- 1Departamento de Nutrição, Universidade Federal de Pernambuco, Recife, Brazil
- 2Departamento de Educação Física, Centro Universitário Católico de Quixadá, Quixadá, Brazil
This study aimed to evaluate the possible protective role of taurine on anxiety-like behavior, brain electrical activity and glial cell immunoreactivity in well-nourished and malnourished rats that were treated with a subconvulsing dose of pilocarpine. Newborn Wistar rats were subjected to normal or unfavorable lactation conditions, represented by the suckling of litters with 9 or 15 pups, resulting in well-nourished and malnourished animals, respectively. Each nutritional group was split into five subgroups that were treated from postnatal day (PND) 35 to 55 with 300 mg/kg/day of taurine + 45 mg/kg/day of pilocarpine (group T + P), taurine only (group T), pilocarpine only (group P), vehicle control (group V), or not treated control (group naïve; Nv). At PND56-58, the groups were subjected to the elevated plus-maze behavioral tests. Glycemia was measured on PND59. Between PND60 and PND65, the cortical spreading depression (CSD) was recorded in the cerebral cortex, and the levels of malondialdehyde and microglial and astrocyte immunoreactivity were evaluated in the cortex and hippocampus. Our data indicate that treatment with taurine and pilocarpine resulted in anxiolytic-like and anxiogenic behavior, respectively, and that nutritional deficiency modulated these effects. Both treatments decelerated CSD propagation and modulated GFAP- and Iba1-containing glial cells. Pilocarpine reduced body weight and glycemia, and administration of taurine was not able to attenuate the effects of pilocarpine. The molecular mechanisms underlying taurine action on behavioral and electrophysiological parameters in the normal and altered brain remain to be further explored.
Introduction
Taurine is an amino sulfonic acid that is found abundantly in several areas of the mammalian central nervous system. It is a structural analog of the inhibitory transmitter gamma-aminobutyric acid (GABA), which is recognized as one of the most important inhibitory amino acids distributed in the brain tissue (Barbeau et al., 1975; Yakimova et al., 1996; Ripps and Shen, 2012). Taurine mediates a myriad of physiological processes in the nervous system, including neuromodulation, maintenance of calcium homeostasis, and neuronal proliferation and differentiation (Chen et al., 1998). Administration of exogenous taurine improves glucose homeostasis in genetically obese animals (Santos-Silva et al., 2015), promotes an anxiolytic-like behavioral profile (Murakami and Furuse, 2010; Francisco and Guedes, 2015), acts as an antioxidant and anti-inflammatory molecule (Oliveira et al., 2010; Marcinkiewicz and Kontny, 2014) and rescues hippocampal long-term potentiation (LTP) from ammonia-induced impairment (Chepkova et al., 2006). Furthermore, taurine reportedly acts as a neuroprotectant in epilepsy, reducing or abolishing seizures (Junyent et al., 2011; Oja and Saransaari, 2013; see a recent review in Hrnčić et al., 2018).
The acute systemic injection of pilocarpine in rodents constitutes an effective, experimental model largely used to study the pathophysiology of seizures and to identify potential therapeutic agents for the treatment of epilepsy (Santos et al., 2000). This model was first described by Turski et al. (1983a, b)); it consists of the single administration of a high dose (300–380 mg/kg; Guedes and Cavalheiro, 1997) or various consecutive low doses of pilocarpine until induction of status epilepticus (Glien et al., 2001). This acute phase is followed by a condition of permanent recurrent spontaneous seizures, altering the central nervous system structure and function (Turski et al., 1989) with behavioral and electroencephalographic changes that are similar to those observed in human temporal lobe epilepsy. In the last decade, some studies had given special attention to possible effects of subconvulsing doses of pilocarpine when administered acutely. Under this condition, no behavioral or electrocorticographic changes indicative of seizures were observed (Guedes and Vasconcelos, 2008). However, under subconvulsing paradigms several reports have described anxiety-like behavioral profiles (Duarte et al., 2014; Francisco and Guedes, 2018), reductions in glycemia (Francisco and Guedes, 2018), increases in brain oxidative stress (Mendes-da-Silva et al., 2018) and impairment of propagation of the excitability-related phenomenon known as cortical spreading depression (CSD) along the cortical rodent tissue (Francisco and Guedes, 2018; Mendes-da-Silva et al., 2018).
Cortical spreading depression is a brain phenomenon that is based on neuronal and glial depolarization and is influenced by conditions that modify neural excitability, including cholinergic agonists (Guedes and Cavalheiro, 1997). CSD has been related to excitability-associated diseases such as migraine with aura (Lauritzen, 1994); CSD in the injured human brain was first shown by Mayevsky et al. (1998) and Strong et al. (2002). CSD has been also associated with ischemic stroke (Dohmen et al., 2008), traumatic brain injury (Hartings et al., 2009), subarachnoid hemorrhage (Dreier, 2011), multiple sclerosis (Pusic et al., 2015), and epilepsy (Vinogradova et al., 2006; Dreier et al., 2012). Evidence suggests that chemicals such as potassium (Grafstein, 1956) or glutamate (Van Harreveld, 1959; Marrannes et al., 1988; Pietrobon and Moskowitz, 2014; Hertelendy et al., 2018), as well as various other neurotransmitters and neuromodulators (Ayata and Lauritzen, 2015; Guedes et al., 2017) might be involved in CSD, either eliciting, or modulating the phenomenon. Experimental evidence demonstrated that CSD can potentiate the brain’s spontaneous and evoked electrical activity, both in vitro (Footitt and Newberry, 1998) and in vivo (Guedes et al., 2005; Souza et al., 2015). Under conditions of environmental, nutritional and pharmacological manipulations, our group has extensively employed the CSD model to evaluate the proper functioning of the brain in health and disease. When occurring early in life, such conditions can affect brain development and functioning, and can substantially alter the ability of the brain to produce and propagate CSD (see Guedes, 2011 for an overview). Recently, we demonstrated that the chronic administration (21 days) of a very low, subconvulsive dose of pilocarpine (45 mg/kg/day) is able to counteract CSD, and this effect is modulated by nutritional deficiency (Francisco and Guedes, 2018).
In the present study we tested the hypothesis that taurine modulates the CSD effects of pilocarpine, in association, or not, with early malnutrition. In addition, we investigated the taurine/pilocarpine/malnutrition interaction on anxiety-like behavior, fasting glycemia and oxidative stress. Finally, some structural correlates of this interaction were investigated by correlating the experimental treatments with the astrocytic and microglial immunostaining pattern in the cerebral cortex and hippocampus.
Materials and Methods
Animals
All experimental procedures were previously approved by the Institutional Ethics Committee for Animal Research of the Federal University of Pernambuco (approval protocol no. 23076.015655/2015-99), whose norms comply with the norms established by the National Institutes of Health Guide for Care and Use of Laboratory Animals (Bethesda, MD, United States). Newborn Wistar rats of both sexes, born from different dams, were assigned to be suckled under normal or unfavorable lactation conditions, represented respectively by litters with nine pups (L9 groups) and litters with 15 pups (L15 groups), as previously described (Francisco and Guedes, 2015). Weaning occurred on postnatal day (PND) 21, when pups were separated by sex and housed in polypropylene cages (51 cm × 35.5 cm × 18.5 cm; three rats per cage) under a 12-h light:12-h dark cycle (lights on at 6:00 a.m.), controlled temperature (23 ± 1°C), and with free access to water and the same commercial lab chow, with 23% protein, that was offered to their dams during the lactation period (Purina, Ltd.). In this study, we analyzed data from male pups only, i.e., 46 L9 and 45 L15 rats.
Administration of Pilocarpine and/or Taurine
Pilocarpine hydrochloride, scopolamine methyl nitrate, and taurine were purchased from Sigma-Aldrich (St. Louis, MO, United States). All solutions were prepared daily, shortly before the injections, dissolved in 0.9% saline and administered from PND35 to PND55. Each lactation condition gave rise to the following five subgroups: (1) Naïve (control group, without any treatment; n = 10 L9 and 7 L15 rats); (2) Vehicle (second control group; treated with saline via i.p. and gavage; n = 10 L9 and 8 L15 rats); (3) Taurine (300 mg/kg/day via gavage; n = 9 L9 and 9 L15 rats), as previously described (Francisco and Guedes, 2015); (4) Pilocarpine (45 mg/kg/day via i.p.; n = 9 L9 and 10 L15 rats), following Mendes-da-Silva et al. (2018); (5) Taurine plus pilocarpine (300 mg/kg/day via gavage and 45 mg/kg/day via i.p, respectively; n = 8 L9 and 11 L15 rats). Scopolamine methyl nitrate, a muscarinic receptor antagonist, was administered (1 mg/kg/day via i.p.) in all groups, with the exception of the naïve group, 30 min before pilocarpine or saline administration to prevent the peripheral cholinergic effects elicited by pilocarpine (Peixinho-Pena et al., 2012). Immediately following pilocarpine administration, the animals were observed over 1 h to confirm the absence of seizures, as evaluated by the Racine scale (Racine, 1972). At this low dose of pilocarpine, 45 mg/kg/d, no behavioral signs of epilepsy were observed in the animals (all rats presented with Racine’s score of zero).
Body and Brain Weights
Body weight was measured using a Filizola MF-3/1 electronic scale (3.0 kg capacity and precision of 0.5 g) at PND7, PND21, PND35, PND49, and PND60. The brain weights were obtained using an analytical balance (Shimadzu, model AUY220, with a sensitivity of up to 0.1 mg) at the end of the transcardiac perfusion procedure (see item 2.8).
Elevated Plus-Maze Test
The elevated plus-maze test (EPM) was conducted at PND56-58. The cross-shaped EPM apparatus was made of varnished wood and consisted of four arms (49 cm × 10 cm each) elevated 55 cm above the ground. Two of the arms were opened and the other two arms were closed (lateral walls of 50 cm of height), arranged perpendicular to the open ones. The arms of the apparatus were joined by a central 10 cm × 10 cm square platform. At the beginning of the test, each animal was placed individually in the central area of the labyrinth, with the head directed toward one of the open arms. Each animal was allowed to freely explore the labyrinth for 5 min, under dim light and in a sound-attenuated room. Before each test, the EPM apparatus was wiped with a paper cloth soaked in 70:30 ethanol:water solution. The animal’s behavioral activity was recorded by a video camera. The video-recorded activity was stored in a computer and subsequently analyzed with the aid of the software ANY mazeTM (version 4.99 m), as previously described (Lima et al., 2017). The following parameters were considered: number of expelled fecal boluses, total distance traveled, total immobility time, number of entries into the open arms and the time spent in the open arms.
Analysis of Blood Glucose
As reported formerly (Francisco and Guedes, 2015), on PND59 the animals were fasted for 6 h and a drop of blood was collected from the animal’s tail and used for measuring the blood glucose level using a portable glucose meter (G-TECH free).
CSD Recording
On the day of the electrophysiological recording (PND60–PND65), animals were anesthetized with a mixture of 1000 mg/kg urethane plus 40 mg/kg chloralose injected intraperitoneally. The level of anesthesia was monitored as previously described (Souza et al., 2015). Three trephine holes were drilled on the right side of the skull, aligned in the frontal-to-occipital direction and parallel to the midline. One hole was positioned on the frontal bone (2 mm in diameter) and used to apply the stimulus (KCl) to elicit CSD. The other two holes were positioned on the parietal bone (3–4 mm in diameter) and used to record the propagating CSD wave. Rectal temperature was continuously monitored and maintained at 37 ± 1°C by means of a heating blanket. The electrophysiological recording session lasted 6 h. We used two Ag–AgCl agar–Ringer electrodes (one in each hole) against a common reference electrode of the same type, placed on the nasal bones. The two initial recording hours constituted the baseline period, during which no KCl stimulus was applied and, consequently, no CSD was elicited. In the remaining four recording hours, CSD episodes were elicited at 30-min intervals by a 1-min application of a cotton ball (1–2 mm in diameter) soaked with 2% KCl solution (approximately 270 mM) to the anterior hole drilled at the frontal region. The ECoG and the DC (direct current) slow potential variation that is typical of CSD were continuously recorded on the cortical surface (on the intact dura mater) through a digital recording system (Biopac MP 150, Goleta, CA, United States). For each animal, the amplifier’s gain was kept constant over the entire recording session, as previously reported (Lopes-de-Morais et al., 2014).
We calculated the CSD velocity of propagation from the time required for a CSD wave to pass the distance between the two cortical electrodes. In the two recording locations, we used the initial point of each DC-negative rising phase as the reference point to calculate the CSD velocities. In addition, we calculated the amplitude and duration of the CSD waves, as previously reported (Lima et al., 2017). As the basis for assessing the occurrence of CSD-dependent potentiation of spontaneous electrical activity, in each animal we compared the amplitudes of the ECoG before (baseline recording) and after starting to regularly elicit CSD. For this comparison, we analyzed six 10-min samples of the record at six time points of the ECoG, i.e., two samples from the baseline period and four samples from the CSD period. These samples were analyzed offline with the aid of an algorithm implemented in MATLABTM software (The Mathworks, Natick, MA, United States), version R2011B. This algorithm calculates the average amplitude of the ECoG waves. For each animal, the averaged ECoG amplitude was normalized in relation to the lowest sample value, which was considered equal to 1, expressed in relative units and compared before and after the episodes of CSD, as a basis for analyzing the occurrence of potentiation of the spontaneous electrical activity, as reported by our group previously (Lopes-de-Morais et al., 2014; Souza et al., 2015).
Lipid Peroxidation Analysis
After the CSD recording session, 50 of the still-anesthetized animals (26 L9 and 24 L15 rats) were decapitated; their brains were rapidly removed and frozen. The cortical tissue was homogenized in a cold Tris buffer solution and centrifuged for 10 min at 1000 g at 4°C. Supernatants were used to estimate the lipid peroxidation by measuring malondialdehyde (MDA) levels using a thiobarbituric acid-reactive substances-based method (Ohkawa et al., 1979), which is a parameter to evaluate the lipid peroxidation. The reaction was developed by the sequential addition of 40 μl 8.1% sodium dodecyl sulfate, 300 μl 20% acetic acid (pH 3.5), and 300 μl 0.8% thiobarbituric acid solutions to the 300 μl homogenate aliquot in a boiling water bath for 50 min. After cooling the tubes with tap water, 300 μl of n-butanol was added to the sample. The tubes were centrifuged at 2500 g for 10 min, and the organic phase was read at 532 nm using a plate reader. Measurements were carried out in triplicate. Total protein concentrations were determined based on the Bradford protein assay; bovine serum albumin was used as a standard. MDA concentrations were determined by using 1,1,3,3-tetraethoxypropane as the standard and expressed as μg/mg protein, as previously reported (Mendes-da-Silva et al., 2014). All measurements were performed in triplicate.
