruer, M. C., Paisán-Ruiz, C., Boddaert, N., Yoon, M. Y., Hama, H., Gregory, A., et al. (2010). Defective FA2H leads to a novel form of neurodegeneration with brain iron accumulation (NBIA). Ann. Neurol. 68, 611–618. doi: 10.1002/ana.22122
Kruska, N., Schönfeld, P., Pujol, A., and Reiser, G. (2015). Astrocytes and mitochondria from adrenoleukodystrophy protein (ABCD1)-deficient mice reveal that the adrenoleukodystrophy-associated very long-chain fatty acids target several cellular energy-dependent functions. Biochim. Biophys. Acta BBA – Mol. Basis Dis. 1852, 925–936. doi: 10.1016/j.bbadis.2015.01.005
Kutkowska-Kaźmierczak, A., Rydzanicz, M., Chlebowski, A., Kłosowska-Kosicka, K., Mika, A., Gruchota, J., et al. (2018). Dominant ELOVL1 mutation causes neurological disorder with ichthyotic keratoderma, spasticity, hypomyelination and dysmorphic features. J. Med. Genet. 55, 408–414. doi: 10.1136/jmedgenet-2017-105172
Laule, C., Vavasour, I. M., Shahinfard, E., Mädler, B., Zhang, J., Li, D. K. B., et al. (2018). Hematopoietic stem cell transplantation in late-onset krabbe disease: no evidence of worsening demyelination and axonal loss 4 years post-allograft. J. Neuroimaging 28, 252–255. doi: 10.1111/jon.12502
Laurenzi, V. D., Rogers, G. R., Hamrock, D. J., Marekov, L. N., Steinert, P. M., Compton, J. G., et al. (1996). Sjögren–Larsson syndrome is caused by mutations in the fatty aldehyde dehydrogenase gene. Nat. Genet. 12, 52–57. doi: 10.1038/ng0196-52
Li, W., Sandhoff, R., Kono, M., Zerfas, P., Hoffmann, V., Ding, B. C.-H., et al. (2007). Depletion of ceramides with very long chain fatty acids causes defective skin permeability barrier function, and neonatal lethality in ELOVL4 deficient mice. Int. J. Biol. Sci. 3, 120–128. doi: 10.7150/ijbs.3.120
Lin, P., Li, J., Liu, Q., Mao, F., Li, J., Qiu, R., et al. (2008). A missense mutation in slc33a1, which encodes the Acetyl-CoA transporter, causes autosomal-dominant spastic paraplegia (SPG42). Am. J. Hum. Genet. 83, 752–759. doi: 10.1016/j.ajhg.2008.11.003
Liu, P., Jiang, B., Ma, J., Lin, P., Zhang, Y., Shao, C., et al. (2017). S113R mutation in SLC33A1 leads to neurodegeneration and augmented BMP signaling in a mouse model. Dis. Model. Mech. 10, 53–62. doi: 10.1242/dmm.026880
Lossos, A., Elazar, N., Lerer, I., Schueler-Furman, O., Fellig, Y., Glick, B., et al. (2015). Myelin-associated glycoprotein gene mutation causes Pelizaeus-Merzbacher disease-like disorder. Brain 138, 2521–2536. doi: 10.1093/brain/awv204
Lüders, K. A., Patzig, J., Simons, M., Nave, K.-A., and Werner, H. B. (2017). Genetic dissection of oligodendroglial and neuronal Plp1 function in a novel mouse model of spastic paraplegia type 2. Glia 65, 1762–1776. doi: 10.1002/glia.23193
Ma, J., Wang, L., Yang, Y.-M., Mao, C.-H., and Wan, X.-H. (2018). Novel SERAC1 mutations in a Chinese patient presenting with parkinsonism and dystonia. Neurol. Sci. 39, 967–969. doi: 10.1007/s10072-018-3247-z
Marelli, C., Lamari, F., Rainteau, D., Lafourcade, A., Banneau, G., Humbert, L., et al. (2018). Plasma oxysterols: biomarkers for diagnosis and treatment in spastic paraplegia type 5. Brain 141, 72–84. doi: 10.1093/brain/awx297
Martin, E., Schüle, R., Smets, K., Rastetter, A., Boukhris, A., Loureiro, J. L., et al. (2013). Loss of function of glucocerebrosidase GBA2 is responsible for motor neuron defects in hereditary spastic paraplegia. Am. J. Hum. Genet. 92, 238–244. doi: 10.1016/j.ajhg.2012.11.021
Maruyama, T., Baba, T., Maemoto, Y., Hara-Miyauchi, C., Hasegawa-Ogawa, M., Okano, H. J., et al. (2018). Loss of DDHD2, whose mutation causes spastic paraplegia, promotes reactive oxygen species generation and apoptosis. Cell Death Dis. 9:797. doi: 10.1038/s41419-018-0815-3
