- Institute of Biochemistry and Biophysics, Polish Academy of Sciences, Warsaw, Poland
The nematode Caenorhabditis elegans expresses the ten-1 gene that encodes teneurin. TEN-1 protein is expressed throughout the life of C. elegans. The loss of ten-1 function results in embryonic and larval lethality, highlighting its importance for fundamental processes during development. TEN-1 is expressed in the epidermis and neurons. Defects in neuronal pathfinding and epidermal closure are characteristic of ten-1 loss-of-function mutations. The molecular mechanisms of TEN-1 function in neurite outgrowth, neuronal pathfinding, and dendritic morphology in C. elegans are largely unknown. Its genetic redundancy with the extracellular matrix receptors integrin and dystroglycan and genetic interactions with several basement membrane components suggest a role for TEN-1 in the maintenance of basement membrane integrity, which is essential for neuronal guidance. Identification of the lat-1 gene in C. elegans, which encodes latrophilin, as an interaction partner of ten-1 provides further mechanistic insights into TEN-1 function in neuronal development. However, receptor-ligand interactions between LAT-1 and TEN-1 remain to be experimentally proven. The present review discusses the function of teneurin in C. elegans, with a focus on its involvement in the formation of receptor signaling complexes and neuronal networks.
Introduction
Teneurins are large single-pass transmembrane glycoproteins that are conserved in most animals with a nervous system (Tucker, 2018). Teneurins were first discovered in Drosophila (Baumgartner and Chiquet-Ehrismann, 1993; Baumgartner et al., 1994) and later described in Caenorhabditis elegans (Drabikowski et al., 2005) and vertebrate models (Minet et al., 1999). Teneurins are involved in several developmental processes in invertebrate models and expressed most prominently in developing neuronal tissues, contributing to neuronal patterning and axon guidance (Drabikowski et al., 2005; Tucker et al., 2007; Silva et al., 2011; Mosca et al., 2012; Mosca, 2015). The family of teneurin proteins is characterized by a distinct protein domain architecture. Their extracellular domain consists of eight epidermal growth factor (EGF)-like repeats, a region of conserved cysteines, and unique tyrosine and aspartate (YD)-repeats and is highly conserved among vertebrates and invertebrates. The structures of some extracellular domains of chicken Ten2 and mouse Ten3 were recently solved, revealing a previously unpredicted TTR transthyretin-related domain that plays roles in protein aggregation and lipid recognition in other teneurin-unrelated proteins (Jackson et al., 2018; Li et al., 2018). Unlike the extracellular domain, the composition of the intracellular domain of teneurin proteins, with exception of some predicted phosphorylation sites, is very different between vertebrates and invertebrates. The intracellular domain can be cleaved off and translocate to the nucleus, whereas the extracellular domain can be released into the extracellular milieu. The ability of the intracellular domain to mediate cellular signaling within the nucleus was first observed in a cell culture model of vertebrate teneurin-2, in which overexpressed variants of teneurin-2 colocalized with promyelocytic leukemia protein (PML) bodies (Bagutti et al., 2003). However, the intracellular domain of the endogenous teneurin protein was found in the nucleus only in C. elegans (Drabikowski et al., 2005). Some studies have described a nuclear function of the teneurin intracellular domain that regulates transcription as a transcriptional repressor or activator (Bagutti et al., 2003; Nunes et al., 2005; Scholer et al., 2015; Glendining et al., 2017). However, the mechanism by which the membrane-spanning full-length teneurin protein is released to the intracellular domain from the plasma membrane is mostly unknown. Furin-cleavage sites between the transmembrane domain and the EGF-like repeats were suggested to be one such processing (Tucker and Chiquet-Ehrismann, 2006; Kenzelmann et al., 2007). This was supported by experiments in which recombinant avian teneurin-2 protein was cleaved by furin protease (Rubin et al., 1999). Similar to the mechanism of processing, signals that trigger the release of the intracellular domain remain to be discovered. Efforts to identify binding partners of the extracellular domain revealed various interactions that contributed to deciphering teneurin function as an organizer of neuronal networks (Mosca, 2015). Vertebrate teneurins form homo- and heterophilic interactions (Feng et al., 2002; Rubin et al., 2002; Beckmann et al., 2013). In Drosophila, teneurins mediate synaptic connections and neuromuscular connections via homophilic interactions (Hong et al., 2012; Mosca et al., 2012). In hippocampal neurons, teneurin-2 acts as a postsynaptic receptor for latrophilin (Silva et al., 2011). However, the ways in which this interaction contributes to synapse formation are unknown. Moreover, teneurin-1 interacts with beta-dystroglycan, resulting in cytoskeletal rearrangements (Chand et al., 2012). Whether teneurin-1 is expressed post- or presynaptically remains unclear. In Drosophila, an interaction between ten-m and integrin in motor neurons and muscles was proposed to be important for normal synaptic function, but the mechanism by which this occurs is unclear (Mosca et al., 2012; Dani et al., 2014). Studies in C. elegans revealed a fundamental role for teneurin in tissue organization and neuronal network development and maintenance (Drabikowski et al., 2005; Trzebiatowska et al., 2008; Morck et al., 2010; Topf and Chiquet-Ehrismann, 2011; Promel et al., 2012).