Immunohistochemistry
After the CSD recording session, forty-one of the still-anesthetized animals were perfused with 0.9% saline solution followed by 4% paraformaldehyde diluted in 0.1M phosphate-buffered saline (pH 7.4). After being immersed in the fixative for 4 h, the brains were subjected to cryoprotection in sucrose solutions of increasing concentrations of 10%, 20% and 30%. Longitudinal serial sections (40-μm thickness) from the left (CSD-free) hemisphere were obtained at −20°C using a cryoslicer (Leica, 1850). One of the 41 animals was processed as a negative control for the immunolabeling. The remaining 40 rats included 20 from the L9 and 20 from the L15 condition. In each lactation condition, 4 rats were from each one of the five treatment groups. Their brains were processed for microglia immunolabeling with anti- ionized calcium binding adapter molecule 1 (Iba1) antibody and astrocyte immunolabeling with anti-GFAP antibody.
For microglia immunostaining, sections were immunolabeled with a polyclonal antibody against Iba-1 (1:1500; anti-Iba-1, #019-19741; Wako Pure Chemical Industries, Ltd., Osaka, Japan). Free-floating sections were subjected to endogenous peroxidase blocking (2% H2O2 in 70% methanol for 10 min) and the sections were incubated for 1 h in blocking buffer (BB) solution containing 0.05M Tris-buffered saline (TBS; pH 7.4), 10% fetal calf serum, 3% bovine serum albumin, and 1% Triton X-100. The sections were then incubated overnight at 4°C with rabbit anti-Iba-1 (1:1500 diluted in BB solution). After three washes with TBS + 1% Triton X-100, sections were incubated at room temperature for 1 h with biotinylated anti-rabbit (1:500) secondary antibodies. Sections were then rinsed in TBS + 1% Triton X-100 and incubated with horseradish peroxidase streptavidin (1:500). The peroxidase reaction was visualized by incubating the sections in Tris buffer containing 0.5 mg/ml 3,3′-diaminobenzidine) and 0.33 μl/ml H2O2. The experimental protocol used for astrocyte immunostaining was similar to that applied for microglial labeling, mentioned above, with the following change: the primary antibody that was used (polyclonal rabbit anti-GFAP-D1F4Q-XP RABBIT MAB; Dako, Denmark) was specific for astrocyte labeling at the ratio of 1:2400.
Finally, the sections were mounted, dehydrated in graded alcohols, and coverslipped with Entellan® after xylene treatment. Densitometric analysis was performed on four parallel longitudinal sections for each animal. A Leica DMLS microscope coupled to a Samsung high-level color camera (model SHC-410NAD) was used to obtain digital images from the brain sections. Images from selected regions of interest (Figure 1) of the parietal cortex and CA1 hippocampus stained for Iba1 and GFAP were obtained using a 20 × microscope objective. In Figure 1, the protocol for double labeling was as follows: the sections were incubated simultaneously with mouse monoclonal anti-GFAP (Sigma-Aldrich; United States, #G3893, 1:1000) and rabbit polyclonal anti-neuN (Novus Biologicals, United States, #NBP1-77686, 1:200) for 18 h. Then, they were rinsed in phosphate buffer 0.1M (PB), pH 7.4 followed by incubation for 4 h with Cy-3 conjugated 546 labeled anti-mouse IgG and FITC-conjugated 488 anti-rabbit IgG (1:500; Jackson ImmunoResearch Labs, United States). After washing twice in PB, they were mounted onto gelatin coated slides and dried at 50°C for 5 min, cleared in xylene for 1 min and coverslipped with Entellan (Merck-Millipore, United States). Digital images were obtained using an epifluorescence microscope (Nikon coupled to a high-level color camera Model SHC-410NAD). The CA1 area and the parietal neocortex were chosen based on morphological and pharmacological studies in pilocarpine-treated animals (Curia et al., 2008; Rossi et al., 2013; Arisi et al., 2015; García-García et al., 2017). In each section, photomicrographs of four fields within the parietal cortex (layers 2 and 3) and three fields of the CA1 hippocampal region (including Stratum Oriens, Stratum Pyramidalis, Stratum Radiatum, and Stratum Lacunosum Moleculare) were analyzed, using the ImageJ software (National Institutes of Health, United States, version 1.46r). Care was taken to obtain the digital images using the same light intensity. The color images were first converted into a gray scale. Based on the color difference, an algorithm of the program, devoted to area selection, identified the darker areas (marked cells) in relation to the lighter areas (background), and the total marked area was calculated. The threshold for selection was manually adjusted such that the background was not marked. All sections that were photographed at the same magnification displayed the same total area in the photographs; therefore, the ratios between the labeled cells’ area and the total picture area could be directly compared. The labeled area was expressed as percentage of the total area in the picture. The immunoreactivity intensity was obtained in the program by calculating the mean gray value (MGV) within the selected area. The MGV can vary numerically from 0 (darkest) to 255 (lightest). Therefore, the reactivity intensity was given by the difference (255-MGV). By multiplying this value by the marked area, we came to the figure (arbitrary unit) that indicated how much of the gray area in the image was due to cell labeling, i.e., mathematically, the more intense the labeling, the greater is the arbitrary unit value. Total immunoreactivity expressed as arbitrary units as well as the percentage of the area occupied by the immunolabeled cells were analyzed, as previously reported (Lima et al., 2017).
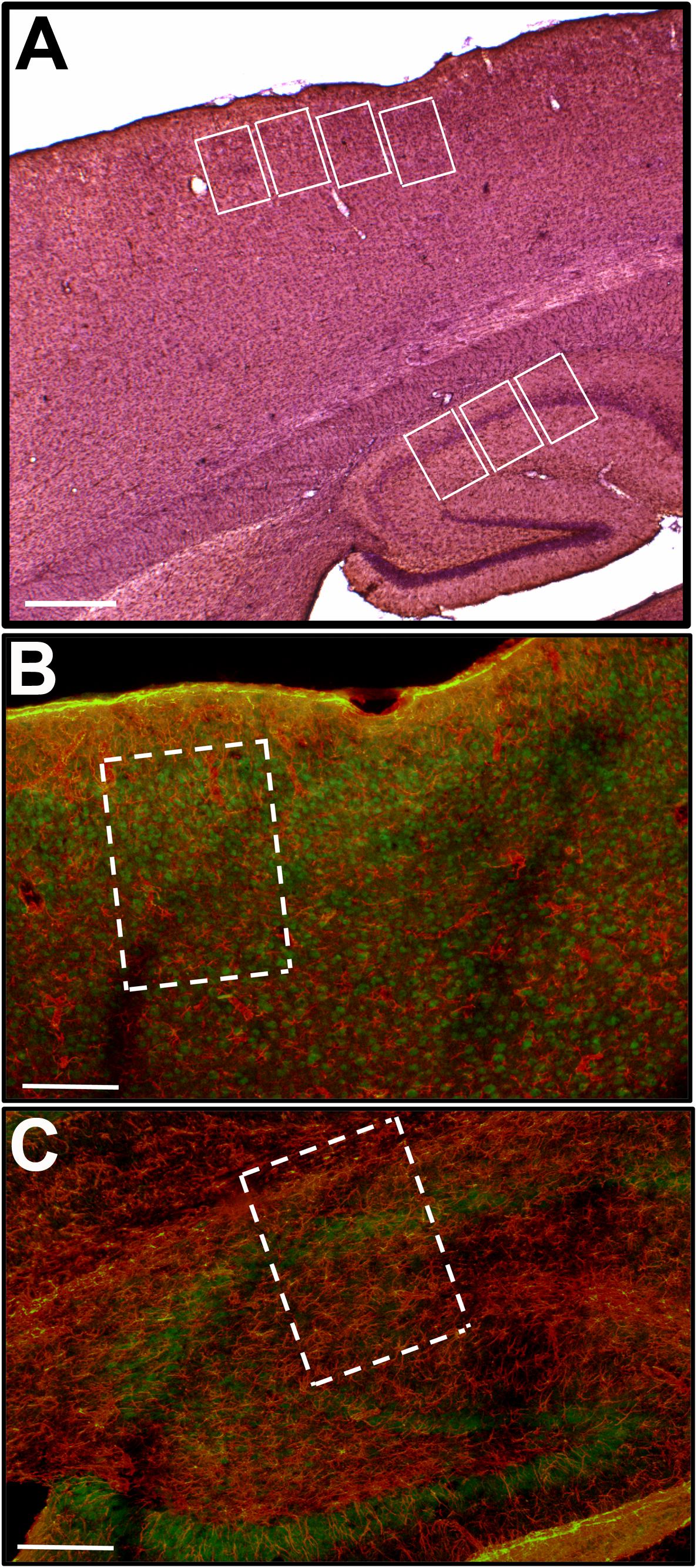
Figure 1. (A) Shows a low magnification (2.5×) image of a representative parasagittal section immunostained for GFAP and counterstained with 5% Hematoxylin. The white rectangles illustrate the position of the sampling windows (470 μm × 340 μm) in the cerebral cortex and CA1 region of hippocampus. Double staining for GFAP (red) and Neu-N (green) was performed in order to better define the cytoarchitectonic limits of layers 2 and 3 in the cerebral cortex (B; 10×) as well as the four layers (Stratum Oriens, Stratum Pyramidalis, Stratum Radiatum, and Stratum Lacunosum Moleculare) in the hippocampus (C; 10×), where the quantitative analysis was carried out. Scale bars equal 500 μm in (A), and 200 μm in (B,C).
Statistical Analysis
Results in all groups are expressed as the means ± standard deviations (SD). Intergroup differences and interactions were first analyzed using a two-way ANOVA and thereafter a MANOVA. In the ANOVA analysis, we consider, as factors, nutritional status (L9 and L15) and treatment (naïve, vehicle, taurine, pilocarpine, and taurine + pilocarpine). In the MANOVA analysis we included nutritional status (L9 and L15), pilocarpine administration and taurine treatment as factors. This was followed by a post hoc test (Holm–Sidak) where indicated. ECoG amplitude values before and after CSD for each animal were normalized and expressed in relative units. Differences in these amplitudes, before and after CSD, were analyzed with the paired t-test, using ANOVA followed by the Holm–Sidak test for intergroup comparisons when indicated. Differences with p < 0.05 were accepted as significant. Since MANOVA confirmed the differences that had been previously indicated by two-way ANOVA, we kept the ANOVA statistics figures in the description of results.
Results
The two control groups – naïve (no treatment) and vehicle (that received gavage and intraperitoneal injection of saline and scopolamine) presented comparable values.
Body and Brain Weights
As shown in Figure 2A, in all treatment groups (Nv, V, T, P, and T + P) ANOVA showed a main effect of the lactation condition on body weight (p < 0.001). The L15 animals presented with lower body weights compared with the corresponding L9 groups. In the control condition (naïve and vehicle groups) the weight reduction ranged from 11.9 to 32.5%. In the L9 condition, intergroup differences were observed at PND49 only [F(4,85) = 15.048; p < 0.001]. At that age, the treatment with pilocarpine was associated with a weight reduction compared to the respective L9 control groups. In the unfavorable (L15) lactation condition, pilocarpine and taurine + pilocarpine treatments reduced body weight at PND49 and PND60 [F(4,79) = 11.993; p < 0.001].
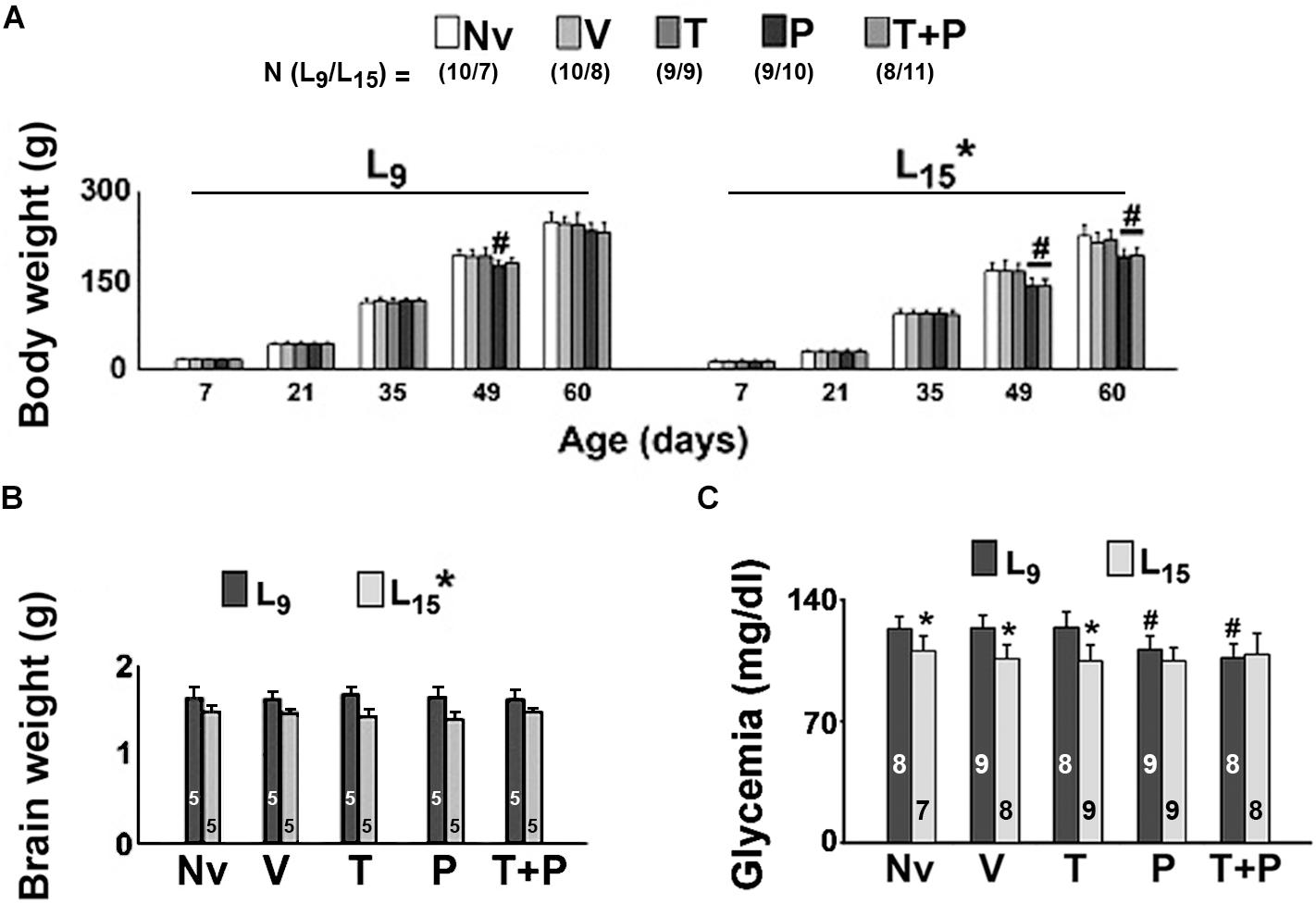
Figure 2. Body (A) and brain weights (B), and glycemia (C) of male rats previously suckled in litters with 9 and 15 pups (respectively, L9 and L15 condition). Naïve (Nv) = no treatment; Vehicle (V) = scopolamine methyl nitrate 1 mg/kg/day + saline; Taurine (T) = 1 mg/kg/day scopolamine methyl nitrate + 300 mg/kg/day of taurine; Pilocarpine (P) = 1 mg/kg/day scopolamine methyl nitrate + 45 mg/kg/day of pilocarpine; Taurine + pilocarpine (T + P) = 1 mg/kg/day scopolamine methyl nitrate + 300 mg/kg/day of taurine + 45 mg/kg/day of pilocarpine. All drugs were dissolved in 0.9% saline. Administration occurred from postnatal days 35 to 55. Data are mean ± standard deviation. ∗p < 0.001 compared with the corresponding L9 condition. #p < 0.001 compared with the control groups in the same lactation condition (ANOVA plus Holm–Sidak test). In panels (B,C), the numbers in each bar indicate the sample size of each measurement.