Mehl, E., and Jatzkewitz, H. (1968). Cerebroside 3-sulfate as a physiological substrate of arylsulfatase A. Biochim. Biophys. Acta BBA – Enzymol. 151, 619–627. doi: 10.1016/0005-2744(68)90008-9
Mignarri, A., Magni, A., Del Puppo, M., Gallus, G. N., Björkhem, I., Federico, A., et al. (2016). Evaluation of cholesterol metabolism in cerebrotendinous xanthomatosis. J. Inherit. Metab. Dis. 39, 75–83. doi: 10.1007/s10545-015-98731
Mileykovskaya, E., and Dowhan, W. (2014). Cardiolipin-dependent formation of mitochondrial respiratory supercomplexes. Chem. Phys. Lipids 179, 42–48. doi: 10.1016/j.chemphyslip.2013.10.012
Mishra, H. K., Prots, I., Havlicek, S., Kohl, Z., Perez-Branguli, F., Boerstler, T., et al. (2016). GSK3ß-dependent dysregulation of neurodevelopment in SPG11-patient induced pluripotent stem cell model: neurodevelopmental defects in motor neuron disease. Ann. Neurol. 79, 826–840. doi: 10.1002/ana.24633
Mitomo, H., Chen, W.-H., and Regen, S. L. (2009). Oxysterol-induced rearrangement of the liquid-ordered phase: a possible link to Alzheimer’s disease? J. Am. Chem. Soc. 131, 12354–12357. doi: 10.1021/ja904308y
Mochel, F. (2018). Lipids and synaptic functions. J. Inherit. Metab. Dis. 41, 1117–1122. doi: 10.1007/s10545-018-02041
Montecchiani, C., Pedace, L., Lo Giudice, T., Casella, A., Mearini, M., Gaudiello, F., et al. (2016). ALS5/SPG11/KIAA1840 mutations cause autosomal recessive axonal Charcot–Marie–Tooth disease. Brain 139, 73–85. doi: 10.1093/brain/awv320
Mosser, J., Douar, A.-M., Sarde, C.-O., Kioschis, P., Feil, R., Moser, H., et al. (1993). Putative X-linked adrenoleukodystrophy gene shares unexpected homology with ABC transporters. Nature 361, 726–730. doi: 10.1038/361726a0
Mueller, N., Sassa, T., Morales-Gonzalez, S., Schneider, J., Salchow, D. J., Seelow, D., et al. (2019). De novo mutation in ELOVL1 causes ichthyosis, acanthosis nigricans, hypomyelination, spastic paraplegia, high frequency deafness and optic atrophy. J. Med. Genet. 56, 164–175. doi: 10.1136/jmedgenet-2018-105711
Murakami, Y., Tawamie, H., Maeda, Y., Büttner, C., Buchert, R., Radwan, F., et al. (2014). Null mutation in PGAP1 impairing gpi-anchor maturation in patients with intellectual disability and encephalopathy. PLoS Genet. 10:e1004320. doi: 10.1371/journal.pgen.1004320
Nie, S., Chen, G., Cao, X., and Zhang, Y. (2014). Cerebrotendinous xanthomatosis: a comprehensive review of pathogenesis, clinical manifestations, diagnosis, and management. Orphanet J. Rare Dis. 9:179. doi: 10.1186/s13023-014-01794
Novarino, G., Fenstermaker, A. G., Zaki, M. S., Hofree, M., Silhavy, J. L., Heiberg, A. D., et al. (2014). Exome sequencing links corticospinal motor neuron disease to common neurodegenerative disorders. Science 343, 506–511. doi: 10.1126/science.1247363
Ofman, R., Dijkstra, I. M. E., van Roermund, C. W. T., Burger, N., Turkenburg, M., van Cruchten, A., et al. (2010). The role of ELOVL1 in very long-chain fatty acid homeostasis and X-linked adrenoleukodystrophy. EMBO Mol. Med. 2, 90–97. doi: 10.1002/emmm.201000061
Olzmann, J. A., and Carvalho, P. (2019). Dynamics and functions of lipid droplets. Nat. Rev. Mol. Cell Biol. 20, 137–155. doi: 10.1038/s41580-018-0085-z
Ong, W.-Y., Yeo, J.-F., Ling, S.-F., and Farooqui, A. A. (2005). Distribution of calcium-independent phospholipase A2 (iPLA2) in monkey brain. J. Neurocytol. 34, 447–458. doi: 10.1007/s11068-006-87304
Orlacchio, A., Babalini, C., Borreca, A., Patrono, C., Massa, R., Basaran, S., et al. (2010). SPATACSIN mutations cause autosomal recessive juvenile amyotrophic lateral sclerosis. Brain 133, 591–598. doi: 10.1093/brain/awp325
Orsini, J. J., Escolar, M. L., Wasserstein, M. P., and Caggana, M. (2000). “Krabbe disease,” in GeneReviews®, eds M. P. Adam, H. H. Ardinger, R. A. Pagon, S. E. Wallace, L. J. Bean, K. Stephens, et al. (Seattle, WA: University of Washington, Seattle).