The present mini review provides an overview of the various identified genetic interactions with the ten-1 gene in C. elegans, providing insights into its ancient function. We focus especially on ten-1-latrophilin connections, which are discussed within the context of recent findings in vertebrate models.
Ten-1 Expression and Loss-Of-Function Phenotype
Most species express several teneurin paralogs (Tucker et al., 2012). Genetic redundancy has impeded investigations of their biological functions because single deletions show minimal phenotypic alterations. In contrast, the C. elegans genome encodes only one teneurin gene, ten-1. This fact, combined with the tremendous genetic tractability of the model organism, makes C. elegans an attractive system to investigate the biological significance of teneurins. Expression of the ten-1 gene is under the control of two promoters that give rise to two transcript versions. These transcripts only differ in the length of the part that codes for the intracellular domain and thus were named short ten-1a and long ten-1b. These two forms of TEN-1 are expressed throughout worm development and in many tissues but have distinct expression patterns. An extensive analysis of TEN-1 expression was performed, in which green fluorescent protein (GFP) was expressed under two different promoters, pten-1a (which controls the expression of TEN-1L) and pten-1b (which controls the expression of TEN-1S). pten-1a was mostly active in the mesoderm, with prominent expression in muscles and the intestine, whereas pten-1b was active in the ectoderm, predominantly in neurons, including the soma and axons (Drabikowski et al., 2005). Using specific antibodies against the N-terminal part of TEN-1L, the intracellular domain was detected in the nucleus (Drabikowski et al., 2005). Expression of the ten-1 transgene that was fused to GFP under the control of pten-1b confirmed epidermal and neuronal expression patterns in the embryonic stage, indicating the potential involvement of TEN-1 in neuronal development (Topf and Chiquet-Ehrismann, 2011).
The depletion of TEN-1 by RNAi results in severe morphological defects. Worms that were injected with RNAi against both transcripts exhibited an increase in embryonic lethality, accompanied by gross defects in hypodermal cell migration. These findings supported the importance of TEN-1 during early worm development. Prominent post-embryonic defects included generally abnormal body morphology, morphological defects in the reproductive system, defects in muscles, and abnormalities in neuronal migration and axonal pathfinding (Drabikowski et al., 2005). A smaller brood size and morphological defects were confirmed in several TEN-1 mutants. Three mutant alleles of ten-1 have been characterized (ok641, tm651, et5; (Drabikowski et al., 2005; Trzebiatowska et al., 2008; Morck et al., 2010). Ten-1(ok641) and ten-1(tm651) are null alleles, and ten-1(et5) is a hypomorphic allele with a weaker post-embryonic phenotype. Neuronal defects in the TEN-1 mutants were not as penetrant as during RNAi depletion but were predominantly observed in mutant worms that exhibited other morphological defects, including epidermal defects (Drabikowski et al., 2005; Morck et al., 2010). Migration defects were observed in some neurons in otherwise healthy-looking animals, suggesting that TEN-1 function is specifically required for some neurons. However, the migration and pathfinding of neurons also strongly depend on an intact basement membrane. The basement membrane is a specialized extracellular matrix that surrounds most tissues in all Metazoa. TEN-1 is expressed in all major tissues in C. elegans and consists of a large extracellular part with several different structural domains, suggesting that it likely interacts with components of the extracellular milieu.