For the brain weight data (Figure 2B), ANOVA showed a main effect of the lactation condition on brain weight [F(1,41) = 51.659, p < 0.001]. L15 animals presented with a lower brain weight compared to the respective L9 groups. The average weight reduction was 17.03% and was independent of the treatment.
Blood Glucose Level
In the L15 condition, the Nv, V, and T groups displayed significantly lower glycemia than the corresponding L9 groups [F(1,77) = 33.484; p < 0.001]. Treatment with P and T + P reduced blood glucose levels in the L9, but not in the L15 groups [F(4,77) = 4.122; p = 0.004] compared with the corresponding Nv, V, and T groups. Data on glycemia are illustrated in Figure 2C.
Behavioral Activity in the Elevated Plus-Maze
The effect of administration of taurine and/or pilocarpine on behavioral activity in the EPM test is shown in Figure 3. Regarding the time spent in the open arms, ANOVA indicated a main effect of treatment [F(4,76) = 10.653, p < 0.001]. In the L9 condition, the Holm–Sidak test revealed that the group treated with taurine remained longer in the open arms compared to the other groups in the same lactation condition. In the L15 condition, the taurine-treated rats remained significantly longer in the EPM open arms, compared to the Nv, V and P groups, but not to the T + P groups.
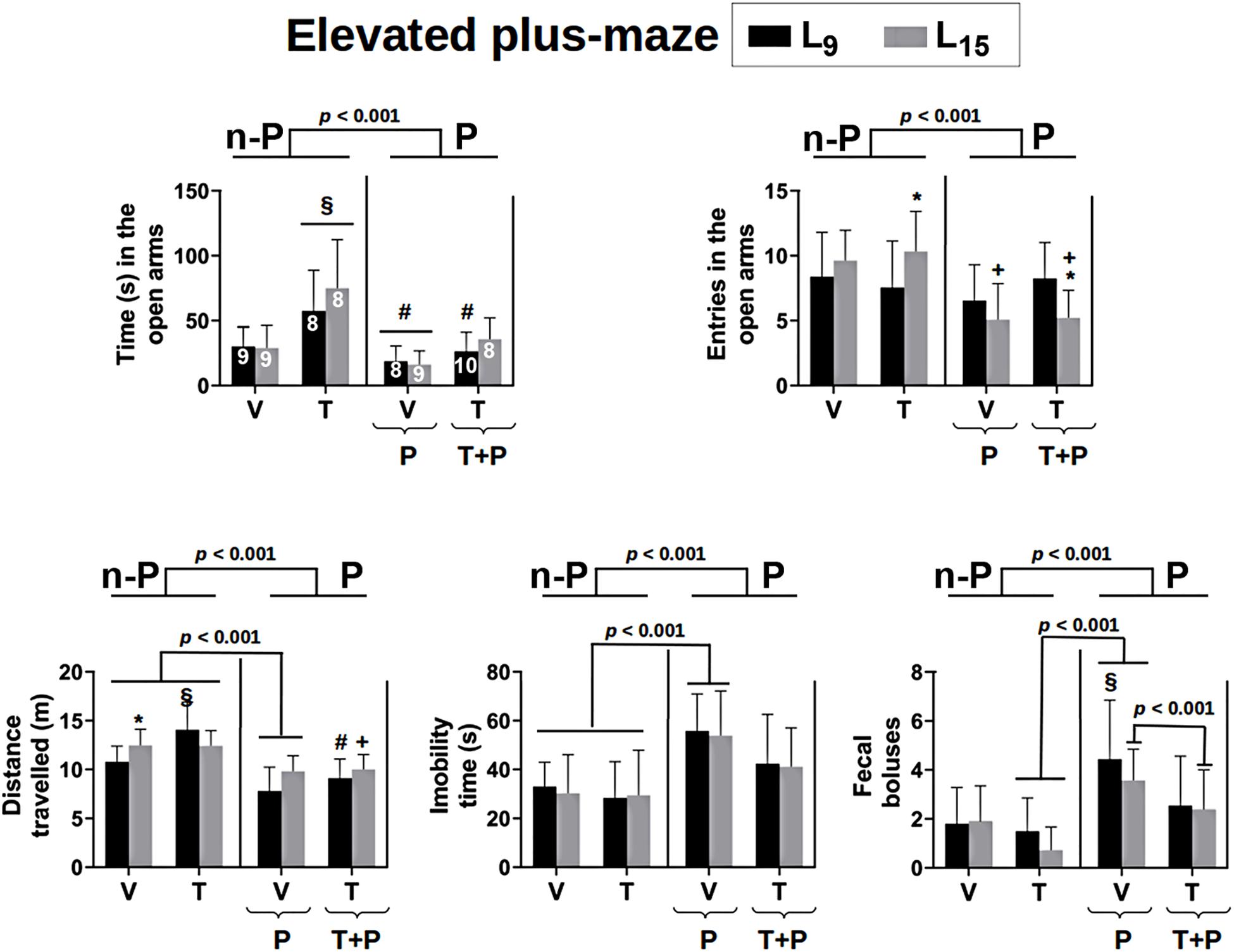
Figure 3. Behavioral activity in the elevated plus-maze test of 56-day-old male rats previously suckled in litters with 9 and 15 pups (respectively, L9 and L15 condition). Group description is as in Figure 2. n-P = no pilocarpine; P = pilocarpine; T = taurine; V = vehicle. Data are mean ± standard deviation. ∗p < 0.001 compared to the corresponding L9 condition. § p < 0.001 in comparison to groups Nv and V in the same lactation condition. #p < 0.001 compared to group T of the same lactation condition. +p < 0.001 compared to groups V and T of the same lactation condition (ANOVA plus Holm–Sidak test). The number in each bar of the upper-left graphic indicates the sample size, which applies to all graphics.
Regarding the number of entries into the open arms, ANOVA revealed a main effect of treatment [F(4,81) = 6.259; p < 0.001] in the L15 condition only; the Holm–Sidak test indicated that the groups P and T + P entered less frequently into the open arms, compared to the Nv, V, and T groups. Statistical analysis also detected interactions between the treatment and lactation condition [F(4,81) = 3.339, p = 0.014], revealing that the group T-L15 entered the open arms a higher number of times and the group T + P-L15 entered a lower number of times when compared to their respective L9 groups.
In relation to the total distance traveled by the animals in the EPM, ANOVA identified a main effect of the lactation condition [F(1,80) = 5.445, p = 0.022], treatment [F(4,80) = 19.010, p < 0.001] and an interaction between these two factors [F(4,80) = 2.697, p = 0.036]. The T-L9 group traveled a greater distance compared to the groups Nv, V, P, and T + P of the same lactation condition and the group P-L9, a smaller distance compared to Nv, V, and T-L9. In the L15 condition the groups P and T + P ran a smaller distance compared to the groups Nv, V, and T. The group Nv-L15 ran a greater distance when compared to the group Nv-L9.
Analysis of variance (ANOVA) identified a main effect of treatment for the total immobility of the animals [F(4,81) = 8.137; p < 0.001] and the Holm–Sidak test revealed that the P group had the longest immobility compared to the Nv, V, and T groups in both lactation conditions.
Regarding the number of fecal boluses expelled by each animal during the behavioral test, there was a main effect of treatment [F(4.81) = 6.498, p < 0.001] and the Holm–Sidak test revealed that the P-L9 group expelled a higher number of fecal boluses compared to the corresponding Nv, V, and T groups. In the L15 condition, the P group expelled a higher number of fecal boluses compared with the corresponding T and T + P groups.
CSD Parameters
CSD-Induced ECoG Potentiation
Examples of ECoG amplitude at recording points 1 (E1) and 2 (E2), before and after CSD, are shown in Figure 4A. In all groups, the paired t-test showed that for each animal the ECoG amplitude in the CSD period was significantly (p < 0.005) higher than in the baseline period (potentiation). This effect was observed for both recording points E1 and E2. The data on CSD-related ECoG potentiation are presented in Table 1.
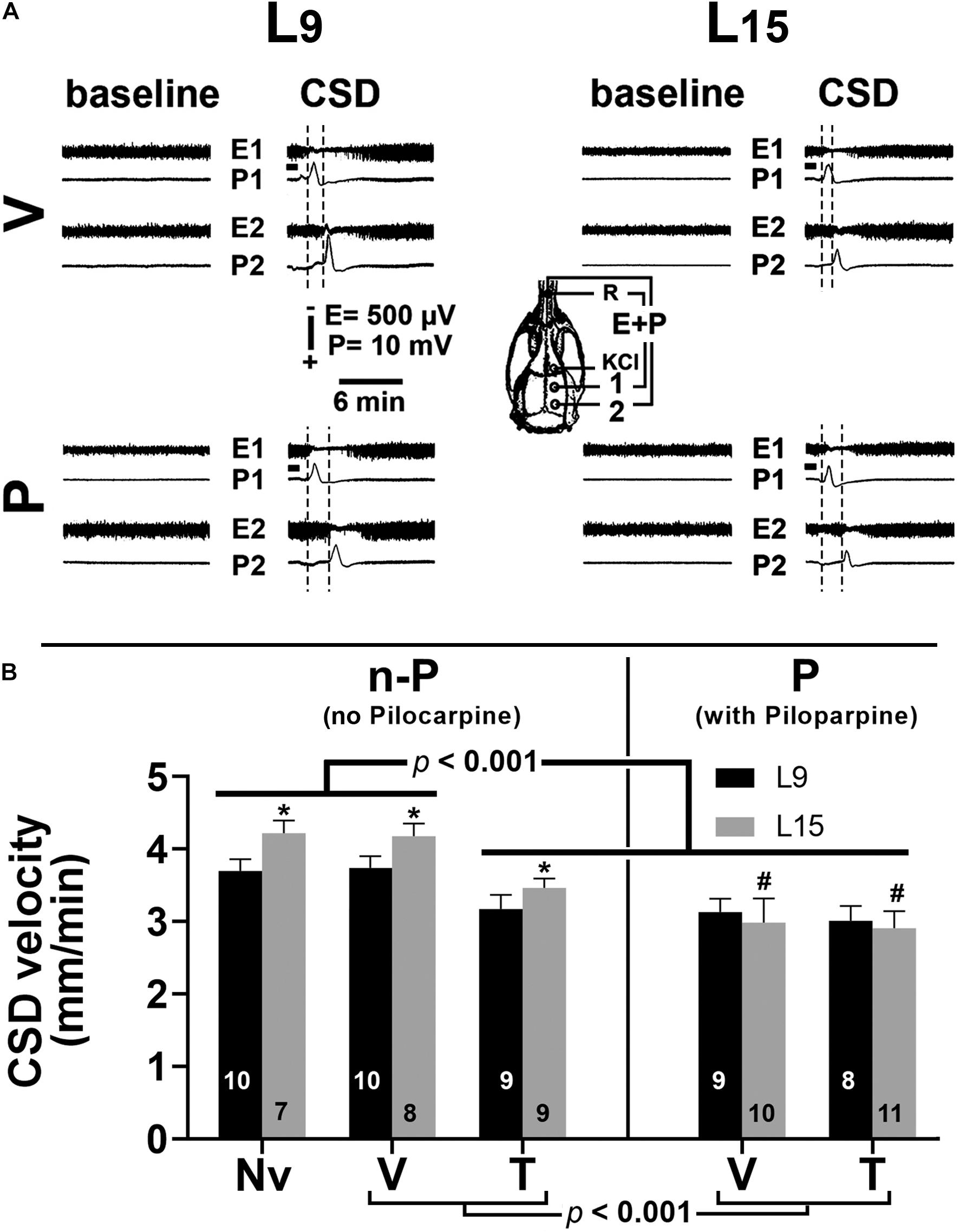
Figure 4. (A) Electrocorticogram (E) and slow potential change (P) on two points of the surface of the right hemisphere before (baseline period) and after the passage of cortical spreading depression (CSD period) in four 60–65-day-old male rats previously suckled in litters with 9 and 15 pups (respectively, L9 and L15 condition). Traces are from two vehicle and two pilocarpine-treated rats in the L9 (left) and L15 nutritional condition (right). Group description is as in Figure 2. The skull diagram shows the recording positions 1 and 2, from which the traces marked with the same numbers were obtained. The position of the common reference electrode (R) on the nasal bones and the application point of the CSD-eliciting stimulus (KCl) are also shown. CSD was elicited in the frontal cortex by chemical stimulation (a 1- to 2-mm diameter cotton ball soaked with 2% KCl) applied for 1 min on the intact dura mater, as indicated by the horizontal bars. ECoG amplitude in the CSD period is higher (potentiation), in comparison with the baseline amplitude for the same animal. (B) Cortical spreading depression velocity (mm/min) of 60–65-day-old male rats previously suckled in litters with 9 and 15 pups (respectively, L9 and L15 condition). Administration of vehicle, taurine and pilocarpine occurred from postnatal day 35 to 55. Data are mean ± standard deviation. ∗p < 0.001 compared with the corresponding L9 condition. #p < 0.001 compared to groups Nv (naïve), V (vehicle), and T (taurine) of the same lactation condition (ANOVA plus Holm–Sidak test). The number in each bar indicates the sample size.