Orthmann-Murphy, J. L., Salsano, E., Abrams, C. K., Bizzi, A., Uziel, G., Freidin, M. M., et al. (2009). Hereditary spastic paraplegia is a novel phenotype for GJA12/GJC2 mutations. Brain 132, 426–438. doi: 10.1093/brain/awn328
Ozaki, K., Doi, H., Mitsui, J., Sato, N., Iikuni, Y., Majima, T., et al. (2015). A novel mutation in ELOVL4 leading to spinocerebellar ataxia (SCA) with the hot cross bun sign but lacking erythrokeratodermia: a broadened spectrum of SCA34. JAMA Neurol. 72, 797–805. doi: 10.1001/jamaneurol.2015.0610
Pan, B., Fromholt, S. E., Hess, E. J., Crawford, T. O., Griffin, J. W., Sheikh, K. A., et al. (2005). Myelin-associated glycoprotein and complementary axonal ligands, gangliosides, mediate axon stability in the CNS and PNS: neuropathology and behavioral deficits in single- and double-null mice. Exp. Neurol. 195, 208–217. doi: 10.1016/j.expneurol.2005.04.017
Papadopoulos, C., Orso, G., Mancuso, G., Herholz, M., Gumeni, S., Tadepalle, N., et al. (2015). Spastin binds to lipid droplets and affects lipid metabolism. PLoS Genet. 11:e1005149. doi: 10.1371/journal.pgen.1005149
Park, S. H., Zhu, P.-P., Parker, R. L., and Blackstone, C. (2010). Hereditary spastic paraplegia proteins REEP1, spastin, and atlastin-1 coordinate microtubule interactions with the tubular ER network. J. Clin. Invest. 120, 1097–1110. doi: 10.1172/JCI40979
Parkinson-Lawrence, E. J., Shandala, T., Prodoehl, M., Plew, R., Borlace, G. N., and Brooks, D. A. (2010). Lysosomal storage disease: revealing lysosomal function and physiology. Physiology 25, 102–115. doi: 10.1152/physiol.00041.2009
Pearce, M. M. P., Wormer, D. B., Wilkens, S., and Wojcikiewicz, R. J. H. (2009). An endoplasmic reticulum (ER) membrane complex composed of SPFH1 and SPFH2 mediates the er-associated degradation of inositol 1,4,5-trisphosphate receptors. J. Biol. Chem. 284, 10433–10445. doi: 10.1074/jbc.M809801200
Pennetta, G., and Welte, M. A. (2018). Emerging links between lipid droplets and motor neuron diseases. Dev. Cell 45, 427–432. doi: 10.1016/j.devcel.2018.05.002
Pérez-Brangulí, F., Buchsbaum, I. Y., Pozner, T., Regensburger, M., Fan, W., Schray, A., et al. (2019). Human SPG11 cerebral organoids reveal cortical neurogenesis impairment. Hum. Mol. Genet. 28, 961–971. doi: 10.1093/hmg/ddy397
Potter, K. A., Kern, M. J., Fullbright, G., Bielawski, J., Scherer, S. S., Yum, S. W., et al. (2011). Central nervous system dysfunction in a mouse model of Fa2h deficiency. Glia 59, 1009–1021. doi: 10.1002/glia.21172
Rafi, M. A., Luzi, P., Chen, Y. Q., and Wenger, D. A. (1995). A large deletion together with a point mutation in the GALC gene is a common mutant allele in patients with infantile Krabbe disease. Hum. Mol. Genet. 4, 1285–1289. doi: 10.1093/hmg/4.8.1285
Rainier, S., Bui, M., Mark, E., Thomas, D., Tokarz, D., Ming, L., et al. (2008). Neuropathy target esterase gene mutations cause motor neuron disease. Am. J. Hum. Genet. 82, 780–785. doi: 10.1016/j.ajhg.2007.12.018