Genetic Interactions of Ten-1 in C. elegans
Several studies have identified multiple genetic interactions with ten-1 (Byrne et al., 2007; Trzebiatowska et al., 2008; Morck et al., 2010; Topf and Chiquet-Ehrismann, 2011; Promel et al., 2012). To date, however, none of these interaction partners have been shown biochemically to interact physically with TEN-1. A high-throughput screen identified glp-1, a receptor of the NOTCH family, as a ten-1 interacting partner (Byrne et al., 2007). Glp-1 is essential for the development of worm gonads as well as TEN-1, and the depletion of glp-1 together with ten-1 is embryonically lethal. Nevertheless, the functional basis of this interaction remains to be determined. Further attempts to investigate the function of ten-1 focused on interactions with genes that encode basement membrane receptors and components and genes that are involved in regulating the cytoskeleton, neuronal guidance, and axon outgrowth (Trzebiatowska et al., 2008; Morck et al., 2010; Topf and Chiquet-Ehrismann, 2011). Table 1 presents an overview on these genetic interactions with ten-1 (The reader is advised to see original publications for further details on the described phenotypes). Based on phenotypical observations of ten-1 mutant worms that showed the loss of basement membrane integrity, which surrounds the developing gonad in post-embryonic worms, Trzebiatowska et al. (2008) applied a candidate approach and found that ten-1 genetically interacted with the basement membrane receptor α-integrin and dystroglycan and basement membrane components lamin and nidogen (Trzebiatowska et al., 2008). Double mutants of all four genes together with ten-1 resulted in a synthetic lethal or sick phenotype that terminated the development of the double-mutant worms during embryogenesis or at an early larval stage. Previous studies in neuroblastoma cells found that the teneurin-2-dependent induction of filopodia formation was more prominent on lamin substrate (Rubin et al., 1999), and chicken teneurin-2 was shown to colocalize with lamin in basement membranes of the optic cup (Tucker et al., 2001). These findings in C. elegans suggested that teneurin is a receptor that might act redundantly with integrin or dystroglycan in basement membrane function. The ten-1 single mutants display pleiotropic phenotype and those seem likely to show genetic interactions with various genes. However, there is specificity of the interaction between ten-1 and basement membrane components. Mutations in cle-1 (CoLlagen with endostatin domain 1; vertebrate type XV/XVIII collagen homolog in C. elegans) or unc-52 (perlecan) did not enhance embryonic lethality, larval arrest, or sterility of the ten-1(ok641) mutant (Trzebiatowska et al., 2008). Loss-of-function phenotypes of nidogen (nid-1), dystroglycan (dgn-1), and integrin (ina-1) in worms involve defects in the nervous system (Baum and Garriga, 1997; Kang and Kramer, 2000; Kim and Wadsworth, 2000). However, Trzebiatowska et al. (2008) did not investigate neuronal defects in double-mutant worms. Such studies may be difficult because of the early death of such mutant animals. An unbiased genetic screen of ten-1-interacting partners identified phy-1, a prolyl-4-hydroxylase that is important for the modification of procollagens, which are secreted into the extracellular milieu, including basement membranes. The ten-1 also genetically interacts with collagen IV (let-2 in C. elegans); (Topf and Chiquet-Ehrismann, 2011). Collagen IV is required for the completion of embryonic development, tissue organization, and structural integrity. Collagen IV is produced in muscle cells, and insufficient maturation results in the intracellular retention and aggregation of procollagen. Consequently, the combined loss of phy-1 and ten-1 resulted in deteriorated connections between the epidermis and muscle tissue. Drosophila Ten-a protein also localizes to muscle attachment structures (Kenzelmann-Broz et al., 2010), and the mouse teneurin isoform TEN3 (Odz3) colocalizes with collagens I and II (Murakami et al., 2010). Epidermal defects in ten-1 and phy-1 double-mutant worms were accompanied by neuronal defects. Further evidence that TEN-1 is involved in neuronal guidance was provided by a candidate approach, in which defects in pharyngeal neurons were quantified, with a focus on M2 neurons (Morck et al., 2010). The loss of ten-1 together with genes that are involved in M2 cell body positioning and axon outgrowth resulted in more sever defects in M2 neuron. Among the interacting genes are sax-3 gene and downstream-acting unc-34 gene, which are involved in multiple aspects of sensory, motor, and interneuron axon guidance.
Genetic interaction data have provided strong evidence that teneurin in C. elegans is required for the maintenance of basement membrane integrity. Whether this function is based on structural tasks of teneurin that involve the binding of extracellular proteins or teneurin as a receptor that provides guidance for migrating cells remains to be determined. Nevertheless, this ancient function of TEN-1 may have served to organize and connect tissues, thus providing a foundation for development of the worm’s neuronal network.
Physical Interactions of Teneurins in Other Species
Recent biochemical and structural studies in vertebrate systems showed physical interactions between teneurins and other membrane receptors. Particularly interesting is the interaction between teneurin and latrophilin. Latrophilins (LPHN1-3) belong to the adhesion-type G-protein-coupled receptor (GPCR) family. LPHN1 was identified as a receptor for α-latrotoxin, a black widow spider toxin that triggers massive neurotransmitter release from neurons and neuroendocrine cells. In vertebrates, latrophilins interact with FLRTs (fibronectin leucine-rich repeat transmembrane proteins), UNC5 (netrin receptor), neurexins, and teneurins (Davletov et al., 1996; Krasnoperov et al., 1997). Latrophilin, UNC5, and FLRT form a super complex (Lu et al., 2015; Jackson et al., 2016). In neurons, latrophilin is presynaptic and teneurin is postsynaptic, and both proteins engage in trans interactions (Figure 1A). A recently published cryo-electron microscopy structure of human latrophilin 1 with teneurin 2 described this interaction in detail (Li et al., 2018).