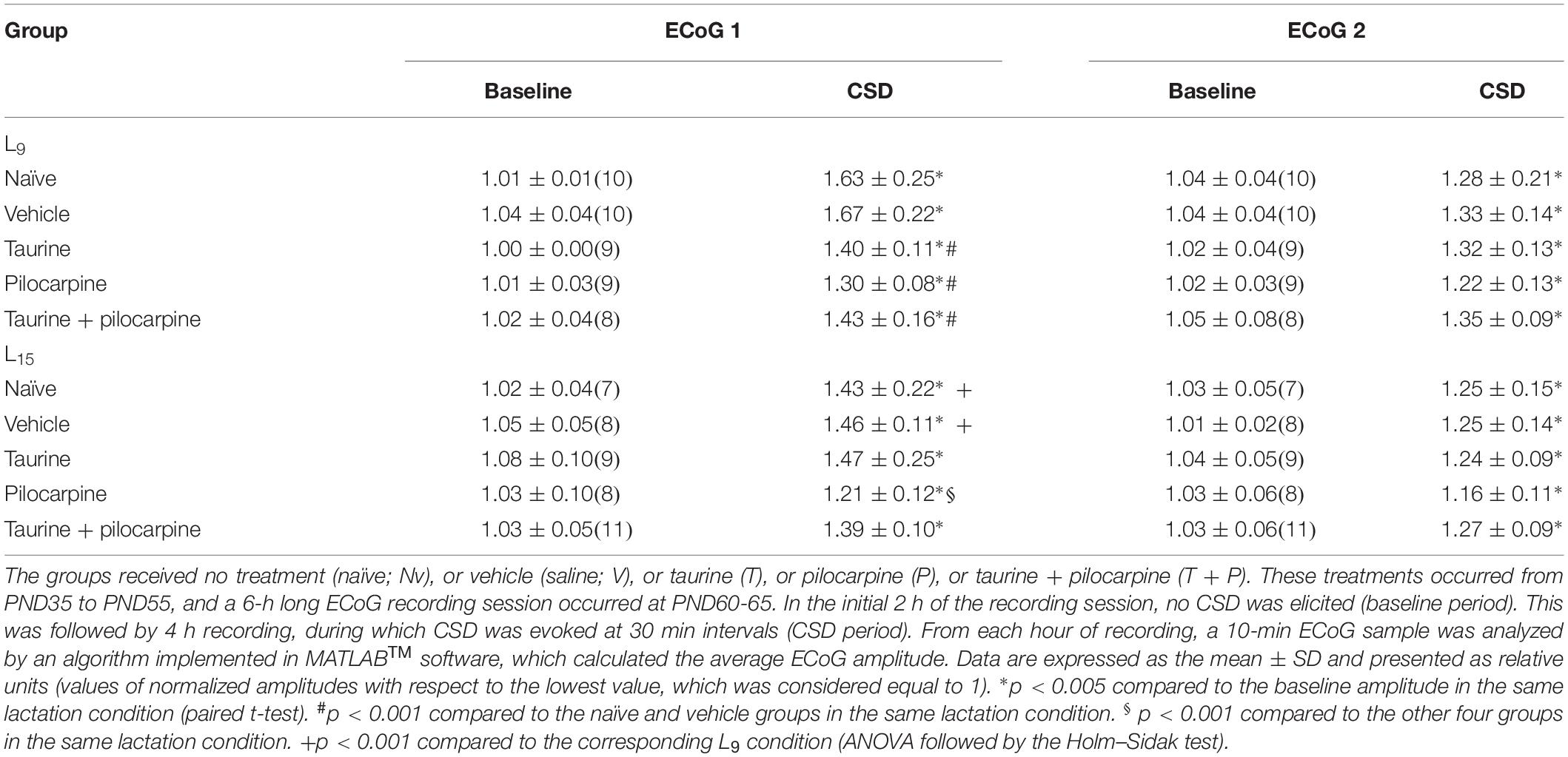
Table 1. Amplitude of ECoG, at the recording points 1 and 2, before (baseline period) and during the period of cortical spreading depression elicitation (CSD period), in rats previously suckled in litters with 9 and 15 pups (respectively, condition L9 and L15).
CSD Velocity of Propagation
In the L9 animals, CSD velocities (mean ± SD in mm/min) in the Nv, V, T, P, and T + P groups were respectively 3.71 ± 0.12, 3.73 ± 0.12, 3.15 ± 0.17, 3.12 ± 0.15, and 3.02 ± 0.20. In the L15 animals, the CSD velocities for the Nv, V, T, P, and T + P groups were respectively 4.20 ± 0.16, 4.17 ± 0.15, 3.40 ± 0.11, 3.04 ± 0.19, and 2.94 ± 0.22. ANOVA indicated a main effect of the lactation condition [F(1,81) = 34.876; p < 0.001], and post hoc (Holm–Sidak) test comparisons showed that the velocities were higher in the L15 groups compared to the L9 for the Nv, V, and T groups. ANOVA also detected a main effect of treatment [F(4,81) = 150.675; p < 0.001], and post hoc testing revealed that T, P, and T + P treatment significantly lowered the CSD propagation velocity compared with the corresponding Nv and V controls. The ANOVA revealed interactions between nutritional status and treatment, in which the L15 P and T + P groups had lower CSD propagation velocities compared to the Nv, V, and T groups [F(4,81) = 12.739, p < 0.001], and no difference was observed between the L9 and L15 animals treated with P and T + P. Data on the CSD propagation velocity are given in Figure 4B.
Amplitude and Duration of the CSD Negative Slow Potential Change
Table 2 shows data on the amplitude and duration of the negative slow potential change, which is the hallmark of CSD. ANOVA indicated a main effect of the lactation condition on the CSD amplitude [F(1,81) = 11.408; p < 0.001], and a post hoc (Holm–Sidak) test comparison showed that the amplitudes were higher in the naïve, vehicle, and taurine L15 groups compared to the corresponding L9 groups. The factor treatment also affected the amplitude [F(4,81) = 3.274; p = 0.015], and a post hoc test showed that the amplitude was lower in the pilocarpine-treated L15, but not in the L9 group, compared with the corresponding naïve, vehicle, and taurine of the same lactation condition. ANOVA also confirmed an interaction between both factors [F(4,81) = 2.934; p = 0.026].
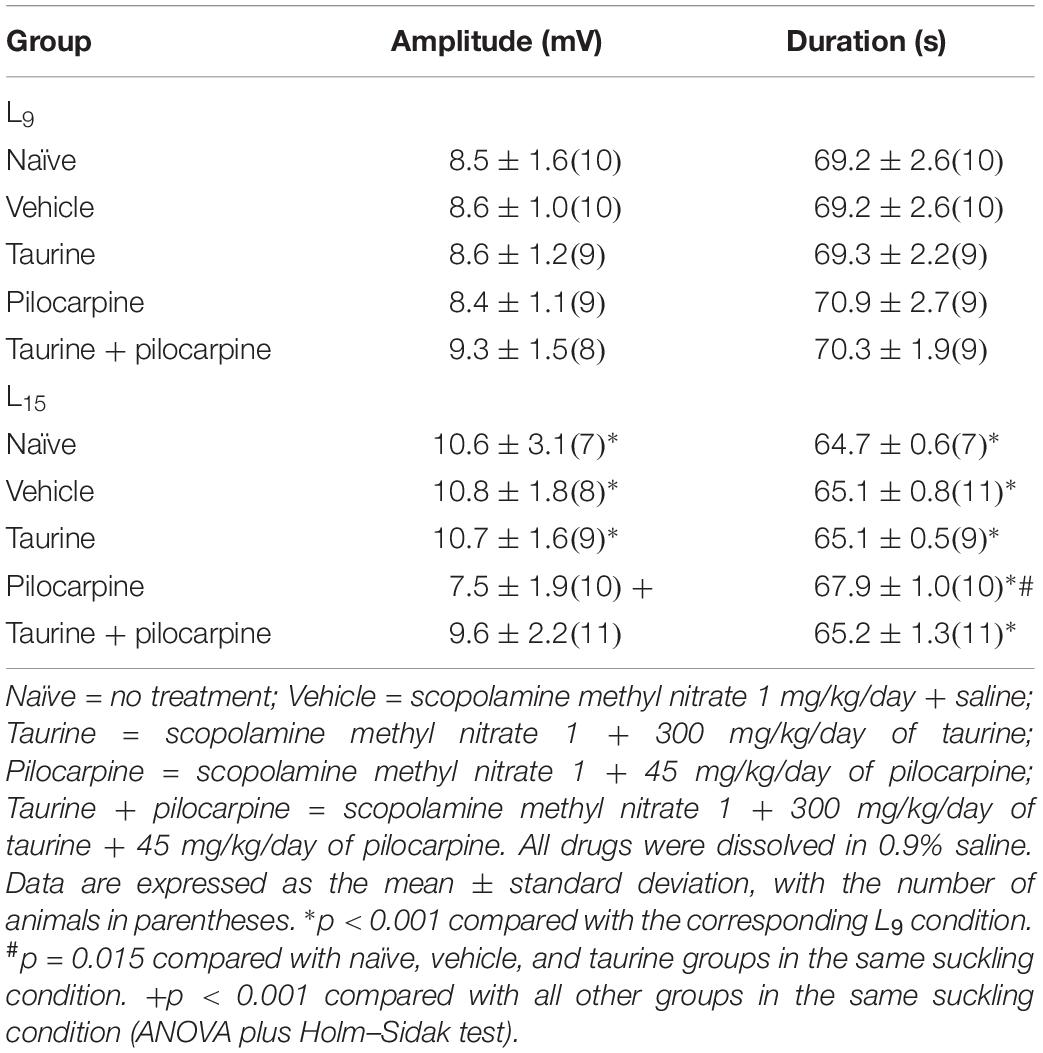
Table 2. Amplitude and duration of the negative slow potential change of CSD in male rats previously suckled in litters with 9 and 15 pups (respectively, L9 and L15 condition).
Analysis of CSD duration indicated a main effect of the lactation condition [F(1,81) = 115.827; p < 0.001] and treatment [F(4,81) = 5.291; p < 0.001]. The Holm–Sidak test indicated a shorter duration in the L15 groups compared with the corresponding L9 groups and a longer duration in the L15 pilocarpine-treated animals compared with the corresponding naïve, vehicle, taurine, and taurine + pilocarpine of the same lactation condition.
MDA Levels in the Cortex and Hippocampus
Measurements of MDA levels in the cerebral cortex and hippocampus are shown in Figure 5. ANOVA revealed no significant differences. MDA levels in the cortex in the L9 animals (mean ± SD in nmol/mg of protein) in the naïve, vehicle, taurine, pilocarpine, and taurine + pilocarpine groups were respectively 1.02 ± 0.23, 1.02 ± 0.30, 0.68 ± 0.20, 1.30 ± 0.26, and 0.97 ± 0.42. In the L15 animals, the MDA levels were respectively 1.27 ± 0.34, 1.27 ± 0.39, 1.07 ± 0.20, 1.63 ± 0.42, and 1.36 ± 0.39.
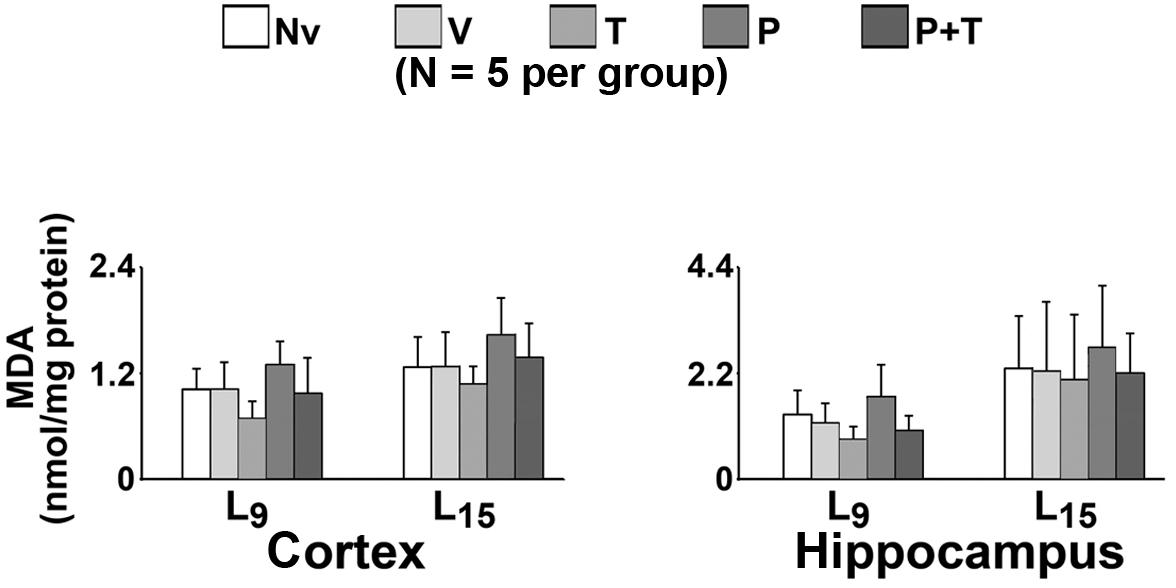
Figure 5. Malondialdehyde (MDA) levels (nmol/mg protein) of 60–65-day-old male rats previously suckled in litters with 9 and 15 pups (respectively, L9 and L15 condition). Group description as in Figure 2. Data are mean ± standard deviation of 5 rats per group. Measurements were performed in triplicate. No significant differences were observed.
Malondialdehyde levels in the hippocampus in the L9 animals (mean ± SD in nmol/mg of protein) in the naïve, vehicle, taurine, pilocarpine, and taurine + pilocarpine groups were respectively 1.34 ± 0.50, 1.16 ± 0.41, 0.84 ± 0.25, 1.72 ± 0.66 and 1.00 ± 0.31. In the L15 animals, the MDA levels were respectively 2.30 ± 1.08, 2.24 ± 1.44, 2.06 ± 1.36, 2.74 ± 1.26, and 2.21 ± 0.80.
Immunohistochemistry for Microglia and Astrocytes
The effect of administration of taurine and/or pilocarpine on the percentage of labeled area and immunoreactivity of astrocyte and microglia cells in the parietal cortex and CA1 hippocampus is shown in the Figure 6 for Iba1, and 7 for GFAP. All data are described as the mean ± standard deviation from four animals per group.