Raju, D., Schonauer, S., Hamzeh, H., Flynn, K. C., Bradke, F., vom Dorp, K., et al. (2015). Accumulation of glucosylceramide in the absence of the beta-glucosidase GBA2 alters cytoskeletal dynamics. PLoS Genet. 11:e1005063. doi: 10.1371/journal.pgen.1005063
Ramirez, A., Heimbach, A., Gründemann, J., Stiller, B., Hampshire, D., Cid, L. P., et al. (2006). Hereditary parkinsonism with dementia is caused by mutations in ATP13A2, encoding a lysosomal type 5 P-type ATPase. Nat. Genet. 38, 1184–1191. doi: 10.1038/ng1884
Rattay, T. W., Lindig, T., Baets, J., Smets, K., Deconinck, T., Söhn, A. S., et al. (2019). FAHN/SPG35: a narrow phenotypic spectrum across disease classifications. Brain 142, 1561–1572. doi: 10.1093/brain/awz102
Raymond, G. V., Aubourg, P., Paker, A., Escolar, M., Fischer, A., Blanche, S., et al. (2019). Survival and functional outcomes in boys with cerebral adrenoleukodystrophy with and without hematopoietic stem cell transplantation. Biol. Blood Marrow Transplant. 25, 538–548. doi: 10.1016/j.bbmt.2018.09.036
Read, D. J., Li, Y., Chao, M. V., Cavanagh, J. B., and Glynn, P. (2009). Neuropathy target esterase is required for adult vertebrate axon maintenance. J. Neurosci. 29, 11594–11600. doi: 10.1523/JNEUROSCI.3007-09.2009
Renvoisé, B., Chang, J., Singh, R., Yonekawa, S., FitzGibbon, E. J., Mankodi, A., et al. (2014). Lysosomal abnormalities in hereditary spastic paraplegia types SPG15 and SPG11. Ann. Clin. Transl. Neurol. 1, 379–389. doi: 10.1002/acn3.64
Renvoisé, B., Malone, B., Falgairolle, M., Munasinghe, J., Stadler, J., Sibilla, C., et al. (2016). Reep1 null mice reveal a converging role for hereditary spastic paraplegia proteins in lipid droplet regulation. Hum. Mol. Genet. 25, 5111–5125. doi: 10.1093/hmg/ddw315
Rinaldi, C., Schmidt, T., Situ, A. J., Johnson, J. O., Lee, P. R., Chen, K., et al. (2015). Mutation in CPT1C Associated With Pure Autosomal Dominant Spastic Paraplegia. JAMA Neurol. 72:561. doi: 10.1001/jamaneurol.2014.4769
Rizzo, W. B., and Craft, D. A. (1991). Sjögren-Larsson syndrome. Deficient activity of the fatty aldehyde dehydrogenase component of fatty alcohol:NAD+ oxidoreductase in cultured fibroblasts. J. Clin. Invest. 88, 1643–1648. doi: 10.1172/JCI115478
Roeben, B., Schüle, R., Ruf, S., Bender, B., Alhaddad, B., Benkert, T., et al. (2018). SERAC1 deficiency causes complicated HSP: evidence from a novel splice mutation in a large family. J. Med. Genet. 55, 39–47. doi: 10.1136/jmedgenet-2017-104622
Rydning, S. L., Dudesek, A., Rimmele, F., Funke, C., Krüger, S., Biskup, S., et al. (2018). A novel heterozygous variant in ERLIN2 causes autosomal dominant pure hereditary spastic paraplegia. Eur. J. Neurol. 25, 943–e71. doi: 10.1111/ene.13625
Salen, G., Shefer, S., and Berginer, V. (1991). Biochemical abnormalities in cerebrotendinous xanthomatosis. Dev. Neurosci. 13, 363–370. doi: 10.1159/000112186
Salen, G., and Steiner, R. D. (2017). Epidemiology, diagnosis, and treatment of cerebrotendinous xanthomatosis (CTX). J. Inherit. Metab. Dis. 40, 771–781. doi: 10.1007/s10545-017-00938