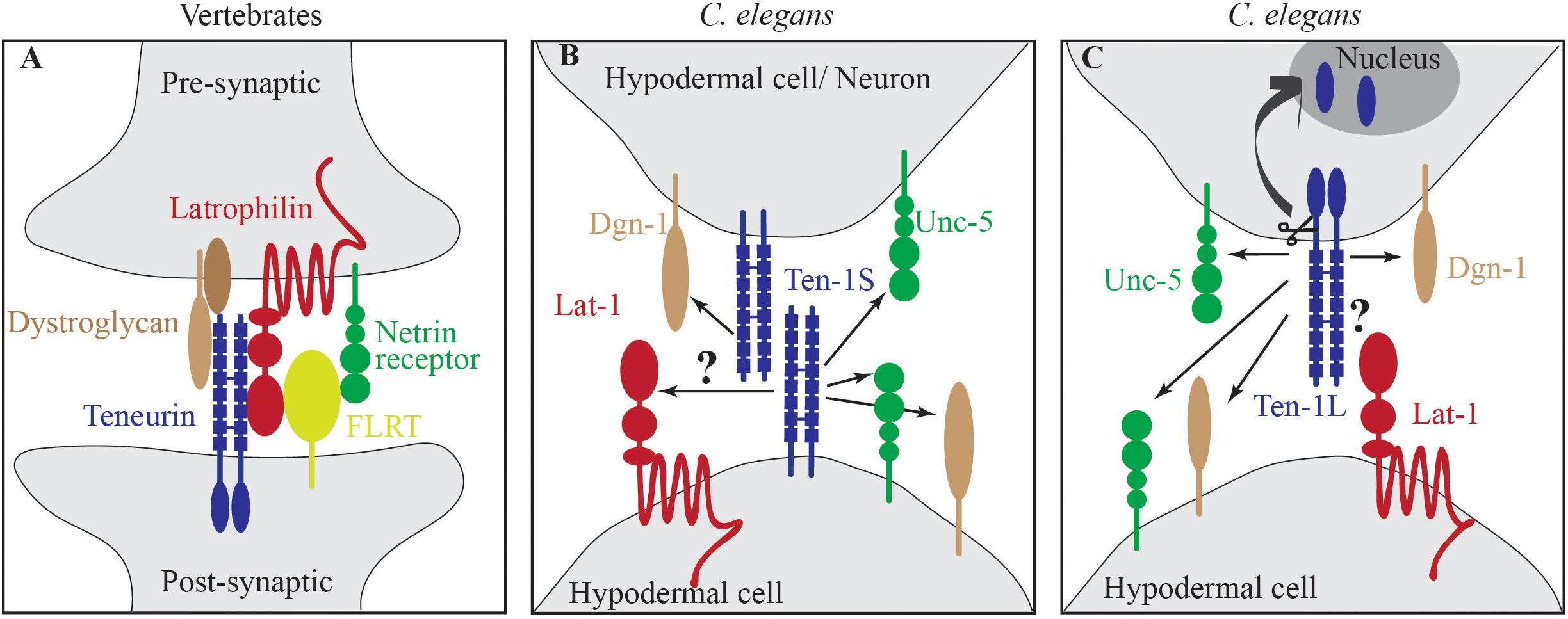
Figure 1. Teneurin-latrophilin interactions. (A) In vertebrates, teneurin is a part of protein complexes that connect pre- and postsynaptic parts of neurons. Teneurin physically interacts with latrophilin and also with the basement membrane receptor dystroglycan. Latrophilin is connected to the netrin receptor via FLRT. (B) C. elegans TEN-1S is expressed in hypodermal cells and neurons and genetically interacts with dystroglycan Dgn-1 and the netrin receptor Unc-5. The latrophilin LAT-1 is expressed in hypodermal cells. Whether TEN-1S and LAT-1 interact is unknown (arrow with question mark). (C) C. elegans TEN-1L is expressed in many more cells and tissues compared with LAT-1. Thus, cis interactions might be possible but have not yet been proven. A presumed interaction between TEN-1L and LAT-1 could trigger the release of the intracellular domain of TEN-1L, initiating cell signaling pathways. FLRT, (fibronectin leucine-rich repeat transmembrane protein), arrows indicate genetic interaction.