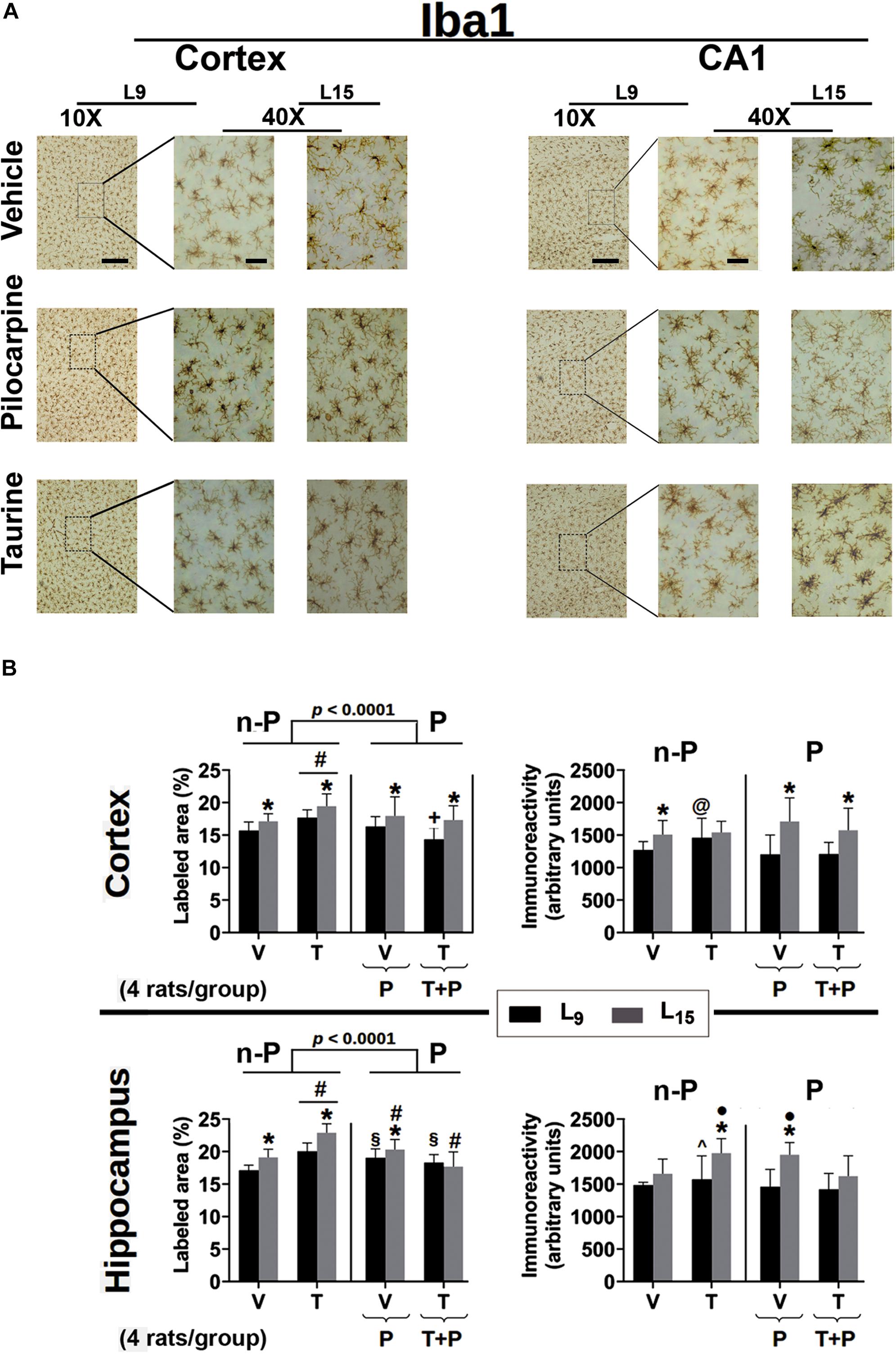
Figure 6. (A) Photomicrographs of immunolabeled Iba1-positive cells in the left cortex and hippocampus of 60–65-day-old male rats previously suckled in litters with 9 and 15 pups (respectively, L9 and L15 condition). Group description is as in Figure 2. Scale bars = 50 and 10 μm for the low (10×) and high magnification pictures (40×), respectively. (B) Percent labeled area (left panels) and immunoreactivity as arbitrary units (right panels) of immunolabeled Iba1-positive cells in the cortex (upper panels) and hippocampus (lower panels) of 60–65-day-old male rats previously suckled in litters with 9 and 15 pups (respectively, L9 and L15 condition). Group description is as in Figure 2. n-P = no pilocarpine; P = with pilocarpine. Data are mean ± standard deviation of four rats per group. ∗p < 0.001 compared to the corresponding L9 condition. § p < 0.001 compared to groups V in the same lactation condition. #p < 0.001 compared to the other three groups in the same lactation condition. +p < 0.001 compared to groups V and P of the same lactation condition. ∙p < 0.001 compared to groups V and T + P of the same lactation condition. @p < 0.001 compared to groups P and T + P of the same lactation condition. ∧p < 0.001 compared to group Nv of the same lactation condition (ANOVA followed by the Holm–Sidak test).
Iba-1 Immunohistochemistry
Data from Iba1 immunohistochemistry are presented in Figure 6. In the cerebral cortex, ANOVA showed in all groups a main effect of the lactation condition on the percentage of Iba1-labeled area [F(1,371) = 5.074; p < 0.001] and post hoc (Holm–Sidak) test comparisons showed that the animals of the L15 condition had a greater percentage of labeled area when compared to the corresponding L9 groups. ANOVA also detected a main effect of treatment [F(4,371) = 23.681; p < 0.001], and post hoc testing revealed in the two lactation conditions that taurine treatment significantly increased the percentage of labeled area, compared to the other four treatment groups. On the other hand, in the L9 condition, the taurine + pilocarpine treated group presented with a lower percentage of the labeled area when compared with the naïve, vehicle, and pilocarpine-treated groups. Regarding the Iba1 immunoreactivity in the cerebral cortex, ANOVA indicated a main effect of the lactation condition [F(1,355) = 59.454; p < 0.001] and post hoc testing comparisons showed that the animals of the L15 condition had greater immunoreactivity when compared to the corresponding L9 condition, except for the taurine-treated groups. ANOVA also revealed interactions between nutritional status and treatment, in which the pilocarpine and taurine + pilocarpine groups in the L15, but not in the L9 condition had higher immunoreactivity compared to the other groups [F(4,355) = 5.954; p < 0.001].
In the hippocampus, ANOVA revealed a main effect of the lactation condition on the percentage of the Iba-1 labeled area [F(1,376) = 64.536; p < 0.001]; the post hoc (Holm–Sidak) test comparisons showed that the groups naïve, vehicle, taurine, and pilocarpine in the L15 condition had a greater percentage of labeled area when compared to the corresponding L9 groups. ANOVA revealed a main effect of treatment [F(4,376) = 65.946; p < 0.001], and post hoc testing detected that in the two lactation conditions taurine treatment significantly increased the percentage of Iba1 labeled area compared to the other four groups.
Furthermore, in the L9 condition, pilocarpine and taurine + pilocarpine treatments resulted in a higher percentage of labeled area when compared with the L9 naïve and vehicle. In the L15 condition, the pilocarpine group displayed a percentage of labeled area that was greater than in the naïve, vehicle and taurine + pilocarpine groups, but was lower than in the taurine group; the L15 taurine + pilocarpine group had the lowest percentage of Iba1-labeled area. ANOVA also revealed interactions between nutritional status and treatment [F(4,376) = 11.742; p < 0.001], in which the L15 taurine group had a higher percentage of Iba1 labeled cortical area compared to the other four groups.
Regarding the Iba-1 immunoreactivity in the hippocampus, ANOVA indicated a main effect of the lactation condition [F(1,350) = 59.217; p < 0.001] and post hoc testing comparisons showed that the animals of the groups naïve, taurine, and pilocarpine in the L15 condition had greater immunoreactivity when compared to the corresponding L9 groups. ANOVA revealed a main effect of taurine treatment [F(4,350) = 14.626; p < 0.001], and post hoc testing showed in the L9 condition a significantly greater immunoreactivity of the taurine-treated animals when compared to the corresponding naïve group. Among the L15 animals, the taurine- and pilocarpine-treated groups had higher Iba1 immunoreactivity compared with the naïve, vehicle, and taurine + pilocarpine groups. ANOVA also identified interactions between nutritional status and treatment, in which the L15 taurine and pilocarpine groups had higher immunoreactivity compared to the other L15 groups [F(4,350) = 4.545; p < 0.001].
GFAP Immunohistochemistry
Data on GFAP immunohistochemistry are shown in Figure 7. ANOVA showed a main effect of the lactation condition on the percentage of GFAP-labeled areas in the cerebral cortex [F(1,434) = 63.276; p < 0.001] and post hoc (Holm–Sidak) test comparisons showed that the naïve, vehicle, and pilocarpine-treated groups of the L15 condition had a lower percentage of labeled area when compared to the corresponding L9 groups. ANOVA also indicated a main effect of treatment [F(4,434) = 6.988; p < 0.001], and post hoc testing revealed that, in the L9 condition, taurine + pilocarpine treatment significantly lowered the percentage of labeled area compared to the naïve, vehicle and pilocarpine groups, and in the L15 condition, taurine treatment resulted in a lower percentage of labeled area when compared with the other four groups in the L15 condition. ANOVA also revealed interactions between nutritional status and treatment [F(4,434) = 5.744; p < 0.001], in which the L15 taurine group had a lower percentage of labeled area in comparison with all other L15 groups.
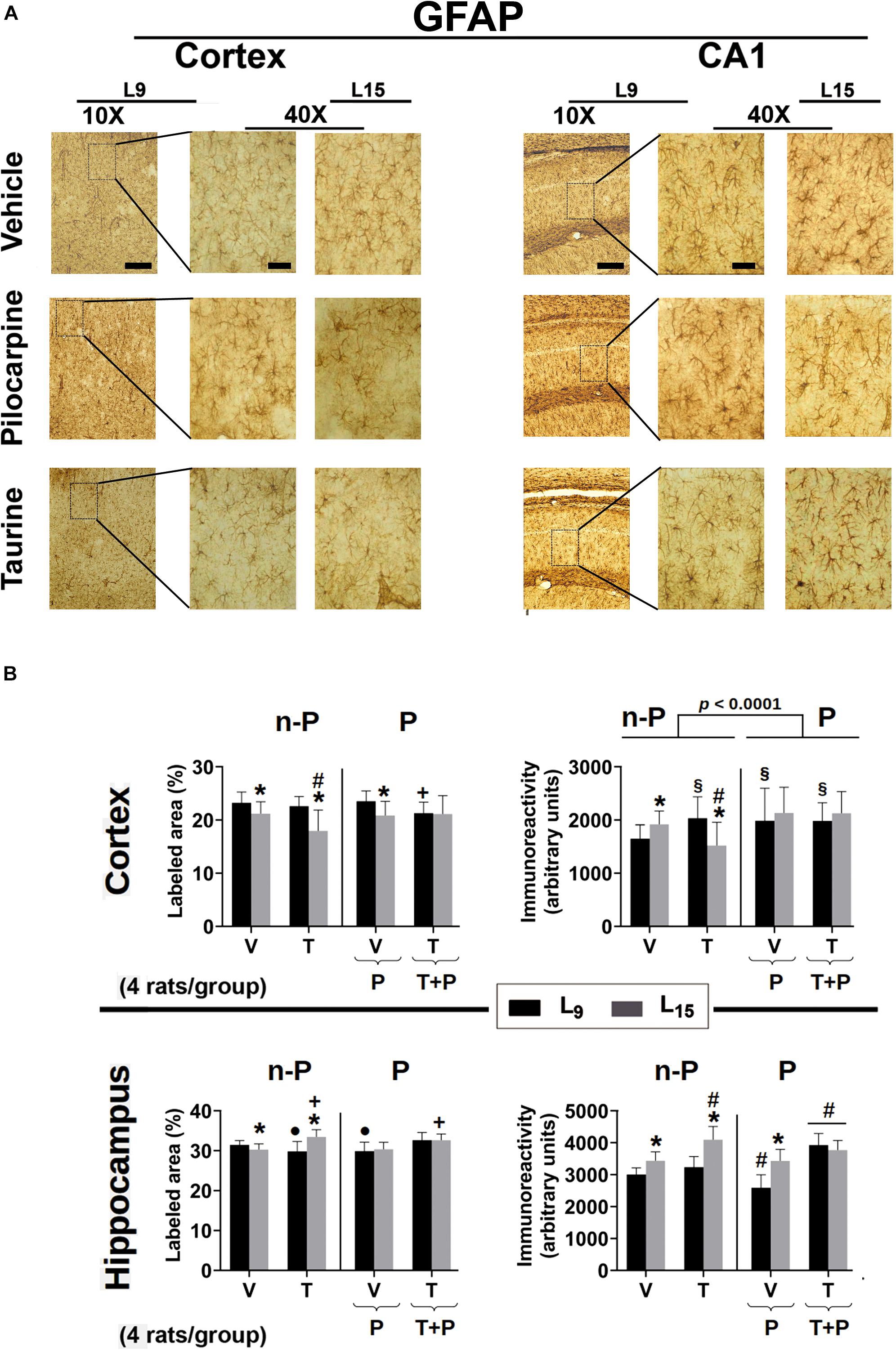
Figure 7. (A) Photomicrographs of immunolabeled GFAP-positive cells in the left cortex and hippocampus of 60–65-day-old male rats previously suckled in litters with 9 and 15 pups (respectively, L9 and L15 condition). Group description is as in Figure 2. Scale bars = 50 and 10 μm for the low (10×) and high magnification pictures (40×), respectively. (B) Percent labeled area (left panels) and immunoreactivity as arbitrary units (right panels) of immunolabeled GFAP-positive cells in the cortex (upper panels) and hippocampus (lower panels) of 60–65-day-old male rats previously suckled in litters with 9 and 15 pups (respectively, L9 and L15 condition). Group description is as in Figure 2. n-P = no pilocarpine; P = with pilocarpine. Data are mean ± standard deviation of four rats per group. ∗p < 0.001 compared to the corresponding L9 condition. § p < 0.001 in comparison to group V in the same lactation condition. #p < 0.001 compared to the other three groups in the same lactation condition. +p < 0.001 compared to groups V and P of the same lactation condition. ∙p < 0.001 compared to groups V and T + P of the same lactation condition (ANOVA followed by the Holm–Sidak test).
In relation to GFAP immunoreactivity quantification in the cerebral cortex, in the L9 condition ANOVA revealed a main effect of treatment [F(4,434) = 6.147; p < 0.001], and post hoc testing showed greater immunoreactivity in the groups treated with taurine, pilocarpine, and taurine + pilocarpine when compared to the naïve and vehicle-treated controls. Among the L15 groups, taurine animals presented with lower immunoreactivity compared to the other four groups. ANOVA also demonstrated interactions between nutritional status and treatment, in which the L15 taurine group had lower immunoreactivity compared to the corresponding naïve, vehicle groups, and with the taurine L9 group [F(4,434) = 10.551; p < 0.001].
Regarding GFAP-labeled cells in the hippocampus, in the L9 condition ANOVA showed a main effect of treatment [F(4,401) = 14.819; p < 0.001], and post hoc testing revealed that taurine and pilocarpine treatments significantly lowered the percentage of labeled area compared to the naïve, vehicle, and taurine + pilocarpine groups. In the L15 condition, taurine and taurine + pilocarpine treatments resulted in a higher percentage of the labeled area when compared with the naïve, vehicle, and pilocarpine groups. ANOVA also revealed interactions between nutritional status and treatment [F(4,401) = 15.918; p < 0.001], in which the taurine treatment resulted in a greater percentage of labeled area in the L15 but not in the L9 condition. Regarding the GFAP immunoreactivity in the hippocampus, ANOVA detected a main effect of the lactation condition [F(1,359) = 107.184; p < 0.001], and the post hoc testing comparisons showed that the groups naïve, vehicle, taurine, and pilocarpine in the L15 condition had greater immunoreactivity when compared to the corresponding L9 groups. The ANOVA detected a main effect of treatment [F(4,359) = 53.366; p < 0.001], and post hoc testing showed a significantly lower immunoreactivity in the L9 pilocarpine group compared to the other L9 groups. In the L15 condition, taurine animals presented with greater immunoreactivity compared to the other four L15 groups. In both lactation conditions, taurine + pilocarpine treatment resulted in greater immunoreactivity compared to the other nutrition-matched treatment groups. ANOVA also detected interactions between nutritional status and treatment, in which the L15 taurine group had greater immunoreactivity compared to the corresponding L9 group [F(4,359) = 21.074; p < 0.001].