Salo, V. T., Belevich, I., Li, S., Karhinen, L., Vihinen, H., Vigouroux, C., et al. (2016). Seipin regulates ER–lipid droplet contacts and cargo delivery. EMBO J. 35, 2699–2716. doi: 10.15252/embj.201695170
Sassa, T., Ohno, Y., Suzuki, S., Nomura, T., Nishioka, C., Kashiwagi, T., et al. (2013). Impaired epidermal permeability barrier in mice lacking Elovl1, the gene responsible for very-long-chain fatty acid production. Mol. Cell. Biol. 33, 2787–2796. doi: 10.1128/mcb.00192-13
Saugier-Veber, P., Munnich, A., Bonneau, D., Rozet, J.-M., Le Merrer, M., Gil, R., et al. (1994). X–linked spastic paraplegia and Pelizaeus–Merzbacher disease are allelic disorders at the proteolipid protein locus. Nat. Genet. 6, 257–262. doi: 10.1038/ng0394-257
Schicks, J., Synofzik, M., Pétursson, H., Huttenlocher, J., Reimold, M., Schöls, L., et al. (2011). Atypical juvenile parkinsonism in a consanguineous SPG15 family: letters to the editor. Mov. Disord. 26, 565–566. doi: 10.1002/mds.23472
Schiffmann, R., FitzGibbon, E. J., Harris, C., DeVile, C., Davies, E. H., Abel, L., et al. (2008). Randomized, controlled trial of miglustat in Gaucher’s disease type 3. Ann. Neurol. 64, 514–522. doi: 10.1002/ana.21491
Schnaar, R. L., and Lopez, P. H. H. (2009). Myelin-associated glycoprotein and its axonal receptors. J. Neurosci. Res. 87, 3267–3276. doi: 10.1002/jnr.21992
Schöls, L., Rattay, T. W., Martus, P., Meisner, C., Baets, J., Fischer, I., et al. (2017). Hereditary spastic paraplegia type 5: natural history, biomarkers and a randomized controlled trial. Brain 140, 3112–3127. doi: 10.1093/brain/awx273
Schüle, R., Siddique, T., Deng, H.-X., Yang, Y., Donkervoort, S., Hansson, M., et al. (2010). Marked accumulation of 27-hydroxycholesterol in SPG5 patients with hereditary spastic paresis. J. Lipid Res. 51, 819–823. doi: 10.1194/jlr.M002543
Schuurs-Hoeijmakers, J. H. M., Geraghty, M. T., Kamsteeg, E.-J., Ben-Salem, S., de Bot, S. T., Nijhof, B., et al. (2012). Mutations in DDHD2, encoding an intracellular phospholipase A1, cause a recessive form of complex hereditary spastic paraplegia. Am. J. Hum. Genet. 91, 1073–1081. doi: 10.1016/j.ajhg.2012.10.017
Sheikh, K. A., Sun, J., Liu, Y., Kawai, H., Crawford, T. O., Proia, R. L., et al. (1999). Mice lacking complex gangliosides develop Wallerian degeneration and myelination defects. Proc. Natl. Acad. Sci. U.S.A. 96, 7532–7537. doi: 10.1073/pnas.96.13.7532
Siller, M., Goyal, S., Yoshimoto, F. K., Xiao, Y., Wei, S., and Guengerich, F. P. (2014). Oxidation of endogenous N-arachidonoylserotonin by human cytochrome P450 2U1. J. Biol. Chem. 289, 10476–10487. doi: 10.1074/jbc.M114.550004
Simons, J. P., Al-Shawi, R., Minogue, S., Waugh, M. G., Wiedemann, C., Evangelou, S., et al. (2009). Loss of phosphatidylinositol 4-kinase 2α activity causes late onset degeneration of spinal cord axons. Proc. Natl. Acad. Sci. U.S.A. 106, 11535–11539. doi: 10.1073/pnas.0903011106
Sjogren, T., and Larsson, T. (1957). Oligophrenia in combination with congenital ichthyosis and spastic disorders; a clinical and genetic study. Acta Psychiatr. Neurol. Scand. Suppl. 113, 1–112.