Ten-1 Interaction With Lat-1/Latrophilin in C. elegans
The C. elegans genome contains two latrophilin paralogs, lat-1 and lat-2 (Willson et al., 2004; Langenhan et al., 2009). The lat-1 is expressed in oocytes, early embryonic blastomeres, and precursors of pharyngeal and hypodermal cells. In larvae and adult worms, lat-1 is expressed in pharynx muscle nerve cells, the gonads, and the vulva. The neuronal and gonadal expression of lat-1 has only been mentioned and not thoroughly described (Langenhan et al., 2009). Lat-2 is expressed in the pharynx and gland cells of the excretory system. The lat-1 deletion is embryonically lethal, and the escapees have a smaller brood size because of defects in sperm development. The lat-2 deletion has no obvious phenotype but enhances the lat-1-null phenotype (Langenhan et al., 2009). LAT-1 in C. elegans has mostly been studied in the context of early embryogenesis, the alignment of mitotic spindle and division planes, and the establishment of anterior-posterior polarity. Comparing the expression of lat-1 and ten-1 is difficult because of insufficient descriptions of lat-1::GFP expression patterns. The expression pattern of lat-1 partially overlaps with ten-1a to a small extent in the developing pharynx. LAT-1 expression in dorsal hypodermis during intercalation partially overlaps with ten-1b promoter expressing Ten1S version of teneurin (Drabikowski et al., 2005).
In C. elegans, lat-1, and ten-1 genetically interact, but the physical interaction has not been demonstrated. In genetic interactions, the alleles displayed non-allelic non-complementation. The loss of any of the alleles of either gene led to developmental defects, and double-heterozygote worms exhibited strong defects in development and fertility (Promel et al., 2012). The authors showed that the ten-1a promoter is active in the same half of intercalating hypodermal cells as lat-1. Thus, according to these authors, LAT-1 is likely not a receptor for TEN-1L (Figure 1B). Interactions between latrophilin and teneurin in trans but not in cis have been proven biochemically, microscopically, and structurally in vertebrate systems (Davletov et al., 1996; Krasnoperov et al., 1997). Drabikowski et al. (2005) showed that ten-1b promotor-expressing TEN-1S and not ten-1a-expressing TEN-1L is expressed in the intercalating dorsal hypodermis in C. elegans in both the left and right rows of cells. The approach to obtain transgenic animals undertaken by Promel et al. (2012) expressing lat-1::GFP and by Morck et al. (2010) expressing ten-1a::GFP promoter fusions often result in random transgene silencing in a subset of cells. Kelly et al. (1997) have shown that simple, highly repetitive extrachromosomal arrays, as in this case used by Morck et al. (2010); Promel et al. (2012), result in transgene silencing. Thus, these expression patterns might reflect only partial expression pattern of LAT-1 and TEN-1 and conclusions drawn from them should be treated with caution. Regardless of whether lat-1 is expressed in all or only half of intercalating hypodermal cells, TEN-1S appears to be expressed in all intercalating hypodermal cells, thus indicating that in trans interactions between LAT-1 and TEN-1S are possible (Figure 1C). TEN-1S protein has a short, 36-amino-acid intracellular domain that does not translocate to the nucleus. Both proteins, TEN-1 and LAT-1, and the processes in which they are involved, are strongly conserved in evolution. Thus, it is highly unlikely that the nature of interactions, in trans or in cis, between these proteins would not be conserved. The elucidation of endogenous expression patterns of both LAT-1 and TEN-1 in C. elegans (e.g., by CRISPR/Cas9 technology) may help resolve these discrepancies.
In C. elegans, possible TEN-1 interactions with LAT-1 that are related to neuronal pathfinding and synapse formation await further investigation. In recent years, teneurin research in vertebrates has focused on neuronal function and interactions with latrophilin. Studies of early expression during mouse and chicken embryogenesis have shown that teneurins function not only in neuronal development but also in non-neuronal tissues during the pattern formation of developing limbs (Tucker et al., 2007), somites, and craniofacial mesenchyme (Tucker et al., 2001). Investigations of teneurins in non-neuronal tissues in vertebrates are still incipient but have already opened new avenues of research on both cancer and congenital diseases. Findings in worms may further guide such research.
Conclusion
Research on teneurin proteins has seen tremendous advances. Teneurins were discovered in 1993 in the labs of Ruth Chiquet-Ehrismann and of Roland Fässler. Since that time, however, the biological role of teneurins in humans has remained elusive. Several excellent studies have been performed in model organisms and cell culture systems, indicating that teneurins play a role as organizers of neuronal networks. Studies of teneurin in C. elegans have demonstrated its importance during development. The elucidation of multiple genetic interactions has shown that teneurin is essential for pattern formation, cell migration, and development of the nervous system. The ancestral function of teneurin in the nervous system in C. elegans is most pronounced through TEN-1 interactions and the maintenance of basement membrane and tissue integrity. In vertebrates, teneurin function evolved in concert with the multiplication of teneurin genes. Further investigations are required to establish the role of TEN-1 as an organizer of neuronal networks in C. elegans and the involvement of LAT-1 in these processes. State-of-the-art genetic tools in worms, combined with detailed descriptions of their development and neuronal connectivity at single-synapse resolution, make this a very promising area of research.
Author Contributions
UT and KD co-wrote the manuscript and reviewed the references. KD drafted Figure 1.