Discussion
The present study extends the previous observations of our group on the effects of taurine (Francisco and Guedes, 2015) and pilocarpine (Mendes-da-Silva et al., 2018) in rats of a younger age (PND7-27) than our rats (PND35-55). Analyzing such effects at another age might be of importance, regarding the time course of drug actions (Guedes and Abadie-Guedes, 2019). Treatment with taurine and pilocarpine resulted in anxiolytic-like and anxiogenic behavior, respectively. In addition, chronic pilocarpine, which was administered on a subconvulsing basis, reduced body weight and glycemia; both taurine and pilocarpine treatment decelerated CSD propagation. Furthermore, these data extend our knowledge about the actions of taurine and pilocarpine on electrophysiological responses and glial cell reactions, as demonstrated by the CSD-related potentiation of ECoG amplitude and microglial and astrocyte immunoreactivity. The previously described in vivo CSD-induced ECoG potentiation (Lopes-de-Morais et al., 2014; Mendes-da-Silva et al., 2018) has been confirmed in this paper, and its modulation by pilocarpine and taurine has been described (Table 1). Data on anxiety-like behavior, MDA measurements and brain immunohistochemical effects that were produced by taurine and/or pilocarpine treatment represent novel evidence of the action of these compounds on 35–55-day-old rats, whereas data on weight gain, glycemia, and CSD confirm the results of a previous study (Francisco and Guedes, 2018). The stress that is associated with drug administration cannot be the cause of the reported alterations because – as first mentioned in the results section – the groups that received gavage and intraperitoneal injection of vehicle (saline and scopolamine) presented values similar to the naïve controls that received no gavage or intraperitoneal injection. The results collectively emphasize the effectiveness of taurine and pilocarpine treatment in modulating behavioral and electrophysiological parameters of the brain, as well as glial cell reactivity.
The ponderal reduction of body and brain weights in the animals under the L15 lactation condition (Figure 2) are consistent with our previous data (Francisco and Guedes, 2015, 2018), confirming the effectiveness of increasing litter size in producing malnutrition during the suckling period (Rocha-de-Melo et al., 2006). In early life, the brain is more vulnerable to environmental challenges, such as unfavorable lactation conditions (Morgane et al., 1978; Rocha-de-Melo et al., 2006). As previously pointed out, the evidence indicates that a body weight reduction implies weight diminution in the brain and other organs, which is usually associated with alterations in the organs’ function (Morgane et al., 2002). In animal models, nutritional deficiency early in life can permanently (or at least in a long-lasting manner) reduce the number of synapses, diminish myelin production, decrease the size and/or number of brain cells, and alter neurotransmitter systems (Morgane et al., 2002). As previously suggested (Francisco and Guedes, 2015), alterations in these processes may interfere with behavioral, electrophysiological and immunohistochemical parameters such as those presently investigated.
Experimental alteration of the blood glucose levels has been shown to correlate inversely with the brain’s ability to produce and propagate CSD (Ximenes-da-Silva and Guedes, 1991; Hoffmann et al., 2013), and the present data are in line with the literature. Our interpretation is that an adequate glucose supply is crucial in providing the energy necessary for the glial mechanisms that counteract CSD propagation, as CSD is an energy-demanding phenomenon (Back et al., 1994; Dohmen et al., 2008).
Elevated plus-maze test is one of the most commonly used behavioral tests to evaluate aspects of anxiety-like reactions in rodents (Ramos, 2008). Our findings indicated that treatment with pilocarpine and taurine were associated with anxiogenic and anxiolytic behavior, respectively, as judged by the frequency and duration of the animals’ visiting the open arms of the EPM (Figure 3). Our EPM data on taurine and pilocarpine administration are consistent with previous reports that showed an anxiolytic effect of taurine administration on animals tested in the EPM (Kong et al., 2006; Murakami and Furuse, 2010; Francisco and Guedes, 2015), and pilocarpine-associated anxiogenic behavior in rats tested in the EPM (Castelhano et al., 2015) and open field apparatus (Francisco and Guedes, 2018). Therefore, we think that it is possible that taurine’s action in the brain counteracts anxiety, as suggested by others (Murakami and Furuse, 2010; Yu et al., 2015). By the same logic, chronic pilocarpine at a subconvulsing dose seems to be anxiogenic (present data). Considering that the pilocarpine dose in this study (45 mg/kg/day) was very low (corresponding to 12–15% of the convulsing dose), we suggest that pilocarpine-induced anxiogenesis does not necessarily require the generation of convulsive episodes, as previously indicated (Duarte et al., 2014).
Nutrient intake in insufficient quantity and/or quality in early life can negatively modify structural, biochemical, and electrophysiological parameters of the brain and these effects may last into adult life (Morgane et al., 1978; Guedes, 2011). In rats, it is well-established that early-in-life malnutrition accelerates CSD propagation, both when malnutrition was induced by maternal diet manipulation (Mendes-da-Silva et al., 2014, 2018) and in an unfavorable lactation condition (Lima et al., 2017; Francisco and Guedes, 2018; present study). The mechanisms by which malnutrition facilitates CSD propagation are still a matter of debate. While a larger volume of brain extracellular space hinders CSD elicitation and propagation (Mazel et al., 2002), malnutrition in early life may increase cell-packing density and reduce the extracellular space in the brain (Morgane et al., 1978, 2002), which may facilitate CSD propagation (Lima et al., 2017). Furthermore, malnutrition reduces brain myelination (Morgane et al., 1978). It is important to note that CSD propagation velocity is increased in the myelin-deficient cortex and decreased in hypermyelinated knock-in animals (Merkler et al., 2009). In addition, early malnutrition may induce impairment of glial function (Morgane et al., 1978), and this condition has been shown to accelerate CSD (Largo et al., 1997). Malnutrition can also enhance the brain levels of the enzyme glutamic acid decarboxylase (Díaz-Cintra et al., 2007) and reduce brain glutamate uptake (Feoli et al., 2006), constituting a scenario that is favorable to CSD elicitation and propagation (Marrannes et al., 1988).
Regarding the redox balance in the brain, previous data suggested that CSD can induce oxidative stress (Viggiano et al., 2011; Shatillo et al., 2013). In the other direction, the accumulation of reactive oxygen species in the nervous system can trigger CSD (Netto and Martins-Ferreira, 1989; El-Bachá et al., 1998; Malkov et al., 2014). Interestingly, various antioxidant agents have been shown to counteract CSD (Abadie-Guedes et al., 2012, 2016; Lopes-de-Morais et al., 2014). As referred above (see introduction), taurine exhibits antioxidant properties and plays a neuroprotective role in epilepsy (Junyent et al., 2011). Because taurine is able to cross the blood–brain barrier, its level in the brain is increased when taurine is administered systemically (Menzie et al., 2013). Taken together, these pieces of evidence could explain the decelerating action of taurine on CSD, as taurine is considered a molecule with antioxidant properties (El-Maraghi et al., 2018; Jafri et al., 2019; Ommati et al., 2019). When administered to pregnant rat dams, taurine is capable of attenuating the impact of maternal food restriction on the progeny (Wang et al., 2017). Our data reinforce a recent suggestion that, in an excitability imbalance condition, taurine plays an inhibitory and neuroprotective role (Hrnčić et al., 2018). As a molecular structure that is very similar to the neurotransmitter GABA, taurine affects the opening of chloride channels, preferably by interactions with GABAA receptors and with lower affinity to the glycine and GABAB receptors in the adult brain (Oja and Saransaari, 2013; Hrnčić et al., 2018). Interestingly, extracellular chloride imbalance has been shown to affect CSD propagation in vitro in the isolated retina (Martins-Ferreira et al., 1974) and in vivo in the rabbit cortex (Guedes and Do Carmo, 1980).
Pilocarpine-induced seizures are correlated with elevated production of reactive oxygen species and by cerebral amino acid level changes, with increased glutamate content and decreased taurine levels in the rat hippocampus (Santos et al., 2011). On the other hand, chronic treatment with a subconvulsive dose of pilocarpine (45 mg/kg/day) did not increase MDA levels in the brain tissue (Mendes-da-Silva et al., 2018), suggesting that under those conditions oxidative stress does not increase, and the present data reinforces this suggestion. Taken together, the findings support the hypothesis that, after pilocarpine administration, brain levels of MDA increase only if pilocarpine induces convulsive seizures (Hamed and Abdellah, 2004; Freitas et al., 2010; Carmona-Aparicio et al., 2016; McElroy et al., 2017).
Microglial cells have the ability to react in response to CNS demands, such as those in epileptic disorders (Eyo et al., 2017) and nutritional imbalance (Rideau Batista Novais et al., 2016). In this study, unfavorable suckling (in large litters) increased microglia activation in the cortex and hippocampus, in addition to the behavioral (anxiety) and electrophysiological (CSD) effects, corroborating the findings of previous studies (Viana et al., 2013; Lima et al., 2017). Furthermore, in line with taurine’s effects on traumatic brain injury (Su et al., 2014), our taurine treatment decreased glial immunoreactivity in the cortex and hippocampus. Interestingly, both the cortex and hippocampus are pathologically affected regions in human temporal lobe epilepsy (Gonçalves Pereira et al., 2005; Das et al., 2009). In the pathogenesis of this disease, astrocyte and microglial cell activation is an important link (Clasadonte et al., 2016; Zhao et al., 2018).
Microglia cells may be activated, not merely by delayed neuronal degeneration, but by neuronal hyperactivity that precedes neuronal demise (Eyo et al., 2017). These cells are the resident immune cells of the CNS. They continuously survey the microenvironment with their highly dynamic processes, thus being able to become activated in response to tissue requirements associated with deleterious conditions, such as those involved in brain inflammatory and behavioral responses (Viana et al., 2013). It is interesting to note that Iba1 immunolabeling also labels macrophages, which may infiltrate the CNS; therefore, it is not a specific marker for microglia, and macrophages may become involved in inflammatory responses in the brain, as well as in behavioral responses (Wohleb et al., 2015).
Similar to microglia, astrocyte activation occurs following nutrition imbalance and seizures, and plays an important role in epileptogenesis. A recent study showed that early in life nutritional imbalance can have a lasting impact on astrocytes. It seems that protein deprivation decreased GFAP expression, whereas other undernutrition models (e.g., large litter size) increased GFAP expression (Abbink et al., 2019). In the temporal lobe epilepsy, hippocampal astrocytes undergo dramatic morphological and molecular changes, similar to the glial morphological changes in the hippocampus seen in the pilocarpine-induced epilepsy model (Shapiro et al., 2008). These hippocampal changes occur during the early latent stage of the pilocarpine model. Regarding the cortical astrocytes however, it has been suggested that molecular changes could be a consequence of the repetitive occurrence of seizures (Clasadonte et al., 2016).
In our study, in the L15 condition taurine increased GFAP immunoreactivity compared to the L15 control groups. It appears that this effect was not harmful to the animals, since in this group the behavioral and electrophysiological parameters were not impaired. Increased immunoreactivity for GFAP has been observed in healthy animals that were subjected to an enriched environment and treated with taurine, suggesting an interaction between these two factors (Rahmeier et al., 2016). Behaviorally, taurine supplementation in rats can improve stress-induced cognitive dysfunction (Jia et al., 2016). Surprisingly, we found lower immunoreactivity for GFAP in the hippocampus of L9 pilocarpine-treated rats. Borges et al. (2006) reported transient loss of astrocytes in mice dentate hilus early after pilocarpine-induced status epilepticus. In rats, they observed about 50% reduction in hilar neurons but no change in astrocyte number (Borges et al., 2006). Another study reported that a sub-threshold dose of pilocarpine (15 mg/kg), following a lithium chloride administration (127 mg/kg), increases GFAP immunoreactivity in the hippocampus (Sun et al., 2016). In view of the controversial reports, we think that further research is necessary for the complete understanding of the effect of non-convulsing dose of pilocarpine on astrocytes.
Conclusion
The results of this study suggest a brain effect of taurine in pilocarpine-treated rats, which is expressed in body weight, glycemia, behavioral (anxiety), electrophysiological parameters related with CSD and glial alterations. For some of these parameters, the data suggest taurine/pilocarpine/malnutrition interactions, whose mechanisms deserve further exploration.
Data Availability
All datasets generated for this study are included in the manuscript and/or the supplementary files.
Ethics Statement
This study was carried out in accordance with the recommendations of the Ethics Committee for Animal Research of the Federal University of Pernambuco, Brazil. The protocol was approved by the same committee (approval protocol no. 23076.015655/2015-99).
Author Contributions
EF and RG conceived the study, performed the experiments, analyzed the data, and wrote the manuscript. RM conducted the biochemical analysis and helped in manuscript revision. GS and CC conducted the immunohistochemical analysis, and helped in manuscript revision and in preparing some illustrations.
Funding
Funding for this project was provided by the Fundação de Amparo à Ciência e Tecnologia de Pernambuco (FACEPE-IBPG-0424-4.05/14), Conselho Nacional de Desenvolvimento Científico e Tecnológico (CNPq no. 303636/2014-9), MCT/FINEP/CT-INFRA – PROINFRA – 01/2008, Instituto Nacional de Ciência e Tecnologia em Excitotoxicidade e Neuroproteção’ – Edital INCT/MCT/CNPq, and Capes (Finance Code 001; Edital 043/2013 Ciências Do Mar II and BEX 2036/15-0).
Conflict of Interest Statement
The authors declare that the research was conducted in the absence of any commercial or financial relationships that could be construed as a potential conflict of interest.
Acknowledgments
The authors thank Professor Belmira L. S. Andrade-da-Costa for helpful advise in the preparation of the Figure 1 and her kind gift of anti-Neu-N antibody is very much appreciated.