Soderblom, C., and Blackstone, C. (2006). Traffic accidents: molecular genetic insights into the pathogenesis of the hereditary spastic paraplegias. Pharmacol. Ther. 109, 42–56. doi: 10.1016/j.pharmthera.2005.06.001
Stelten, B. M. L., Huidekoper, H. H., van de Warrenburg, B. P. C., Brilstra, E. H., Hollak, C. E. M., Haak, H. R., et al. (2019). Long-term treatment effect in cerebrotendinous xanthomatosis depends on age at treatment start. Neurology 92, e83–e95. doi: 10.1212/WNL.0000000000006731
Stevanin, G., Azzedine, H., Denora, P., Boukhris, A., Tazir, M., Lossos, A., et al. (2008). Mutations in SPG11 are frequent in autosomal recessive spastic paraplegia with thin corpus callosum, cognitive decline and lower motor neuron degeneration. Brain 131, 772–784. doi: 10.1093/brain/awm293
Stevanin, G., Rastetter, A., Esteves, T., Hanein, S., Depienne, C., Brice, A., et al. (2019). Dominant negative heterozygous mutation in Erlin2 prevents degradation of IP3 receptors and is responsible for hereditary spastic paraplegia 37. Eur. J. Hum. Genet. 1174–1813. doi: 10.1038/s41431-019-04942
Stevanin, G., Santorelli, F. M., Azzedine, H., Coutinho, P., Chomilier, J., Denora, P. S., et al. (2007). Mutations in SPG11, encoding spatacsin, are a major cause of spastic paraplegia with thin corpus callosum. Nat. Genet. 39, 366–372. doi: 10.1038/ng1980
Sultana, S., Reichbauer, J., Schüle, R., Mochel, F., Synofzik, M., and van der Spoel, A. C. (2015). Lack of enzyme activity in GBA2 mutants associated with hereditary spastic paraplegia/cerebellar ataxia (SPG46). Biochem. Biophys. Res. Commun. 465, 35–40. doi: 10.1016/j.bbrc.2015.07.112
Suzuki, S. O., Iwaki, T., Arakawa, K., Furuya, H., Fujii, N., and Iwaki, A. (2011). An autopsy case of adult-onset hereditary spastic paraplegia type 2 with a novel mutation in exon 7 of the proteolipid protein 1 gene. Acta Neuropathol. (Berl.) 122, 775–781. doi: 10.1007/s00401-011-0916-x
Svennerholm, L., Vanier, M. T., and Månsson, J. E. (1980). Krabbe disease: a galactosylsphingosine (psychosine) lipidosis. J. Lipid Res. 21, 53–64.
Synofzik, M., Hufnagel, R. B., and Züchner, S. (2014). “PNPLA6-related disorders,” in GeneReviews®, eds M. P. Adam, H. H. Ardinger, R. A. Pagon, S. E. Wallace, L. J. Bean, K. Stephens, et al. (Seattle, WA: University of Washington, Seattle).
Szymanski, K. M., Binns, D., Bartz, R., Grishin, N. V., Li, W.-P., Agarwal, A. K., et al. (2007). The lipodystrophy protein seipin is found at endoplasmic reticulum lipid droplet junctions and is important for droplet morphology. Proc. Natl. Acad. Sci. U.S.A. 104, 20890–20895. doi: 10.1073/pnas.0704154104
Takahashi, H., and Suzuki, K. (1984). Demyelination in the spinal cord of murine globoid cell leukodystrophy (the twitcher mouse). Acta Neuropathol. (Berl.) 62, 298–308. doi: 10.1007/BF00687612
Tanaka, S., Maeda, Y., Tashima, Y., and Kinoshita, T. (2004). Inositol deacylation of glycosylphosphatidylinositol-anchored proteins is mediated by mammalian PGAP1 and yeast Bst1p. J. Biol. Chem. 279, 14256–14263. doi: 10.1074/jbc.M313755200