Funding
UT was supported by National Science Centre grant 2015/19/B/NZ1/03444.
Conflict of Interest Statement
The authors declare that the research was conducted in the absence of any commercial or financial relationships that could be construed as a potential conflict of interest.
References
Bagutti, C., Forro, G., Ferralli, J., Rubin, B., and Chiquet-Ehrismann, R. (2003). The intracellular domain of teneurin-2 has a nuclear function and represses zic-1-mediated transcription. J. Cell Sci. 116, 2957–2966. doi: 10.1242/jcs.00603
Baum, P. D., and Garriga, G. (1997). Neuronal migrations and axon fasciculation are disrupted in ina-1 integrin mutants. Neuron 19, 51–62. doi: 10.1016/S0896-6273(00)80347-5
Baumgartner, S., and Chiquet-Ehrismann, R. (1993). Tena, a Drosophila gene related to tenascin, shows selective transcript localization. Mech. Dev. 40, 165–176. doi: 10.1016/0925-4773(93)90074-8
Baumgartner, S., Martin, D., Hagios, C., and Chiquet-Ehrismann, R. (1994). Tenm, a drosophila gene related to tenascin, is a new pair-rule gene. EMBO J. 13, 3728–3740. doi: 10.1002/j.1460-2075.1994.tb06682.x
Beckmann, J., Schubert, R., Chiquet-Ehrismann, R., and Muller, D. J. (2013). Deciphering teneurin domains that facilitate cellular recognition, cell-cell adhesion, and neurite outgrowth using atomic force microscopy-based single-cell force spectroscopy. Nano Lett. 13, 2937–2946. doi: 10.1021/nl4013248
Byrne, A. B., Weirauch, M. T., Wong, V., Koeva, M., Dixon, S. J., Stuart, J. M., et al. (2007). A global analysis of genetic interactions in Caenorhabditis elegans. J. Biol. 6:8.
Chand, D., Song, L., Delannoy, L., Barsyte-Lovejoy, D., Ackloo, S., Boutros, P. C., et al. (2012). C-Terminal region of teneurin-1 co-localizes with dystroglycan and modulates cytoskeletal organization through an extracellular signal-regulated kinase-dependent stathmin- and filamin A-mediated mechanism in hippocampal cells. Neuroscience 219, 255–270. doi: 10.1016/j.neuroscience.2012.05.069
Dani, N., Zhu, H., and Broadie, K. (2014). Two protein N-acetylgalactosaminyl transferases regulate synaptic plasticity by activity-dependent regulation of integrin signaling. J. Neurosci. 34, 13047–13065. doi: 10.1523/JNEUROSCI.1484-14.2014
Davletov, B. A., Shamotienko, O. G., Lelianova, V. G., Grishin, E. V., and Ushkaryov, Y. A. (1996). Isolation and biochemical characterization of a Ca2+-independent alpha-latrotoxin-binding protein. J. Biol. Chem. 271, 23239–23245. doi: 10.1074/jbc.271.38.23239
Drabikowski, K., Trzebiatowska, A., and Chiquet-Ehrismann, R. (2005). ten-1, an essential gene for germ cell development, epidermal morphogenesis, gonad migration, and neuronal pathfinding in Caenorhabditis elegans. Dev. Biol. 282, 27–38. doi: 10.1016/j.ydbio.2005.02.017
Feng, K., Zhou, X. H., Oohashi, T., Morgelin, M., Lustig, A., Hirakawa, S., et al. (2002). All four members of the Ten-m/Odz family of transmembrane proteins form dimers. J. Biol. Chem. 277, 26128–26135. doi: 10.1074/jbc.M203722200
Glendining, K. A., Liu, S. C., Nguyen, M., Dharmaratne, N., Nagarajah, R., Iglesias, M. A., et al. (2017). Downstream mediators of Ten-m3 signalling in the developing visual pathway. BMC Neurosci. 18:78. doi: 10.1186/s12868-017-0397-5
Hong, W., Mosca, T. J., and Luo, L. (2012). Teneurins instruct synaptic partner matching in an olfactory map. Nature 484, 201–207. doi: 10.1038/nature10926
Jackson, V. A., Mehmood, S., Chavent, M., Roversi, P., Carrasquero, M., Del Toro, D., et al. (2016). Super-complexes of adhesion GPCRs and neural guidance receptors. Nat. Commun. 7:11184. doi: 10.1038/ncomms11184
Jackson, V. A., Meijer, D. H., Carrasquero, M., Van Bezouwen, L. S., Lowe, E. D., Kleanthous, C., et al. (2018). Structures of Teneurin adhesion receptors reveal an ancient fold for cell-cell interaction. Nat. Commun. 9:1079. doi: 10.1038/s41467-018-03460-0
Kang, S. H., and Kramer, J. M. (2000). Nidogen is nonessential and not required for normal type IV collagen localization in Caenorhabditis elegans. Mol. Biol. Cell 11, 3911–3923. doi: 10.1091/mbc.11.11.3911
Kelly, W. G., Xu, S., Montgomery, M. K., and Fire, A. (1997). Distinct requirements for somatic and germline expression of a generally expressed Caernorhabditis elegans gene. Genetics 146, 227–238.