References
Abadie-Guedes, R., Bezerra, R. S., and Guedes, R. C. A. (2016). Alpha-tocopherol counteracts the effect of ethanol on cortical spreading depression in rats of various ages, with and without ethanol abstinence. Alcohol Clin. Exp. Res. 40, 728–733. doi: 10.1111/acer.12998
Abadie-Guedes, R., Guedes, R. C. A., and Bezerra, R. S. (2012). The impairing effect of acute ethanol on spreading depression is antagonized by astaxanthin in rats of 2 young-adult ages. Alcohol Clin. Exp. Res. 36, 1563–1567. doi: 10.1111/j.1530-0277.2012.01766.x
Abbink, M. R., van Deijk, A. F., Heine, V. M., Verheijen, M. H., and Korosi, A. (2019). The involvement of astrocytes in early-life adversity induced programming of the brain. Glia 67, 1637–1653. doi: 10.1002/glia.23625
Arisi, G. M., Foresti, M. L., Katki, K., and Shapiro, L. A. (2015). Increased CCL2, CCL3, CCL5, and IL-1β cytokine concentration in piriform cortex, hippocampus, and neocortex after pilocarpine-induced seizures. J. Neuroinflamm. 12:129. doi: 10.1186/s12974-015-0347-z
Ayata, C., and Lauritzen, M. (2015). Spreading depression, spreading depolarizations, and the cerebral vasculature. Physiol. Rev. 95, 953–993. doi: 10.1152/physrev.00027.2014
Back, T., Kohno, K., and Hossmann, K. A. (1994). Cortical negative DC deflections following middle cerebral artery occlusion and KCl-induced spreading depression: effect on blood flow, tissue oxygenation, and electroencephalogram. J. Cereb. Blood Flow Metab. 14, 12–19. doi: 10.1038/jcbfm.1994.3
Barbeau, A., Inoue, N., Tsukada, Y., and Butterworth, R. F. (1975). The neuropharmacology of taurine. Life Sci. 17, 669–677.
Borges, K., McDermott, D., Irier, H., Smith, Y., and Dingledine, R. (2006). Degeneration and proliferation of astrocytes in the mouse dentate gyrus after pilocarpine-induced status epilepticus. Exp. Neurol. 2006, 416–427. doi: 10.1016/j.expneurol.2006.04.031
Carmona-Aparicio, L., Zavala-Tecuapetla, C., González-Trujano, M. E., Sampieri, A. I., Montesinos-Correa, H., Granados-Rojas, L., et al. (2016). Status epilepticus: using antioxidant agents as alternative therapies. Exp. Ther. Med. 12, 1957–1962. doi: 10.3892/etm.2016.3609
Castelhano, A. S. S., Ramos, F. O., Scorza, F. A., and Cysneiros, R. M. (2015). Early life seizures in female rats lead to anxiety-related behavior and abnormal social behavior characterized by reduced motivation to novelty and deficit in social discrimination. J. Neural Transm. 122, 349–355. doi: 10.1007/s00702-014-1291-2
Chen, X. C., Pan, Z. L., Liu, D. S., and Han, X. (1998). Effect of taurine on human fetal neuron cells: proliferation and differentiation. Adv. Exp. Med. Biol. 442, 397–403. doi: 10.1007/978-1-4899-0117-0_49
Chepkova, A. N., Sergeeva, O. A., and Haas, H. L. (2006). Taurine rescues hippocampal long-term potentiation from ammonia-induced impairment. Neurobiol. Dis. 23, 512–521. doi: 10.1016/j.nbd.2006.04.006
Clasadonte, J., Morel, L., Barrios-Camacho, C. M., Chiang, M. S., Zhang, J., Iyer, L., et al. (2016). Molecular analysis of acute and chronic reactive astrocytes in the pilocarpine model of temporal lobe epilepsy. Neurobiol. Dis. 91, 315–325. doi: 10.1016/j.nbd.2016.03.024
Curia, G., Longo, D., Biagini, G., Jones, R. S. G., and Avoli, M. (2008). The pilocarpine model of temporal lobe epilepsy. J. Neurosci. Methods 172, 143–157. doi: 10.1016/j.jneumeth.2008.04.019
Das, S. R., Mechanic-Hamilton, D., Korczykowski, M., Pluta, J., Glynn, S., Avants, B. B., et al. (2009). Structure specific analysis of the hippocampus in temporal lobe epilepsy. Hippocampus 19, 517–525. doi: 10.1002/hipo.20620
Díaz-Cintra, S., González-Maciel, A., Morales, M. A., Aguilar, A., Cintra, L., and Prado-Alcalá, R. A. (2007). Protein malnutrition differentially alters the number of glutamic acid decarboxylase-67 interneurons in dentate gyrus and CA1–3 subfields of the dorsal hippocampus. Exp. Neurol. 208, 47–53. doi: 10.1016/j.expneurol.2007.07.003
Dohmen, C., Sakowitz, O. W., Fabricius, M., Bosche, B., Reithmeier, T., Ernestus, R., et al. (2008). Spreading depolarizations occur in human ischemic stroke with high incidence. Ann. Neurol. 63, 720–728. doi: 10.1002/ana.21390
Dreier, J. P. (2011). The role of spreading depression, spreading depolarization and spreading ischemia in neurological disease. Nat. Med. 17, 439–447. doi: 10.1038/nm.2333
Dreier, J. P., Major, S., Pannek, H.-W., Woitzik, J., Scheel, M., Wiesenthal, D., et al. (2012). Spreading convulsions, spreading depolarization and epileptogenesis in human cerebral cortex. Brain 135, 259–275. doi: 10.1093/brain/awr303
Duarte, F. S., Hoeller, A. A., Duzzioni, M., Gavioli, E. C., Canteras, N. S., and De Lima, T. C. (2014). NK1 receptors antagonism of dorsal hippocampus counteract the anxiogenic-like effects induced by pilocarpine in non-convulsive Wistar rats. Behav. Brain Res. 265, 53–60. doi: 10.1016/j.bbr.2014.01.050
El-Bachá, R. S., De-Lima-Filho, J. L., and Guedes, R. C. A. (1998). Dietary antioxidant deficiency facilitates cortical spreading depression induced by photoactivated riboflavin. Nutr. Neurosci. 1, 205–212. doi: 10.1080/1028415X.1998.11747230
El-Maraghi, E. F., Abdel-Fattah, K. L., Soliman, S. M., and El-Sayed, W. M. (2018). Taurine provides a time-dependent amelioration of the brain damage induced by y-irradiation in rats. J. Hazard Mater 359, 40–46. doi: 10.1016/j.jhazmat.2018.07.005
Eyo, U. B., Murugan, M., and Wu, L. J. (2017). Microglia-neuron communication in epilepsy. Glia 65, 5–18. doi: 10.1002/glia.23006
Feoli, A. M., Siqueira, I. R., Almeida, L., Tramontina, A. C., Vanzella, C., Sbaraini, S., et al. (2006). Effects of protein malnutrition on oxidative status in rat brain. Nutrition 22, 160–165. doi: 10.1016/j.nut.2005.06.00
Footitt, D. R., and Newberry, N. R. (1998). Cortical spreading depression induces an LTP-like effect in rat neocortex in vitro. Brain Res. 781, 339–342. doi: 10.1016/S0006-8993(97)01359-0
Francisco, E. S., and Guedes, R. C. A. (2015). Neonatal taurine and alanine modulate anxiety-like behavior and decelerate cortical spreading depression in rats previously suckled under different litter sizes. Amino Acids 47, 2437–2445. doi: 10.1007/s00726-015-2036-8
Francisco, E. S., and Guedes, R. C. A. (2018). Sub-convulsing dose administration of pilocarpine reduces glycemia, increases anxiety-like behavior and decelerates cortical spreading depression in rats suckled on various litter sizes. Front. Neurosci. 12:897. doi: 10.3389/fnins.2018.00897
Freitas, R. L., Santos, I. M., de Souza, S. G. F., Ada, R., Saldanha, G. B., da Freitas, R. M., et al. (2010). Oxidative stress in rat hippocampus caused by pilocarpine-induced seizures is reversed by buspirone. Brain Res. Bull. 81, 505–509. doi: 10.1016/j.brainresbull.2009.09.014
García-García, L., Shiha, A. A., Fernández de la Rosa, R., Delgado, M., Silván, Á, Bascuñana, P., et al. (2017). Metyrapone prevents brain damage induced by status epilepticus in the rat lithium-pilocarpine model. Neuropharmacology 123, 261–273. doi: 10.1016/j.neuropharm.2017.05.007
Glien, M., Brandt, C., Potschka, H., Voigt, H., Ebert, U., and Löscher, W. (2001). Repeated low dose treatment of rats with pilocarpine: low mortality but high proportion of rats developing epilepsy. Epilepsy Res. 46, 111–119. doi: 10.1016/s0920-1211(01)00272-8
Gonçalves Pereira, P. M., Insausti, R., Artacho-Perula, E., Salmenpera, T., Kalviainen, R., and Pitkanen, A. (2005). MR volumetric analysis of the piriform cortex and cortical amygdala in drug-refractory temporal lobe epilepsy. AJNR Am. J. Neuroradiol. 26, 319–332.
Grafstein, B. (1956). Mechanism of spreading cortical depression. J. Neurophysiol. 19, 154–171. doi: 10.1152/jn.1956.19.2.154
Guedes, R. C. A. (2011). “Cortical spreading depression: a model for studying brain consequences of malnutrition,” in Handbook of Behavior, Food and Nutrition, eds V. R. Preedy, R. R. Watson, and C. R. Martin (Berlin: Springer), 2343–2355. doi: 10.1007/978-0-387-92271-3_148
Guedes, R. C. A., and Abadie-Guedes, R. (2019). Brain aging and electrophysiological signaling: revisiting the spreading depression model. Front. Aging Neurosci. 11:136. doi: 10.3389/fnagi.2019.00136
Guedes, R. C. A., Araújo, M. G. R., Verçosa, T. C., Bion, F. M., Sá, A. L., and Pereira, A. Jr., et al. (2017). Evidence of an inverse correlation between serotonergic activity and spreading depression propagation in the rat cortex. Brain Res. 1672, 29–34. doi: 10.1016/j.brainres.2017.07.011
Guedes, R. C. A., and Cavalheiro, E. A. (1997). Blockade of spreading depression in chronic epileptic rats: reversion by diazepam. Epilepsy Res. 27, 33–40. doi: 10.1016/s0920-1211(96)01017-0
Guedes, R. C. A., and Do Carmo, R. J. (1980). Influence of ionic alterations produced by gastric washing on cortical spreading depression. Exp. Brain Res. 39, 341–349. doi: 10.1007/bf00237123
Guedes, R. C. A., Tsurudome, K., and Matsumoto, N. (2005). Spreading depression in vivo potentiates electrically-driven responses in frog optic tectum. Brain Res. 1036, 109–114. doi: 10.1016/j.brainres.2004.12.033
Guedes, R. C. A., and Vasconcelos, C. A. C. (2008). Sleep-deprivation enhances in adult rats the antagonistic effects of pilocarpine on cortical spreading depression: a dose–response study. Neurosci. Lett. 442, 118–122. doi: 10.1016/j.neulet.2008.07.011
Hamed, S. A., and Abdellah, M. M. (2004). Trace elements and electrolytes homeostasis and their relation to antioxidant enzyme activity in brain hyperexcitability of epileptic patients. J. Pharmacol. Sci. 96, 349–359. doi: 10.1254/jphs.CRJ04004X
Hartings, J. A., Strong, A. J., Fabricius, M., Manning, A., Bhatia, R., Dreier, J. P., et al. (2009). Spreading depolarizations and late secondary insults after traumatic brain injury. J. Neurotrauma. 26, 1857–1866. doi: 10.1089/neu.2009.0961
Hertelendy, P., Varga, D. P., Menyhárt, A., Bari, F., and Farkas, E. (2018). Susceptibility of the cerebral cortex to spreading depolarization in neurological disease states: the impact of aging. Neurochem. Int. 127, 125–136. doi: 10.1016/j.neuint.2018
Hoffmann, U., Sukhotinsky, I., Eikermann-Haerter, K., and Ayata, C. (2013). Glucose modulation of spreading depression susceptibility. J. Cereb. Blood Flow Metab. 33, 191–195. doi: 10.1038/jcbfm.2012.132
Hrnčić, D., Rašić-Marković, A., Macut, D., Mladenović, D., Šušić, V., Djurić, D., et al. (2018). Sulfur-containing amino acids in seizures: current state of the art. Curr. Med. Chem. 25, 378–390. doi: 10.2174/0929867324666170609090613
Jafri, A. J. A., Agarwal, R., Iezhitsa, I., Agarwal, P., and Ismail, N. M. (2019). Taurine protects against NMDA-induced retinal damage by reducing retinal oxidative stress. Amino Acids 51, 641–646. doi: 10.1007/s00726-019-02696-4
Jia, N., Qinru Sunb, Q., Suc, Q., Dangc, S., and Chen, D. (2016). Taurine promotes cognitive function in prenatally stressed juvenile rats via activating the Akt-CREB-PGC1α pathway. Redox Biol. 10, 179–190. doi: 10.1016/j.redox.2016.10.004
Junyent, F., Lemos, D. L., Utrera, J., Paco, S., Aguado, F., Camins, A., et al. (2011). Content and trafic of taurine in hippocampal reactive astrocytes. Hippocampus 21, 185–197. doi: 10.1002/hipo.20739
Kong, W. X., Chen, S. W., Li, Y. L., Zhang, Y. J., Wang, R., Min, L., et al. (2006). Effects of taurine on rat behaviors in three anxiety models. Pharmacol. Biochem. Behav. 83, 271–276. doi: 10.1016/j.pbb.2006.02.007
Largo, C., Ibarz, J. M., and Herreras, O. (1997). Effects of the gliotoxin fluorocitrate on spreading depression and glial membrane potential in rat brain in situ. J. Neurophysiol. 78, 295–307. doi: 10.1152/jn.1997.78.1.295
Lauritzen, M. (1994). Pathophysiology of the migraine aura: the spreading depression theory. Brain 117, 199–210. doi: 10.1093/brain/117.1.199
Lima, D. S. C., Francisco, E. S., Lima, C. B., and Guedes, R. C. A. (2017). Neonatal L-glutamine modulates anxiety-like behavior, cortical spreading depression, and microglial immunoreactivity: analysis in developing rats suckled on normal size-and large size litters. Amino Acids 49, 337–346. doi: 10.1007/s00726-016-2365-2
Lopes-de-Morais, A. A. C., Mendes-da-Silva, R. F., Santos, E. M., and Guedes, R. C. A. (2014). Neonatal dexamethasone accelerates spreading depression in the rat, and antioxidant vitamins counteract this effect. Brain Res. 1591, 93–101. doi: 10.1016/j.brainres.2014.09.075
Malkov, A., Ivanov, A. I., Popova, I., Mukhtarov, M., Gubkina, O., Waseem, T., et al. (2014). Reactive oxygen species initiate a metabolic collapse in hippocampal slices: potential trigger of cortical spreading depression. J. Cereb. Blood Flow Metab. 34, 1540–1549. doi: 10.1038/jcbfm.2014.121
Marcinkiewicz, J., and Kontny, E. (2014). Taurine and inflammatory diseases. Amino Acids 46, 7–20. doi: 10.1007/s00726-012-1361-4
Marrannes, R., Willems, R., De Prins, E., and Wauquier, A. (1988). Evidence for a role of the N-methyl-d-aspartate (n.d.) receptor in cortical spreading depression in the rat. Brain Res. 457, 226–240. doi: 10.1016/0006-8993(88)90690-7
Martins-Ferreira, H., Castro, G. O., Struchiner, C. J., and Rodrigues, P. S. (1974). Circling spreading depression in isolated chick retina. J. Neurophysiol. 37, 773–784. doi: 10.1152/jn.1974.37.4.773
Mayevsky, A., Meilin, S., Manor, T., Ornstein, E., Zarchin, N., and Sonn, J. (1998). Multiparametric monitoring of brain oxygen balance under experimental and clinical conditions. Neurol. Res. 20(Suppl. 1), S76–S80.