Tesson, C., Nawara, M., Salih, M. A. M., Rossignol, R., Zaki, M. S., Al Balwi, M., et al. (2012). Alteration of fatty-acid-metabolizing enzymes affects mitochondrial form and function in hereditary spastic paraplegia. Am. J. Hum. Genet. 91, 1051–1064. doi: 10.1016/j.ajhg.2012.11.001
Theda, C., Moser, A. B., Powers, J. M., and Moser, H. W. (1992). Phospholipids in X-linked adrenoleukodystrophy white matter: fatty acid abnormalities before the onset of demyelination. J. Neurol. Sci. 110, 195–204. doi: 10.1016/0022-510X(92)90028-J
Theofilopoulos, S., Griffiths, W. J., Crick, P. J., Yang, S., Meljon, A., Ogundare, M., et al. (2014). Cholestenoic acids regulate motor neuron survival via liver X receptors. J. Clin. Invest. 124, 4829–4842. doi: 10.1172/JCI68506
Trotta, N., Orso, G., Rossetto, M. G., Daga, A., and Broadie, K. (2004). The Hereditary Spastic Paraplegia Gene, Spastin, Regulates Microtubule Stability To Modulate Synaptic Structure And Function. Curr. Biol. 14, 1135–1147. doi: 10.1016/j.cub.2004.06.058
Tsaousidou, M. K., Ouahchi, K., Warner, T. T., Yang, Y., Simpson, M. A., Laing, N. G., et al. (2008). Sequence alterations within CYP7B1 implicate defective cholesterol homeostasis in motor-neuron degeneration. Am. J. Hum. Genet. 82, 510–515. doi: 10.1016/j.ajhg.2007.10.001
Twig, G., Elorza, A., Molina, A. J. A., Mohamed, H., Wikstrom, J. D., Walzer, G., et al. (2008). Fission and selective fusion govern mitochondrial segregation and elimination by autophagy. EMBO J. 27, 433–446. doi: 10.1038/sj.emboj.7601963
van Rappard, D. F., Boelens, J. J., van Egmond, M. E., Kuball, J., van Hasselt, P. M., Oostrom, K. J., et al. (2016). Efficacy of hematopoietic cell transplantation in metachromatic leukodystrophy: the Dutch experience. Blood 127, 3098–3101. doi: 10.1182/blood-2016-03-708479
van Roermund, C. W. T., Visser, W. F., IJlst, L., van Cruchten, A., Boek, M., Kulik, W., et al. (2008). The human peroxisomal ABC half transporter ALDP functions as a homodimer and accepts acyl-CoA esters. FASEB J. 22, 4201–4208. doi: 10.1096/fj.08-110866
Vantaggiato, C., Panzeri, E., Castelli, M., Citterio, A., Arnoldi, A., Santorelli, F. M., et al. (2019). ZFYVE26/SPASTIZIN and SPG11/SPATACSIN mutations in hereditary spastic paraplegia types AR-SPG15 and AR-SPG11 have different effects on autophagy and endocytosis. Autophagy 15, 34–57. doi: 10.1080/15548627.2018.1507438
Varga, R.-E., Khundadze, M., Damme, M., Nietzsche, S., Hoffmann, B., Stauber, T., et al. (2015). In vivo evidence for lysosome depletion and impaired autophagic clearance in hereditary spastic paraplegia type SPG11. PLoS Genet. 11:e1005454. doi: 10.1371/journal.pgen.1005454
Waldhüter, N., Köhler, W., Hemmati, P. G., Jehn, C., Peceny, R., Vuong, G. L., et al. (2019). Allogeneic hematopoietic stem cell transplantation with myeloablative conditioning for adult cerebral X-linked adrenoleukodystrophy. J. Inherit. Metab. Dis. 42, 313–324. doi: 10.1002/jimd.12044
Wang, H., Lo, W.-T., and Haucke, V. (2019). Phosphoinositide switches in endocytosis and in the endolysosomal system. Curr. Opin. Cell Biol. 59, 50–57. doi: 10.1016/j.ceb.2019.03.011
Watkins, P. A., Gould, S. J., Smith, M. A., Braiterman, L. T., Wei, H. M., Kok, F., et al. (1995). Altered expression of ALDP in X-linked adrenoleukodystrophy. Am. J. Hum. Genet. 57, 292–301.