Kenzelmann, D., Chiquet-Ehrismann, R., and Tucker, R. P. (2007). Teneurins, a transmembrane protein family involved in cell communication during neuronal development. Cell. Mol. Life Sci. 64, 1452–1456. doi: 10.1007/s00018-007-7108-9
Kenzelmann-Broz, D., Tucker, R. P., Leachman, N. T., and Chiquet-Ehrismann, R. (2010). The expression of teneurin-4 in the avian embryo: potential roles in patterning of the limb and nervous system. Int. J. Dev. Biol. 54, 1509–1516. doi: 10.1387/ijdb.103139dk
Kim, S., and Wadsworth, W. G. (2000). Positioning of longitudinal nerves in C. elegans by nidogen. Science 288, 150–154.
Krasnoperov, V. G., Bittner, M. A., Beavis, R., Kuang, Y., Salnikow, K. V., Chepurny, O. G., et al. (1997). alpha-Latrotoxin stimulates exocytosis by the interaction with a neuronal G-protein-coupled receptor. Neuron 18, 925–937. doi: 10.1016/S0896-6273(00)80332-3
Langenhan, T., Promel, S., Mestek, L., Esmaeili, B., Waller-Evans, H., Hennig, C., et al. (2009). Latrophilin signaling links anterior-posterior tissue polarity and oriented cell divisions in the C. elegans embryo. Dev. Cell 17, 494–504. doi: 10.1016/j.devcel.2009.08.008
Li, J., Shalev-Benami, M., Sando, R., Jiang, X., Kibrom, A., Wang, J., et al. (2018). Structural basis for teneurin function in circuit-wiring: a toxin motif at the synapse. Cell 173:e715. doi: 10.1016/j.cell.2018.03.036
Lu, Y. C., Nazarko, O. V., Sando, R. III, Salzman, G. S., Li, N. S., Sudhof, T. C., et al. (2015). Structural basis of latrophilin-FLRT-UNC5 Interaction in Cell Adhesion. Structure 23, 1678–1691. doi: 10.1016/j.str.2015.06.024
Minet, A. D., Rubin, B. P., Tucker, R. P., Baumgartner, S., and Chiquet-Ehrismann, R. (1999). Teneurin-1, a vertebrate homologue of the Drosophila pair-rule gene ten-m, is a neuronal protein with a novel type of heparin-binding domain. J. Cell Sci. 112(Pt 12), 2019–2032.
Morck, C., Vivekanand, V., Jafari, G., and Pilon, M. (2010). C. elegans ten-1 is synthetic lethal with mutations in cytoskeleton regulators, and enhances many axon guidance defective mutants. BMC Dev. Biol. 10:55. doi: 10.1186/1471-213X-10-55
Mosca, T. J. (2015). On the teneurin track: a new synaptic organization molecule emerges. Front. Cell. Neurosci. 9:204. doi: 10.3389/fncel.2015.00204
Mosca, T. J., Hong, W., Dani, V. S., Favaloro, V., and Luo, L. (2012). Trans-synaptic Teneurin signalling in neuromuscular synapse organization and target choice. Nature 484, 237–241. doi: 10.1038/nature10923
Murakami, T., Fukunaga, T., Takeshita, N., Hiratsuka, K., Abiko, Y., Yamashiro, T., et al. (2010). Expression of Ten-m/Odz3 in the fibrous layer of mandibular condylar cartilage during postnatal growth in mice. J. Anat. 217, 236–244. doi: 10.1111/j.1469-7580.2010.01267.x
Nunes, S. M., Ferralli, J., Choi, K., Brown-Luedi, M., Minet, A. D., and Chiquet-Ehrismann, R. (2005). The intracellular domain of teneurin-1 interacts with MBD1 and CAP/ponsin resulting in subcellular codistribution and translocation to the nuclear matrix. Exp. Cell Res. 305, 122–132. doi: 10.1016/j.yexcr.2004.12.020
Promel, S., Frickenhaus, M., Hughes, S., Mestek, L., Staunton, D., Woollard, A., et al. (2012). The GPS motif is a molecular switch for bimodal activities of adhesion class G protein-coupled receptors. Cell Rep. 2, 321–331. doi: 10.1016/j.celrep.2012.06.015
Rubin, B. P., Tucker, R. P., Brown-Luedi, M., Martin, D., and Chiquet-Ehrismann, R. (2002). Teneurin 2 is expressed by the neurons of the thalamofugal visual system in situ and promotes homophilic cell-cell adhesion in vitro. Development 129, 4697–4705.