Mazel, T., Richter, F., Vargová, L., and Syková, E. (2002). Changes in extracellular space volume and geometry induced by cortical spreading depression in immature and adult rats. Physiol. Res. 51, 85–93.
McElroy, P. B., Liang, L. P., Day, B. J., and Patel, M. (2017). Scavenging reactive oxygen species inhibits status epilepticus-induced neuroinflammation. Exp. Neurol. 298, 13–22. doi: 10.1016/j.expneurol.2017.08.009
Mendes-da-Silva, R. F., Lopes-de-Morais, A. A. C., Bandim-da-Silva, M. E., Cavalcanti, G. D. A., Rodrigues, A. R. O., Andrade-da-Costa, B. L. S., et al. (2014). Prooxidant versus antioxidant brain action of ascorbic acid in well-nourished and malnourished rats as a function of dose: a cortical spreading depression and malondialdehyde analysis. Neuropharmacology 86, 155–160. doi: 10.1016/j.neuropharm.2014.06.027
Mendes-da-Silva, R. S., Francisco, E. S., and Guedes, R. C. A. (2018). Pilocarpine/ascorbic acid interaction in the immature brain: electrophysiological and oxidative effects in well-nourished and malnourished rats. Brain Res. Bull. 142, 414–421. doi: 10.1016/j.brainresbull.2018.09.008
Menzie, J., Prentice, H., and Wu, J. Y. (2013). Neuroprotective mechanisms of taurine against ischemic stroke. Brain Sci. 3, 877–907. doi: 10.3390/brainsci3020877
Merkler, D., Klinker, F., Jürgens, T., Glaser, R., Paulus, W., Brinkmann, B. G., et al. (2009). Propagation of spreading depression inversely correlates with cortical myelin content. Ann. Neurol. 66, 355–365. doi: 10.1002/ana.21746
Morgane, P. J., Miller, M., Kemper, T., Stern, W., Forbes, W., Hall, R., et al. (1978). The effects of protein malnutrition on the developing nervous system in the rat. Neurosci. Biobehav. Rev. 2, 137–230. doi: 10.1016/0149-7634(78)90059-3
Morgane, P. J., Mokler, D. J., and Galler, J. R. (2002). Effects of prenatal protein malnutrition on the hippocampal formation. Neurosci. Biobehav. Rev. 26, 471–483. doi: 10.1016/s0149-7634(02)00012-x
Murakami, T., and Furuse, M. (2010). The impact of taurine-and beta alanine-supplemented diets on behavioral and neurochemical parameters in mice: antidepressant versus anxiolytic-like effects. Amino Acids 39, 427–434. doi: 10.1007/s00726-009-0458-x
Netto, M., and Martins-Ferreira, H. (1989). Elicitation of spreading depression by rose Bengal photodynamic action. Photochem. Photobiol. 50, 229–234. doi: 10.1111/j.1751-1097.1989.tb04153.x
Ohkawa, H., Ohishi, N., and Yagi, K. (1979). Assay for lipid peroxides in animal tissues by thiobarbituric acid reaction. Anal. Biochem. 95, 351–358. doi: 10.1016/0003-2697(79)90738-3
Oja, S. S., and Saransaari, P. (2013). Taurine and epilepsy. Epilepsy Res. 104, 187–194. doi: 10.1016/j.eplepsyres.2013.01.010
Oliveira, M. W., Minotto, J. B., de-Oliveira, M. R., Zanotto-Filho, A., Behr, G. A., Rocha, R. F., et al. (2010). Scavenging and antioxidant potential of physiological taurine concentrations against different reactive oxygen/nitrogen species. Pharmacol. Rep. 62, 185–193. doi: 10.1016/s1734-1140(10)70256-5
Ommati, M. M., Heidari, R., Ghanbarinejad, V., Abdoli, N., and Niknahad, H. (2019). Taurine treatment provides neuroprotection in a mouse model of manganism. Biol. Trace. Elem. Res. 190, 384–395. doi: 10.1007/s12011-018-1552-2
Peixinho-Pena, L. F., Fernandes, J., Almeida, A. A., Novaes-Gomes, F. G., Cassilhas, R., Venancio, D. P., et al. (2012). A strength exercise program in rats with epilepsy is protective against seizures. Epilepsy. Behav. 25, 323–328. doi: 10.1016/j.yebeh.2012.08.011
Pietrobon, D., and Moskowitz, A. (2014). Chaos and commotion in the wake of cortical spreading depression and spreading depolarizations. Nat. Rev. Neurosci. 15, 379. doi: 10.1038/nrn3770
Pusic, A. D., Mitchell, H. M., Kunkler, P. E., Klauer, N., and Kraig, R. P. (2015). Spreading depression transiently disrupts myelin via interferon-gamma signaling. Exp. Neurol. 264, 43–54. doi: 10.1016/j.expneurol.2014.12.001
Racine, R. J. (1972). Modification of seizure activity by electrical stimulation. II: motor seizure. Electroencephalogr. Clin. Neurophysiol. 32, 281–294. doi: 10.1016/0013-4694(72)90177-0
Rahmeier, F. L., Zavalhia, L. S., Tortorelli, L. S., Huf, F., Géa, L. P., Meurer, R. T., et al. (2016). The effect of taurine and enriched environment on behaviour, memory and hippocampus of diabetic rats. Neurosci. Lett. 630, 84–92. doi: 10.1016/j.neulet.2016.07.032
Ramos, A. (2008). Animal models of anxiety: do i need multiple tests? Trends Pharmacol. Sci. 29, 493–498. doi: 10.1016/j.tips.2008.07.005
Rideau Batista Novais, A., Pham, H., Van de Looij, Y., Bernal, M., Mairesse, J., Zana-Taieb, E., et al. (2016). Transcriptomic regulations in oligodendroglial and microglial cells related to brain damage following fetal growth restriction. Glia. 64, 2306–2320. doi: 10.1002/glia.23079
Ripps, H., and Shen, W. (2012). Review: taurine: a “very essential” amino acid. Mol. Vis. 18, 2673–2686.
Rocha-de-Melo, A. P., Cavalcanti, J. B., Barros, A. S., and Guedes, R. C. A. (2006). Manipulation of rat litter size during suckling influences cortical spreading depression after weaning and at adulthood. Nutr. Neurosci. 9, 155–160. doi: 10.1080/10284150600903602
Rossi, A. R., Angelo, M. F., Villarreal, A., Lukin, J., and Ramos, A. J. (2013). Gabapentin administration reduces reactive gliosis and neurodegeneration after pilocarpine-induced status epilepticus. PLoS One 8:e78516. doi: 10.1371/journal.pone.0078516
Santos, N. F., Arida, R. M., Filho, E. M., Priel, M. R., and Cavalheiro, E. A. (2000). Epileptogenesis in immature rats following recurrent status epilepticus. Brain Res. Brain Res. Rev. 32, 269–276. doi: 10.1016/s0165-0173(99)00089-2
Santos, P. S., Campêlo, L. M., Freitas, R. L., Feitosa, C. M., Saldanha, G. B., and Freitas, R. M. (2011). Lipoic acid effects on glutamate and taurine concentrations in rat hippocampus after pilocarpine-induced seizures. Arq. Neuropsiquiatr. 69, 360–364. doi: 10.1590/s0004-282x2011000300018
Santos-Silva, J. C., Ribeiro, R. A., Vettorazzi, J. F., Irles, E., Rickli, S., Borck, P. C., et al. (2015). Taurine supplementation ameliorates glucose homeostasis, prevents insulin and glucagon hypersecretion, and controls β, α, and δ-cell masses in genetic obese mice. Amino Acids 47, 1533–1548. doi: 10.1007/s00726-015-1988-z
Shapiro, L. A., Wang, L., and Ribak, C. E. (2008). Rapid astrocyte and microglial activation following pilocarpine-induced seizures in rats. Epilepsia 49, 33–41. doi: 10.1111/j.1528-1167.2008.01491.x
Shatillo, A., Koroleva, K., Giniatullina, R., Naumenko, N., Slastnikova, A. A., Aliev, R. R., et al. (2013). Cortical spreading depression induces oxidative stress in the trigeminal nociceptive system. Neuroscience 253, 341–349. doi: 10.1016/j.neuroscience.2013.09.002
Souza, T. K. M., Silva-Gondim, M. B., Rodrigues, M. C., and Guedes, R. C. A. (2015). Anesthetic agents modulate ECoG potentiation after spreading depression, and insulin-induced hypoglycemia does not modify this effect. Neurosci. Lett. 592, 6–11. doi: 10.1016/j.neulet.2015.02.018
Strong, A. J., Fabricius, M., Boutelle, M. G., Hibbins, S. J., Hopwood, S. E., Jones, R., et al. (2002). Spreading and synchronous depressions of cortical activity in acutely injured human brain. Stroke 33, 2738–2743. doi: 10.1161/01.str.0000043073.69602.09
Su, Y., Fan, W., Ma, Z., Wen, X., Wang, W., Wu, Q., et al. (2014). Taurine improves functional and histological outcomes and reduces inflammation in traumatic brain injury. Neuroscience 266, 56–65. doi: 10.1016/j.neuroscience.2014.02.006
Sun, H. L., Deng, D. P., Pan, X. H., Wang, C. Y., Zhang, X. L., Chen, X. M., et al. (2016). A sub-threshold dose of pilocarpine increases glutamine synthetase in reactive astrocytes and enhances the progression of amygdaloid-kindling epilepsy in rats. Neuroreport 27, 213–219. doi: 10.1097/WNR.0000000000000511
Turski, L., Ikonomidou, C., Turski, W. A., Bortolotto, Z. A., and Cavalheiro, E. A. (1989). Review: cholinergic mechanisms and epileptogenesis. the seizures induced by pilocarpine: a novel experimental model of intractable epilepsy. Synapse 3, 154–171. doi: 10.1002/syn.890030207
Turski, W. A., Cavalheiro, E. A., Schwarz, M., Czuczwar, S. J., Kleinrok, Z., and Turski, L. (1983a). Limbic seizures produced by pilocarpine in rats: behavioural, electroencephalographic and neuropathological study. Behav. Brain Res. 9, 315–335. doi: 10.1016/0166-4328(83)90136-5
Turski, W. A., Czuczwar, S. J., Kleinrok, Z., and Turski, L. (1983b). Cholinomimetics produce seizures and brain damage in rats. Experientia 39, 1408–1411. doi: 10.1007/bf01990130
Van Harreveld, A. (1959). Compounds in brain extracts causing spreading depression of cerebral cor- tical activity and contraction of crustacean muscle. J. Neurochem. 3, 300–315. doi: 10.1111/j.1471-4159.1959.tb12636.x
Viana, L. C., Lima, C. M., Oliveira, M. A., Borges, R. P., Cardoso, T. T., Almeida, I. N., et al. (2013). Litter size, age-related memory impairments, and microglial changes in rat dentate gyrus: stereological analysis and three dimensional morphometry. Neuroscience 238, 280–296. doi: 10.1016/j.neuroscience.2013.02.019
Viggiano, A., Viggiano, E., Valentino, I., Monda, M., Viggiano, A., and De Luca, B. (2011). Cortical spreading depression affects reactive oxygen species production. Brain Res. 1368, 11–18. doi: 10.1016/j.brainres.2010.10.062
Vinogradova, L. V., Vinogradov, V. Y., and Kuznetsova, G. D. (2006). Unilateral cortical spreading depression is an early marker of audiogenic kindling in awake rats. Epilepsy Res. 71, 64–75. doi: 10.1016/j.eplepsyres.2006.05.014
Wang, Y., Li, X., Liu, J., and Fu, W. (2017). Antenatal taurine supplementation in fetal rats with growth restriction improves neural stem cell proliferation by inhibiting the activities of Rho family factors. J. Matern. Fetal Neonatal Med. 31, 1454–1461. doi: 10.1080/14767058.2017.1319353
Wohleb, E. S., McKim, D. B., Sheridan, J. F., and Godbout, J. P. (2015). Monocyte trafficking to the brain with stress and inflammation: a novel axis of immune-to-brain communication that influences mood and behavior. Front. Neurosci. 8:447. doi: 10.3389/fnins.2014.00447
Ximenes-da-Silva, A., and Guedes, R. C. A. (1991). Differential effect of changes in blood glucose levels on the velocity of propagation of cortical spreading depression in normal and malnourished rats. Braz. J. Med. Biol. Res. 24, 1277–1281.
Yakimova, K., Sann, H., Schimid, H. A., and Pierau, F. K. (1996). Effects of GABA agonists and antagonists on temperature-sensitive neurons in the rat hypothalamus. J. Physiol. 494, 217–230. doi: 10.1113/jphysiol.1996.sp021486
Yu, C., Mei, X. T., Zheng, Y. P., and Xu, D. H. (2015). Taurine zinc solid dispersions protect against cold-restraint stress-induced gastric ulceration by upregulating HSP70 and exerting an anxiolytic effect. Eur. J. Pharmacol. 762, 63–71. doi: 10.1016/j.ejphar.2015.05.033
Keywords: taurine, pilocarpine, anxiety-like behavior, blood glucose, brain excitability, glial cells, nutritional deficiency, spreading depression
Citation: Francisco EdS, Mendes-da-Silva RF, Castro CBLd, Soares GdSF and Guedes RCA (2019) Taurine/Pilocarpine Interaction in the Malnourished Rat Brain: A Behavioral, Electrophysiological, and Immunohistochemical Analysis. Front. Neurosci. 13:981. doi: 10.3389/fnins.2019.00981
Received: 13 February 2019; Accepted: 30 August 2019;
Published: 18 September 2019.
Edited by:
George E. Barreto, University of Limerick, IrelandReviewed by:
Eszter Farkas, University of Szeged, HungaryDaniele Lana, University of Florence, Italy
Copyright © 2019 Francisco, Mendes-da-Silva, Castro, Soares and Guedes. This is an open-access article distributed under the terms of the Creative Commons Attribution License (CC BY). The use, distribution or reproduction in other forums is permitted, provided the original author(s) and the copyright owner(s) are credited and that the original publication in this journal is cited, in accordance with accepted academic practice. No use, distribution or reproduction is permitted which does not comply with these terms.
*Correspondence: Rubem Carlos Araújo Guedes, Z3VlZGVzLnJjYUBnbWFpbC5jb20=; cmd1ZWRlc0B1ZnBlLmJy