Wei, S., Soh, S. L.-Y., Xia, J., Ong, W.-Y., Pang, Z. P., and Han, W. (2014). Motor neuropathy-associated mutation impairs Seipin functions in neurotransmission. J. Neurochem. 129, 328–338. doi: 10.1111/jnc.12638
Wittke, D., Hartmann, D., Gieselmann, V., and Lüllmann-Rauch, R. (2004). Lysosomal sulfatide storage in the brain of arylsulfatase A-deficient mice: cellular alterations and topographic distribution. Acta Neuropathol. (Berl.) 108, 261–271. doi: 10.1007/s00401-004-08836
Wnętrzak, A., Makyła-Juzak, K., Filiczkowska, A., Kulig, W., and Dynarowicz-Łątka, P. (2017). Oxysterols versus cholesterol in model neuronal membrane. I. The case of 7-ketocholesterol, the langmuir monolayer study. J. Membr. Biol. 250, 553–564. doi: 10.1007/s00232-017-99848
Woeste, M. A., Stern, S., Raju, D. N., Grahn, E., Dittmann, D., Gutbrod, K., et al. (2019). Species-specific differences in nonlysosomal glucosylceramidase GBA2 function underlie locomotor dysfunction arising from loss-of-function mutations. J. Biol. Chem. 294, 3853–3871. doi: 10.1074/jbc.RA118.006311
Wong, J. C., Walsh, K., Hayden, D., and Eichler, F. S. (2018). Natural history of neurological abnormalities in cerebrotendinous xanthomatosis. J. Inherit. Metab. Dis. 41, 647–656. doi: 10.1007/s10545-018-01529
Wood, P. L., Smith, T., Pelzer, L., and Goodenowe, D. B. (2011). Targeted metabolomic analyses of cellular models of pelizaeus-merzbacher disease reveal plasmalogen and myo-inositol solute carrier dysfunction. Lipids Health Dis. 10:102. doi: 10.1186/1476-511X-10102
Wortmann, S. B., Vaz, F. M., Gardeitchik, T., Vissers, L. E. L. M., Renkema, G. H., Schuurs-Hoeijmakers, J. H. M., et al. (2012). Mutations in the phospholipid remodeling gene SERAC1 impair mitochondrial function and intracellular cholesterol trafficking and cause dystonia and deafness. Nat. Genet. 44, 797–802. doi: 10.1038/ng.2325
Yahalom, G., Tsabari, R., Molshatzki, N., Ephraty, L., Cohen, H., and Hassin-Baer, S. (2013). Neurological outcome in cerebrotendinous xanthomatosis treated with chenodeoxycholic acid: early versus late diagnosis. Clin. Neuropharmacol. 36, 78–83. doi: 10.1097/WNF.0b013e318288076a
Yang, L. J., Zeller, C. B., Shaper, N. L., Kiso, M., Hasegawa, A., Shapiro, R. E., et al. (1996). Gangliosides are neuronal ligands for myelin-associated glycoprotein. Proc. Natl. Acad. Sci. U.S.A. 93, 814–818. doi: 10.1073/pnas.93.2.814
Yao, D., McGonigal, R., Barrie, J. A., Cappell, J., Cunningham, M. E., Meehan, G. R., et al. (2014). Neuronal expression of GalNAc transferase is sufficient to prevent the age-related neurodegenerative phenotype of complex ganglioside-deficient mice. J. Neurosci. 34, 880–891. doi: 10.1523/JNEUROSCI.3996-13.2014
Yoshii, Y., Furukawa, T., Saga, T., and Fujibayashi, Y. (2015). Acetate/acetyl-CoA metabolism associated with cancer fatty acid synthesis: overview and application. Cancer Lett. 356, 211–216. doi: 10.1016/j.canlet.2014.02.019
Yung, Y. C., Stoddard, N. C., Mirendil, H., and Chun, J. (2015). Lysophosphatidic acid signaling in the nervous system. Neuron 85, 669–682. doi: 10.1016/j.neuron.2015.01.009
Zhang, K., Kniazeva, M., Han, M., Li, W., Yu, Z., Yang, Z., et al. (2001). A 5-bp deletion in ELOVL4 is associated with two related forms of autosomal dominant macular dystrophy. Nat. Genet. 27, 89–93. doi: 10.1038/83817
Zoller, I., Meixner, M., Hartmann, D., Bussow, H., Meyer, R., Gieselmann, V., et al. (2008). Absence of 2-hydroxylated sphingolipids is compatible with normal neural development but causes late-onset axon and myelin sheath degeneration. J. Neurosci. 28, 9741–9754. doi: 10.1523/JNEUROSCI.0458-08.2008
Keywords: phospholipids, sphingolipids, fatty acids, cholesterol, metabolism, myelin, lysosome
Citation: Darios F, Mochel F and Stevanin G (2020) Lipids in the Physiopathology of Hereditary Spastic Paraplegias. Front. Neurosci. 14:74. doi: 10.3389/fnins.2020.00074
Received: 30 September 2019; Accepted: 20 January 2020;
Published: 28 February 2020.
Edited by:
Patrícia Maciel, University of Minho, PortugalReviewed by:
Javier Fernández-Ruiz, Complutense University of Madrid, SpainJürgen Winkler, University of Erlangen-Nuremberg, Germany
Copyright © 2020 Darios, Mochel and Stevanin. This is an open-access article distributed under the terms of the Creative Commons Attribution License (CC BY). The use, distribution or reproduction in other forums is permitted, provided the original author(s) and the copyright owner(s) are credited and that the original publication in this journal is cited, in accordance with accepted academic practice. No use, distribution or reproduction is permitted which does not comply with these terms.
*Correspondence: Frédéric Darios, frederic.darios@upmc.fr