Rubin, B. P., Tucker, R. P., Martin, D., and Chiquet-Ehrismann, R. (1999). Teneurins: a novel family of neuronal cell surface proteins in vertebrates, homologous to the Drosophila pair-rule gene product Ten-m. Dev. Biol. 216, 195–209. doi: 10.1006/dbio.1999.9503
Scholer, J., Ferralli, J., Thiry, S., and Chiquet-Ehrismann, R. (2015). The intracellular domain of teneurin-1 induces the activity of microphthalmia-associated transcription factor (MITF) by binding to transcriptional repressor HINT1. J. Biol. Chem. 290, 8154–8165. doi: 10.1074/jbc.M114.615922
Silva, J. P., Lelianova, V. G., Ermolyuk, Y. S., Vysokov, N., Hitchen, P. G., Berninghausen, O., et al. (2011). Latrophilin 1 and its endogenous ligand Lasso/teneurin-2 form a high-affinity transsynaptic receptor pair with signaling capabilities. Proc. Natl. Acad. Sci. U.S.A. 108, 12113–12118. doi: 10.1073/pnas.1019434108
Topf, U., and Chiquet-Ehrismann, R. (2011). Genetic interaction between Caenorhabditis elegans teneurin ten-1 and prolyl 4-hydroxylase phy-1 and their function in collagen IV-mediated basement membrane integrity during late elongation of the embryo. Mol. Biol. Cell 22, 3331–3343. doi: 10.1091/mbc.E10-10-0853
Trzebiatowska, A., Topf, U., Sauder, U., Drabikowski, K., and Chiquet-Ehrismann, R. (2008). Caenorhabditis elegans teneurin, ten-1, is required for gonadal and pharyngeal basement membrane integrity and acts redundantly with integrin ina-1 and dystroglycan dgn-1. Mol. Biol. Cell 19, 3898–3908. doi: 10.1091/mbc.E08-01-0028
Tucker, R. P. (2018). Teneurins: domain architecture, evolutionary origins, and patterns of expression. Front. Neurosci. 12:938. doi: 10.3389/fnins.2018.00938
Tucker, R. P., Beckmann, J., Leachman, N. T., Scholer, J., and Chiquet-Ehrismann, R. (2012). Phylogenetic analysis of the teneurins: conserved features and premetazoan ancestry. Mol. Biol. Evol. 29, 1019–1029. doi: 10.1093/molbev/msr271
Tucker, R. P., and Chiquet-Ehrismann, R. (2006). Teneurins: a conserved family of transmembrane proteins involved in intercellular signaling during development. Dev. Biol. 290, 237–245. doi: 10.1016/j.ydbio.2005.11.038
Tucker, R. P., Chiquet-Ehrismann, R., Chevron, M. P., Martin, D., Hall, R. J., and Rubin, B. P. (2001). Teneurin-2 is expressed in tissues that regulate limb and somite pattern formation and is induced in vitro and in situ by FGF8. Dev. Dyn. 220, 27–39. doi: 10.1002/1097-0177(2000)9999:9999<::AID-DVDY1084>3.0.CO;2-B
Tucker, R. P., Kenzelmann, D., Trzebiatowska, A., and Chiquet-Ehrismann, R. (2007). Teneurins: transmembrane proteins with fundamental roles in development. Int. J. Biochem. Cell Biol. 39, 292–297. doi: 10.1016/j.biocel.2006.09.012
Keywords: teneurin, TEN-1, C. elegans, basement membrane, latrophilin, LAT-1, axon guidance
Citation: Topf U and Drabikowski K (2019) Ancient Function of Teneurins in Tissue Organization and Neuronal Guidance in the Nematode Caenorhabditis elegans. Front. Neurosci. 13:205. doi: 10.3389/fnins.2019.00205
Received: 22 December 2018; Accepted: 22 February 2019;
Published: 08 March 2019.
Edited by:
Antony Jr. Boucard, Centro de Investigación y de Estudios Avanzados (CINVESTAV), MexicoReviewed by:
Andrew Chisholm, University of California, San Diego, United StatesTobias Langenhan, Leipzig University, Germany
Copyright © 2019 Topf and Drabikowski. This is an open-access article distributed under the terms of the Creative Commons Attribution License (CC BY). The use, distribution or reproduction in other forums is permitted, provided the original author(s) and the copyright owner(s) are credited and that the original publication in this journal is cited, in accordance with accepted academic practice. No use, distribution or reproduction is permitted which does not comply with these terms.
*Correspondence: Krzysztof Drabikowski, ZHJhYmlrb3dza2lAaWJiLndhdy5wbA==